Soil Degassing From the Xianshuihe–Xiaojiang Fault System at the Eastern Boundary of the Chuan–Dian Rhombic Block, Southwest China
- 1Hebei Key Laboratory of Strategic Critical Mineral Resources, Hebei GEO University, Shijiazhuang, China
- 2College of Earth Sciences, Heibei GEO University, Hebei Province, Shijiazhuang, China
- 3Institute of Earthquake Forecasting, China Earthquake Administration, Beijing, China
- 4Seismological Bureau of Inner Mongolia Autonomous Region, Hohhot, China
The Chuan–Dian region, situated in the middle part of the north-south seismic zone of mainland China in a highly deformed area of the eastern margin of the Tibetan Plateau, is one of the principal areas for monitoring earthquake activities in China. In this study, the geochemical characteristics of soil degassing (of CH4, H2, CO2, Rn, and Hg) and, the relationship between degassing and fault activity, were investigated in the Xianshuihe–Xiaojiang fault system (XXFS) at the eastern boundary of the Chuan–Dian rhombic block. The mean soil-gas concentrations of CH4, H2, CO2, Rn, and Hg in the XXFS were 8.1 ppm, 9.9 ppm, 0.5%, 15.1 kBq/m3 and 12.9 ng/m3, respectively. The δ13CCO2 and δ13CCH4 values of the hot-spring gases varied from −11.9‰ to −3.7‰ and −62.5‰ to 17‰, respectively. The He-C isotopic ratios indicate that the carbon in the northern and middle parts of the XXFS may have originated from deep fluids, whereas the carbon in the southern part of the XXFS is of organic origin. The high concentrations of soil gas were distributed near the faults, indicating that the faults could act as channels for gas migration. The distributions of the high soil-gas concentrations in the XXFS coincide with the highest stress and maximum strain rates, indicating that the fault activity enhanced permeability and increased the emission rates of the gases. The results of this study will be helpful for degassing in active fault zones and earthquake monitoring.
Introduction
Degassing (of gases such as Rn, He, CO2, CH4, CO, H2, N2, and Hg) from the solid Earth to the atmosphere can be enhanced by varying the crustal stress (King, 1986; Toutain and Baubron, 1999; Du et al., 2008; Bohnhoff and Zokack, 2010). Previous studies and field observations have demonstrated that faults and/or fractures are highly permeable pathways for gas migration from gas sources, which retain the signatures of gas sources in the soil cover (e.g. Fu et al., 2005; Ciotoli et al., 2014, 2016; Sciarra et al., 2015, 2017, 2018, 2020; Yuce et al., 2017; Xiang et al., 2020). Gas anomalies are well-correlated with earthquakes (e.g. King, 1986; Du et al., 2008; Einarsson et al., 2008; Li et al., 2009) and are much more sensitive to stress variations (King, 1986; Einarsson et al., 2008; Toutain and Baubron, 1999). Therefore, soil-gas measurement is an efficient tool for the study of fault activity (e.g. Toutain and Baubron, 1999; Walia et al., 2010; Yang et al., 2011).
Northward subduction of the Indian plate beneath the Tibetan Plateau makes south-eastern Tibet an important domain involved in the convergence and interaction between the Eurasian and Indian plates (Figure 1A, Tapponnier et al., 2001). The Chuan–Dian region, in the southern part of the north-south seismic zone of mainland China, is tectonically located in the highly deformed area of the eastern Tibetan Plateau. Enclosed by the Xianshuihe–Xiaojiang fault system (XXFS), the Red River fault, and the Jinshajiang fault (Figure 1B), the Chuan–Dian rhombic block is one of the principal areas of earthquake monitoring in China.
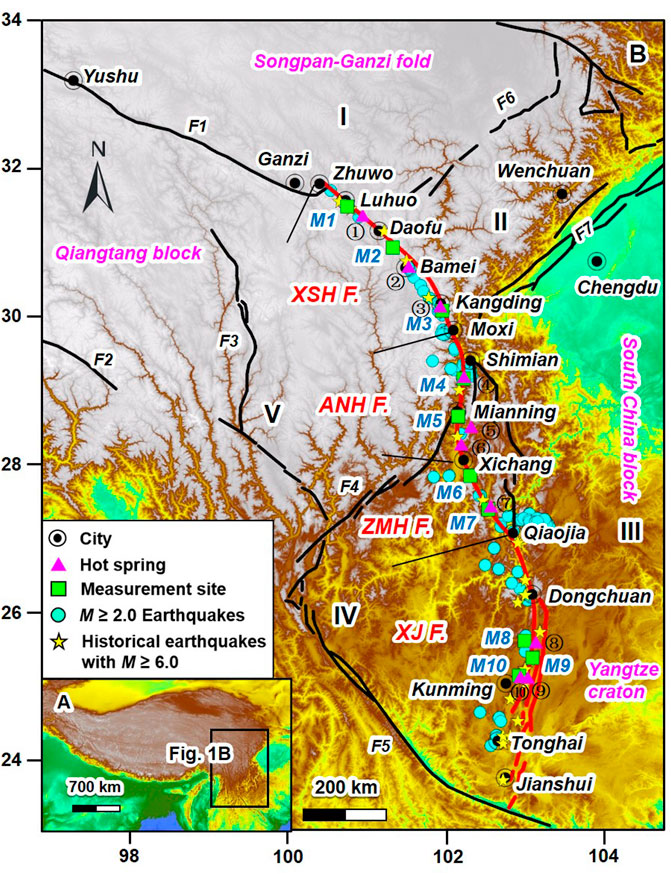
FIGURE 1. (A) Geological map showing the location of the study area. (B) Tectonic and topographic map in the Chuan–Dian rhombic block. I, Ahba block; II, Longmenshan block; III, south China block; IV, the southern subblock of the Chuan–Dian terrace; V, the northern subblock of the Chuan–Dian terrane. F1, Ganzi-Yushu fault; F2, Jiali fault; F3, Jinshajiang fault; F4, Xiaojinhe fault; F5, Red River fault; F6, Longriba fault; F7, Longmenshan fault zone. Modified after Jiang et al. (2014). M1-M10 are the soil-gas measurement sites in this study. Abbreviations of the faults: XSH, Xianshuihe fault; ANH, Anninghe fault; ZMH, Zemuhe fault; XJ, Xiaojiang fault. Numbers ①–⑩ represent the bubbling hot springs distributed along the XXFS (Kama, Guanding, Zimakua, Xide, Hongmo, Liutie, Xundiantang, Yiliang, and Tangchi hot springs, respectively). The M ≥ 2.0 earthquakes occurred from January 2012 to October 2020 within 50 km of the XXFS. Earthquake data are from China Earthquake Networks Center (CENE, http://www.ceic.ac.cn). Data of the histotical earthquakes are from Wang et al. (2008) and Wen et al. (2011).
The XXFS is located at the eastern boundary of the Chuan–Dian rhombic block (Figure 1B) and has a curved left-lateral strike-slip structure extending at least 1,400 km into the eastern margin of the Tibetan Plateau (Allen et al., 1991). Fieldwork confirms that the XXFS is one of the largest and most seismically active fault systems in China. The XXFS has experienced at least 35 earthquakes of M > 6 in the last 300 years, and almost all segments of the system have been the locus of major earthquakes within the historical record (BGMRSP, 1993; Figure 1B).
Previous studies primarily focused on the soil gas and hot-spring gas at the western boundary of the Chuan–Dian rhombic block (e.g. Li et al., 2018; Zhou et al., 2020). However, at the eastern boundary of the rhombic block, only the geochemical characteristics of several gas species (CO2, Rn, and Hg) in the middle part of the XXFS have been reported (Zhang et al., 2019a; Zhange, 2019b). So far, systematic investigation of multi-gas components in soil gases at the eastern boundary of the rhombic block has rarely been reported. In this study, the geochemical characteristics of soil degassing of CH4, H2, CO2, Rn, and Hg and the relationship between degassing and fault activity in the XXFS were reported.
Geological Setting
The XXFS consists of the Xianshuihe (XSH), Anninghe (ANH), Zemuhe (ZMH), and Xiaojiang (XJ) faults from north to south (Figure 1B).
The NW-striking XSH fault is a highly active strike-slip fault in the eastern margin of the Tibetan Plateau that begins in Luding, passes through Kangding and Luhuo, and extends into Qinghai Province (Figure 1B). The XSH fault has a long active history. The regional uplift tectonic motion of the Tibetan Plateau in the Neogene produced the XSH NW–NNW striking left-lateral slip fault that cuts through the sialsphere, producing the belts of intensive faults and cleavage. The XSH fault is very active and is accompanied by many destructive earthquakes, such as the M = 7.9 earthquake that occurred in Luhuo in 1973 (Figure 1B, BGMRSP, 1993).
The ANH fault starts from the town of Jintang, passes through Mianning, Dechang, and Huili, and extends into Yunnan Province. The ANH fault is multi-segmented. The northern, middle, and southern segments are separated at Shimian and Dechang. In this study, the northern and middle segments of the ANH fault were selected for targeting. The northern segment of the ANH fault is characterized by thrust and ductile shear, whereas the middle segment is characterized by deep faulting. Seismic activities in these segments are severe, with many M ≥ 6 earthquakes occurring (BGMRSP, 1993).
The ZMH NW-striking fault conjoins with the ANH fault at Xichang and is currently active (BGMRSP, 1993). The basement of the ZMH fault primarily consists of metamorphic rocks derived from granite, rhyolite, and basalt (Ren et al., 2010). Quaternary alluvial sediments, which are primarily composed of sandy gravel, fine sand, silt, and clay, are widely distributed in the study area (Ren et al., 2010).
The XJ fault is a zone of intense seismic activity that starts from Zhaojue in Sichuan Province and terminates in Yunnan Province. The fault divides into two branches south of the city of Dongchuan (Figure 1B). A wide crushed zone formed along the fault. The XJ fault is distinctly currently active and is characterized by frequent seismic activities and many hot springs (BGMRSP, 1993).
The field survey showed hot springs widely distributed along the XXFS (Zhou et al., 2015). In this study, the Jinkagu (①), Kama (②), and Guanding (③) hot springs in the XSH fault; the Zimakua (④), Xide (⑤), and Hongmo (⑥) hot springs in the ANH fault; the Liutie (⑦) hot spring in the ZMH fault; and the Xundian (⑧), Yiliang (⑨), and Tangi (⑩) hot springs in the XJ fault (Figure 1B) are distributed near the soil-gas measurement sites (Figure 1B); bubbling gas from these hot springs was sampled.
Measurement and Sampling Method
Measurement Method of Soil Gas
The soil-gas measurements in the XXFS were conducted on 1–13 March 2019 to minimize the effect of weather factors. Ten measurement sites (M1 to M10 in Figure 1B) along the XXFS were selected for measurement. The measurement sites of M1–M3, M4–M5, M6–M7, and M8–M10 were in the XSH, ANH, ZMH, and XJ faults, respectively (Figure 1B). M8 and M10 were in the western branch of the XJ fault, whereas M9 was in the eastern branch of the XJ fault (Figure 1B).
Four parallel survey lines with an interval of approximately 10 m were laid across the fault at each measurement site. Gas concentrations were measured at 16 points on each survey line; 72 measurement points at M3 were laid out across the XSH fault. The distance between each measurement point was approximately 10 m, whereas those far from the fault were separated by approximately 20–100 m at places that were confined by local conditions. A total of 648 soil-gas measurement points for each gas species were measured in the XXFS.
The measurement method of the soil-gas concentration was the same as that reported by Zhou et al. (2016). Before measurement, two samplers (S1 and S2), 1-m in length and 2.3-cm in diameter, were hammered into the soil at a depth of ∼80 cm (Figure 2). The concentrations of soil gas at a depth of ∼80 cm were measured. The distance between the two samplers was 50 cm. S1 was used to measure CO2, CH4, H2, and Rn concentrations. The CO2 analyser was connected to S1 to first measure the CO2 concentration. The CH4 analyser was connected to the outlet of the CO2 analyser, and the H2 analyser was connected to the outlet of the CH4 analyser (Figure 2A). When the measurements of CO2, CH4, and H2 concentrations were finished, the Rn analyser was connected to S1 for the measurement (Figure 2A). S2 was only used to measure Hg concentration (Figure 2B).
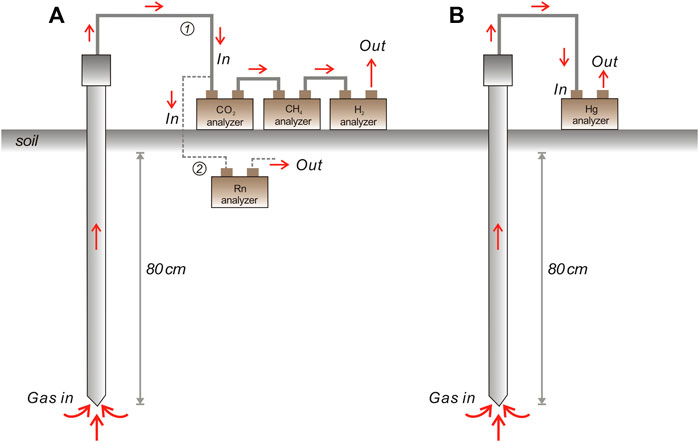
FIGURE 2. Sketch map showing the measurement method of soil gases in the XXFS. ① and ② represent the first and second steps of the measurement at S1. Details of the measurement method can be found in the text.
The CO2 concentration was measured using the non-dispersive infrared gas analyser GXH-3010 with a measuring resolution and accuracy of 0.001% and 4%, respectively (Zhou et al., 2016). The concentration of CH4 was measured using the Huberg LASER ONE with a measurement resolution and accuracy of 1 ppm and 5%, respectively. The concentration of H2 in the soil gas was measured using the ATG-300H analyser, which has been widely used for the measurement of fault degassing. The measurement range and resolution of the H2 analyser were 0–5,000 ppm and 0.1 ppm, respectively. An RAD7 Radon Detector and an RA-915+ Zeeman Mercury Analyser were used to measure Rn and Hg concentrations in soil gas, respectively.
Sampling and Analyzing Methods of Hot-Spring Gas
Ten bubbling hot-spring gas samples were collected from the hot springs of ①–⑩ in the XXFS (Figure 1B) in 2017 and 2018 using the water displacing method. The gases were collected in soda-lime glass bottles with a volume of 500 ml. An inverted funnel was submerged and placed on top of the bubbles to collect the hot-spring gas. The collected gas in the funnel flowed into a bottle filled with hot-spring water and replaced the water in the bottle (Zhou et al., 2015; Zhang et al., 2017). Details of the sampling method can be found in Zhou et al. (2015) and Zhang et al. (2017).
The δ13CCO2 and δ13CCH4 values of the gas samples were analysed at the Key Laboratory of Petroleum Resources Research, Institute of Geology and Geophysics, Chinese Academy of Science. A GC-IRMS analytical system gas chromatograph (Agilent 6890)-stable isotope ratio mass spectrometer (Thermo-Fisher Scientific Delta Plus XP) was used for carbon isotopic ratio analysis. The δ13CCO2 and δ13CCH4 values were reported relative to PDB.
Results and Discussion
Statistical Results and Spatial Distributions of Soil Gases in the XXFS
The statistical results of the CH4, H2, CO2, Rn, and Hg concentrations at each measurement site are listed in Table 1. The concentrations of CH4, H2, CO2, Rn, and Hg in the XXFS range from 0–1,130 ppm, 0.2–193.3 ppm, 0.01–25%, 0.03–258 kBq/m3, and 1–78 ng/m3, respectively (Table 1).
We used the normal probability method proposed by Sinclair (1991) to objectively identify the threshold, which distinguishes different populations (background, mixing, and anomalous) well (e.g. Sinclair, 1974, 1991; Sciarra et al., 2015, 2017, 2018, 2020; Zhou et al., 2015; Yuce et al., 2017). The background population is related to surface processes, such as soil respiration, air dilution, and biological gas origin. The anomaly population is primarily related to internal processes, such as fault/magma degassing (e.g. Chiodini et al., 2004; Sciarra et al., 2015, 2017, Sciarra, 2018, Sciarra, 2020; Di Martino et al., 2016; Yuce et al., 2017). The mixing population represents soil gas with mixing sources by external and internal processes (e.g. Yuce et al., 2017).
The lower anomaly thresholds (LAT) of CH4, H2, CO2, Rn, and Hg in the XXFS were 1.6 ppm, 2.0 ppm, 0.2%, 2.3 kBq/m3, and 5.0 ng/m3, respectively (Figure 3 and Table 2). The upper anomaly thresholds (UAT) of CH4, H2, CO2, Rn, and Hg were 8.1 ppm, 47.7 ppm, 0.7%, 24.9 kBq/m3, and 29.0 ng/m3, respectively (Figure 3 and Table 2). Accordingly, the data of each soil-gas species in the XXFS could be divided into the background (A), mixing (B), and anomaly (C) populations. The proportion and mean concentrations of each population of CH4, H2, CO2, Rn, and Hg in the XXFS are listed in Table 2. The mean concentration of each gas species was calculated using Eq. 1 (Sinclair, 1974):
where PM is the mean concentration of each gas species; fA, fB and fC are the proportion of each population, and PA, PB, and PC are the mean gas concentrations of each population. Therefore, the mean concentrations of CH4, H2, CO2, Rn, and Hg in the XXFS were calculated to be 8.1 ppm, 9.9 ppm, 0.5%, 15.1 kBq/m3, and 12.9 ng/m3, respectively (Table 2), which were close to the soil-gas concentrations measured in the surface rupture zone produced by the Wenchuan Ms 8.0 earthquake in 2008 and 2010 (Figure 4, Zhou et al., 2010; Zhou, et al., 2017).
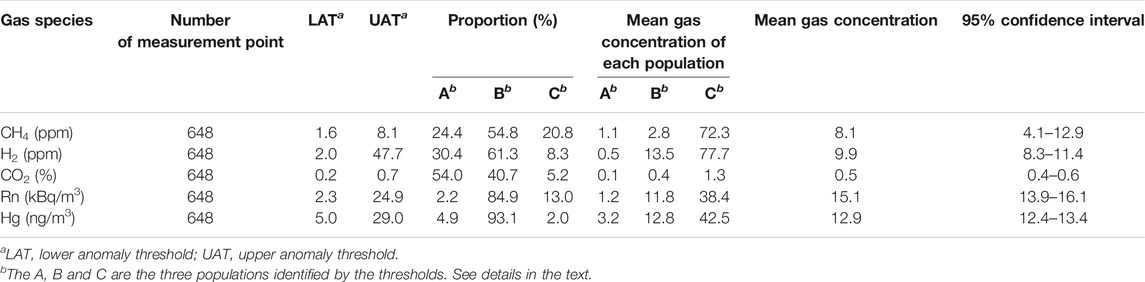
TABLE 2. Threshold values, proportions, mean concentration of each population, and 95% confidence interval of soil gases in the XXFS obtained by statistical approach.
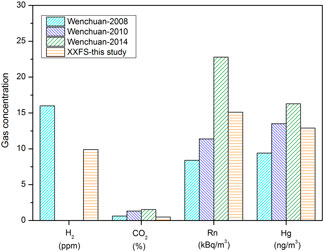
FIGURE 4. Comparations of H2, CO2, Rn, and Hg concentrations in the XXFS and the surface rupture zone produced by the Wenchuan Ms 8.0 earthquake. Data sources: Zhou et al. (2010); Zhou et al. (2015); Zhou et al. (2016).
The spatial distributions of soil-gas concentrations at the 10 measurement sites (obtained by using Kriging interpolation) are shown in Figure 5. From the spatial distributions of each gas species, high concentrations of soil gas were spatially coincident with the location of the fault and appeared close to the fault (Figure 5).
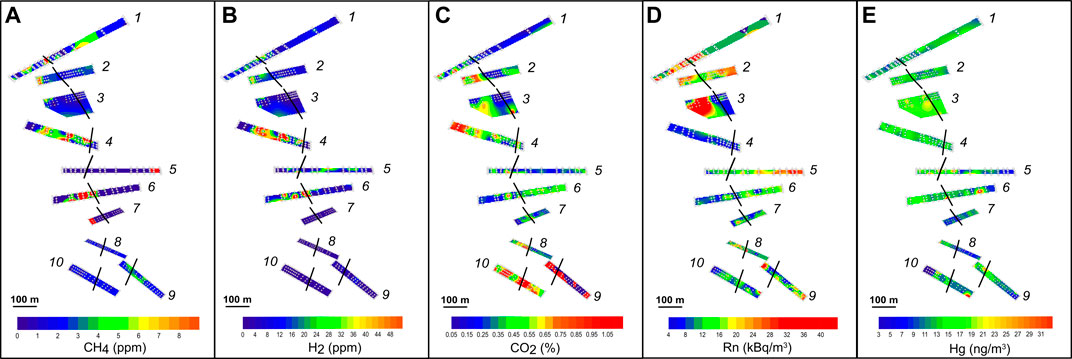
FIGURE 5. Spatial distribution of soil-gas concentrations from M1–M10 in the XXFS. The white cross shows the distribution of the measurement points.
Origin of Carbon in the CO2 and CH4
Gas emissions from the fault zones are from multiple sources (e.g. Williams-Jones et al., 2000; Hong et al., 2010). Mantle, metamorphism of carbonate-bearing rocks, decomposition of organic material and surface biological activity are the principal sources of CO2 in active fault zones (Irwin and Barnes, 1980; Toutain and Baubron, 1999), whereas microbiological and thermogenic genesis are the major processes of CH4 genesis (e.g. Toutain and Baubron, 1999; Milkov and Etiope, 2018).
In this study, the δ13CCO2 and δ13CCH4 values of the hot-spring gas samples varied from -11.9‰ to -3.7‰ and -62.5‰ to 17‰, respectively (Table 3), and are consistent with the δ13CCO2 and δ13CCH4 values reported in previous studies (Figure 6A; Zhou et al., 2015; Shen et al., 2007). Combined with the carbon isotopic values reported in previous studies, the δ13CCO2 and δ13CCH4 values in the XXFS varied from -21.37‰ to -2.9‰ and -62.5‰ to 19‰, respectively (Figure 6A and Supplementary Table S1; Zhou et al., 2015; Shen et al., 2007).
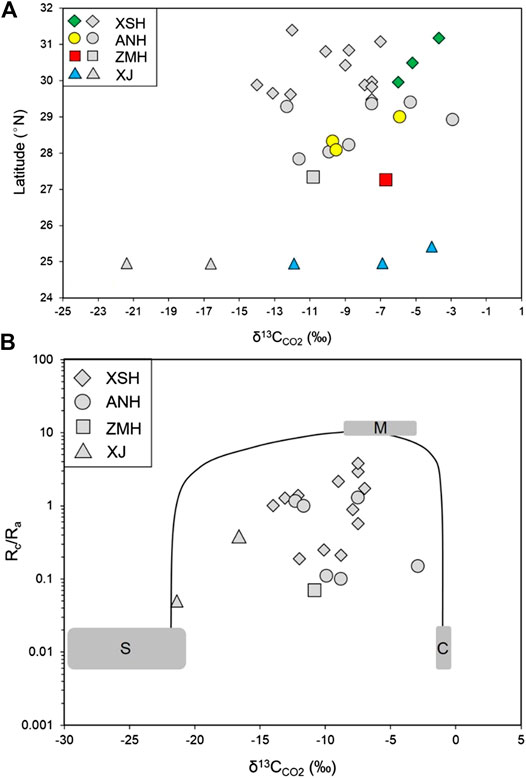
FIGURE 6. (A) Plot of the δ13CCO2 values from south to north along the XXFS. (B) Rc/Ravs. δ13CCO2 plot showing mixing of the end-members of mantle (M), crust (C), and sediment (S) of the bubbling gas samples in the XXFS. The colour symbols are data from bubbling hot-spring gas in this study. The grey symbols are hot-spring gas data reported in Shen et al. (2007) and Zhou et al. (2015). The He-C isotopic data in June 2009 were selected in this study in consideration of the significant influence of earthquake on the variations of He-C isotopic ratios (Zhou et al., 2015).
Previous studies indicated that upper mantle-derived CO2 has δ13CCO2 values between −3‰ and −9‰ with an average value of −6.5‰ (Javoy et al., 1986; Marty and Jambon, 1987). Soil organic matter derived from C3 and C4 plants have δ13CCO2 values varying from −12‰ to −14‰ and −24‰ to −27‰, respectively (Cheng, 1996). The Rc/Ra-δ13CCO2 plot reveals that the gas samples in the XXFS show characteristics of mixing among the end-members of the mantle, crust, and sediment (Figure 6B). However, the He-C isotopic ratios in the XSH and ANH faults showed obvious characteristics of mixing between the end-members of the mantle and crust, whereas those in the ZMH and XJ faults showed greater addition of organic sources (Figure 6B). The δ13CCO2 values near M9 and M10 in the XJ fault are −21.37‰ to −6.9‰ (Table 3 and Supplementary Table S1; Shen et al., 2007), with majority of the δ13CCO2 values within the domain of organic carbon source. The spatial distributions of the CO2 concentration in the XXFS show that the highest concentrations of CO2 were found at M9 and M10 in the XJ fault (Figure 5C). Therefore it could deduce that the carbon in the XJ fault in southern part of the XXFS has an organic carbon source.
CH4 of microbial origin usually has δ13CCH4 values < -55‰, whereas δ13CCH4 values of thermogenic origin vary from −30‰ to −50‰ (Milkov and Etiope, 2018 and references therein). Abiotic CH4 is 13C-enriched with δ13CCH4 values > −20‰ (Milkov and Etiope, 2018 and references therein). The δ13CCH4-δ13CCO2 diagram shows that the majority of the gas samples in the XXFS are plotted within the thermogenic and abiotic domains (Figure 7), indicating the mixing sources of CH4 in the XXFS.
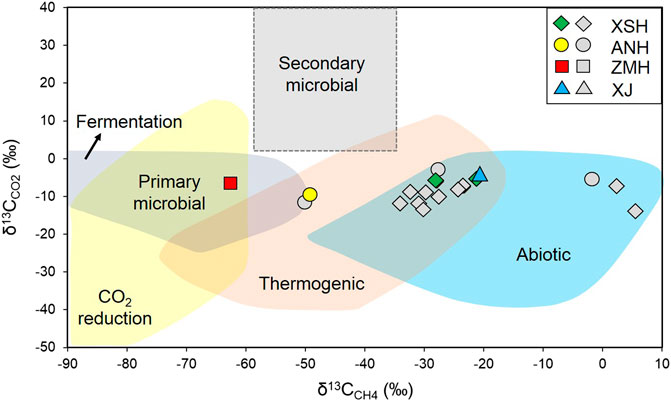
FIGURE 7. Plot of δ13CCO2vs. δ13CCH4. The colour symbols are data from bubbling hot-spring gas in this study. The grey symbols are hot-spring gas data reported in Shen et al. (2007) and Zhou et al. (2015). Modified after Milkov and Etiope (2018).
Geophysical studies (e.g. Liu and Wang, 2020) indicate that anomalies of low velocity and high Poisson’s ratio are present at the XSH, ANH, and ZMH faults in the XXFS (26.5° N, 28° N, 29.5° N, 31.5° N, Figure 1B and Liu and Wang, 2020) and are interpreted as fluid intrusions (partial melt of deep material) from the lower crust and/or uppermost mantle. The δ13CCO2 values in the XSH, ANH, and ZMH faults are higher than those in the XJ fault (Figure 8A; Table 3 and Supplementary Table S1). According to previous studies, the 3He/4He ratios in the XXFS are 0.05–3.8 Ra (where Ra is the air-corrected 3He/4He ratios, Supplementary Table S1; Shen et al., 2007; Zhou et al., 2015), which lie within the typical crust and mantle domains (e.g. Sano and Marty, 1995; Zhang et al., 2017). The 3He/4He ratios in the XSH, ANH, and ZMH faults range from 0.19 to 3.8 Ra, 0.1–1.3 Ra, and 0.07 Ra, respectively, which are higher than those in the XJ fault (0.05–0.35 Ra) (Figure 8B and Supplementary Table S1). Analysis of He-C isotopic ratios in the XXFS was used to deduce that the carbon in the XSH, ANH, and ZMH faults might have originated from deep fluids, whereas the carbon in the XJ fault is of organic origin.
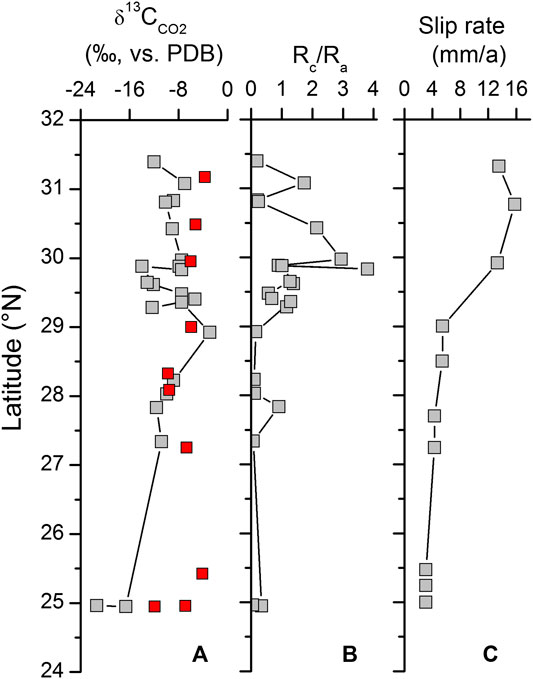
FIGURE 8. Variations of δ13CCO2 values (A), Rc/Ra ratios (B), and fault slip rates (C) from north to south in the XXFS. The colour symbols are data reported in this study. The grey symbols are data reported by previous studies. Data sources: Chen (2006), Shen et al. (2007), Shi et al. (2012), Zhou et al. (2015), and Yue et al. (2017).
Anomaly and Degassing of Rn in the XXFS
Rn is inert and has low mobility in the geological environment (Tanner, 1964; Dubois et al., 1995). The uranium decay and the upward migration of Rn along the fault could represent the two sources of Rn in the fault zone (Toutain and Baubron, 1999; Sciarra et al., 2015; Sciarra et al., 2017; Sciarra et al., 2018; Sciarra et al., 2020). Previous studies indicate that the emanating power of the rock and soil, the permeability of the host rock, and the flow of the carrying gas are the primary factors that influence Rn activity in a diffusive system (e.g. Ball et al., 1991; Morawska and Phillips, 1993). Therefore, we used the formula of Åkerblom (1993) (Eq. 2), which is based on the 222Rn activity at equilibrium with parent radionuclides (radium) in the soil, by verifying the anomaly threshold to determine the origin of Rn in the XXFS (Åkerblom, 1993; Sciarra et al., 2018):
where CRn and CRa are the concentrations of Rn and radium in the soil gas (Bq/m3) and soil (Bq/kg), respectively. ε is the emanation power coefficient (non-dimensional), ρ is the soil density (kg/m3), and n is the effective porosity coefficient (non-dimensional).
Previous studies show that the average content of soil radium-226 in China is 36.5 Bq/kg (e.g. Sun et al., 2004). The ε values are variable in different rock and soil types (e.g. Righi and Bruzzi, 2006; Jobbágy et al., 2009; Qin, 2012; Shen et al., 2012). The surrounding rocks and strata outcrop in the XXFS can be divided into four types: 1) volcanic rocks and acidic intrusions; 2) dolomite, limestone and carbonate; 3) sandstone; and 4) polyterrigenous materials (Table 4, BGMRSP, 1993). Therefore, the ε values of the four types of surrounding rocks and strata in the XXFS have values of 0.48 (Qin, 2012), 0.074 (Righi and Bruzzi, 2006; Jobbágy et al., 2009), 0.4 (Qin, 2012), and 0.12 (Shen et al., 2012), respectively (Table 5). The values of the soil porosity (n) were calculated using Eq. (3):
where 2.65 g/cm3 is the mean density of soil porosity. The values of subsoil bulk density (kg/m3) at a depth of 100 cm in the XXFS were selected from the Harmonized World Soil database (HWSD), which is elaborated by the Food and Agriculture Organization (FAO) (FAO/IIASA/ISRIC/ISSCAS/JRC, 2012). Therefore, the calculated Rn concentrations from M1–M10 in the XXFS ranged from 8.5 kBq/m3 to 61.1 kBq/m3 (Table 5), with mean value of 40.2 kBq/m3. The calculated Rn concentration in the XXFS was higher than the statistically defined anomaly threshold (24.9 kBq/m3) (Table 2). Therefore, the Rn concentrations that exceeded the calculated value were likely not supported by in-situ production.
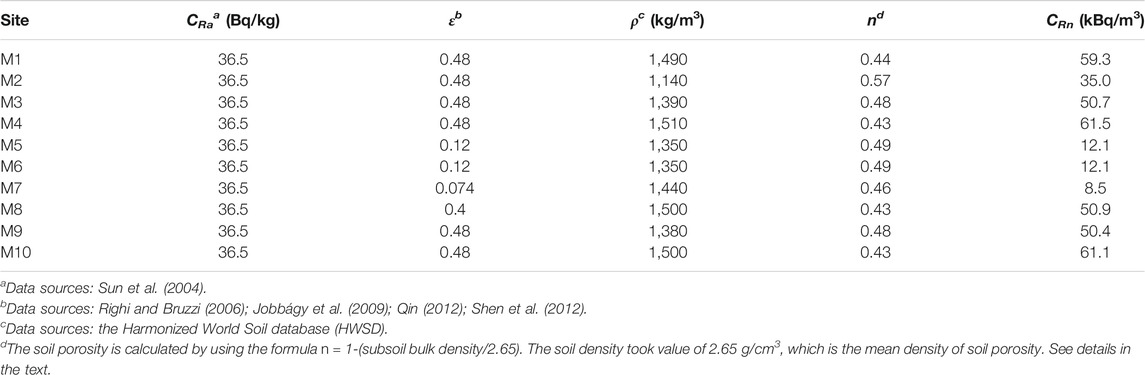
TABLE 5. Parameters of radium concentration (CRa), emanation power coefficient (ε), soil density (ρ), and effective porosity coefficient (n) in the XXFS.
The measurement sites with high Rn concentrations were spatially consistent with the distributions of the volcanic and acidic intrusions in the XXFS (Figure 5D and Table 4). The abovementioned analysis concluded that the concentrations of Rn lower than the UAT in the XXFS might originate from in-situ production. Rn concentrations higher than the UAT in the XXFS might be related to the upward migration of Rn along the faults. Rn degassing from the fault was also verified by the spatial distributions of Rn concentrations in the XXFS (Figure 5D). Additionally, the high concentrations of Rn in M5 (Figure 5D) demonstrate the degassing of Rn from the fault according to the field survey.
Relationship Between Soil-Gas Concentration and Fault Activity
In our study, high concentrations of Rn were primarily found at the XSH fault (Figure 5D and Table 1), which has the highest stress, fault slip rate, and maximum strain rate in the XXFS (Figure 8C and Chen., 2006; He and Lu, 2007; Shi et al., 2012; Zhao et al., 2015; Yue et al., 2017; Li et al., 2019). Fault activity can facilitate the transport of fluids due to episodic fracturing events that can release quantities of deep fluids (Evans et al., 1997; Kulongoski et al., 2005; Tanikawa et al., 2010; Zhang et al., 2016).
In our study, granites are well-developed in the XSH fault (BGMRSP, 1993; Table 4). In a previous study, the permeability of granites increased by three orders of magnitude as the slip rate increased, indicating that increasing the slip rate could create and maintain elevated fault permeability (Zhang et al., 2017).
From the spatial distributions of soil gases in the XXFS (Figure 5), the anomalously high concentrations of CH4, H2, CO2, Rn, and Hg appeared near the fault, which might indicate that the fault slip-rate influenced degassing from the active fault zone. The elevated permeability of the fault facilitates the migration of gases in the active fault zone. The different patterns of soil-gas concentrations across the fault might be influenced by the permeability of the fault zone, such as the porosity of the fault and soil characteristics of the measurement site (e.g. Fu et al., 2005).
Conclusion
In this study, the geochemical characteristics of soil gas and the relationship between degassing and fault activity in the XXFS were examined. Summarising:
(1) The mean concentrations of CH4, H2, CO2, Rn, and Hg in the XXFS are 8.1 ppm, 9.9 ppm, 0.5%, 15.1 kBq/m3, and 12.9 ng/m3, respectively. Anomalously high concentrations of soil gases primarily appeared near the fault, indicating the degassing of faults in the XXFS.
(2) In the XXFS, the δ13CCO2 and δ13CCH4 values varied from −11.9‰ to -3.7‰ and −62.5‰ to 17‰, respectively. He-C isotopic analysis indicates that the soil gas in the XSH, ANH, and ZMH faults might have originated from deep fluids, whereas the carbon in the XJ fault is of organic origin. The CH4 in the XXFS might have thermogenic and abiotic origins.
(3) The concentrations of Rn lower than the UAT in the XXFS might be due to the in-situ production. The anomaly of Rn concentrations (higher than the UAT) in the XXFS might be related to the upward migration of Rn along the faults.
(4) The distributions of the high concentrations of soil gases were spatially coincident with the highest stress and the maximum strain rate in the XXFS, which might indicate that the fault activity enhanced permeability and, thus, increased gas emission rates.
Data Availability Statement
The original contributions presented in the study are included in the article/Supplementary Material, further inquiries can be directed to the corresponding author.
Author Contributions
YS: Methodology, data analysis, conceptualization and writing XZ: Investigation, methodology and conceptualization YY: Investigation JL: Investigation WF: Investigation WW: Investigation YL: Investigation.
Funding
This study was supported by the National Key Research and Development Project (2017YFC1500501), the National Natural Science Foundation of China (41673106, 41902312), the Special Fund of the Institute of Earthquake Forecasting, China Earthquake Administration (2017IES010205, 2016IES010304, 2018IES010104, 2020IEF0604, 2020IEF0703) and the Natural Science Foundation of Hebei Province (D2020403050).
Conflict of Interest
The authors declare that the research was conducted in the absence of any commercial or financial relationships that could be construed as a potential conflict of interest.
Acknowledgments
The authors are grateful to Fang Du and Hong Fu for their assistance with fieldwork. Two anonymous referees are acknowledged for their extremely constructive reviews of an earlier version of the paper. We thank the journal editor, Francesco Italiano, for his support. Harmonized World Soil Database (HWSD) is thanked for providing the soil data in this research. The CGIAR Consortium for Spatial Information (CGIAR-CSI) provided the STRM data.
Supplementary Material
The Supplementary Material for this article can be found online at: https://www.frontiersin.org/articles/10.3389/feart.2021.635178/full#supplementary-material.
References
Åkerblom, G. (1993). Ground radon: monitoring procedure in Sweden: lecture at the“JAG” discussion meeting on “radon workshop, Geology, environment, technology”. London,United Kingdom: Royal Astronomical Society.
Allen, C. R., Luo, Z. L., Qian, H., Wen, X. Z., Zhou, H. W., and Huang, W. S. (1991). Field study of a highly active fault zone: the Xianshuihe fault of southwestern China. Geol. Soc. Am. Bull. 103, 1178–1199.
Ball, T. K., Cameron, D. G., Colman, T. B., and Roberts, P. D. (1991). Behaviour of radon in the geological environment: a review. Q. J. Eng. Geol.y 24, 169–182.
BGMRSP (1993). Regional Geology of xizang (Tibet) autonomous region. Beijing, China: Geological Publishing House.
Bohnhoff, M., and Zoback, M. D. (2010). Oscillation of fluid‐filled cracks triggered by degassing of CO2 due to leakage along wellbores. J. Geophys. Res. 115, B11305. doi:10.1029/2010JB000848
Chen, G. (2006). Structural transformation and strain partitioning along the northeast boundary belt of the Sichuan-Yunnan Block. Doctor’s Thesis. Beijing (China): Institute of Geology, China Earthquake Administration.
Cheng, W. (1996). Measurement of rhizosphere respiration and organic matter decomposition using natural 13C. Plant and Soil 183, 263–268.
Chiodini, G., Cardellini, C., Amato, A., Boschi, E., Caliro, S., Frondini, F., et al. (2004). Carbon dioxide Earth degassing and seismogenesis in central and southern Italy. Geophys. Res. Lett. 31, L07615. doi:10.1029/2004GL019480
Ciotoli, G., Bigi, S., Tartarello, C., Sacco, P., Lombardi, S., Ascione, A., et al. (2014). Soil gas distribution in the main coseismic surface rupture zone of the 1980, Ms = 6.9, Irpinia earthquake (southern Italy). J. Geophys. Res. Solid Earth 119, 2440–2461. doi:10.1002/2013JB010508
Ciotoli, G., Etiope, G., Marra, F., Florindo, F., Giraudi, C., and Ruggiero, L. (2016). Tiber delta CO2-CH4 degassing: a possible hybrid, tectonically active Sediment‐Hosted Geothermal System near Rome. J. Geophys. Res. Solid Earth 121, 48–69. doi:10.1002/2015JB012557
Di Martino, R. M. R., Capasso, G., and Camarda, M. (2016). Spatial domain analysis of carbon dioxide from soils on Vulcano Island: implications for CO2 output evaluation. Chem. Geol. 444, 59–70. doi:10.1016/j.chemgeo.2016.09.037
Du, J., Si, X., Chen, Y., Fu, H., and Jian, C. (2008). “Geochemical anomalies connected with great earthquakes in China,” in Geochemistry research advances. Editor Stefánssonó (New York, NY: Nova Science Publishers, 57–92.
Dubois, C., Alvarez Calleja, A., Bassot, S., and Chambaudet, A., (1995). “Modelling the 3-dimensional microfissure network in quartz in a thin section of granite,” in Gas geochemistry. Editor C. Dubois (Northwood, London: Science Reviews), 357–368.
Einarsson, P., Theodórsson, P., Hjartardóttir, Á. R., and Guðjósson, G. I. (2008). Radon changes associated with the earthquake sequence in June 2000 in the south Iceland seismic zone. Pure Appl. Geophys. 1, 63–74. doi:10.1007/s00024-007-0292-6
Evans, J. P., Foster, C. B., and Goddard, J. V. (1997). Permeability of fault-related rocks, and implications for hydraulic structure of fault zones. J. Struct. Geol. 19, 1393–1404.
FAO/IIASA/ISRIC/ISSCAS/JRC (2012). Harmonized World soil Database (version 1.2). RomeLaxenburg, Austria: FAO.
Fu, C., Yang, T. F., Walia, V., and Chen, C. (2005). Reconnaissance of soil gas composition over the buried fault and fracture zone in southern Taiwan. Geochem. J. 39, 427–439. doi:10.2343/geochemj.39.427
He, J., and Lu, S. (2007). Lower friction of the Xianshuihe–Xiaojiang fault system and its effect on active deformation around the south-eastern Tibetan margin. Terra Nova 19, 204–210. doi:10.1111/j.1365-3121.2007.00735.x
Hong, W., Yang, T., Walia, V., Lin, S., Fu, C., Chen, Y., et al. (2010). Nitrogen as the carrier gas for helium emission along an active fault in NW Taiwan. J. Appl. Geochem. 25, 593–601. doi:10.1016/j.apgeochem.2010.01.016
Irwin, W. P., and Barnes, I. (1980). Tectonic relations of carbon dioxide discharges and earthquakes. J. of Geo. Res. 85, 3115–3121. doi:10.1029/JB085iB06p03115
Javoy, M., Pineau, F., and Delorme, H. (1986). Carbon and nitrogen isotopes in the mantle. Chem. Geol 57, 41–62. doi:10.1016/0009-2541(86)90093-8
Jiang, G., Xu, X., Chen, G., Liu, Y., Fukahata, Y., Wang, H., et al. (2014). Geodetic imaging of potential seismogenic asperities on the Xianshuihe-Anninghe-Zemuhe fault system, southwest China, with a new 3-D viscoelastic interseismic coupling model. J. Geophys. Res. Solid Earth 120, 1855–1873. doi:10.1002/2014JB011492
Jobbágy, V., Somlai, J., Kovács, J., Szeiler, G., and Kovács, T. (2009). Dependence of radon emanation of red mud bauxite processing wastes on heat treatment. J. Hazard. Mater 172, 1258–1263. doi:10.1016/j.jhazmat.2009.07.131
King, C. Y. (1986). Gas geochemistry applied to earthquake prediction: an overview. J. Geophys. Res. Solid Earth 91, 12269–12281. doi:10.1029/JB091iB12p12269
Kulongoski, J., Hilton, D., and Izbicki, J. (2005). Source and movement of helium in the eastern Morongo groundwater Basin: the influence of regional tectonics on crustal and mantle helium fluxes. Geochim. Cosmochim. Acta 69, 3857–3872. doi:10.1016/j.gca.2005.03.001
Li, Q., Wang, Y., Zhou, Y., and Zhao, C. (2018). Geochemical characteristics of hot spring gases from the Deqing-Zhongdian area, northwestern Yunnan, China. Bull. Mineral. Petrol. Geochemistry 37, 645–651. doi:10.19658/j.issn.1007-2802.2018.37.097
Li, Y., Du, J., Wang, F., Zhou, X., Pan, X., and Wei, R. (2009). Geochemical characteristics of soil gas in Yanqing-Huailai basin, North China. Acta Seismologica Sinica 31, 82–91.
Li, Y., Liu, M., Li, Y., and Chen, L. (2019). Active crustal deformation in southeastern Tibetan Plateau: the kinematics and dynamics. Earth Planet. Sci. Lett. 523, 115708. doi:10.1016/j.epsl.2019.07.010
Liu, G., and Wang, Z. (2020). Correlations of the deep structural characteristics, tidal stress variation and earthquake initiation along the Xianshuihe-Anninghe fault zone. Chinese J. of Geophy. 63, 928–943. doi:10.6038/cjg2020N0206
Marty, B., and Jambon, A. (1987). C/3He in volatile fluxes from the solid Earth: implications for carbon geodynamics. Earth and Planetary Science Letters 83, 16–26.
Milkov, A. V., and Etiope, G. (2018). Revised genetic diagrams for natural gases based on a global dataset of >20,000 samples. Organic Geochemistry 125, 109–120. doi:10.1016/j.orggeochem.2018.09.002
Morawska, L., and Phillips, C. R. (1993). Dependance of the radon emanation coefficient on radium distribution and internal structure of the material. Geochimica et Cosmochimica Acta 57, 1783–1797.
Qin, C. (2012). Study on distribution and model of soil radon in South China. Beijing, China: Master degree thesis of China University of Geosciences (Beijing), 1–41.
Ren, Z., Lin, A., and Rao, G. (2010). Late pleistocene–holocene activity of the Zemuhe fault on the southeastern margin of the Tibetan plateau. Tectonophysics 495, 324–336. doi:10.1016/j.tecto.2010.09.039
Righi, S., and Bruzzi, L. (2006). Natural radioactivity and radon exhalation in building materials used in Italian dwellings. J Environ Radioact 88, 158–170. doi:10.1016/j.jenvrad.2006.01.009
Sano, Y., and Marty, B. (1995). Origin of carbon in fumarolic gas from island arcs. Chemical Geology 119, 265–274.
Sciarra, A., Cantucci, B., and Coltorti, M. (2017). Learning from soil gas change and isotopic signatures during 2012 Emilia seismic sequence. Sci Rep. 7, 14187. doi:10.1038/s41598-017-14500-y
Sciarra, A., Cantucci, B., Sapia, V., De Ritis, R., Ricci, T., Civico, R., et al. (2020). Geochemical and geoelectrical characterization of the Terre Calde di Medolla (Emilia-Romagna, northern Italy) and relations with 2012 seismic sequence. J. of Geochemical Exploration 221, 106688. doi:10.1016/j.gexplo.2020.106678
Sciarra, A., Fascetti, A., Moretti, A., Cantucci, B., Pizzino, L., Lombardi, S., et al. (2015). Geochemical and radiometric profiles through an active fault in the Sila Massif (Calabria, Italy). J. for Geochemical Exploration 148, 128–137. doi:10.1016/j.gexplo.2014.08.015
Sciarra, A., Mazzini, A., Inguaggiato, S., Vita, F., Lupi, A., and Hadi, S. (2018). Radon and carbon gas anomalies along the Watukosek fault system and Lusi mud eruption, Indonesia. Marine and Petroleum Geology 90, 77–90. doi:10.1016/j.marpetgeo.2017.09.031
Shen, C., Shen, L., Xiao, D., and Tan, Y. (2012). Numerical computation of radon distribution in different soils. Atomic Energy Science and Technology 46, 370–374.
Shen, L., Yuan, D., Ding, T., Li, Y., Le, G., and Lin, Y. (2007). Distributing inhomogeneity of helium isotope of CO2 degasification point and its geotectogenesis in southwest of China. Acta Geologica Sinica 81, 475–487.
Shi, F., You, W., and Yun, W. (2012). Recent crustal movement characteristics of Xiaojiang River fault based on the GPS data. J. of Seismological Research 35, 207–212.
Sinclair, A. J. (1991). A fundamental approach to threshold estimation in exploration geochemistry: probability plots revisited. J. of Geochemical Exploration 41, 1–22. doi:10.1016/0375-6742(91)90071-2
Sinclair, A. J. (1974). Selection of threshold values in geochemical data using probability graphs. J. Geochem. Explor. 3, 129–149.
Sun, K., Guo, Q., and Zhuo, W. (2004). Feasibility for mapping radon exhalation rate from soil in China. J Nucl Sci Technol 41, 86–90. doi:10.1080/18811248.2004.9715462
Tanikawa, W., Sakaguchi, M., Tadai, O., and Hirose, T. (2010). Influence of fault slip rate on shear‐induced permeability. J. of Geophys. Res. 115, B07412. doi:10.1029/2009JB007013
Tanner, A. B. (1964). “Radon migration in the ground: a review,” in Natural radiation environment. Editors J. A. Adams, and W. Lowder (Illinois, IL: University of Chicago Press), 161–190.
Tapponnier, P., Zhiqin, X., Roger, F., Meyer, B., Arnaud, N., Wittlinger, G., et al. (2001). Oblique stepwise rise and growth of the Tibet plateau. Science 294, 1671–1677. doi:10.1126/science.105978
Toutain, J. P., and Baubron, J. C. (1999). Gas geochemistry and seismotectonics: a review. Tectonophysics 304, 1–27.
Walia, V., Lin, S. J., Fu, C. C., Yang, T. F., Hong, W., Wen, H., et al. (2010). Soil–gas monitoring: a tool for fault delineation studies along Hsinhua Fault (Tainan), Southern Taiwan. J. Appl. Geochem. 25, 602–607. doi:10.1016/j.apgeochem.2010.01.017
Wang, H., Liu, J., Shi, Y., Zhang, H., and Zhang, G. (2008). Dynamic simulation of interactions between major earthquakes on the Xianshuihe fault zone. Science in China Series D: Earth Sciences 51, 1388–1400. doi:10.1007/s11430-008-0110-8
Wen, X., Du, F., Long, F., and Zhu, H. (2011). Tectonic dynamics and correlation of major earthquake sequences of the Xiaojiang and Qujiang-Shiping fault systems, Yunnan, China. Sci China Earth Sci 54, 1563–1575. doi:10.1007/s11430-011-4231-0
Williams-Jones, G., Stix, J., Heiligmann, M., Charland, A., Sherwood Lood, B., Arner, N., et al. (2000). A model of diffuse degassing at three subduction-related volcanoes. Bull. Volcanol. 62, 130–142. doi:10.1007/S004450000075
Xiang, Y., Sun, X., Liu, D., Yan, L., Wang, B., and Gao, X. (2020). Spatial distribution of Rn, CO2, Hg, and H2 concentrations in soil gas across a thrust fault in Xinjiang, China. Front. Earth Sci. 8, 554924. doi:10.3389/feart.2020.554924
Yang, T. F., Wen, H., Fu, C., Lee, H., Lan, T., Chen, A., et al. (2011). Soil radon flux and concentrations in hydrothermal area of the tatun volcano group, northern taiwan. Geochem. J. 45, 483–490.
Yuce, G., Fu, C. C., D’Alessandro, W., Gulbay, A. H., Lai, C. W., Bellomo, S., et al. (2017). Geochemical characteristics of soil radon and carbon dioxide within the dead sea fault and karasu fault in the mik basin (hatay), Turkey. Chem. Geol. 469, 129–146.
Yue, C., Dang, Y., Yang, Q., and Zou, B. (2017). Analysis of the current activity in Sichuan-Yunnan Region and its sub blocks of main faults. Geod Geodyn. 37, 176–181.
Zhang, L., Liu, Y., Bao, C., Gao, X., Su, Q., and Fang, Z. (2019b). Characteristics of fault soil gas in Manning of western Sichuan Province, China. Environmental Chemistry 38, 777–783. doi:10.7524/j.issn.0254-6108.2018100501
Zhang, L., Liu, Y. W., Bao, C., and Guo, L. S. (2019a). Distribution characteristics of soil mercury in Anninghe fault zone. Acta Seismologica Sinica 41, 249–258. doi:10.11939/jass.20180141
Zhang, M., Guo, Z., Zhang, L., Sun, Y., and Cheng, Z. (2017). Geochemical constraints on origin of hydrothermal volatiles from southern Tibet and the Himalayas: understanding the degassing systems in the India-Asia continental subduction zone. Chemical Geology 469, 19–33. doi:10.1016/j.chemgeo.2017.02.023
Zhang, W., Du, J., Zhou, X., and Wang, F. (2016). Mantle volatiles in spring gases in the Basin and Range Province on the west of Beijing, China: constraints from helium and carbon isotopes. J Volcanol Geotherm Res. 309, 45–52. doi:10.1016/j.jvolgeores.2015.10.024
Zhao, J., Jiang, Z. S., Niu, A. F., Liu, J., Wu, Y. Q., Wei, W. X., et al. (2015). Study on dynamic characteristics of fault locking and fault slip deficit in the eastern boundary of the Sichuan-Yunnan rhombic block. Chinese J. of Geophy. 58, 872–885. doi:10.6038/cjg20150316
Zhou, X., Chen, Z., and Cui, Y. (2016). Environmental impact of CO2, Rn, Hg degassing from the rupture zones produced by Wenchuan M s 8.0 earthquake in western Sichuan, China. Environ Geochem Health 38, 1067. doi:10.1007/s10653-015-9773-1
Zhou, X., Du, J., Chen, Z., Cheng, J., Tang, Y. L., et al. (2010). Geochemistry of soil gas in the seismic fault zone produced by the Wenchuan Ms 8.0 earthquake, southwestern China. Geochem Trans 11, 5. doi:10.1186/1467-4866-11-5
Zhou, X. C., Sun, F. X., Chen, Z., Lv, C. J., Li, J., Wu, K. T., et al. (2017). Degassing of CO2, CH4, Rn, and Hg in the rupture zones produced by Wenchuan Ms 8.0 earthquake. Acta Petrologica Sinica 33, 291–303.
Zhou, X. C., Wang, W. L., Li, L. W., Hou, J. M., Xing, L. T., Li, Z. P., et al. (2020). Geochemical features of hot spring gases in the Jinshajiang-Red River fault zone, Southeast Tibetan Plateau. Acta Petrologica Sinica 36, 2197–2214. doi:10.18654/1000-0569/2020.07.18
Keywords: soil gas, CO2, CH4, H2, Rn, Hg
Citation: Sun Y, Zhou X, Yan Y, Li J, Fang W, Wang W and Liu Y (2021) Soil Degassing From the Xianshuihe–Xiaojiang Fault System at the Eastern Boundary of the Chuan–Dian Rhombic Block, Southwest China. Front. Earth Sci. 9:635178. doi: 10.3389/feart.2021.635178
Received: 30 November 2020; Accepted: 21 January 2021;
Published: 18 March 2021.
Edited by:
Francesco Italiano, National Institute of Geophysics and Volcanology, ItalyReviewed by:
Alessandra Sciarra, Istituto Nazionale di Geofisica e Vulcanologia (INGV), ItalyGalip, Yuce, Hacettepe University, Turkey
Copyright © 2021 Sun, Zhou, Yan, Li, Fang, Wang and Liu. This is an open-access article distributed under the terms of the Creative Commons Attribution License (CC BY). The use, distribution or reproduction in other forums is permitted, provided the original author(s) and the copyright owner(s) are credited and that the original publication in this journal is cited, in accordance with accepted academic practice. No use, distribution or reproduction is permitted which does not comply with these terms.
*Correspondence: Xiaocheng Zhou, zhouxiaocheng188@163.com