- 1Department of Marine Biology, University of Haifa, Haifa, Israel
- 2Department of Earth, Planetary, and Space Sciences, University of California – Los Angeles, Los Angeles, CA, United States
- 3Department of Ecology and Evolutionary Biology, University of California – Los Angeles, Los Angeles, CA, United States
- 4Department of Atmospheric and Oceanic Sciences, Institute of the Environment and Sustainability, University of California – Los Angeles, Los Angeles, CA, United States
- 5Université de Brest Occidentale, Institut Universitaire Européen de la Mer, Plouzané, France
Scleractinian corals typically form a robust calcium carbonate skeleton beneath their living tissue. This skeleton, through its trace element composition and isotope ratios, may record environmental conditions of water surrounding the coral animal. While bulk unrecrystallized aragonite coral skeletons can be used to reconstruct past ocean conditions, corals that have undergone significant diagenesis have altered geochemical signatures and are typically assumed to retain insufficient meaningful information for bulk or macrostructural analysis. However, partially recrystallized skeletons may retain organic molecular components of the skeletal organic matrix (SOM), which is secreted by the animal and directs aspects of the biomineralization process. Some SOM proteins can be retained in fossil corals and can potentially provide past oceanographic, ecological, and indirect genetic information. Here, we describe a dataset of scleractinian coral skeletons, aged from modern to Cretaceous plus a Carboniferous rugosan, characterized for their crystallography, trace element composition, and amino acid compositions. We show that some specimens that are partially recrystallized to calcite yield potentially useful biochemical information whereas complete recrystalization or silicification leads to significant alteration or loss of the SOM fraction. Our analysis is informative to biochemical-paleoceanographers as it suggests that previously discounted partially recrystallized coral skeletons may indeed still be useful at the microstructural level.
Introduction
Fossil stony corals (phylum Cnidaria, class Anthozoa) are valuable archives for paleoclimatic studies. Elemental and isotopic compositions of their aragonite skeleton can reflect environmental and chemical conditions of seawater in which they grew (e.g., Cohen and McConnaughey, 2003), allowing reconstruction of past seawater conditions (e.g., Watanabe et al., 2001; Gothmann et al., 2015). Proteins, polysaccharides, and lipids comprise the skeletal organic matrix (SOM), which is secreted by cells of the calicoblastic tissue layer of the animal (Puverel et al., 2005; Mass et al., 2014; reviewed by Drake et al. (2020a). Of these matrix components, proteins are the best studied; their preservation in the mineral can lead to inferences of function and provide indirect genetic information in the absence of preserved DNA (e.g., Schroeter et al., 2017). Recent analyses have shown that intact endogenous peptides remain in invertebrate skeletons for 1000s–100,000s of years (Sakalauskaite et al., 2019; Drake L. et al., 2020b; Sakalauskaite et al., 2020). Across various taxa, intact peptides may retain amino acids longer in fossil specimens than if those biomolecules exist individually as free amino acids (Bada et al., 1999), allowing for detection of ecological and ocean chemistry signatures including the occurrence of photosymbiosis in fossil scleractinian corals (Muscatine et al., 2005; Tornabene et al., 2017) and past local nitrogen cycling regimes (Wang et al., 2015).
Nevertheless, the utility of either component of a fossil coral, inorganic or organic, is limited by its preservation (Nothdurft et al., 2007; Rabier et al., 2008). Fossil corals can undergo submarine and/or subaerial post-depositional alterations including dissolution of primary aragonite, precipitation of secondary aragonite cements and/or high-Mg calcite, and recrystallization from aragonite to calcite, with subsequent alteration of the original aragonitic geochemical signature (Brand and Veizer, 1980; Sayani et al., 2011; Griffiths et al., 2013) and potential degradation of proteins with loss of amino acids (Tomiak et al., 2013; Tomiak et al., 2016; Drake et al., 2020b). All primary aragonite corals with minimal macro- and micro-structural inorganic degradation are considered equally, but partially recrystallized corals are often wholly excluded from bulk analysis (e.g., Gothmann et al., 2015), or their recrystallized portions are removed prior to further analysis (e.g., Tornabene et al.). The organic fraction of coral skeleton is typically ∼1% or less (Wainwright, 1963), providing insufficient material for microanalysis if it is significantly degraded, particularly if peptide sequences are the target (Peled et al., 2020). While geochemical signatures may be altered in partially recrystallized samples (McGregor and Gagan, 2003; Allison et al., 2007; Sayani et al., 2011), it is not yet clear whether amino acid data from those samples remain useful.
Methods for determining mineralogy, trace element composition, and amino acid characteristics of coral skeletons are well-established but are not frequently used together. Crystallographic determination of calcium carbonate minerals can be performed non-destructively using x-ray diffraction (XRD) on ground skeleton powders and can be conducted quantitatively (Chung, 1974). Geochemical analysis of element/Ca in such powders by mass spectrometry requires small sample sizes (<100 µg) and is destructive, yielding relative abundance information for traditionally measured elements including Li, B, Mg, Sr, Cd, Ba, U, Na, Mn, Fe that are then standardized to the Ca signal (Yu et al., 2005; Misra et al., 2014; Guillermic et al., 2020). Amino acid composition and racemization analysis is a destructive method and requires a larger amount of skeleton material (typically 1–10 s mg), yielding data about a subset of the 20 common naturally occurring amino acids (Kaufman and Manley, 1998). Amino acid racemization is the reversible process wherein one enantiomer converts to the other over time and is affected by environmental conditions such as temperature (Neuberger, 1948). As most organisms on Earth produce and use the L enantiomer of amino acids (Bai et al., 2009; reviewed by Genchi (2017), the initial conversion is generally considered to be from the L to D form; D/L ratios of certain amino acids can thus be applied as a relative or specific dating tool for fossils from the late Pleistocene to the present (Bada, 1985; Kaufman, 2003; Demarchi, 2020). Use of all three tools for the same specimens can therefore clarify the preservation of not just the abiotic component of fossil corals, but the biomolecular component as well, while using a relatively small amount of material.
Here we describe our analysis of coral skeletons ranging in age from modern to putatively Cretaceous, plus a Carboniferous rugosan coral. We analyzed the mineralogy, geochemistry, and amino acid characteristics for these corals and show that even partially recrystallized fossil coral skeletons likely retain important biochemical information. This work provides a framework for biochemists and paleoceanographers to consider expanding the pool of potential specimens for analysis.
Methods
Samples
Fossils specimens were loaned from the Natural History Museum of Los Angeles County (NHMLAC) Invertebrate Paleontology Department, American Museum of Natural History (AMNH) Paleontology Division, Yale Peabody Museum (Peabody) Invertebrate Paleontology Division, and Harvard Museum of Comparative Zoology (MCZ) Invertebrate Paleontology Collection (Table 1) (Zoology, 2020). One modern coral was loaned from the NHMLAC Marine Biodiversity Center while another was provided from a private research collection. Provenance of fossil corals is reliant on collection location information on file at the loaning museums and in some cases age assignments are questionable based on the scientific literature (Table 1). For instance, we can with some confidence assign the Pleistocene Montastraea cavernosa and Orbicella annularis corals to stage 5e of the Key Largo formation (Hendy et al., 2020); however, we cannot absolutely rule out that it was actually derived from stage 4 (Multer et al., 2002; Muhs et al., 2011). Elemental, mineralogical, amino acid, and proteomic data for two fossil O. annularis specimens and one M. cavernosa have been previously reported (Drake et al., 2020b).
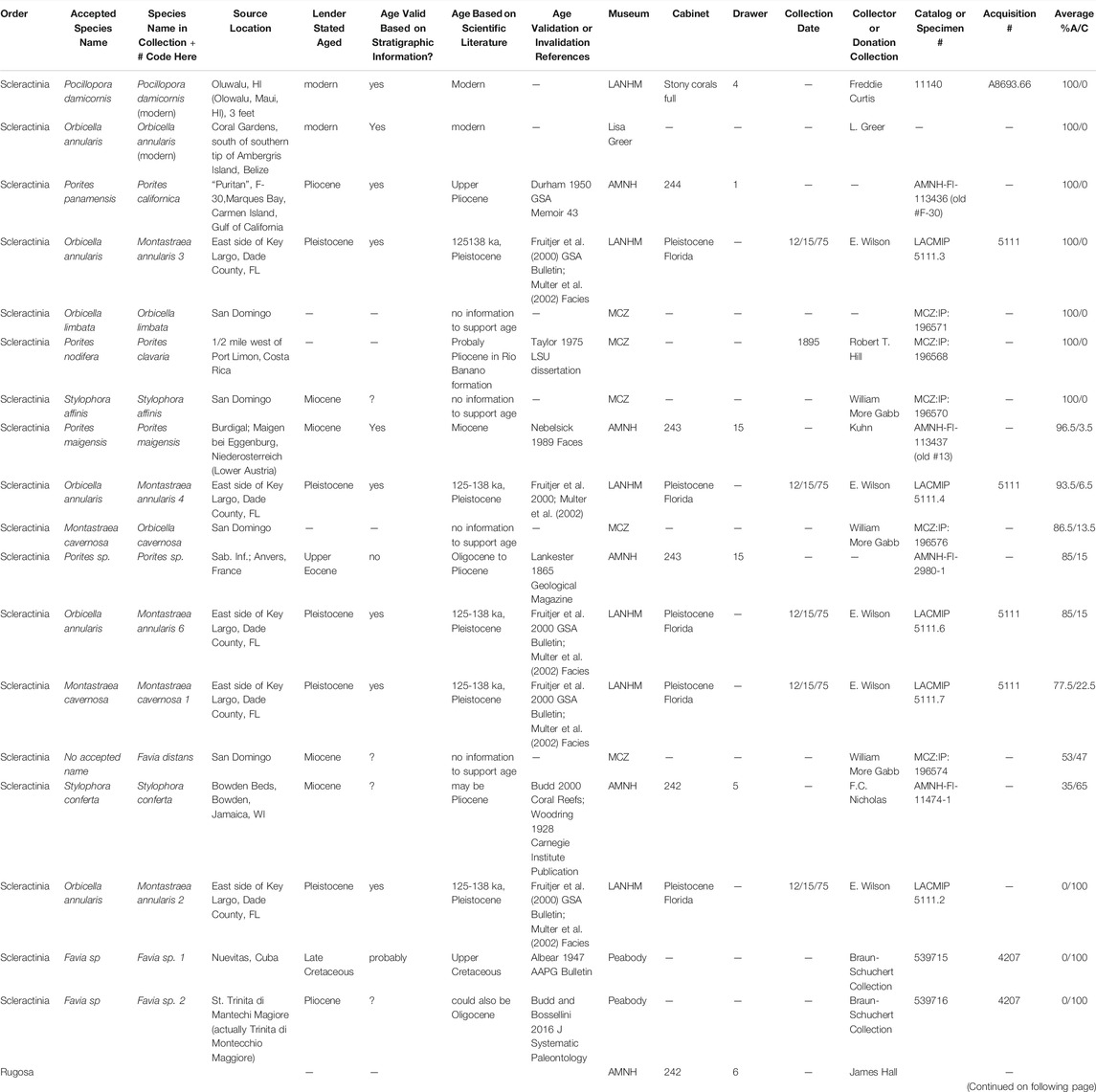
TABLE 1. Modern and fossil coral specimen descriptions including data provided by the lender, verification of stated ages based on stratigraphic information confirmed in the literature, and mineralogy (A = aragonite, C = calcite) averaged between samples milled within Vs. between corallites or at different layers within the sample. Specimens loaned by the AMNH, NHMLAC, Peabody and MCZ have been returned to the lenders; specimens from the private research collection remain in the possession of the authors at this time.
Cleaning
All specimens were slabbed on a water saw to use <∼10% of the borrowed coral head. Fragments were cleaned of organic material in equal parts 3% bleach and 50% hydrogen peroxide, milled or ground, and cleaned three further times in bleach/hydrogen peroxide for 30 min (milled powder) or overnight (ground powder) each cleaning followed by copious rinsing using modified methods of (Stoll et al., 2001). Several checks of the final product suggest that only endogenous intracrystalline SOM remained (Drake et al., 2020b). Samples for XRD were milled using a #2 carbide bur while samples for amino acid analysis were ground to <125 um on an agate mortar and pestle and sieved. Cleaned coral powder analyzed for amino acid parameters was always handled in a glove bag or laminar flow hood to avoid biological contamination.
X-Ray Diffraction
Sub-samples, ∼5–20 mg, of cleaned coral powder from inside vs. between corallites, newer vs. older material, or on two randomly chosen spots per slab were analyzed for mineral content by XRD on a Panalytical X’Pert Pro X-ray Powder Diffractometer. Powders were run on a zero diffraction background plate. Spectra were analyzed in X’Pert Hi-Score software and relative amounts of aragonite and calcite were determined by the Reference Intensity Ratio method (Chung, 1974) using 01-072–1652 calcite and 00-041–1475 aragonite references for all samples. An internal reference standard was not applied as powders were also intended for further mass spectrometry analysis. Sample preparation by milling could induce recrystallization from aragonite to calcite (Gill et al., 1995; Foster et al., 2008; Waite and Swart, 2015); we therefore also performed XRD analyses on ground and cleaned powders from a subset of samples with mixed mineralogy, yielding similar results between the two preparation methods (Supplementary Figure S1). This suggests that preparation in our study did not impact our mineralogy data.
Elemental Analysis
Sub-samples used for XRD analysis were then analyzed for trace elemental composition. Powders were cleaned following (Barker et al., 2003) including a clay removal step, an oxidative step and a final dilute acid leach in 0.001 N HCl. Samples were then dissolved in 1N HCl and analyzed for Ca concentration and trace elements (e.g., X/Ca ratios). Ca concentrations were analyzed using an ICP-AES ® Ultima 2 HORIBA and trace elements using a Thermo Scientific ® Element XR (HR-ICP-MS) at Ifremer (Plouzané, France). Samples were diluted to fixed calcium concentrations (30 ppm Ca) using 0.1 M HNO3 and 0.3 M HF matching multi-element standards Ca concentration to avoid any matrix effects (Misra et al., 2014; Guillermic et al., 2020). External reproducibility and analytical uncertainty were determined on the consistency standard Cam-Wuellestorfi (courtesy of the University of Cambridge) (Misra et al., 2014).
Amino Acid Content and Racemization
Proteins in ground skeleton powder were hydrolyzed to amino acids following methods of Penkman et al. (2008) with modifications. Briefly, ∼20–30 mg cleaned skeleton powder was dissolved in 2 M HCl for 1 h at room temperature (10 ml acid/mg powder). The sample was then dried under a stream of N2 (Ultra High Purity) and stored at -30°C until hydrolysis. Residues were hydrolyzed to amino acids in 7 M HCl (20 ml acid/mg starting material) at 110°C for 24 h, allowed to cool, then dried first under an N2 stream and then in a Speed Vac at room temperature. Samples terminated after the leaching phase were analyzed directly for free amino acids (FAA). Samples carried through to full hydrolysis in concentrated acid were analyzed for total hydrolyzable amino acids (THAA).
All samples were prepared in duplicate and analyzed at the Northern Arizona University Amino Acid Geochronology Laboratory using standard methods with modifications optimized for microfossils (Kaufman and Manley, 1998; Kaufman, 2000; Kaufman, 2003). Rehydrated samples were spiked with L-homo-arginine as an internal standard and then injected into an HPLC fitted with a reverse-phase C18-packed column. “Blank” samples were included. D and L enantiomers of Asx (aspartic acid plus asparagine), Glx (glutamic acid plus glutamine), Ser (serine), Ala (alanine), Val (valine), Phe (phenylalanine), Leu (leucine), and Ile (isoleucine) were quantified for each sample and we calculated the average relative proportion of each total amino acid (D plus L) within that pool.
Scanning Electron Microscopy
Thin sections 30 μm thick were prepared with ¼-μm diamond polishing. These were gold-coated and then analyzed on a Tescan Vega-3 SMU variable-pressure scanning electron microscope.
Statistics
Amino acid data were log-transformed and analyzed by principal component analysis in Past4.01 with 1,000 bootstrap replicates (Hammer et al., 2001). Wilcoxon rank sum tests and Kruskal-Wallis H tests of amino acid data were performed in R Studio (R Studio Team, 2019). Statistical comparisons for the trace elements between mineralogies were made using unpaired t-tests (GraphPad Prism version 7.03, GraphPad Software, San Diego, CA United States).
Results
Elemental Ratios
All samples except the rugosan, Zaphrenthis dalli, were calcium carbonate although some had recrystallized partially or fully to calcite (Table 1; Supplementary Figure S2). Z. dalli was almost completely silicified and was therefore not analyzed for elemental composition but was processed for amino acid analysis to represent an extreme outlier in terms of the effects of diagenesis on amino acid properties of hexacoral exoskeletons. We compared the B/Ca, Sr/Ca, and Mg/Ca values of all corals to the range known from modern corals (Figure 1, Supplementary Material, Supplementary Table S1). Samples that are >99% aragonite present B/Ca, Mg/Ca, Sr/Ca, and Ba/Ca in the range of modern corals (95% confidence interval), supporting that they remain primary aragonite. This was also observed in the Li/Ca and Mn/Ca compositions except for M. annularis 4 and O. limbata presenting higher Li/Ca and Mn/Ca. Samples partially recrystallized to calcite (between 7 and 50%) also fell in the range of modern corals for Mg/Ca, Sr/Ca, Li/Ca, and/Ba/Ca (95% confidence interval); this is also true for B/Ca except for Porites sp., S. conferta, and M. cavernosa 1. Samples completely recrystallized to calcite (>99%) present significantly lower B/Ca, Sr/Ca and higher Mg/Ca in comparison to primary aragonitic samples and modern corals.
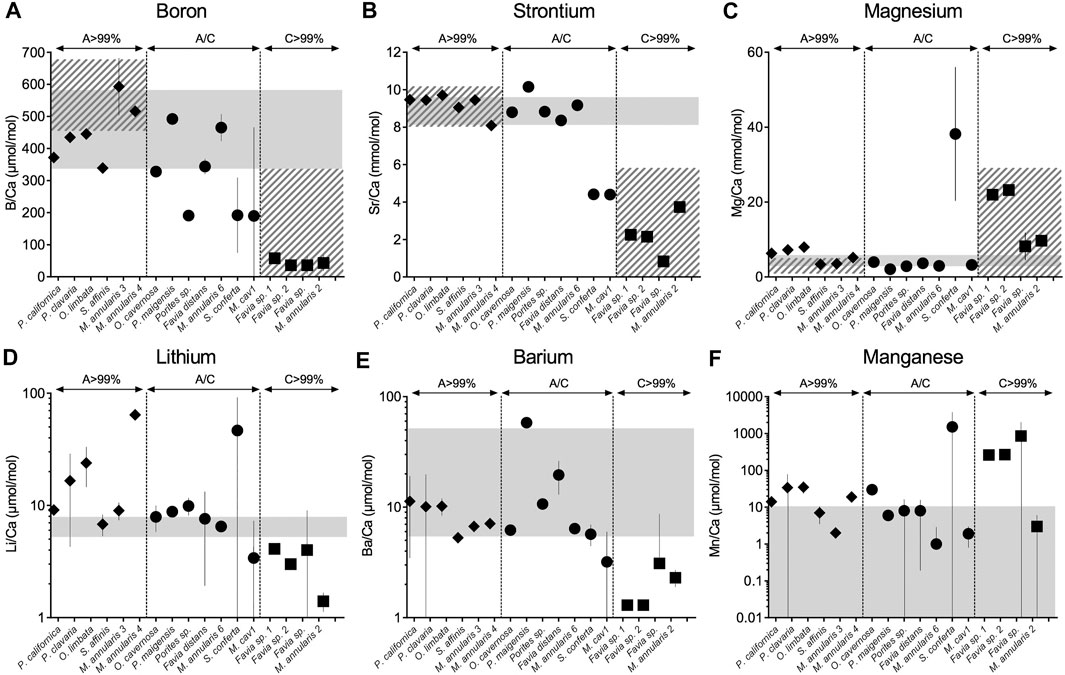
FIGURE 1. Boron (A), strontium (B), magnesium (C), lithium (D), barium (E), and manganese (F) relative abundance in fossil coral skeleton by individual specimen (A–C). Solid grey bars indicate the range of element/Ca ratios from modern corals (see Supplementary Material). Hatched grey bars represent the range of boron, strontium and magnesium in published fossil corals for aragonite (>99%) and calcite (>99%) (de Villiers et al., 1994; Quinn and Sampson, 2002; Swart et al., 2002; Allison et al., 2007; Hendy et al., 2007; Nothdurft and Webb, 2009; Griffiths et al., 2013; Hathorne et al., 2013; Gothmann et al., 2015). In line with previous studies, fully recrystallized skeletons from aragonite to calcite show disruption of their elemental compositions. Except for Porites sp. and S. conferta, mixed mineralogies (up to 50% aragonite/calcite), present boron, strontium and magnesium within the range of aragonitic modern samples, samples recrystallized to calcite (>99%) present lower B/Ca, lower Sr/Ca and higher Mg/Ca relative to modern corals (unpaired t-test, p < 0.001).
Amino Acids
Next we took a targeted approach to the amino acid data from all fossils, including Z. dalli, and some modern coral skeletons used in previous coral skeletal proteomics analyses (Drake et al., 2020b) using principal component analysis (PCA). We examined the amino acid relative composition (% THAA and FAA Asx, Glx), fraction of THAA that is FAA, total pmol amino acids per mass of skeleton, racemization (D/L THAA and D/L FAA Asx, Glx, and Ser) and THAA and FAA L-Ser/L-Asx across the samples. As expected, D/L THAA and FAA Asx were generally reflective of coral stratigraphic ages, with higher D/L ratios for most of the older corals. We observed a pattern in which 1) some specimens partially recrystallized to calcite clustered in the general direction of unmodified modern and fossil specimens from which SOM proteins have successfully been sequenced, and 2) several fully primary aragonite specimens that were not a part of this broad cluster despite exhibiting trace element composition within the range of modern aragonitic specimens (Figure 2; Supplementary Table S2). Further, we observed that all corals fully recrystallized to calcite except one, or silicified, showed completely unique amino acid characteristic patterns. Combined, PCs 1 and 2 explain 83% of the variance within the PCA. Going forward, we now classify each fossil specimen as “Good candidate-aragonite,” “Poor candidate-aragonite,” “Good candidate-other,” “Poor candidate-other,” or “Silicified,” and we separately group the modern specimens for which a skeletal proteome has been sequenced.
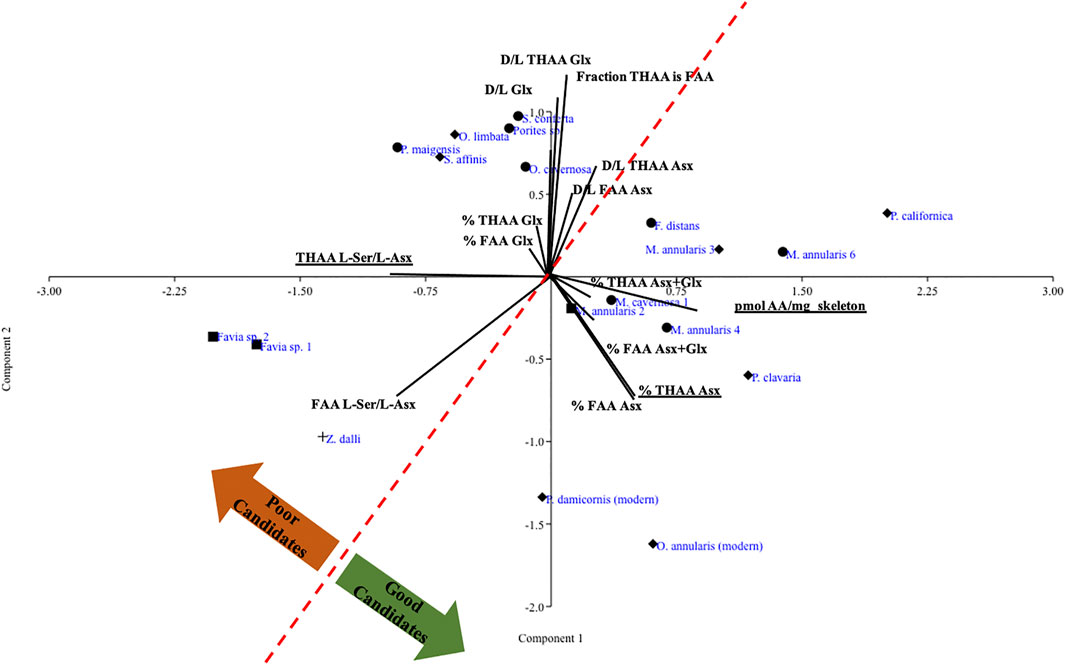
FIGURE 2. PCA of log transformed amino acid relative composition and racemization data for all fossil corals plus representative modern corals. Specimens that are >99% aragonite are noted by diamonds, specimens that are a blend of aragonite and calcite are noted by circles, and specimens fully recrystallized to calcite are noted by squares, following the same symbol coding as in Figure 1; the silicified specimen is noted by a plus sign.
To examine the driving patterns of these broad clusters, we specifically looked for differences between the corals according to mineralogy for % THAA Asx, THAA L-Ser/L-Asx, and total pmol peptide amino acids per mass of skeleton. For these three parameters, coral skeletal proteins are known to be biased toward the acidic residues although relative abundances of all amino acids vary across taxa (Young, 1971; Mass et al., 2012; Tomiak et al., 2016), these values may reflect contamination by modern amino acids (Kaufman, 2006), and a lower proportion of FAA in the THAA pool may reflect the maintenance of primary peptide sequence information, respectively. All fossil specimens considered to be “good candidates”, regardless of mineralogy, displayed similar % THAA Asx to modern specimens from which skeletal proteomes have been successfully sequenced, whereas “poor candidates” exhibited significantly lower % THAA Asx (p < 0.05; Figure 3A; Supplementary Table S3). Similarly, “good candidates” and modern specimens did not differ in their THAA L-Ser/L-Asx whereas “poor candidates” exhibited significantly higher values than aragonitic or mixed mineralogy “good candidates” (p < 0.05; Figure 3A; Supplementary Table S3). We also note a clear trend in pmol peptide-associated amino acids per mg skeleton to be higher in the “good candidates”, although our statistical power for this measure was not high enough to detect significant differences.
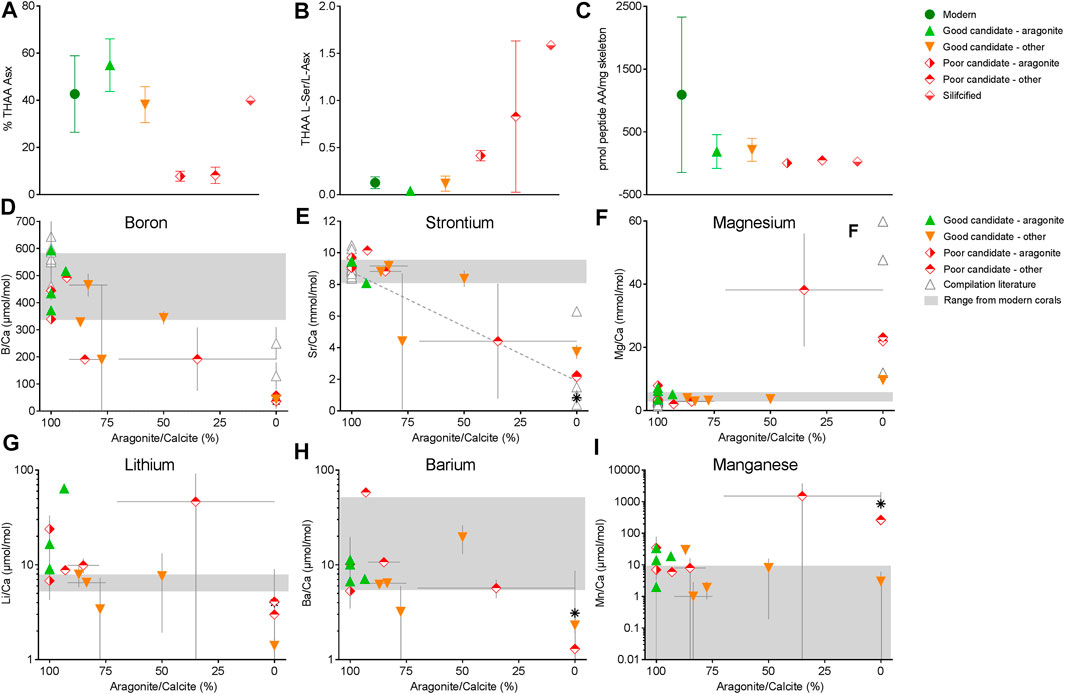
FIGURE 3. Amino acids % THAA Asx (A), THAA L-Ser/L-Asx (B), pmol peptide-AA/mg skeleton (C), boron (D), strontium (E), magnesium (F), lithium (G), barium (H), and manganese (I) abundance of fossil coral skeleton by % aragonite (D–F). In (D–F), Solid grey bars indicate the range of element/Ca ratios from modern corals (see Supplementary Material), (E) dotted line is the linear regression between Sr/Ca and % aragonite from McGregor and Gagan, (2003). With few exceptions, those results suggest that some recrystallized skeletons can be suitable for peptide analyses and that trace element data are useful indicators of potential good and poor candidates for organic matter analyses. In this study, most “poor candidates” showed disruption of elemental ratios compared to modern aragonitic samples (e.g., lower B/Ca, lower Sr/Ca and higher Mg/Ca relative to modern corals).
Discussion
Past paleoceanographic reconstruction studies using fossil corals have safely limited their specimen choices to those that contain <1% non-aragonite material (e.g., Allison et al., 2007; Gothmann et al., 2015). This is warranted as, for example, inclusion of 1% high-Mg calcite leads to a 1°C temperature reconstruction from geochemical data (Allison et al., 2007). However, based on data presented here, we propose that selecting corals with <50% recrystallization to calcite that have trace element ratios and amino acid composition in the range of modern coral skeletons will allow the expansion of specimens for biomineral organic matter analysis.
Mineral Preservation, Diagenesis and Elemental Composition
XRD data show clear evidence of diagenetic effects, with some samples partially or completely recrystallized to calcite, or in the case of Z. dalli, silicified. The elemental compositions of the 100% aragonite and 100% calcite samples are consistent with diagenetic effects and associated mineralogies; however, samples recrystallized up to 50% calcite present B/Ca, Sr/Ca and Mg/Ca signatures within the range of modern aragonitic corals and the 100% aragonite fossil samples (Figure 3). There appears to be a transition to more block-like crystal morphologies with increasing amounts of calcite (Supplementary Figure S3). Samples completely recrystallized to calcite present elemental composition outside the range of modern corals but in line with inorganic precipitation experiments and thermodynamic redistribution of the trace elements (McGregor and Gagan, 2003; Allison et al., 2007; Griffiths et al., 2013; Gothmann et al., 2015 among others).
The discrimination of an element substituting for Ca2+ in the crystal lattice is predicted by its size and the thermodynamic equilibrium of the reactions (Brand and Veizer, 1980; Menadakis et al., 2009). If this element is larger than Ca2+, it is predicted to be discriminated against in tighter rhombohedral calcite compared to orthorhombic aragonite. This is observed from inorganic precipitation experiments for Sr2+, Ba2+, Li+ (e.g., higher concentration in aragonite than calcite), and Mg2+ and Mn2+ (e.g., lower concentration in aragonite than calcite) (Shen et al., 1991; Gabitov et al., 2011; DeCarlo et al., 2015; Mavromatis et al., 2018; Gabitov et al., 2019). For boron, inorganic precipitations also present higher concentrations in aragonite than calcite (Mavromatis et al., 2015). Across studies examining diagenetic effects on fossil corals on geochemical patterns, subaerial diagenesis (e.g., dissolution and precipitation of secondary calcite from meteoric water) has been shown to cause a decrease in Sr/Ca and increase in Mg/Ca. Submarine diagenesis, including precipitation of secondary aragonite presents higher Sr/Ca (Sayani et al., 2011) but lower Mg/Ca, and/or precipitation of high-Mg calcite presents a decrease in Sr/Ca (Allison et al., 2007) and an increase in Mg/Ca, in line with inorganic precipitation.
Trace element composition of all samples fully recrystallized to calcite are in line with dominant subaerial diagenesis in an open system as we observe elemental ratios consistent with inorganic calcite precipitation (e.g., low Sr/Ca, Ba/Ca, B/Ca, Li/Ca, and high Mg/Ca). This suggests that all should be excluded from further analysis, but we do note that one fully recrystallized sample (M. annularis 2) is classified as a potential good candidate based on amino acid analysis. In contrast, partially recrystallized samples’ trace element and amino acid data suggest that some useful organic matter may remain as described below. Although we do not have the original geochemical signal of the fossil samples, some partially recrystallized to calcite (up to 50% for Favia distans) seem to retain the aragonitic signature based on the range of modern corals (Figures 1, 3). This can potentially be attributed to simultaneous dissolution/precipitation (e.g., closed system) from subaerial diagenesis which is thought to retain the primary signature (Sayani et al., 2011). Li/Ca and Mn/Ca ratios are more variable than the other elemental ratios explored in this study. Specifically, the >99% aragonite corals O. limbata and M. annularis 4 present Li/Ca and Mn/Ca ratios, potentially reflecting the presence of secondary aragonite precipitated from a reducing diagenetic fluid (Gothmann et al., 2015). In all cases, the disruptions of the Li/Ca and Mn/Ca ratios do not appear correlated with the preservation of the SOM, since O. limbata is considered a poor candidate and M. annularis 4 a good candidate for amino acid analyses. Further investigations of the isotopic composition (for example δ18O) would be useful to deconvolve the diagenesis patterns and their link with amino acid preservation.
Amino Acid Preservation and Degradation
Inorganic and organic alteration of biominerals, including both protein fragmentation and amino acid loss and increases in crystal size, respectively, occur simultaneously and continuously (Hare, 1980; Tuross et al., 1989). Retention of the organic phase implies that the inorganic component has remained stable, with organic molecules only available for microbial degradation and loss upon alteration of the inorganic phase (Collins et al., 2002). However, organic molecules can become altered within intact mineral, with higher rates observed with increased temperatures, increased absolute and varying amounts of water, marine vs. freshwater conditions, and presence of oxygen and/or sugars (Hare, 1962; Hare, 1974; 1980; Painter, 2003; McCobb et al., 2004), all of which are associated with aerial localization or microbial degradation of the specimen (McGregor and Gagan, 2003; Sayani et al., 2011). Specifically in invertebrates, alteration of the inorganic phase may also allow the intrusion of exogenous amino acids, particularly the abundant metabolic by-product Ser (review by (Mitterer, 1993). Four of seven fossil coral specimens studied here were partially (up to 50%) recrystallized to calcite, yet retained more Asx (THAA fraction) and peptide-associated amino acids and exhibited lower L-Ser/L-Asx (THAA fraction) than other calcite-recrystallized specimens and also fell within the 95% confidence interval of modern corals’ B/Ca, Sr/Ca and Mg/Ca (Figures 2, 3), suggesting that the recrystallization in these “good candidate” <99% aragonite specimens likely occurred quickly in a sub-aerial locale, minimizing both degradation and loss of proteinaceous matter and modification of trace element content. B/Ca, Sr/Ca and Mg/Ca analyses may therefore be useful in revealing a larger pool of potential fossil coral candidates including samples partially recrystallized to calcite for organic matter analyses. However, geochemical metrics alone do have limitations, with two of five samples that have fully aragonitic mineralogical and elemental signatures, plus two partially recrystallized samples with aragonitic trace element composition, displaying likely highly degraded proteins. This indicates that the processes that lead to geochemical alteration cannot be assumed to equally alter organic matter. Further, major mineral alterations such as silicification may lead to changes to amino acid content similar to conversion to >50% calcite, as exhibited by high L-Ser/L-Asx (THAA fraction) and low pmol amino acids per mass of skeleton of the 95% silicified rugosan coral, Z. dalli, collected from the Burlington formation which is lower to middle Mississippean in age (240–250 mya). There are numerous records of the genus Zaphrenthis from the Burlington (Bassler, 1950) Examinations of hypercalcifying non-scleractinian hexacorals such as rugose and tabulate corals, particularly of varying degrees of preservation, will clarify the degree of organic matter preservation in these important fossils as well.
Coral skeletons are not homogenous and the solubility of the different inorganic phases may impact mineral organic matter alteration. Rapid accretion deposits (RADs) display disorganized crystal orientations, presence of amorphous calcium carbonate, higher Sr/Ca and Mg/Ca, higher skeletal organic matter content, and larger defect sizes compared with thickening deposits (TDs) (e.g., Cuif and Dauphin, 1998; Meibom et al., 2006; Meibom et al., 2008; Brahmi et al., 2012; Rollion-Bard and Blamart, 2015; Mass et al., 2017), likely making them more soluble. Incorporation of specific highly acidic proteins such as the coral acid rich proteins 1 and coral acid rich protein 4, which are abundant in Asp, is also apparent in RADs (Mass et al., 2014). Therefore, coral skeletons exposed to environmental conditions that encourage inorganic phase alterations would likely display those changes preferentially in the RADs yielding localized element/Ca composition alteration, protein fragments and amino acids loss, loss of specific Asp-containing proteins in particular, and Ser intrusion. However, the altered trace element signal may not be detectable by the analysis of bulk skeleton conducted here, as the modern species-specific variation in trace elements between RADs and TDs falls within the modern intra-specific range in bulk values (Cuif and Dauphin, 1998; Figure 1). Hence, geochemical analyses may suggest that the bulk skeleton is unaltered or minimally altered (Figures 1, Figures 3D–F), whereas microstructural changes (not tested here) and consequent degradation and loss of organic matter may be observed (Figures 3A–C). Future studies that focus on the organic content of these microstructures will further reveal the effects of localized biomineral degradation on SOM preservation.
Conclusion
As the biological components of fossil bio-carbonates are increasingly used to reconstruct past ocean chemical conditions and ecological processes (Muscatine et al., 2005; Wang et al., 2015; Tornabene et al., 2017), it is important that biomolecules be in an optimal state of preservation. In the present study, combined XRD and elemental ratios were used to assess the preservation and bulk diagenesis of fossil coral samples. Percent THAA Asx, THAA L-Ser/L-Asx and the peptide AA/mg skeleton were then used to assess the potential “good candidates” for organic matter analyses in fossil corals presenting various degrees of recrystallisation. Our results support the exclusion of fully calcite-recrystallized and silicified samples in studies of fossil organic matter. However, some samples partially recrystallized to calcite (up to 50%) were characterized as potential “good candidates” for organic matter analyses.
The present work shows that geochemical analysis is a good first step toward detecting organic matter diagenesis of fossil biominerals. However, this alone does not capture the entire story, not ruling out fully primary aragonite specimens that appear to have experienced localized organic matter degradation and loss while rejecting partially recrystallized samples that appeared to retain useful biological information. We are not the first to advocate for a multidisciplinary approach when choosing biominerals that have gone through some degree of degradation and/or preservation (Tuross et al., 1989). We suggest that standard amino acid analysis, including the calculation of peptide-associated amino acid content from both THAA and FAA analyses, be added to geochemists’ and paleoceanographers’ methodological repertoire to carefully expand the pool of bio-carbonates used to study biologically-associated oceanographic processes.
Data Availability Statement
The original contributions presented in the study are included in the article/Supplementary Material, further inquiries can be directed to the corresponding authors.
Author Contributions
JD and MG designed the study, and collected and analyzed data. Geochemical method development and analyses were supported as part of projects led by RE. DJ provided guidance on interpreting stated fossil coral ages. All authors contributed to writing the manuscript.
Funding
JD was supported by an NSF Postdoctoral Research Fellowship in Biology award #1611943 and the Zuckerman STEM postdoctoral leadership program. MG and the trace element analysis was supported by IAGC (grant no. IAGC student grant 2017), a LabexMer (grant no. ANR-10-LABX-19-01 to Robert Eagle and Aradhna Tripati (UCLA and Institut Universitaire Européen de la Mer) and a DOE BES grant (grant no. DE-FG02-13ER16402) to Aradhna Tripati (UCLA). RE also acknowledges support from National Science Foundation Grant #1437166 and the Pritzker Endowment to UCLA.
Conflict of Interest
The authors declare that the research was conducted in the absence of any commercial or financial relationships that could be construed as a potential conflict of interest.
Acknowledgments
We thank Austin Hendy, Lindsay Walker, and Kathy Omura (Natural History Museum of Los Angeles County), Melanie Hopkins and Bushra Hussaini (American Museum of Natural History), Susan Butts and Jessica Utrup (Yale Peabody Museum), and Jessica Cundiff (Harvard Museum of Comparative Zoology) for access to coral skeleton samples held in museum collections; Lisa Greer (Washington and Lee University) for the modern Orbicella annularis specimen; Saeed Kahn (UCLA) for assistance with XRD; Céline Liorzou (IUEM, France) and Yoan Germain (Ifremer, France) for technical assistance; Jordon Bright, Katherine Whitacre, and Darrell Kaufman (Northern Arizona University Amino Acid Geochronology Laboratory) for amino acid analysis; the SIMS facility at UCLA for assistance with scanning electron microscopy; and Claire Divola (UCLA) for assistance with data management. We thank the reviewers for their helpful comments.
Supplementary Material
The Supplementary Material for this article can be found online at: https://www.frontiersin.org/articles/10.3389/feart.2021.643864/full#supplementary-material
References
Allison, N., Finch, A. A., Webster, J. M., and Clague, D. A. (2007). Palaeoenvironmental Records from Fossil Corals: the Effects of Submarine Diagenesis on Temperature and Climate Estimates. Geochimica et Cosmochimica Acta. 71, 4693–4703. doi:10.1016/j.gca.2007.07.026
Bada, J. L. (1985). Amino Acid Racemization Dating of Fossil Bones. Annu. Rev. Earth Planet. Sci. 13, 241–268. doi:10.1146/annurev.ea.13.050185.001325
Bada, J. L., Wang, X. S., and Hamilton, H. (1999). Preservation of Key Biomolecules in the Fossil Record: Current Knowledge and Future Challenges. Phil. Trans. R. Soc. Lond. B. 354, 77–87. doi:10.1098/rstb.1999.0361
Bai, L., Sheeley, S., and Sweedler, J. V. (2009). Analysis of Endogenous D-Amino Acid-Containing Peptides in Metazoa. Bioanal. Rev. 1, 7–24. doi:10.1007/s12566-009-0001-2
Barker, S., Greaves, M., and Elderfield, H. (2003). A Study of Cleaning Procedures Used for Foraminiferal Mg/Ca Paleothermometry. Geochem. Geophys. Geosystems. 4, 8407. doi:10.1029/2003gc000559
Bassler, R. S. (1950). Faunal Lists and Descriptions of Paleozoic Corals. Boulder, Colorado: Geological Society of America.
Brahmi, C., Kopp, C., Domart-Coulon, I., Stolarski, J., and Meibom, A. (2012). Skeletal Growth Dynamics Linked to Trace-Element Composition in the Scleractinian Coral Pocillopora Damicornis. Geochimica et Cosmochimica Acta. 99, 146–158. doi:10.1016/j.gca.2012.09.031
Brand, U., and Veizer, J. (1980). Chemical Diagenesis of a Multicomponent Carbonate System; 1, Trace Elements. J. Sediment. Res. 50, 1219–1236. doi:10.1306/212F7BB7-2B24-11D7-8648000102C1865D
Chung, F. H. (1974). Quantitative Interpretation of X-ray Diffraction Patterns of Mixtures. I. Matrix-flushing Method for Quantitative Multicomponent Analysis. J. Appl. Cryst. 7, 519–525. doi:10.1107/s0021889874010375
Cohen, A. L., and McConnaughey, T. A. (2003). “6. Geochemical Perspectives on Coral Mineralization,” in Biomineralization. Editors P.M. Dove, S. Weiner, and J. Deyoreo, 151–188. doi:10.1515/9781501509346-011
Collins, M. J., Nielsen-Marsh, C. M., Hiller, J., Smith, C. I., Roberts, J. P., Prigodich, R. V., et al. (2002). The Survival of Organic Matter in Bone: a Review. Archaeometry. 44, 383–394. doi:10.1111/1475-4754.t01-1-00071
Cuif, J.-P., and Dauphin, Y. (1998). Microstructural and Physico-Chemical Characterization of ‘centers of Calcification' in Septa of Some Recent Scleractinian Corals. Paläontol. Z. 72, 257–269. doi:10.1007/bf02988357
de Villiers, S., Shen, G. T., and Nelson, B. K. (1994). The -temperature Relationship in Coralline Aragonite: Influence of Variability in and Skeletal Growth Parameters. Geochimica et Cosmochimica Acta 58, 197–208. doi:10.1016/0016-7037(94)90457-x
DeCarlo, T. M., Gaetani, G. A., Holcomb, M., and Cohen, A. L. (2015). Experimental Determination of Factors Controlling U/Ca of Aragonite Precipitated from Seawater: Implications for Interpreting Coral Skeleton. Geochimica et Cosmochimica Acta. 162, 151–165. doi:10.1016/j.gca.2015.04.016
Demarchi, B. (2020). Amino Acids and Proteins in Fossil Biominerals: An Introduction for Archaeologists and Palaeontologists. New Jersey, United States: John Wiley & Sons. doi:10.1002/9781119089537
Drake, J. L., Mass, T., Stolarski, J., Von Euw, S., Schootbrugge, B., and Falkowski, P. G. (2020a). How Corals Made Rocks through the Ages. Glob. Change Biol. 26, 31–53. doi:10.1111/gcb.14912
Drake, J. L., Whitelegge, J. P., and Jacobs, D. K. (2020b). First Sequencing of Ancient Coral Skeletal Proteins. Scientific Rep. 10, 1–11. doi:10.1038/s41598-020-75846-4
Foster, L., Andersson, C., Høie, H., Allison, N., Finch, A., and Johansen, T. (2008). Effects of Micromilling on δ18O in Biogenic Aragonite. Geochem. Geophys. Geosystems. 9. doi:10.1029/2007gc001911
Gabitov, R., Schmitt, A., Rosner, M., McKeegan, K., Gaetani, G. A., Cohen, A. L., et al. (2011). In Situ δ7Li, Li/Ca, and Mg/Ca Analyses of Synthetic Aragonites. Geochem. Geophys. Geosystems 12. doi:10.1029/2010gc003322
Gabitov, R., Sadekov, A., Yapaskurt, V., Borrelli, C., Bychkov, A., Sabourin, K., et al. (2019). Elemental Uptake by Calcite Slowly Grown from Seawater Solution: an Iin-Ssitu Study via Depth Profiling. Front. Earth Sci. 7, 51. doi:10.3389/feart.2019.00051
Genchi, G. (2017). An Overview on D-Amino Acids. Amino Acids. 49, 1521–1533. doi:10.1007/s00726-017-2459-5
Gill, I., Olson, J. J., and Hubbard, D. K. (1995). Corals, Paleotemperature Records, and the Aragonite-Calcite Transformation. Geol. 23, 333–336. doi:10.1130/0091-7613(1995)023<0333:cprata>2.3.co;2
Gothmann, A. M., Stolarski, J., Adkins, J. F., Schoene, B., Dennis, K. J., Schrag, D. P., et al. (2015). Fossil Corals as an Archive of Secular Variations in Seawater Chemistry since the Mesozoic. Geochimica et Cosmochimica Acta. 160, 188–208. doi:10.1016/j.gca.2015.03.018
Griffiths, N., Müller, W., Johnson, K. G., and Aguilera, O. A. (2013). Evaluation of the Effect of Diagenetic Cements on element/Ca Ratios in Aragonitic Early Miocene (∼16Ma) Caribbean Corals: Implications for ‘deep-Time' Palaeo-Environmental Reconstructions. Palaeogeogr. Palaeoclimatol. Palaeoecol. 369, 185–200. doi:10.1016/j.palaeo.2012.10.018
Guillermic, M., Misra, S., Eagle, R., Villa, A., Chang, F., and Tripati, A. (2020). Seawater pH Reconstruction Using boron Isotopes in Multiple Planktonic Foraminifera Species with Different Depth Habitats and Their Potential to Constrain pH and pCO2 Gradients. Biogeosciences. 17, 3487–3510. doi:10.5194/bg-17-3487-2020
Hammer, Ø., Harper, D. A., and Ryan, P. D. (2001). PAST: Paleontological Statistics Software Package for Education and Data Analysis. Palaeontol. electronica. 4, 9. Available at: http://palaeo-electronica.org/2001_1/past/issue1_01.htm
Hare, P. (1974). Amino Acid Dating of Bone—The Influence of Water. Washington, DC: Carnegie Institute of Washington Year Book, 73. 576–581.
Hare, P. (1980). “Organic Geochemistry of Bone and its Relation to the Survival of Bone in the Natural Environment,” in Fossils in the Making: Vertebrate Taphonomy and Paleoecology. Editors AK Behrensmeyer, and AP Hill (Chicago: University of Chicago Press).
Hare, P. E. (1962). The Amino Acid Composition of the Organic Matrix of Some Recent and Fossil Shells of Some West Coast Species of Mytilus. Dissertation: California Institute of Technology
Hathorne, E. C., Gagnon, A., Felis, T., Adkins, J., Asami, R., Boer, W., et al. (2013). Interlaboratory Study for Coral Sr/Ca and Other element/Ca Ratio Measurements. Geochem. Geophys. Geosyst. 14, 3730–3750. doi:10.1002/ggge.20230
Hendy, A., Walker, L., and Mertz, W. (2020). LACM Invertebrate Paleontology. California: Natural History Museum of Los Angeles County.
Hendy, E., Gagan, M., Lough, J., McCulloch, M., and DeMenocal, P. (2007). Impact of Skeletal Dissolution and Secondary Aragonite on Trace Element and Isotopic Climate Proxies in Porites Corals. Paleoceanography. 22. doi:10.1029/2007pa001462
Kaufman, D. S. (2000). 12. Amino Acid Racemization in Ostracodes. Perspectives in Amino Acid and Protein Geochemistry, 145.
Kaufman, D. (2003). Dating Deep-lake Sediments by Using Amino Acid Racemization in Fossil Ostracodes. Geol. 31, 1049–1052. doi:10.1130/g20004.1
Kaufman, D. (2006). Temperature Sensitivity of Aspartic and Glutamic Acid Racemization in the Foraminifera Pulleniatina. Quat. Geochronol. 1, 188–207. doi:10.1016/j.quageo.2006.06.008
Kaufman, D. S., and Manley, W. F. (1998). A New Procedure for Determining DL Amino Acid Ratios in Fossils Using Reverse Phase Liquid Chromatography. Quat. Sci. Rev. 17, 987–1000. doi:10.1016/s0277-3791(97)00086-3
Mass, T., Drake, J. L., Haramaty, L., Rosenthal, Y., Schofield, O. M. E., Sherrell, R. M., et al. (2012). Aragonite Precipitation by “Proto-polyps” in Coral Cell Cultures. PLoS ONE. 7, e35049. doi:10.1371/journal.pone.0035049
Mass, T., Drake, J. L., Peters, E. C., Jiang, W., and Falkowski, P. G. (2014). Immunolocalization of Skeletal Matrix Proteins in Tissue and mineral of the Coral Stylophora Pistillata. Proc. Natl. Acad. Sci. 111, 12728–12733. doi:10.1073/pnas.1408621111
Mass, T., Giuffre, A. J., Sun, C.-Y., Stifler, C. A., Frazier, M. J., Neder, M., et al. (2017). Amorphous Calcium Carbonate Particles Form Coral Skeletons. Proc. Natl. Acad. Sci. USA. 114, E7670–E7678. doi:10.1073/pnas.1707890114
Mavromatis, V., Goetschl, K. E., Grengg, C., Konrad, F., Purgstaller, B., and Dietzel, M. (2018). Barium Partitioning in Calcite and Aragonite as a Function of Growth Rate. Geochimica et Cosmochimica Acta. 237, 65–78. doi:10.1016/j.gca.2018.06.018
Mavromatis, V., Montouillout, V., Noireaux, J., Gaillardet, J., and Schott, J. (2015). Characterization of boron Incorporation and Speciation in Calcite and Aragonite from Co-precipitation Experiments under Controlled pH, Temperature and Precipitation Rate. Geochimica et Cosmochimica Acta. 150, 299–313. doi:10.1016/j.gca.2014.10.024
McCobb, L. M. E., Briggs, D. E. G., Hall, A. R., and Kenward, H. K. (2004). The Preservation of Invertebrates in 16th-Century Cesspits at St Saviourgate, York. Archaeometry. 46, 157–169. doi:10.1111/j.1475-4754.2004.00150.x
McGregor, H. V., and Gagan, M. K. (2003). Diagenesis and Geochemistry of Porites Corals from Papua New Guinea. Geochimica et Cosmochimica Acta. 67, 2147–2156. doi:10.1016/s0016-7037(02)01050-5
Meibom, A., Cuif, J.-P., Houlbreque, F., Mostefaoui, S., Dauphin, Y., Meibom, K. L., et al. (2008). Compositional Variations at Ultra-structure Length Scales in Coral Skeleton. Geochimica et Cosmochimica Acta. 72, 1555–1569. doi:10.1016/j.gca.2008.01.009
Meibom, A., Yurimoto, H., Cuif, J. P., Domart‐Coulon, I., Houlbreque, F., Constantz, B., et al. (2006). Vital Effects in Coral Skeletal Composition Display Strict Three‐dimensional Control. Geophys. Res. Lett. 33. doi:10.1029/2006gl025968
Menadakis, M., Maroulis, G., and Koutsoukos, P. G. (2009). Incorporation of Mg2+, Sr2+, Ba2+ and Zn2+ into Aragonite and Comparison with Calcite. J. Math. Chem. 46, 484–491. doi:10.1007/s10910-008-9490-4
Misra, S., Greaves, M., Owen, R., Kerr, J., Elmore, A. C., and Elderfield, H. (2014). Determination of B/Ca of Natural Carbonates by HR-ICP-MS. Geochem. Geophys. Geosyst. 15, 1617–1628. doi:10.1002/2013gc005049
Mitterer, R. M. (1993). The Diagenesis of Proteins and Amino Acids in Fossil Shells. Organic Geochemistry. Berlin, Germany: Springer, 739–753. doi:10.1007/978-1-4615-2890-6_35
Muhs, D. R., Simmons, K. R., Schumann, R. R., and Halley, R. B. (2011). Sea-level History of the Past Two Interglacial Periods: New Evidence from U-Series Dating of Reef Corals from South Florida. Quat. Sci. Rev. 30, 570–590. doi:10.1016/j.quascirev.2010.12.019
Multer, H. G., Gischler, E., Lundberg, J., Simmons, K. R., and Shinn, E. A. (2002). Key Largo limestone Revisited: Pleistocene Shelf-Edge Facies, Florida Keys, USA. Facies. 46, 229–271. doi:10.1007/bf02668083
Muscatine, L., Goiran, C., Land, L., Jaubert, J., Cuif, J.-P., and Allemand, D. (2005). Stable Isotopes (13C and 15N) of Organic Matrix from Coral Skeleton. Proc. Natl. Acad. Sci. 102, 1525–1530. doi:10.1073/pnas.0408921102
Neuberger, A. (1948). Stereochemistry of Amino Acids. Advances in Protein Chemistry. Amsterdam, Netherlands: Elsevier, 297–383. doi:10.1016/s0065-3233(08)60009-1
Nothdurft, L. D., Webb, G. E., Bostrom, T., and Rintoul, L. (2007). Calcite-filled Borings in the Most Recently Deposited Skeleton in Live-Collected Porites (Scleractinia): Implications for Trace Element Archives. Geochimica et Cosmochimica Acta. 71, 5423–5438. doi:10.1016/j.gca.2007.09.025
Nothdurft, L. D., and Webb, G. E. (2009). Earliest Diagenesis in Scleractinian Coral Skeletons: Implications for Palaeoclimate-Sensitive Geochemical Archives. Facies. 55, 161–201. doi:10.1007/s10347-008-0167-z
Painter, T. J. (2003). “Sphagnum-dominated Peat Bogs,” in Paleobiology II. Editors D.E.G. Briggs, and P.R. Crowther (Malden, MA: Blackwell Science), 321–325.
Peled, Y., Drake, J. L., Malik, A., Almuly, R., Lalzar, M., Morgenstern, D., et al. (2020). Optimization of Skeletal Protein Preparation for LC–MS/MS Sequencing Yields Additional Coral Skeletal Proteins in Stylophora Pistillata. BMC Mater. 2, 8. doi:10.1186/s42833-020-00014-x
Penkman, K. E. H., Kaufman, D. S., Maddy, D., and Collins, M. J. (2008). Closed-system Behaviour of the Intra-crystalline Fraction of Amino Acids in Mollusc Shells. Quat. Geochronol. 3, 2–25. doi:10.1016/j.quageo.2007.07.001
Puverel, S., Tambutt, E., Zoccola, D., Domart-Coulon, I., Bouchot, A., Lotto, S. v., et al. (2005). Antibodies against the Organic Matrix in Scleractinians: A New Tool to Study Coral Biomineralization. Coral Reefs 24, 149–156. doi:10.1007/s00338-004-0456-0
Quinn, T. M., and Sampson, D. E. (2002). A Multiproxy Approach to Reconstructing Sea Surface Conditions Using Coral Skeleton Geochemistry. Paleoceanography. 17, 1–14. doi:10.1029/2000pa000528
Rabier, C., Anguy, Y., Cabioch, G., and Genthon, P. (2008). Characterization of Various Stages of Calcitization in Porites Sp Corals from Uplifted Reefs - Case Studies from New Caledonia, Vanuatu, and Futuna (South-West Pacific). Sediment. Geology. 211, 73–86. doi:10.1016/j.sedgeo.2008.08.005
Rollion-Bard, C., and Blamart, D. (2015). Possible Controls on Li, Na, and Mg Incorporation into Aragonite Coral Skeletons. Chem. Geology. 396, 98–111. doi:10.1016/j.chemgeo.2014.12.011
Sakalauskaite, J., Andersen, S. H., Biagi, P., Borrello, M. A., Cocquerez, T., Colonese, A. C., et al. (2019). Palaeoshellomics’ Reveals the Use of Freshwater Mother-Of-Pearl in Prehistory. eLife. 8, e45644. doi:10.7554/elife.45644
Sakalauskaite, J., Marin, F., Pergolizzi, B., and Demarchi, B. (2020). Shell Palaeoproteomics: First Application of Peptide Mass Fingerprinting for the Rapid Identification of Mollusc Shells in Archaeology. J. Proteomics. 227, 103920. doi:10.1016/j.jprot.2020.103920
Sayani, H. R., Cobb, K. M., Cohen, A. L., Elliott, W. C., Nurhati, I. S., Dunbar, R. B., et al. (2011). Effects of Diagenesis on Paleoclimate Reconstructions from Modern and Young Fossil Corals. Geochimica et Cosmochimica Acta. 75, 6361–6373. doi:10.1016/j.gca.2011.08.026
Schroeter, E. R., DeHart, C. J., Cleland, T. P., Zheng, W., Thomas, P. M., Kelleher, N. L., et al. (2017). Expansion for the Brachylophosaurus canadensis Collagen I Sequence and Additional Evidence of the Preservation of Cretaceous Protein. J. Proteome Res. 16, 920–932. doi:10.1021/acs.jproteome.6b00873
Shen, G. T., Campbell, T. M., Dunbar, R. B., Wellington, G. M., Colgan, M. W., and Glynn, P. W. (1991). Paleochemistry of Manganese in Corals from the Galapagos Islands. Coral Reefs. 10, 91–100. doi:10.1007/bf00571827
Stoll, H. M., Ruiz Encinar, J., Ignacio Garcia Alonso, J., Rosenthal, Y., Probert, I., and Klaas, C. (2001). A First Look at Paleotemperature Prospects from Mg in Coccolith Carbonate: Cleaning Techniques and Culture Measurements. Geochem. Geophys. Geosystems. 2, (5). doi:10.1029/2000gc000144
Swart, P. K., Elderfield, H., and Greaves, M. J. (2002). A High-Resolution Calibration of Sr/Ca Thermometry Using the Caribbean coralMontastraea Annularis. Geochem.-Geophys.-Geosyst. 3, 1–11. doi:10.1029/2002gc000306
Tomiak, P. J., Andersen, M. B., Hendy, E. J., Potter, E. K., Johnson, K. G., and Penkman, K. E. H. (2016). The Role of Skeletal Micro-architecture in Diagenesis and Dating of Acropora Palmata. Geochimica et Cosmochimica Acta. 183, 153–175. doi:10.1016/j.gca.2016.03.030
Tomiak, P. J., Penkman, K. E. H., Hendy, E. J., Demarchi, B., Murrells, S., Davis, S. A., et al. (2013). Testing the Limitations of Artificial Protein Degradation Kinetics Using Known-Age Massive Porites Coral Skeletons. Quat. Geochronol. 16, 87–109. doi:10.1016/j.quageo.2012.07.001
Tornabene, C., Martindale, R. C., Wang, X. T., and Schaller, M. F. (2017). Detecting Photosymbiosis in Fossil Scleractinian Corals. Scientific Rep. 7, 9465. doi:10.1038/s41598-017-09008-4
Tuross, N., Behrensmeyer, A. K., Eanes, E. D., Fisher, L. W., and Hare, P. E. (1989). Molecular Preservation and Crystallographic Alterations in a Weathering Sequence of Wildebeest Bones. Appl. Geochem. 4, 261–270. doi:10.1016/0883-2927(89)90027-9
Wainwright, S. A. (1963). Skeletal Organization in the Coral, Pocillopora Damicornis. Q. J. Microscopical Sci. s3-104, 169–183. doi:10.1242/jcs.s3-104.66.169
Waite, A. J., and Swart, P. K. (2015). The Inversion of Aragonite to Calcite during the Sampling of Skeletal Archives: Implications for Proxy Interpretation. Rapid Commun. Mass. Spectrom. 29, 955–964. doi:10.1002/rcm.7180
Wang, X. T., Sigman, D. M., Cohen, A. L., Sinclair, D. J., Sherrell, R. M., Weigand, M. A., et al. (2015). Isotopic Composition of Skeleton-Bound Organic Nitrogen in Reef-Building Symbiotic Corals: a New Method and Proxy Evaluation at Bermuda. Geochimica et Cosmochimica Acta. 148, 179–190. doi:10.1016/j.gca.2014.09.017
Watanabe, T., Winter, A., and Oba, T. (2001). Seasonal Changes in Sea Surface Temperature and Salinity during the Little Ice Age in the Caribbean Sea Deduced from Mg/Ca and 18O/16O Ratios in Corals. Mar. Geology. 173, 21–35. doi:10.1016/s0025-3227(00)00166-3
Young, S. D. (1971). Organic Material from Scleractinian Coral Skeletons-I. Variation in Composition between Several Species. Comp. Biochem. Physiol. B: Comp. Biochem. 40, 113–120. doi:10.1016/0305-0491(71)90067-8
Keywords: amino acids, trace elements, X-ray diffraction, mineralogy, scleractinians
Citation: Drake JL, Guillermic M, Eagle RA and Jacobs DK (2021) Fossil Corals With Various Degrees of Preservation Can Retain Information About Biomineralization-Related Organic Material. Front. Earth Sci. 9:643864. doi: 10.3389/feart.2021.643864
Received: 19 December 2020; Accepted: 10 June 2021;
Published: 23 June 2021.
Edited by:
Claire Rollion-Bard, UMR7154 Institut de Physique du Globe de Paris (IPGP), FranceReviewed by:
Frederic Marin, Délégation Centre-Est (CNRS), FranceSergio Rodríguez, Universidad Complutense de Madrid, Spain
Copyright © 2021 Drake, Guillermic, Eagle and Jacobs. This is an open-access article distributed under the terms of the Creative Commons Attribution License (CC BY). The use, distribution or reproduction in other forums is permitted, provided the original author(s) and the copyright owner(s) are credited and that the original publication in this journal is cited, in accordance with accepted academic practice. No use, distribution or reproduction is permitted which does not comply with these terms.
*Correspondence: Jeana L. Drake, amVhbmFkcmFrZUBnLnVjbGEuZWR1; David K. Jacobs, ZGphY29ic0BnLnVjbGEuZWR1
†These authors have contributed equally to this work and share first authorship