- 1Department of Geosciences, The Pennsylvania State University, University Park, PA, United States
- 2Department of Geological Sciences, University of Colorado, Boulder, CO, United States
Carbonates are ubiquitous in the rock record and provide a broad array of stable isotope-based paleoclimatic proxies (i.e., δ18O, δ13C, ∆17O, ∆47, ∆48) that provide information on stratigraphy, carbon cycling, temperature, hydrology, and the altitude of ancient land surfaces. Thus, carbonates are an essential archive of environmental and topographic histories of continental terranes. However, carbonate minerals are highly susceptible to post-depositional alteration of primary isotopic values via fluid-mediated and solid-state reactions. We propose a hierarchical suite of techniques to comprehensively assess alteration in carbonates, from essential and readily accessible tools to novel, high-resolution techniques. This framework provides a means of identifying preserved textures in differentially altered samples that contain high-value environmental information. To illustrate this progressive approach, we present a case study of Tethyan nearshore carbonates from the Paleocene Tso Jianding Group (Tibet). We demonstrate the utility of each technique in identifying chemical and crystallographic indicators of post-depositional alteration at progressively finer spatial scales. For example, secondary ionization mass spectrometry (SIMS) oxygen isotope maps of micrite and bioclasts reveal significant isotopic heterogeneity due to grain-scale water-rock exchange in textures that were labeled “primary” by optical inspection at coarser spatial resolution. Optical and cathodoluminescence microscopy should be the minimum required assessment of carbonate samples used in stable isotope analyses, but supplemented when necessary by SIMS, PIC mapping, and other yet untapped technologies that may allow distinction of primary and altered fabrics at finer spatial resolutions.
Introduction
Carbonate rocks provide an archive of geochemical information related to the climatic and tectonic setting of their depositional environment. In particular, oxygen isotopes of terrestrial carbonates (δ18Oc) have been used as a proxy for ancient rainwater (δ18Ow) in the field of stable isotope paleoaltimetry. The relationship between altitude and meteoric water composition is defined by the orogressive distillation of the heavy isotopes of water (18O, D) as a moisture source rains out over mountains (∆δ18Ow = δ18Ow,sample altitude-δ18Ow,sea level; Chamberlain and Poage, 2000; Rowley et al., 2001). This approach has enabled reconstructions of the tectonic evolution of the Andes, western North American Cordillera, Tibetan Plateau and other continental terranes (e.g., Rowley and Currie, 2006; Garzione, 2008; Chamberlain et al., 2012). Clumped isotope thermometry (T (∆47)) advanced the use of carbonates for stable isotope paleoaltimetry by 1) facilitating direct calculations of δ18Ow from primary δ18Oc values, which reduces uncertainties on traditional δ18O paleoaltimetry estimates by accounting for mineral formation temperature (e.g., Snell et al., 2014; Huntington et al., 2015; Ingalls et al., 2017; Ingalls et al., 2020), and 2) providing temperatures that can be used with lapse rates to provide an additional estimate of elevation that is independent of ancient precipitation isotopes (e.g., Huntington et al., 2010).
The paradox of carbonate stable isotope paleoaltimetry is the need for preservation of primary isotopic signatures in rocks that experienced the complex burial and thermal histories associated with regions of active tectonics. Carbonate minerals are highly susceptible to both water-rock and solid-state isotopic exchange during burial (e.g., Banner and Hanson, 1990; Passey and Henkes, 2012). This susceptibility led Quade et al. (2020) to question whether burial alteration reactions, driven by the very tectonic activity stable isotope paleoaltimetry intends to reconstruct, erases the original elevation signal in the proxy record. Samples that have been inferred to retain primary information based on minimal assessment of diagenetic histories have been used to reconstruct paleoaltitudes. Conversely, entire stratigraphic packages are dismissed as problematic recorders of primary environments when alteration is suspected based on burial and thermal histories (e.g., stratigraphic thickness, vitrinite reflectance, thermochronology) or alteration fabrics. To enable reconstructions of previously unsolvable tectonic histories, we sought to answer the questions: can analyses at high spatial resolution identify zones of preserved primary carbonate within high-value samples that have been previously classified as altered? Are there finer-scale textural or geochemical indicators of isotopic exchange in the absence of classic alteration fabrics at coarse resolution?
Here, we present a tool kit to guide rigorous assessment of alteration in carbonates at cm-to µm-scales. We intend for this hierarchical framework to improve stable isotope paleoaltimetry through critical assessment of the signs of diagenetic alteration at a range of spatial resolutions. We review textures and chemical signatures indicative of primary, early diagenesis, and burial alteration identifiable by standard optical petrography and cathodoluminescence (CL) microscopy. We suggest complementary analyses commonly employed in diagenesis research, e.g., electron probe microanalyses (EPMA) and scanning electron microscopy electron backscatter diffraction (SEM-EBSD). These techniques provide chemical and crystallographic indicators of post-depositional alteration at <20 µm resolution. Additionally, we propose the use of novel analytical techniques with order of magnitude higher spatial resolution (e.g., secondary ionization mass spectrometry [SIMS] and polarization-dependent imaging contrast [PIC] mapping) to identify textural and chemical alteration that can occur at a finer scale than achievable by standard optical techniques. If primary domains identified by these high-resolution techniques exceed the size of a drill bit, primary isotopic values from key samples previously dismissed as altered by coarser optical techniques could now be attainable. More rigorous and comprehensive assessment of isotopic alteration should ultimately improve and expand our carbonate records of ancient climate and tectonics, and improve our understanding of carbonate alteration mechanisms. Finally, to provide an example of the utility of the analytical framework presented herein, we apply the framework to Tethyan shallow marine carbonates from the Paleocene Jialazi Formation of southern Tibet to characterize the extent of textural and chemical alteration.
Hierarchical Framework for Alteration Assessment Techniques
The assessment techniques are categorized by spatial resolution, which generally coincides with ease of access and cost (Table 1). Category one techniques should be applied in all carbonate proxy research, whereas Category three techniques, which are costlier and/or available at a limited number of institutions, are meant to be reserved for assessing and obtaining high-value geochemical information not available by other means. Our intention is to provide a brief overview of the techniques that illustrates their primary utility and caveats for assessing diagenesis, and to show how they can be used hierarchically to holistically. Further information about the techniques can be found in the Supplement and in the references herein.
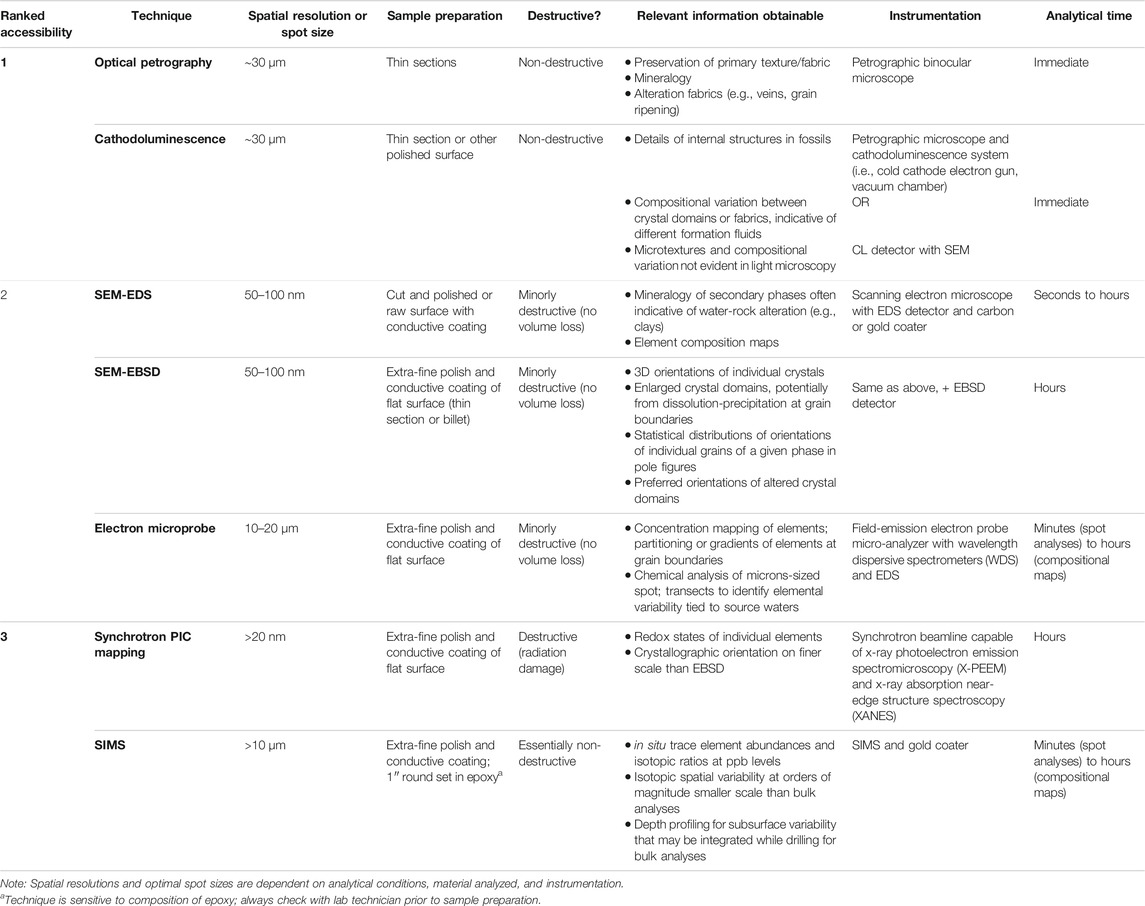
TABLE 1. A toolbox of optical and microanalytical techniques for studying the alteration history of a carbonate sample. Spatial resolutions and optimal spot sizes are dependent on the analytical conditions, materials analyzed, and instrumentation, but commonly accepted resolutions are given. Examples of necessary instrumentation are given, although there are often multiple options from different companies for a given instrument. Similarly, we present relative data acquisition time for one datum, but this will vary significantly depending on whether the analysis is a single point or a compositional map, and with user-determined measurement resolution. The techniques are categorized by increasing spatial resolution, which typically coincides with decreasing accessibility and increasing fees.
It is important to note that terrestrial carbonates are typically more variable geochemically than marine carbonates, and are generally less well characterized than marine carbonates. To maximize the ability to interpret data from any of the techniques mentioned below, it will be helpful to measure modern analogs of specific terrestrial carbonates or biogenic material to establish an informative baseline for a given sample.
Category 1—mm- to µm-Scale
Optical Petrography
Optical microscopy of carbonate thin sections is the most essential and accessible method for qualitative assessment of alteration. With plane polar (PPL) and cross polarized light (XPL), one can identify mineralogy, crystal domains and habit, and textures that can be associated with primary formation or water-rock alteration. Some features that may indicate water-rock alteration are secondary porosity, microfractures, meteoric and vadose zone blocky or meniscus cements, pressure dissolution stylolites, dolomitization and microspar or spar-filled veins crosscutting the host rock (Wanless, 1979; Buxton and Sibley, 1981; Moore, 1989; Budd and Land, 1990; Budd et al., 2002; Moore, 2002).
Optical identification of primary domains allows for fabric-specific subsampling from the corresponding slab for geochemical analyses (Table 1). In marine or lacustrine limestones, a dense micritic fabric is often considered a primary fabric, and commonly preferred in stable isotope studies. However, micrite can also represent diagenesis via micritization. Biogenic carbonates (e.g., shells, foraminifera tests) have clade-specific growth habits that are typically optically identifiable, which makes bioclasts a good starting point in assessing water-rock alteration of fossiliferous samples. However, some alteration is fabric retentive, and thus, during mineral replacement and isotopic exchange, the crystal domain can retain its internal structure (e.g. fabric-retentive dolomitization). Further, some materials are more reticent to water-rock exchange (e.g., brachiopods; Grossman et al., 1996). Thus, careful fabric-specific sampling combined with isotopic analyses of the different fabrics in a sample can aid in discerning altered vs. primary values.
Optical Cathodoluminescence
Cold-cathode optical-CL allows one to pair textural information to geochemical information inaccessible via light microscopy alone. Luminescence is the response of a material to bombardment of high energy electrons generated by the discharge between a negative high voltage cathode and an anode at ground potential in ionized gas under vacuum (∼10−2 Torr), and requires only a well-polished surface. Impurity ions and lattice defects are common in carbonate minerals (Nielsen et al., 2013) and act as electron traps, resulting in increased CL (Boggs and Krinsley, 2006). Trace metals can be incorporated into carbonate by sorption onto growing crystal surfaces, occlusion into lattice defects, substitution for a major element, and as impurity ions (Sun and Hanson, 1975). Mn2+ is an “activator” that increases CL emission and appears yellow to orange-red, whereas Fe2+ in the presence of Mn2+ is a “quencher” resulting in dull to non-luminescence (Gorobets and Walker, 1995). CL has long been a popular tool for identifying carbonate components least altered by recrystallization (dull to non-luminescence; e.g., Lohmann and Walker, 1989), and overprinting of primary chemical zonation within calcite crystals (mottled or blotchy luminescence; Budd et al., 2002; Machel, 2000). An additional benefit of optical-CL is the ability to directly compare CL to PPL and XPL images of the same crystal domains in a thin section (Table 1). Observations of larger, geochemically distinct domains of dull luminescence, such as within a shell or micrite, would signal an ideal location for microdrilling or further investigation by microbeam techniques (e.g., Snell et al., 2013).
Category 2—µm-to 100s of nm-scale
Scanning Electron Microscopy–Secondary Electron and Energy Dispersive X-Ray Spectroscopy (SEM—SE and -EDS)
The interactions between carbonate surfaces and a focused electron beam reveal chemical and morphological information by detection of secondary electrons (SE) and energy dispersive x-ray spectroscopy (EDS). A benefit of SEM imaging is the achievement of up to 30,000x magnification with 50–100 nm spatial resolution (Table 1). Chemical point analyses and maps enable qualitative identification of carbonate alteration, such as changes in elemental distribution in multiphase systems, phase replacement or neomorphism, and high-resolution characterization of chemical relationships at grain boundaries tied to the high-resolution textural information provided by the SEM images themselves (Figure 1).
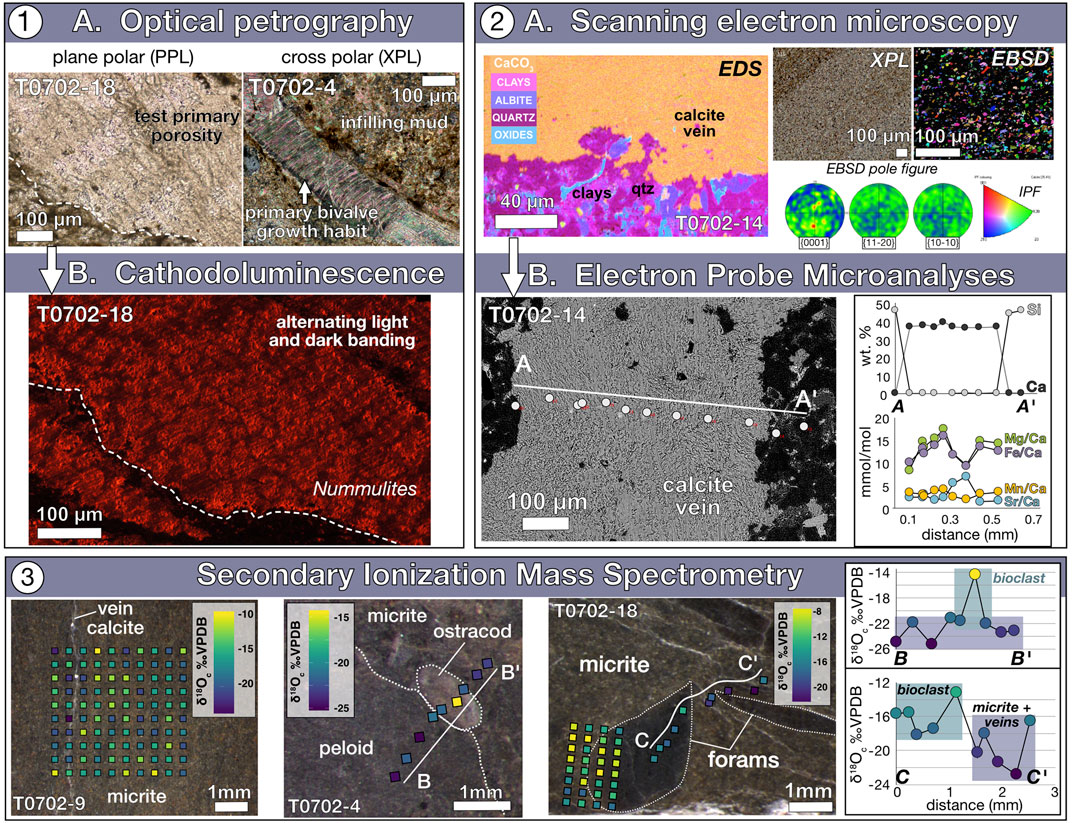
FIGURE 1. Optical and geochemical investigations of carbonate alteration increasing in spatial resolution (1→3). (1) Preservation of primary porosity in a foram test (T0702-18) in plane polar light; secondary carbonate precipitation within the test microporosity can be identified by the brighter red luminescence, indicative of elemental chemistry different from the water in which the foram grew. The test walls preserve alternating light and dark banding in CL, although it is patchier than is likely for the original fabric. Preserved primary bivalve growth habit (T0702-4) can be identified in cross polar light. (2) Scanning electron microscopy (SEM) energy dispersive spectroscopy (EDS) of a calcite vein cutting through a mudstone (T0702-14) displays phase mixing on the 10s of microns scale. Electron probe microanalysis (EPMA) shows elevated Sr/Ca at the core of the same vein (T0702-14), potentially indicative of two episodes of water-rock alteration. A sample from the Lunpola basin analyzed by EBSD demonstrates the expected no preferred c-axis orientation for a primary lacustrine limestone composed of micrite. IPF = inverse pole figure. (3) On the finest scale, ∼10 to 15‰ variability in carbonate δ18O values are seen within limestones (T0702-9), and across abiotic and biotic carbonate components (T0702-4 and -18). Generally, micrite and allochems yielded lower δ18O values than bioclasts. Overall, in this study, bioclasts were more fabric retentive and less divergent from expected values of marine carbonate than other carbonate components.
SEM–Electron Backscatter Diffraction (EBSD)
SEM electron beams can be diffracted by atomic layers within a carbonate crystal to generate visible backscatter patterns as they pass through a phosphor screen. The backscatter patterns are projections of the lattice plane geometry of individual carbonate crystals. These data are used to generate misorientation maps and pole figures displaying statistical distributions of crystallographic c-axis orientations of all individual grains of a given phase (Figure 1), superimposed on the SE and/or EDS images. The statistical data can be used to test alteration hypotheses and reconstruct the history of crystal reorientation related to water-rock alteration, including processes like grain ripening, calcite zonation, and overprinting of a unified c-axis orientation during reprecipitation. For example, micrite in a lacustrine limestone from the Lunpola basin had no preferred c-axis orientation, as seen in the pole figure in Figure 1. Following EBSD and optical petrographic observations, Ingalls et al. (2020) interpreted that this sample likely preserved primary fabrics and isotope values, since alteration would likely produce greater alignment of c-axis orientations within the micrite (e.g., Ryb et al., 2017).
Electron Probe Microanalysis (EPMA)
EPMA is a non-destructive tool that operates by the same principles as SEM, but is capable of quantitative, spatially resolved elemental analyses by wavelength dispersive spectroscopy (WDS). These 2D element maps can be overlaid on SE, BSE, and CL images to directly compare chemical variability between textural, mineralogical, crystallographic, and redox domains. Element maps and <10 µm spot analyses can reveal micron-scale chemical variability (Table 1), thereby serving as a test for subsurface water-rock interaction that may occur from pore fluids at a fine spatial scale. Quantitative analyses of major, minor and trace element ratios (e.g., Mg/Ca, Sr/Ca, Mn/Sr) provide evidence for carbonate formation from a different fluid composition than primary Earth surface water (Figure 1).
Category 3—µm-to 10s of nm-scale
Secondary Ionization Mass Spectrometry (SIMS)
SIMS and nanoSIMS provide one of the highest spatial resolution, in situ isotopic and elemental measurements currently available. SIMS provides oxygen and carbon isotope values at <20 µm scale (Table 1), similar to the spatial resolution of EPMA, while nanoSIMS can provide geochemical information on spots as small as 10s of nanometers. A primary positive (133Cs+) or negative (16O2-) ion beam focused on a sample surface generates secondary ions of specific elements that are measured by a mass spectrometer. SIMS analyses enable isotopic differentiation between microtextures and observation of isotopic gradients over areas that are integrated during bulk sample drilling. SIMS could open doors to characterizing extent of water-rock alteration and paragenesis from pore fluids, as well as potentially revealing residual primary carbonate material in differentially altered samples.
Polarization-dependent Imaging Contrast (PIC) Mapping
PIC mapping is an outgrowth of X-ray absorption near-edge structure (XANES) spectroscopy, in which spectra vary depending on the orientation of a carbonate mineral’s c-axis due to X-ray linear dichroism (Gilbert, 2012; DeVol et al., 2014). Crystallographic orientations can be quantified for domains >20 nm (DeVol et al., 2014), which is why this technique is commonly applied to biogenic carbonates to investigate biomineralization. Different modes of alteration result in different distributions of reoriented c-axes, which can then be explicitly tied to geochemical data from nanoSIMS on a comparable spatial scale. This is analogous to pairing EBSD-derived maps with SIMS on even finer spatial scales. A recent application of PIC mapping revealed reorientations of c-axes in individual nacre within fossil values, which demonstrated that altered biogenic aragonite can preserve original biomineral structures and mineralogy (Gilbert et al., 2017). This type of alteration would not be detectable at the scale of optical petrography, which could result in isotope values from altered material being used erroneously to reconstruct paleoenvironmental conditions. In the future, nanoSIMS analyses could reveal whether geochemical changes correspond to this nacre-scale alteration.
Modeling Potential Extent of Solid-State Bond Reordering in Clumped Isotope applications
At high temperatures, atoms can migrate within the carbonate lattice by solid-state diffusion, thereby randomizing the distribution of 13C–18O pairs and altering ∆47, and in turn, the inferred formation temperature (Passey and Henkes, 2012; Stolper and Eiler, 2015; Henkes et al., 2018). Importantly, solid-state alteration retains primary fabrics and bulk δ13C and δ18O while reducing ∆47 via diffusion. Samples experiencing solid-state alteration would pass all previously described assessments of textural and isotopic alteration, making it difficult to identify. Thus, it is critical to sufficiently rule out effects of fluid-based alteration before inferring that solid-state reordering is responsible for erroneous ∆47 temperatures.
Fluid-mediated recrystallization also resets T (∆47) to the recrystallization temperature. However, the extent of ∆47 alteration by solid-state 13C–18O reordering is dependent on the integrated time above a mineral-specific threshold. For example, calcite held at 75°C for 100 My would only increase T (∆47) by < 1 °C, but would increase T (∆47) by ∼40 °C if held at 120 °C for 100 My (Stolper and Eiler, 2015). Independent constraints on the burial histories of sedimentary basins and application of mineral-specific kinetic parameters in reordering models provide means for assessing the potential extent of solid-state reordering (e.g., Lawson et al., 2018; Lloyd et al., 2018; Lacroix and Niemi, 2019), for samples that show no other signs of fluid-based alteration that could account for erroneous ∆47 values.
Case Study: Differential Alteration of Tethyan Shallow Marine carbonate
The shallow marine Paleocene-Eocene Jialazi Formation of the Tso Jianding Group (southern Tibet) is ideal for investigating the expression of water-rock and solid-state alteration in micritic and biogenic carbonate at a variety of spatial scales because of the incongruity of its isotope values and textural characteristics. The Jialazi Formation records the transition from marine to terrestrial deposition within the Xigaze forearc basin following the India-Asia collision (Orme et al., 2014; Orme, 2017). Currently, no low-altitude δ18Ow or δDw estimate of precipitation south of Tibet exists for Paleogene ∆δ18Ow-based reconstructions during a critical period of Tibetan tectonics. Ingalls (2019) aimed to provide a low-altitude δDw from terrestrially derived organics preserved within the nearshore sediments if effects of alteration could be more concretely assessed.
Ingalls (2019) found that textural and isotopic data presented contradictory cases for the preservation of primary information in the Jialazi Formation. While primary biofabrics of shells and foraminifera were identified by traditional optical techniques (Figure 1), δ18Oc values were ∼10–20‰ lower than any primary marine carbonate, and similar to carbonates that precipitate from Tibetan meteoric water today (Ingalls, 2019). Specifically, optical petrography showed preserved primary foraminifera test porosity in plane polarized light and primary bivalve growth habit with banding perpendicular to growth laminae crossed polars (T0702–4; Figure 1). Contrary to these indicators of textural preservation, test edges appeared to be partially dissolved or weathered and pores were infilled with cements with increased luminescence (T0702–18; Figure 1; Supplementary Figure S1). The dull luminescence of the tests and micritic matrix suggested those domains may preserve geochemical information of the depositional environment, but the red luminescence within pore spaces indicated carbonate precipitation from a fluid with different redox chemistry. Subsampling material from the Nummulites tests without integrating altered pore-filling carbonate is beyond the spatial capability of a micromill, so traditional isotope analysis would likely integrate primary and altered material.
Instead, using EPMA, we identified secondary carbonate formed from two generations of alteration fluids in sample T0702–14. Across a ∼500 µm transect with 12 elemental spot analyses (10–20 µm diameter; Figure 1), we identified two populations: 1) a primary trend of increasing Mg/Ca and Fe/Ca away from the vein-mudstone interface on both sides, and 2) consistently low Mn/Ca and Sr/Ca that later overprinted via dissolution-precipitation. This is seen in a spike in Sr/Ca paired with a relative decrease in Mg/Ca and Fe/Ca along the central axis of the vein. We suspect that interaction with a subsurface brine caused Sr/Ca enrichment in the second alteration event because saline burial fluids typically carry significantly higher Sr concentrations than seawater (Banner, 1995).
The clumped isotope-derived temperatures ranged from ∼80 ° to 10 °C (Ingalls, 2019), reflecting the complex water-rock and solid-state alteration history. Ingalls (2019) assessed the potential extent of solid-state reordering using thermochronologic data from the Xigaze forearc (Orme, 2017) in an exchange-diffusion model (Lloyd, 2020) partnered with fabric-specific analyses. The model results suggest that late-stage, low-temperature, fluid-based alteration superseded partial solid-state reordering in the Jialazi Formation, overprinting the deep burial history with low T (∆47) values. Perhaps most importantly for paleoenvironmental reconstruction, the extent to which δ18O values departed from original marine compositions varied in the Jialazi Formation (e.g., δ18Oc values of ∼ –10 to –25‰ VPDB; Figure 1). Additionally, transects in samples T0702-4 and -18 revealed a trend of higher δ18Oc values (∼−12 to −18‰) in bioclasts than in micrite (>−26‰; Figure 1). Although the Jialazi Formation as a whole was altered beyond use for palaeoenvironmental reconstruction, we determined that biogenic materials were either more reticent to water-rock exchange than micritic carbonate or were altered by a different fluid than the micrite. SIMS analyses of micrite, bioclasts and diagenetic cements in the Paris Basin yielded similar magnitude δ18O variability (Vincent et al., 2017), demonstrating that intrasample geochemical variability on a finer scale than can be resolved by micromilling is not unique to the Jialazi Formation. Thermal maturation indices of organic matter and benchmark values for marine carbonate δ18O (∼0‰ VPDB) clearly identified these rocks as strongly impacted by isotopic exchange reactions. Had these samples been derived from a terrestrial environment whose expected values could have been similar to the values obtained, and only screened by optical petrography, the altered δ18O values might have been used erroneously to make stable isotope-based calculations of ancient elevations.
To demonstrate the magnitude of error that can arise from misinterpretation of altered δ18O values, we performed ∆(δ18Ow)-based calculations using the endmember Jialazi Fm δ18Ow values for sea level estimates (−19.1, −12.8‰ VSMOW, noting that seawater δ18Ow, recorded by marine carbonate, is typically higher than meteoric δ18Ow), and both Paleocene (−13.6‰; Ingalls et al., 2017) and modern compositions (−18.1‰; Bershaw et al., 2012) from the high-altitude margin of Tibet (see Supplement). Using Jialazi Fm δ18Ow values as the sea level proxy, we calculated Paleocene elevations of −5,288 and 548 m. a.s.l. for the southern Tibetan margin, with the former being physically implausible (Supplementary Table S1). Differencing Jialazi Fm values with modern precipitation yielded elevations of –755 and 2,874 m. a.s.l. for a land surface currently >4,000 m. a.s.l. Therefore, incorrectly using altered δ18O values as an estimate for low elevation meteoric water in paleoaltimetry calculations can underestimate elevations by several kilometers (Supplementary Table S1). By the same logic, alteration of high-altitude samples can result in inaccurate paleo-elevations by similar magnitudes.
Summary
We present a hierarchical framework for assessing alteration in carbonates used for environmental and tectonic reconstructions. As an example, we built on the exchange-diffusion modeling and texture-specific isotopic measurements of Ingalls (2019) to construct a more detailed alteration history for the Jialazi Formation. We demonstrated that apparent textural preservation can coincide with differential alteration of primary isotopic values, which can lead to significant errors in paleoaltimetric reconstructions. Had the Jialazi Formation been nonmarine, the application of the full suite of screening techniques and consideration of thermal history would have been essential to ruling out the use of δ18O and ∆47 for paleoaltimetry. The adoption of this hierarchical framework for assessing carbonate alteration could improve our understanding of the carbonate record and processes of carbonate isotopic alteration, as well as resolve previously unsolvable tectonic problems by enabling access to finite domains of preserved primary carbonate.
Data Availability Statement
The datasets presented in this study can be found in online repositories. The names of the repository/repositories and accession numbers can be found below: https://osf.io/34k8y doi: 10.17605/OSF.IO/34K8Y.
Author Contributions
MI conceived of the hierarchical assessment framework, performed analyses, interpreted data and wrote the manuscript. KS contributed to the conception of the alteration framework, and contributed writing and feedback on the manuscript.
Funding
This work was funded by NSF EAR 2040145 to MI and KS, and funding to KS from the University of Colorado.
Conflict of Interest
The authors declare that the research was conducted in the absence of any commercial or financial relationships that could be construed as a potential conflict of interest.
Acknowledgments
MI thanks Chi Ma and Yunnan Guan for assistance with SEM and SIMS microanalyses at the California Institute of Technology, Julien Allaz for assistance with EPMA at the University of Colorado, and David Budd for discussions of primary and diagenetic fabrics in large foraminifera. We thank the editors for the invitation to contribute to Reaching New Heights: Recent Progress in Paleotopography, and constructive comments from three reviewers.
Supplementary Material
The Supplementary Material for this article can be found online at: https://www.frontiersin.org/articles/10.3389/feart.2021.623982/full#supplementary-material.
References
Banner, J. L. (1995). Application of the trace element and isotope geochemistry of strontium to studies of carbonate diagenesis. Sedimentology 42, 805–824. doi:10.1111/j.1365-3091.1995.tb00410.x
Banner, J. L., and Hanson, G. N. (1990). Calculation of simultaneous isotopic and trace element variations during water-rock interaction with applications to carbonate diagenesis. Geochem. Cosmochim. Acta. 54, 3123–3137. doi:10.1016/0016-7037(90)90128-8
Bershaw, J., Penny, S., and Garzione, C. N. (2012). Stable isotopes of modern water across the Himalaya and eastern Tibetan Plateau: implications for estimates of paleoelevation and paleoclimatic. J. Geophys. Res. 117, D02110. doi:10.1029/2011JD016132
Boggs, S., and Krinsley, D. (2006). Application of cathodoluminescence imaging to the study of sedimentary rocks. Cambridge University Press.
Budd, D. A., and Land, L. S. (1990). Geochemical imprint of meteoric diagenesis in holocene ooid sands, schooner cays, bahamas: correlation of calcite cement geochemistry with extant groundwaters. J. Sediment. Petrol. 60, 361–378. doi:10.1007/s00204-003-0469-4
Budd, D. A., Pack, S. M., and Fogel, M. L. (2002). The destruction of paleoclimatic isotopic signals in Pleistocene carbonate soil nodules of Western Australia. Palaeogeogr. Palaeoclimatol. Palaeoecol. 188, 249–273.
Buxton, T. M., and Sibley, D. F. (1981). Pressure solution features in a shallow buried limestone. J. Sediment. Res. 51, 19–26. doi:10.1306/212F7BF8-2B24-11D7-8648000102C1865D
Chamberlain, C. P., Mix, H. T., Mulch, A., Hren, M. T., Kent-Corson, M. L., Davis, S. J., et al. (2012). The Cenozoic climatic and topographic evolution of the western North American cordillera. Am. J. Sci. 312, 213–262. doi:10.2475/02.2012.05
Chamberlain, C. P., and Poage, M. A. (2000). Reconstructing the paleotopography of mountain belts from the isotopic composition of authigenic minerals. Geology. 28, 115–118. doi:10.1130/0091-7613(2000)28<115:RTPOMB>2.0.CO;2
DeVol, R. T., Metzler, R. A., Kabalah-Amitai, L., Pokroy, B., Politi, Y., Gal, A., et al. (2014). Oxygen spectroscopy and polarization-dependent imaging contrast (PIC)- mapping of calcium carbonate minerals and biominerals. J. Phys. Chem. B. 118, 8449–8457. doi:10.1021/jp503700g
Gilbert, C. N. (2008). Surface uplift of Tibet and Cenozoic global cooling. Geology. 36, 1003–1004. doi:10.1130/focus122008.1
Gilbert, P. U. P. A., Bergmann, K. D., Myers, C. E., Marcus, M. A., DeVol, R. T., Sun, C.-Y., et al. (2017). Nacre tablet thickness records formation temperature in modern and fossil shells. Earth Planet Sci. Lett. 460, 281–292. doi:10.1016/j.epsl.2016.11.012
Gilbert, P. U. P. A. (2012). Polarization-dependent imaging contrast (PIC) mapping reveals nanocrystal orientation patterns in carbonate biominerals. J. Electron. Spectrosc. Relat. Phenom. 185, 395–405. doi:10.1016/j.elspec.2012.06.001
Gorobets, B. S., and Walker, G. (1995). “Origins of luminescence in minerals: a summary of fundamental studies and applications,” in Advanced mineralogy. Berlin: Springer-Verlag, 124–135.
Grossman, E. L., Mii, H.-S., Zhang, C. L., and Yancey, T. E. (1996). Chemical variation in pennsylvanian brachiopod shells; diagenetic, taxonomic, microstructural, and seasonal effects. J. Sediment. Res. 66, 1011–1022. doi:10.1306/D4268469-2B26-11D7-8648000102C1865D
Henkes, G. A., Passey, B. H., Grossman, E. L., Shenton, B. J., Yancey, T. E., and Pérez-Huerta, A. (2018). Temperature evolution and the oxygen isotope composition of phanerozoic oceans from carbonate clumped isotope thermometry. Earth Planet Sci. Lett. 490, 40–50. doi:10.1016/j.epsl.2018.02.001
Huntington, K. W., Saylor, J., Quade, J., and Hudson, A. M. (2015). High late Miocene-Pliocene elevation of the Zhada Basin, southwestern Tibetan Plateau, from carbonate clumped isotope thermometry. Geol. Soc. Am. Bull. 127, 181–199. doi:10.1130/B31000.1
Huntington, K. W., Wernicke, B. P., and Eiler, J. M. (2010). Influence of climate change and uplift on colorado plateau paleotemperatures from carbonate clumped isotope thermometry. Tectonics 29, TC3005. doi:10.1029/2009TC002449
Ingalls, M. (2019). Reconstructing carbonate alteration histories in orogenic sedimentary basins: Xigaze forearc, southern Tibet. Geochem. Cosmochim. Acta. 251, 284–300. doi:10.1016/j.gca.2019.02.005
Ingalls, M., Rowley, D. B., Currie, B. S., and Colman, A. S. (2020). Reconsidering the uplift history and peneplanation of the northern lhasa terrane. Tibet. 320, 479–532. doi:10.2475/06.2020.01
Ingalls, M., Rowley, D. B., Olack, G., Currie, B. S., Li, S., Schmidt, J., et al. (2017). Paleocene to Pliocene low-latitude, high-elevation basins of southern Tibet: implications for tectonic models of India-Asia collision, Cenozoic climate, and geochemical weathering. Geol. Soc. Am. Bull. 130, 307–330. doi:10.1130/B31723.1
Lacroix, B., and Niemi, N. A. (2019). Investigating the effect of burial histories on the clumped isotope thermometer: an example from the Green River and Washakie Basins, wyoming. Geochem. Cosmochim. Acta. 247, 40–58. doi:10.1016/J.GCA.2018.12.016
Lawson, M., Shenton, B. J., Stolper, D. A., Eiler, J. M., Rasbury, E. T., Becker, T. P., et al. (2018). Deciphering the diagenetic history of the El Abra formation of eastern Mexico using reordered clumped isotope temperatures and U-Pb dating. Bull. Geol. Soc. Am. 130, 617–629. doi:10.1130/B31656.1
Lloyd, M., Ryb, U., and Eiler, J. M. (2018). Experimental determination of the preserve- tion potential of the dolomite clumped isotope thermometer. Geochem. Cosmochim. Acta. 242, 1–20.
Lohmann, K. C., and Walker, J. C. G. (1989). The δ18O record of phanerozoic abiotic marine calcite cements. Geophys. Res. Lett. 16, 319–322. doi:10.1029/GL016i004p00319
Machel, H. G. (2000). “Application of cathodoluminescence to carbonate diagenesis,” in Cathodoluminescence in Geosciences. Berlin, Heidelberg: Springer, 271–301.
Moore, C. H. (1989). “Carbonate diagenesis and porosity,” in Developments in Sedimentology. Elsevier, 337.
Moore, C. H. (2002). Marine and petroleum geology carbonate reservoirs porosity evolution and diagenesis in a sequence stratigraphic framework. Marine Petrol. Geology doi:10.1016/S0264-8172(03)00037-0
Nielsen, L. C., De Yoreo, J. J., and DePaolo, D. J. (2013). General model for calcite growth kinetics in the presence of impurity ions. Geochem. Cosmochim. Acta. 115, 100–114. doi:10.1016/j.gca.2013.04.001
Orme, D. A. (2017). Burial and exhumation history of the xigaze forearc basin, yarlung suture zone, Tibet. Geosci. Front. 1–15. doi:10.1016/j.gsf.2017.11.011
Orme, D. A., Carrapa, B., and Kapp, P. (2014). Sedimentology, provenance and geochronology of the upper cretaceous-lower eocene western xigaze forearc basin, southern Tibet. Basin Res. 27, 387–411. doi:10.1111/bre.12080
Passey, B. H., and Henkes, G. A. (2012). Carbonate clumped isotope bond reordering and geospeedometry. Earth Planet Sci. Lett. 351–352, 223–236. doi:10.1016/j.epsl.2012.07.021
Quade, J., Leary, R., Dettinger, M. P., Orme, D., Krupa, A., DeCelles, P. G., et al. (2020). Resetting southern tibet: the serious challenge of obtaining primary records of paleoaltimetry. Global Planet. Change. 191, 103194. doi:10.1016/j.gloplacha.2020.103194
Rowley, D. B., and Currie, B. S. (2006). Palaeo-altimetry of the late eocene to miocene lunula basin, central Tibet. Nature. 439, 677–681. doi:10.1038/nature04506
Rowley, D. B., Pierrehumbert, R. T., and Currie, B. S. (2001). A new approach to stable isotope-based paleoaltimetry: implications for paleoaltimetry and paleohypsometry of the high himalaya since the late miocene. Earth Planet Sci. Lett. 5836, 1–17. doi:10.1016/S0012-821X(01)00324-7
Ryb, U., Lloyd, M. K., Stolper, D. A., and Eiler, J. M. (2017). The clumped-isotope geochemistry of exhumed marbles from Naxos, Greece. Earth Planet Sci. Lett. 470, 1–12. doi:10.1016/j.epsl.2017.04.026
Snell, K. E., Koch, P. L., Druschke, P., Foreman, B. Z., and Eiler, J. M. (2014). High elevation of the “nevadaplano” during the late cretaceous. Earth Planet Sci. Lett. 386, 52–63. doi:10.1016/j.epsl.2013.10.046
Snell, K. E., Thrasher, B. L., Eiler, J. M., Koch, P. L., Sloan, L. C., and Tabor, N. J. (2013). Hot summers in the bighorn basin during the early Paleogene. Geology. 41, 55–58. doi:10.1130/G33567.1
Stolper, D. A., and Eiler, J. M. (2015). The kinetics of solid-state isotope-exchange reactions for clumped isotopes: a study of inorganic calcites and apatites from natural and experimental samples. Am. J. Sci. 315, 363–411. doi:10.2475/05.2015.01
Sun, S. S., and Hanson, G. N. (1975). Origin of Ross Island basanitoids and limitations on the heterogeneity of mantle sources of alkali basalts and nephelinites. Contrib. Mineral. Petrol. 54, 77–106. doi:10.1007/BF00395006
Vincent, B., Brigaud, B., Emmanuel, L., and Loreau, J.-P. (2017). High resolution ion microprobe investigation of the δ18O of carbonate cements (Jurassic, Paris Basin, France): new insights and pending questions. Sediment. Geol. 350, 42–54. doi:10.1016/j.sedgeo.2017.01.008
Keywords: carbonate alteration, carbonate diagenesis, oxygen isotope (δ18O), paleoaltimetry, petrography, clumped isotope thermometry
Citation: Ingalls M and Snell KE (2021) Tools for Comprehensive Assessment of Fluid-Mediated and Solid-State Alteration of Carbonates Used to Reconstruct Ancient Elevation and Environments. Front. Earth Sci. 9:623982. doi: 10.3389/feart.2021.623982
Received: 30 October 2020; Accepted: 07 January 2021;
Published: 25 February 2021.
Edited by:
Alexis Licht, University of Washington, United StatesReviewed by:
David Bajnai, University of Cologne, GermanyJustine Briard, Université de Bourgogne, France
Fuyun Cong, China University of Geosciences Wuhan, China
Copyright © 2021 Ingalls and Snell. This is an open-access article distributed under the terms of the Creative Commons Attribution License (CC BY). The use, distribution or reproduction in other forums is permitted, provided the original author(s) and the copyright owner(s) are credited and that the original publication in this journal is cited, in accordance with accepted academic practice. No use, distribution or reproduction is permitted which does not comply with these terms.
*Correspondence: Miquela Ingalls, aW5nYWxsc0Bwc3UuZWR1