- 1The Dinosaur Institute, Natural History Museum of Los Angeles County, Los Angeles, CA, United States
- 2Department of Biological Sciences, California Polytechnic State University, Pomona, CA, United States
- 3Unidad de Paleontología, Departamento de Biología, Universidad Autónoma de Madrid, Madrid, Spain
- 4Department of Earth Sciences, University of Oxford, Oxford, United Kingdom
Birds are one of the most diverse clades of extant terrestrial vertebrates, a diversity that first arose during the Mesozoic as a multitude of lineages of pre-neornithine (stem) birds appeared but did not survive into the Cenozoic Era. Modern birds (Neornithes) inhabit an extensive array of ecologically distinct habitats and have specific and varied foraging strategies. Likewise, the morphological disparity among Mesozoic lineages appears to underscore a significant degree of ecological diversity, yet attempts to determine lineage-specific ecologies have mainly been limited to superficial narratives. In recent years, numerous studies have used various morphometric proxies to interpret the paleoecology of Mesozoic bird lineages, but largely without evaluating the interplay between ecological and phylogenetic signals. Moreover, most studies of this sort transform the original data into logarithms to control dimensionality, underestimating the biases induced upon such transformations. The goal of this study is to quantitatively address the ecomorphology of crown-group Neornithes using a dense sample of raw forelimb and hindlimb measurements, and to examine if such results can be used to infer the ecologies of Mesozoic bird lineages. To that end, scaling of limb measurements and ecological data from modern birds was assessed statistically using phylogenetic comparative methods, followed by the inclusion of fossil taxa. A strong relationship was recovered between humerus and hindlimb allometric scaling and phylogeny. Our results indicate that while some ecological classes of modern birds can be discriminated from each other, phylogenetic signature can overwhelm ecological signal in morphometric data, potentially limiting the inferences that can be made from ecomorphological studies. Furthermore, we found differential scaling of leg bones among Early Cretaceous enantiornithines and ornithuromorphs, a result hinting that habitat partitioning among different lineages could be a pervasive phenomenon in avian evolution.
Introduction
Modern birds comprise one of the most diverse clades of vertebrates alive today, yet members of this crown group, the Neornithes, are poorly represented in the fossil record before the Tertiary (Fountaine et al., 2005; Brocklehurst et al., 2012; Field et al., 2020). Instead, a wealth of pre-Tertiary fossils reveals the existence of numerous Mesozoic lineages outside of the crown clade Neornithes (Brusatte et al., 2015; Mayr 2016; Wang and Zhou 2017; Chiappe 2018; Chiappe and Bell 2020). These stem lineages represent an enormous diversity of forms, from the long-legged, cursorial Hollanda (Bell et al., 2010) to the long-winged, soaring Sapeornis (Serrano and Chiappe 2017), and from the small, flighted enantiornithines (O’Connor and Chiappe 2011; Liu et al., 2017) to the large, flightless hesperornithiforms (Bell and Chiappe 2016). This diversity in form has hinted at the possibility that collectively these stems birds could have approached the spectacular ecological diversity we see today in modern birds (Chiappe 2018).
Evidence for this impressive ecological diversity comes from a range of sources, such as specimens that preserve gut contents, which provide first-hand evidence of what some of these birds ate, yielding direction information regarding their lifestyles. Additionally, a number of pre-modern birds exhibit specific morphological features that can be interpreted as indicators of a particular lifestyle. A classic example of this sort of ecomorphological inference pertains to the hesperornithiforms, whose many skeletal similarities to modern loons and grebes have long led to interpretations of an aquatic, foot-propelled diving lifestyle (i.e., Marsh 1880; Bell et al., 2019). Aside from the hesperornithiforms, which were flightless, recent studies have revealed that stem birds evolved most flight modes (e.g., soaring, flap and glide, bounding, and continuous flapping) typical of modern birds (Serrano and Chiappe 2017; Serrano et al., 2017). Finally, because the relationship between morphological traits and ecological characteristics is not always straightforward, quantitative analysis of comparative datasets for modern and fossil birds is a promising avenue of inquiry.
As our understanding of Mesozoic avian diversity has grown through the study of individual taxa, some generalizations have been proposed as well. One study of the Early Cretaceous Jehol Biota found ecological diversity was low in comparison to taxonomic diversity, (Mitchell and Makovicky 2014). The study in question, however, focused exclusively on the Jehol Biota and used a very limited number of taxa from that assemblage. A number of studies have posited a trophic bias among Early Cretaceous birds, with gut contents, facial morphology, tooth patterns and form, and other indicators of diet or ecological niche suggesting that many enantiornithines from this time period may have had an arboreal lifestyle and an insectivorous diet (although there are notable counterexamples among the longipterygids; see O’Connor et al., 2011), while ornithuromorphs are more commonly interpreted as land-dwellers or amphibious birds that ate fish or probed the substrate in search of crustaceans and worms (Zhou 2006; Wang et al., 2013; Field et al., 2018; O’Connor 2019; Wang et al., 2020). These generalizations, however, are far from certain, and to date quantitative data has not been used to test these observations.
This study will first summarize the literature that has explored ecological diversity in Mesozoic birds and then present a new ecomorphological analysis of the largest database of modern and fossil birds collected to date. The results from these avenues of research demonstrate the enormous diversity of pre-modern birds, as well as a number of difficulties in reconstructing an animal’s ecology from fossil remains, including important ways that data transformations alter results and can obscure underlying scaling relationships.
Previous Analyses of Mesozoic Avian Ecology
The past twenty years has seen a wealth of literature aimed at better understanding the ecology of Mesozoic birds. Studies have varied from qualitative or quantitative ecological assessments of specific taxa (i.e., You et al., 2006; Chiappe et al., 2007; Bell et al., 2010; Hu et al., 2015) to quantitative analyses of large datasets of modern and Mesozoic birds as well as non-avian theropods (i.e., Gatesy and Middleton 1997; Hopson 2001; Nudds et al., 2004; Bell and Chiappe 2011; Falk et al., 2020). Quantitative analyses that include Mesozoic birds have explored the use of a wide variety of data as proxies or indicators of ecological niche occupation: cortical bone thickness (Habib and Ruff 2008), furcula shape (Close and Rayfield 2012), curvature of the pedal ungual phalanges (Pike and Maitland 2004; Glen and Bennett 2007; Cobb and Sellers 2020), and various measurements of the forelimb and/or hindlimb (i.e., Nudds et al., 2004; Bell and Chiappe 2011; Wang et al., 2011; Falk et al., 2020). These studies have built on a substantial body of ecomorphological work on modern taxa (see reviews by Bock 1994; Tobias et al., 2020), particularly modern birds (e.g., Barbosa 1993; Hertel 1995; Zeffer 2002; Felice et al., 2019; Navalón et al., 2019; Sheard et al., 2020).
Ecological Inferences for Individual Taxa
A number of studies have used specific morphological features to infer the ecological habits of individual Mesozoic birds or clades. For instance, the inferred aquatic lifestyle of hesperornithiforms is based on a number of osteological features, such as the expanded fourth trochlea of the tarsometatarsus, extreme reduction of the forelimb, and expansion of the tibiotarsal cnemial crests and patella (Bell et al., 2019). Another classic example is the inference that enantiornithines occupied a wide variety of ecological niches, which rests on specific morphological features such as anisodactyly (indicating perching and arboreality) in Sinornis, Concornis, and Neuquenornis, and wading adaptations in the pedal morphology of Lectavis and Yungavolucis (Chiappe and Walker 2002).
More recently, the presence of expanded cnemial crests on the tibiotarsus, proximal displacement of metatarsal II, extremely elongate toes, proximal phalanges that are more elongate than the distal phalanges, unrecurved unguals with large flexor tubercles, and potentially webbed feet were all identified as morphological features indicative of an aquatic habitat in the ornithurine Gansus (You et al., 2006). Similarly, the long leg bones of Hongshanornis and Jianchangornis were used without formal analysis to propose an aquatic lifestyle (Hongshanornis;Zhou and Zhang 2005) and a terrestrial lifestyle (Jianchangornis; Zhou et al., 2009) lifestyle for these taxa. Another example of an ecological inference for a specific taxon is the flightless, terrestrial lifestyle posited for Patagopteryx, based on extreme reduction of the wing (Alvarenga and Bonaparte 1992; Chiappe 2002). Additionally, an extremely short and broad pygostyle, unique among Mesozoic birds but seen in modern woodpeckers, was used to infer a scansorial lifestyle for Parapengornis (Hu et al., 2015).
Moving beyond osteology, the growing record of Cretaceous avian ichnofossils has provided evidence for ecological niche diversification. Sites in the Korean peninsula have revealed feeding traces (probing and others) similar to those left by mud probers and spoonbills (Kim et al., 2012). Likewise, a variety of tracks from these sites and others have been interpreted as belonging to web-footed (e.g., Lim et al., 2000) and terrestrial or climbing (e.g., Lockley et al., 2007) birds. Today, a global record of fossil traces (Lockley and Harris 2010) reinforces the skeletal evidence indicating that birds evolved a significant level of ecological diversity as early as the Early Cretaceous. While assigning specific trackmakers to these trace fossils is not possible to date, the existence of specialized ecological features in what were likely stem lineages points to a degree of diversification in these early birds that might have approached that seen in modern birds today.
A final line of evidence bearing on the ecology of individual specimens comes from the gut contents of particularly well-preserved individuals. O’Connor (2019) reviewed the fossil record of gut contents in Mesozoic birds, taking advantage of the exceptional preservation of numerous species with gut contents preserved in the Lower Cretaceous Jehol Lagerstätte of China. This analysis identified a variety of trophic guilds among early birds, including granivores (e.g., Jeholornis, Sapeornis), piscivores (e.g., Yanornis), and birds that fed on invertebrates (Eoalulavis) (O’Connor 2019). The elongate, thin rostrum and mandible of Longirostravis (Hou et al., 2004) and Xinghaiornis (Wang et al., 2013) has been used to infer a shore-dwelling, mud-probing lifestyle for both these birds.
While the presence of specific morphological features or preserved gut contents can make interpreting the ecology of some taxa relatively straightforward, in many cases such clear ecological indicators are not preserved in the fossil record.
Statistical Analyses of Individual Elements
In order to elucidate the complex relationships among morphology, function and ecology, a second type of paleoecological study has focused on quantitative analysis of the morphology of single elements, to evaluate them as proxies for particular aspects of avian ecology. An advantage of this approach is the ability to include fragmentary specimens, provided the focal element is preserved. The ungual phalanges or claws of birds have been used qualitatively by several studies as an indicator of ecological habit in modern birds (Richardson 1942; Yalden 1985; Peters and Görgner 1992). The first study to include a Mesozoic bird in a quantitative analysis focused on the interior curvature of the claws on the third toe and the manus of Archaeopteryx and modern birds (Feduccia 1993). The results of this study were interpreted as indicating an arboreal lifestyle for Archaeopteryx (Feduccia 1993). Other studies have expanded on Feduccia’s work by examining the scaling relationship of body mass with pedal claw shape, finding that while claw size appears to scale geometrically with body mass in most birds, the degree of overlap among most ecological groups made assigning specific ecological niches to fossil birds, such as Archaeopteryx, problematic (Pike and Maitland 2004). Glen and Bennett (2007) refined Feduccia (1993) ecological categories as segments of a continuum from completely arboreal (woodpeckers) to completely terrestrial (ostriches), and included multiple Mesozoic birds as well as non-avian theropods in the analysis. The results indicated that limited curvature of the pedal claw was the ancestral state in theropods, and most Mesozoic birds displayed pedal claw geometry consistent with a primarily terrestrial lifestyle (Glen and Bennett 2007). More recently, linear discriminant analysis of a large quantitative dataset of the curvature of the pedal ungual of modern and stem birds as well as non-avian theropods predicted an arboreal lifestyle for Archaeopteryx and Microraptor, and a predatory lifestyle for Confuciusornis (Cobb and Sellers 2020). However, a recent study has identified the remains of conifer cones among the gut contents of the confuciusornithid Eoconfuciusornis, thus casting doubts on the latter interpretation (Mayr et al., 2020).
The furcula has also been proposed as an indicator of ecology in modern birds, as the shape of the furcula varies widely across birds and is closely involved in the musculature of the flight apparatus (Hui 2002). Close and Rayfield (2012) expanded on Hui (2002) study of modern birds by performing an eigenshape analysis on the furcula of modern birds, sorted into five categories on the basis of flight style, as well as those of Mesozoic birds and non-avian theropods. This study found that Mesozoic ornithurines shared morphospace with modern flapping and flap-gliding birds, while all other Mesozoic taxa occurred at either the fringes of or outside the morphospace defined by modern birds (Close and Rayfield 2012).
Analyses of Multiple Elements
A large body of literature uses length comparisons of multiple elements in concert to correlate morphology with ecology in modern and Mesozoic birds. While individual studies usually vary in the choice of analyses and the elements included, all use similar methods. The morphospace occupied by modern birds is generally visualized using ternary diagrams or plots derived from Principal Components Analysis (PCA), which summarize the total variation described by the data. The location of the Mesozoic taxa in relation to that of modern birds in the morphospace is then described, although explicit statistical inferences are needed before drawing conclusions regarding ecological features. Ecological partitioning is often investigated by assigning modern taxa to ecological categories. Datasets can include measurements of the wing, leg, or toes, or some combination of those parts of the skeleton. Ecological bin choices vary widely across studies, ranging across general “terrestrial” and “arboreal” categories, specific descriptions of flight styles, and categories of habitat usage.
Wing Measurements
One of the primary areas of interest in early bird research is the evolution of flight and the flight capabilities of Mesozoic birds. Stemming from this is the reconstruction of flight strategies used by Mesozoic birds, and investigation of how varying flight capabilities can be tied to ecological role. While a discussion of the literature on the evolution of flight is beyond the scope of this review, a number of papers specifically address the correlation of morphometric data from the wing with flight styles associated with specific ecological niches in modern birds. Two main types of data have been utilized thus far in the literature: relative lengths of the main skeletal elements of the wing (humerus + ulna/radius + manus/carpometacarpus) and relative lengths of the skeletal elements and primary feathers (humerus + ulna/radius + manus/carpometacarpus + primary feathers).
One of the earliest studies of the correlation between form and function in the wings of ancient and modern birds was undertaken by Middleton and Gatesy (2000). In this study, ternary diagrams were utilized to visualize the measurements of modern and Mesozoic birds and non-avian theropods in morphospace (Middleton and Gatesy 2000). Mesozoic taxa fell within a small portion of the morphospace defined by modern birds, with the chosen ecological groups not overlapping substantially in the morphospace. A later study that followed these methods expanded the analysis to include a wider variety of enantiornithine birds, as well as hindlimb data (Dyke and Nudds 2009; as forelimb and hindlimb data were analyzed separately, the hindlimb data will be discussed below with other studies of the hindlimb). This study also found that most Mesozoic birds overlapped in morphospace with modern birds, but specific ecological characterizations were not used (Dyke and Nudds 2009). Furthermore, this study highlighted the importance of body mass in ecologic interpretation. For example, while the Early Cretaceous Otogornis falls near hummingbirds and swifts in the morphospace, the large size of Otogornis as compared to hummingbirds makes a similar flight strategy and ecology unlikely (Dyke and Nudds 2009).
A number of studies have focused on the use of the brachial index (BI: humerus length/ulna length) as an indicator of flight capability or style, and as a correlate of ecological role. Such research initially focused on disparity in BI across modern and fossil birds (Nudds et al., 2004), and subsequently refining correlations between BI and ecological niche partitioning (Chiappe et al., 2007; Nudds et al., 2007). Nudds et al. (2007) found that BI varied among three groups of modern birds, classified according to flight style. Chiappe et al. (2007) used BI to infer a flightless lifestyle for the Late Cretaceous bird Elsornis.
In addition to the lengths of the skeletal elements of the wing, some studies have considered the length of the primary feathers, which contribute to the overall dimensions and erodynamic properties of the wings in modern birds. Nudds et al. (2007) found a negative scaling relationship between primary feather length and total arm length in modern birds, but also noted that differences in the lengths of all elements could function to alter the relative positions of joints, thus affecting wing kinematics and flight style. Research incorporating Mesozoic birds identified relatively short primary feathers as the ancestral state in theropods, and correlated disparity in the ratio of feather length to total arm length with the ecological divergence of modern birds (Wang et al., 2011). Further research found that variation in proportional primary feather lengths was correlated with flight style and could therefore be used to predict flight style in Mesozoic birds (Wang et al., 2011). A later study contradicted these results, finding that feather length did not alter the positioning of taxa in morphospace (comparing analyses which did and did not include primary feather length) and that wing data were insufficient to distinguish flight styles among modern birds (Chan et al., 2012).
One final line of research investigating the flight modalities of stem birds is based on the combined analysis of linear measurements of the wing skeletal elements and the length of the longest primary feather (Serrano et al., 2017), structures which are often preserved among the spectacular avifauna from the Jehol Biota of northeastern China (Chiappe and Meng 2016). Serrano et al. (2017) approach used multivariate models that made possible the accurate determination of key aerodynamic parameters (wing loading and aspect ratio) in a variety of stem birds. The strength of these predictions was supported by the fact that the variables used in their study showed similar scaling patterns in both modern and stem birds, as well as the similarity of the results obtained from the multivariate models to measurements from fossils preserving wing outlines (e.g., wing surface area and wing aspect ratio). The methodology designed by Serrano et al. (2017) has been applied to a variety of stem taxa (e.g., Sapeornis, Confuciusornis, various enantiornithines), indicating that these birds collectively covered much of the spectrum of fundamental flight modes seen in modern birds (e.g., soaring, gliding, flap and glide, soaring) (Serrano and Chiappe 2017; Serrano et al., 2017; Chiappe et al., 2019). Studies by Serrano and collaborators have clearly shown that stem birds had already evolved a diversity of flight modalities, with the accompanying implied ecological diversity, by the Early Cretaceous. Additional work has focused on specific taxa; for example, authors have proposed Sapeornis was most likely a thermal soaring bird (Serrano and Chiappe 2017) and that bohaiornithids were likely continuous flapping birds (Chiappe et al., 2019). While significantly advancing our understanding of the flight properties of stem birds, these interpretations do not consider the significant musculoskeletal differences with respect to modern birds, or how such differences may have influenced flight performance. However, the stark differences in musculoskeletal arrangement of the flight apparatus between modern and stem birds (e.g., Chiappe and Meng 2016; Mayr 2017) calls into question the validity of some of these direct ecomorphological inferences and suggests we might need a deeper knowledge of the mechanics of the wingstroke in pre-modern birds.
Hindlimb Measurements
A variety of studies have focused on the hindlimb instead of the wing as a correlate of ecological role in modern and ancient birds. One early study found birds to occupy a much broader hindlimb morphospace than non-avian theropods, suggesting that reliance on the wing allowed adaptive changes in the hindlimb that might correlate with ecological diversity (Gatesy and Middleton 1997). Later work, however, found that Mesozoic enantiornithine birds only partially overlapped in morphospace with modern birds and in general displayed less variation in hindlimb measurements, suggesting less ecological diversity among the group (Dyke and Nudds 2009).
Another study successfully used the proportions of the pedal phalanges of the third toe to separate terrestrial from arboreal modern birds (Hopson 2001). Subsequent studies utilized toe proportions to identify Hongshanornis (Zhou and Zhang 2005), Jianchangornis (Zhou et al., 2009), and Archaeorhynchus (Zhou et al., 2013) as terrestrial. A later study that expanded the original database of Hopson (2001), confirmed the initial findings, and inferred Hollanda as a terrestrial bird (Bell et al., 2010).
While some of these studies covered a broad swath of the phylogeny of early birds, Bell et al. (2019) focused on a narrower clade, the hesperornithiforms. These authors utilized a database of 15 measurements from the hindlimb (exclusive of the foot) of modern foot-propelled divers as well as the extinct Hesperornithiformes to investigate morphometric variation among avian families that had convergently evolved foot-propelled diving, while focusing on the extinct hesperornithiforms. Their results revealed that loons and grebes often clustered together in morphospace, as did cormorants and diving ducks, with hesperornithiforms sometimes occupying a unique area of the morphospace and sometimes overlapping with diving ducks or cormorants, depending upon which element was considered. In contrast to the conventional analogy between hesperornithiforms and living loons and grebes, Bell et al. (2019) demonstrated that hesperornithiforms are closer in morphospace (and perhaps functionally, with regard to foot-propelled locomotion) to diving ducks and cormorants.
Wing and Hindlimb Measurements
While a large number of studies have analyzed the wing and hindlimb separately, as discussed above, relatively few studies have examined the wing and hindlimb in combination. One of the first studies to do this focused on the use of CT data to measure the cortical thickness of the humerus and femur of modern birds, finding this sort of structural data to be more useful than element length one at identifying the primary force generator (wing vs. leg) (Habib and Ruff 2008). This study had the benefit of requiring only two elements for analysis, but was limited in requiring three-dimensional preservation.
Two studies to date have combined wing and hindlimb data from both modern and stem birds. Hinic-Frlog and Motani (2009) successfully combined a wide variety of skeletal measurements to sort modern aquatic birds into several locomotor-based ecological categories and confirm the interpretation of Hesperornis as a foot-propelled diver. Bell and Chiappe (2011) demonstrated the division in morphospace of modern birds into several ecological categories based on foraging strategies and specified possible ecological niches for a number of Mesozoic birds.
Phylogenetic Signal in Morphometric Data
The degree to which evolutionary history contributes to the observed variation in morphometric datasets for modern and stem birds is often overlooked. The majority of quantitative studies described above did not control for phylogeny in their analyses of morphometric data. Some recent analyses have identified a significant phylogenetic signature in modern avian morphometric datasets, thus indicating the importance of including such methods in ecomorphological studies.
While various wing metrics have been used to infer flight styles or foraging strategies, as described above, Wang and Clarke (2015) analyzed wing shape and covert feather patterning in modern birds, finding that both wing shape variation and the extent of covert feather patterning were significantly correlated with phylogeny, but only weakly correlated with flight style. A study of airspeed in modern taxa also identified a strong phylogenetic signal (Alerstam et al., 2007).
A number of ecomorphological studies have focused on the utility of various skull and beak measurements for distinguishing foraging strategies or trophic guilds among modern birds. Benson et al. (2017) identified correlations between the size of the vestibular system and body mass as well as allometric changes in the shape of semicircular canals. The latter, however, exhibited a strong phylogenetic, and evidence for a link between flight style and labyrinth shape was ambiguous. Felice and Goswami (2018) identified a significant phylogenetic signal in three-dimensional geometric morphometric data on cranial shape in modern birds. Using this same dataset, Felice et al. (2019) found that, among modern birds, cranial morphology was poorly predicted by diet when phylogenetically transformed trait values were used. The beak is the region of the skull directly in contact with objects in the environment and might therefore be expected to be more strongly adapted to interacting with particular food items than other cranial regions. However, Navalón et al. (2019) found only a weak link between beak morphology and trophic ecology. This relationship seems to be more complex than often assumed (Gill 1995) and riddled with both trade-offs (the beak is used for many tasks beyond feeding) and many-to-one relationships between feeding autecology and morphology (a single beak shape could be associated with disparate ecologies).
A broad study that investigated a variety of morphological metrics of modern birds, including beak parameters, body size, tail remiges length, and tarsus size, found that increasing the number of morphometric dimensions in models increased their predictive abilities, but that foraging niches were less predictable than trophic levels or niches, with phylogenetic signal accounting for a significant portion of the match between phenotype models and trophic niches (Pigot et al., 2020).
Taken together, these studies highlight the importance of evaluating phylogenetic signal in morphometric data, something the majority of previous studies of Mesozoic avian ecomorphology did not do. An exception to this trend is a recent preliminary analysis by Falk et al. (2020) of approximately 60 modern and Mesozoic bird species, which found a significant phylogenetic signal in hindlimb length data, whether analyzed in terms of leg and foot modules or included in a combined analysis.
The present study therefore analyzes measurements of the forelimb and hindlimb in modern and Mesozoic birds in order to quantify the role of phylogenetic signal in these morphometric data, and examines the possibility of using this large dataset to infer the ecology of Mesozoic birds.
Materials and Methods
Because most prior work in avian paleoecomorphology has not taken phylogenetic hypotheses into account when developing ecological niche hypotheses for Mesozoic birds, this study aims to incorporate phylogenetic independent contrasts into an analysis of a large database of forelimb and hindlimb measurements from both modern and extinct birds in order to identify trends in the skeletal proportions of the limb that may have significance for inferring the ecology of extinct birds.
Morphometric Data
The data used in this analysis constitute an expansion of a previous database developed by Bell and Chiappe (2011), with 107 modern taxa added to more fully represent the phylogenetic diversity of Neornithes (Supplemental Table S1). Measurements of modern taxa were taken from the skeletal collections of the Ornithology Department at the Natural History Museum of Los Angeles County (NHMLAC). Length measurements were collected from the forelimb (humerus, ulna, radius, and carpometacarpus) and hindlimb (femur, tibiotarsus, tarsometatarsus, and phalanges I–III of pedal digit III). All measurements were taken from the same side of a single individual for each species. Measurements were taken from 583 modern bird species representing 28 orders (see Supplemental Table S1). Species were selected for inclusion based on availability in the collection of the NHMLAC, with the intent of sampling as broad a range of modern avian diversity (both phylogenetic and ecological) as possible.
Mesozoic taxa were added to the database using measurements reported in the primary literature or gathered from photographs using ImageJ (Schneider et al., 2012), with inclusion limited to taxa for which the full set of measurements listed above were available. Twenty-four taxa were added to the 16 taxa in the database of Bell and Chiappe (2011), for a total of 42 Mesozoic taxa (see Supplemental Table S1 for complete list of specimens included).
Foraging Strategies
Modern taxa were assigned to one of eight foraging strategies, as defined by Bell et al. (2010) and Bell and Chiappe (2011). Classification into foraging categories was made on the basis of the ecological descriptions provided in Hoyo (2007). When necessary, the primary literature was consulted for clarification on specific habits. It is understood that the birds used in this study are not limited to the behavior described by the bins below. Rather, the foraging bins are designed to describe the primary means by which the bird obtains food, as follows.
1. Terrestrial foragers: birds that forage on the ground, such as roadrunners or ostriches. Birds in this category gather food primarily or entirely through leg-powered foraging or stalking, thus presumably experiencing selective pressures for improved hindlimb performance. This category is not restricted to flightless birds.
2. Arboreal foragers: birds that forage in trees, such as woodpeckers or some species of parrot. Birds in this category perform activities such as fruit/seed collection or bark probing in trees. Selective pressures experienced by birds in this category might favor traits which enhance perching capabilities or short vertical flights.
3. Aerial foragers: birds that forage during flight, as well as birds that utilize flight to capture prey, such as hummingbirds or hawks. Birds in this category utilize fliight to either spot food (whether live or carrion), or engage in foraging activities such as hawking, in which prey is captured on the wing. Selective pressures experienced by birds in this category would favor enhanced forelimb development for flight capabilities such as soaring or hovering.
4. Foot-propelled divers: birds that carry out extended powered dives underwater using their legs for propulsion, such as grebes or loons. Selective pressures on these birds might favor hindlimb adaptations for swimming.
5. Wing-propelled divers: birds that carry out extended powered dives underwater using their wings for propulsion, such as penguins or auks. Selective pressures on these birds might favor forelimb adaptations for swimming.
6. Dabblers: birds that primarily swim on the surface of the water while foraging and only briefly (if at all) submerge, such as dabbling ducks. Birds in this category engage in a different style of swimming than foot or wing-propelled divers, and are therefore expected to experience different selective pressures.
7. Waders: birds that forage for food walking in shallow water, such as spoonbills or flamingoes. These birds have many foraging behaviors in common with the terrestrial foragers described above, and are therefore expected to experience very different selective pressures from aquatic birds that swim or dive.
Phylogenetic Categories
All avian taxa were clustered into one of 14 phylogenetic categories that correspond to clades supported by most current phylogenetic hypotheses (Jarvis et al., 2014; Prum et al., 2015; Wang et al., 2016): non-ornithothoracines, Enantiornithes, stem Ornithuromorpha (exclusive of Neornithes), Palaeognathae, Galloanserae, Strisores, Otidimorphae, Columbimorphae, Gruiformes, Mirandornithes, Ardeae, Charadriiformes, Telluraves (exclusive of passerines), Passeriformes.
This categorization was undertaken to subdivide the taxa in the morphometric database into a manageable number of clades that each retained a statistically meaningful sample size.
Phylogenetic Hypothesis
An informal supertree was constructed by combining two phylogenies. First, for the modern birds in the database, a maximum clade credibility (MCC) time-calibrated consensus tree was generated using TreeAnnotator (Rambaut and Drummond 2015) from a population of 10,000 Hackett’s all species trees (see www.birdtree.org and Jetz et al., 2012 for further details on these source trees) (Figure 1). Branch lengths were set equal to “Common ancestor” node heights. Second, for stem birds, the topology was constructed following recent phylogenetic hypotheses (Wang et al., 2016; Chiappe et al., 2019; Hu et al., 2020), with branch lengths calibrated using stratigraphic range data with the function bin_timePaleoPhy from the R package palaeotree (Bapst and Wagner 2019). Finally, the two phylogenies were integrated, taking the stem bird phylogeny as the receptor and rooting the modern bird phylogeny in the Neornithes terminal branch of the former using the interactive function bind. tree from the R package “ape” (Paradis and Schliep 2019). The phylogeny of modern birds was placed within the stem bird phylogeny at the neornithine node divergence time that was obtained from the original calibration (Jetz et al., 2012).
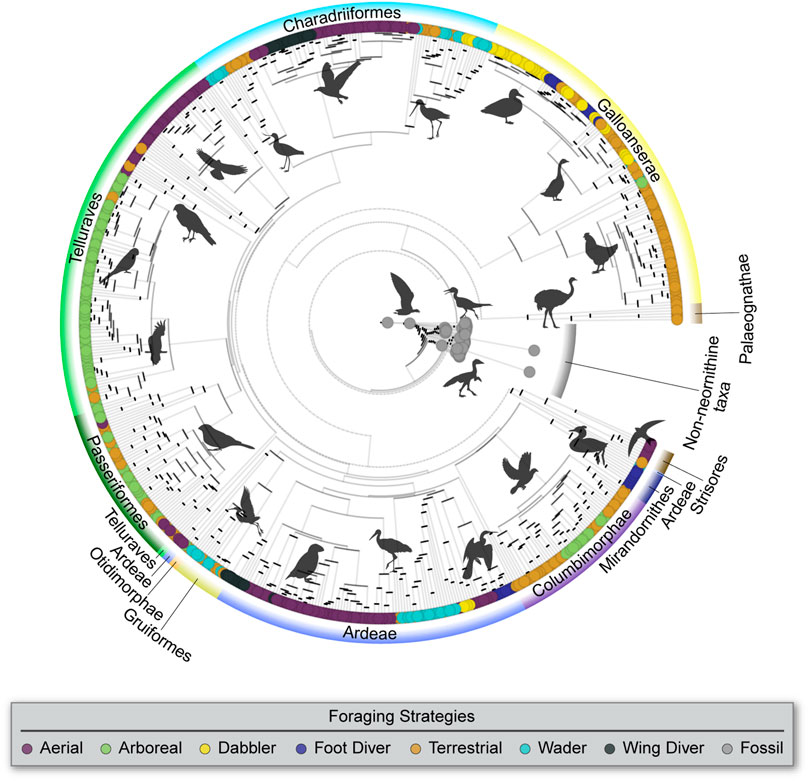
FIGURE 1. Phylogenetic tree compiled from the study of Hackett et al. (2008) for modern birds and from Wang et al. (2016), Chiappe et al. (2019), and Hu et al. (2020) for Mesozoic birds, with branches color coded by foraging strategy and phylogenetic grades used in this analysis labeled.
Statistical Analyses
All statistical analyses were conducted in R (R Core Team 2020). To account for the several orders of magnitude of size variation among lineages, all measurement length data were log10-transformed. All analyses were conducted using both raw length and log10-transformed data to gain insight about the biases introduced by each kind of data.
A Principal Components Analysis (PCA) was run to summarize the variation in skeletal element proportions among the birds in the database. Regular and phylogenetic least squares (PGLS) regressions were used to explore the allometric scaling among the main limb elements between the following pairs of bones: femur-humerus (interlimb proportions), femur-tibiotarsus (upper leg proportions), and tibiotarsus-tarsometatarsus (lower leg proportions). Allometric slopes were then compared among all possible pairs of foraging and phylogenetic groups. These analyses were done using procD.pgls and pairwise functions from the packages geomorph (Adams and Otarola-Castillo 2013) and RRPP (Collyer and Adams 2018), respectively. For the foraging groups, regression scores were plotted by taxa, such that the data were projected onto the coefficients of the regression fit, each dot representing a unique taxon colored by its foraging strategy. For the sake of clarity, only the regression lines were plotted for the 14 phylogenetic groups, such that the data were projected by their predicted values onto the regression lines for these phylogenetic groups, showing the allometric slopes for the various groups clearer.
The phylogenetic signal in the morphometric data was measured by calculating Blomberg’s K (Blomberg et al., 2003) for the entire dataset as well as for the femur/humerus, femur/tibiotarsus, and tibiotarsus/tarsometatarsus proportions, using the function physignal from the package phytools (Revell 2012). Empirical values were then compared with the predicted value of K = 1 for a constant Brownian Motion model of evolution for all the tree, which assumes diffusive evolution in which lineages exhibit no strong tendency to evolve toward certain trait values. Values of K less than one indicate weaker phylogenetic signal than expected and values close to or surpassing one indicate stronger phylogenetic signal than expected. To further visualize the phylogenetic structure of our proportional data we plotted the values of the three proportions over our phylogeny using the function contMap from the package phytools (Revell 2012).
Results
Principal Components Analysis
PCA results (Figure 2) show little separation among birds with different foraging strategies in morphospace. This is largely true for both untransformed (Figure 2) and log10-transformed (Figure 2) data. An exception is the separation of most waders and terrestrial birds from most aerial and dabbling birds along PC2. This separation is seen more clearly in the untransformed data than in the log10-transformed data. Mesozoic taxa largely fall within the region of morphospace where ecological groups overlap; however, Patagopteryx does plot outside that region, in the area occupied by modern waders and terrestrial birds. Phylogenetic groups exhibit a similar pattern but with even greater overlap in morphospace. Paleognaths were mostly separated from the other taxa and some members of Ardeae fall outside of the main cluster of birds into a unique area of morphospace for both the untransformed (Figure 2) and log10-transformed (Figure 2) data. Some members of Strisores and Passeriformes also fall into unique regions of the morphospace when considering log10-transformed data, but they fall closer to the main cluster of birds when using untransformed data.
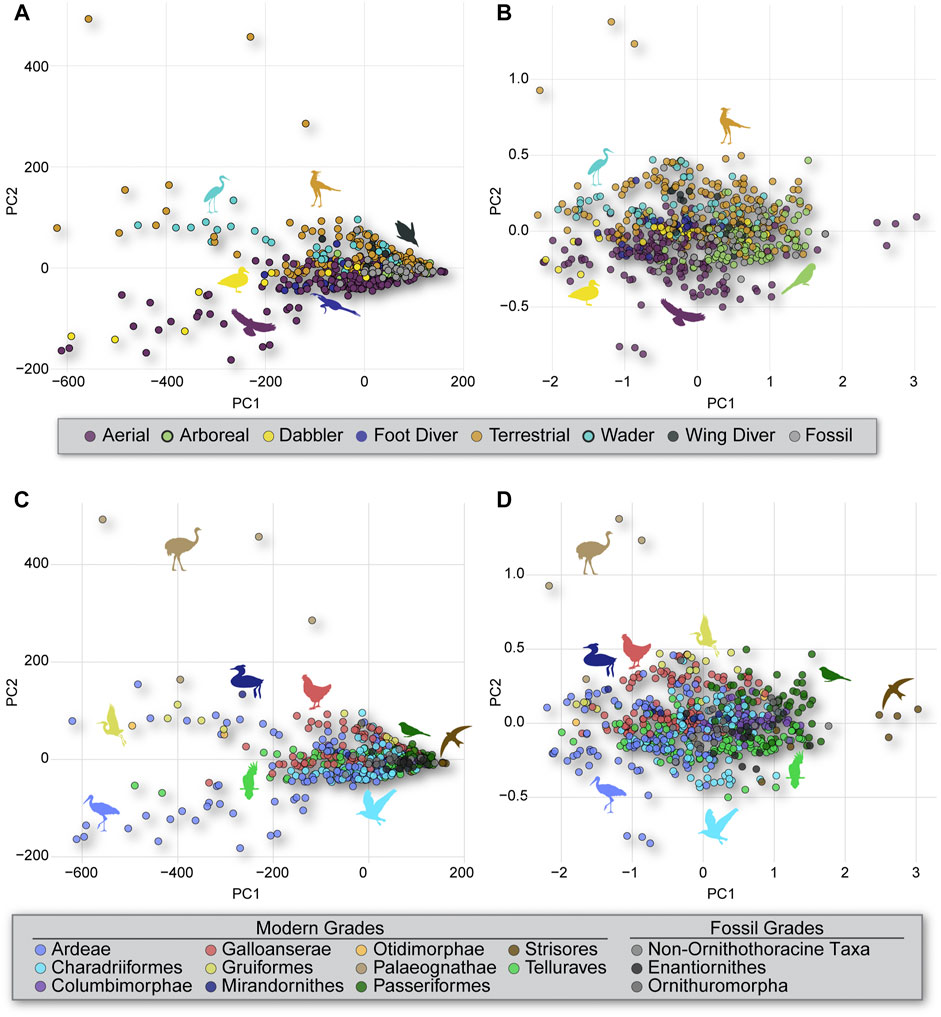
FIGURE 2. PCA results of untransformed (A) and log10-transformed (B) morphometric data color-coded by foraging strategy. The same data color coded by clade, with untransformed (C) and log10-transformed (D) morphometric data. Notice how the raw data shown in (A,C) clearly display slope differences reflecting the scaling patterns between dimensions (PCs), whereas the log10-transformed data in (B,D) obscure those relationships.
Phylogenetic Signal
Blomberg’s K (Blomberg et al., 2003) was calculated for all morphometric data in order to assess the overall phylogenetic signal in these data. This analysis returned a K-value of 0.4753 for the untransformed data and a K-value of 0.5175 for the log10-transformed data. This indicates a weak phylogenetic signal overall, but the K-values for individual elements varied, as described below. This highlights the importance of considering skeletal elements separately when assessing the covariation of phylogeny and morphometric patterns, rather than simply treating the data as a whole.
Interlimb Proportions and Scaling
The influence of phylogeny on the observed morphometric patterns is indicated by the K-values determined in this study and can be readily visualized using heatmaps of interlimb proportions mapped onto the consensus phylogeny used in this study (Figures 3, 4) or by mapping foraging strategy onto phylogeny (Figure 1). The proportion of the femur to the humerus exhibits a very high degree of phylogenetic signal [K = 1.669 and 1.456, for untransformed (Figure 3) and log10-transformed (Figure 4) data, respectively]. This indicates that most variation in the proportion between these two bones can be attributed to lineage-specific factors or allometry, and should not be straightforwardly interpreted as representing an ecomorphological signal. This notwithstanding, further scrutiny of this pattern reveals that certain aerial birds like frigatebirds and tubenoses (albatrosses, shearwaters, fulmars), as well as foot propelled-divers like loons and grebes exhibit the most extreme values, having very large humeri but comparatively very short femora (Figure 5). Some phylogenetic analyses place these birds in a clade with Charadriiformes called Aequorlitornithes (e.g., Prum et al., 2015), and so it is unsurprizing that are the most allometrically distinct with regard to interlimb scaling proportions (Figure 5; Supplemental Tables S2 and S3). Further confirming this pattern, dabblers, aerial foragers, and foot-propelled divers are the most statistically distinctive foraging groups, although this varies depending on whether untransformed or log10-transformed data are used, indicating differences in overall size are an important factor affecting this allometric pattern (Figure 5; Supplemental Tables S2 and S4).
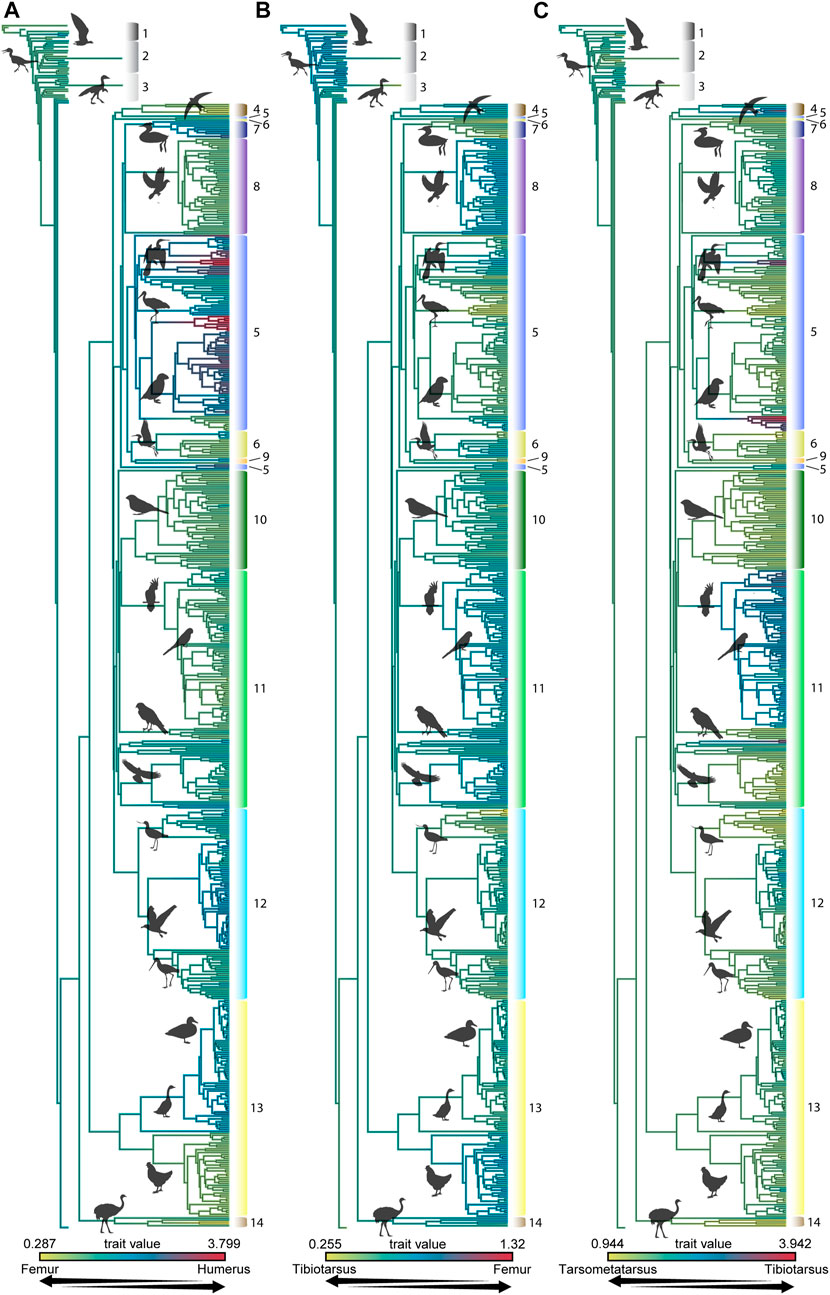
FIGURE 3. Proportions of the untransformed lengths of the humerus to femur (A), femur to tibiotarsus (B), and tibiotarsus to tarsometatarsus (C) mapped onto the phylogeny created for this analysis. Phylogenetic grade numbers are as follows: 1 Non-ornithothoracine taxa, 2 Enantiornithes, 3 Ornithuromorpha, 4 Strisores, 5 Ardeae, 6 Gruiformes, 7 Mirandornithes, 8 Columbimorphae, 9 Otidimorphae, 10 Passeriformes, 11 Telluraves, 12 Charadriiformes, 13 Galloanserae, 14 Palaeognathae.
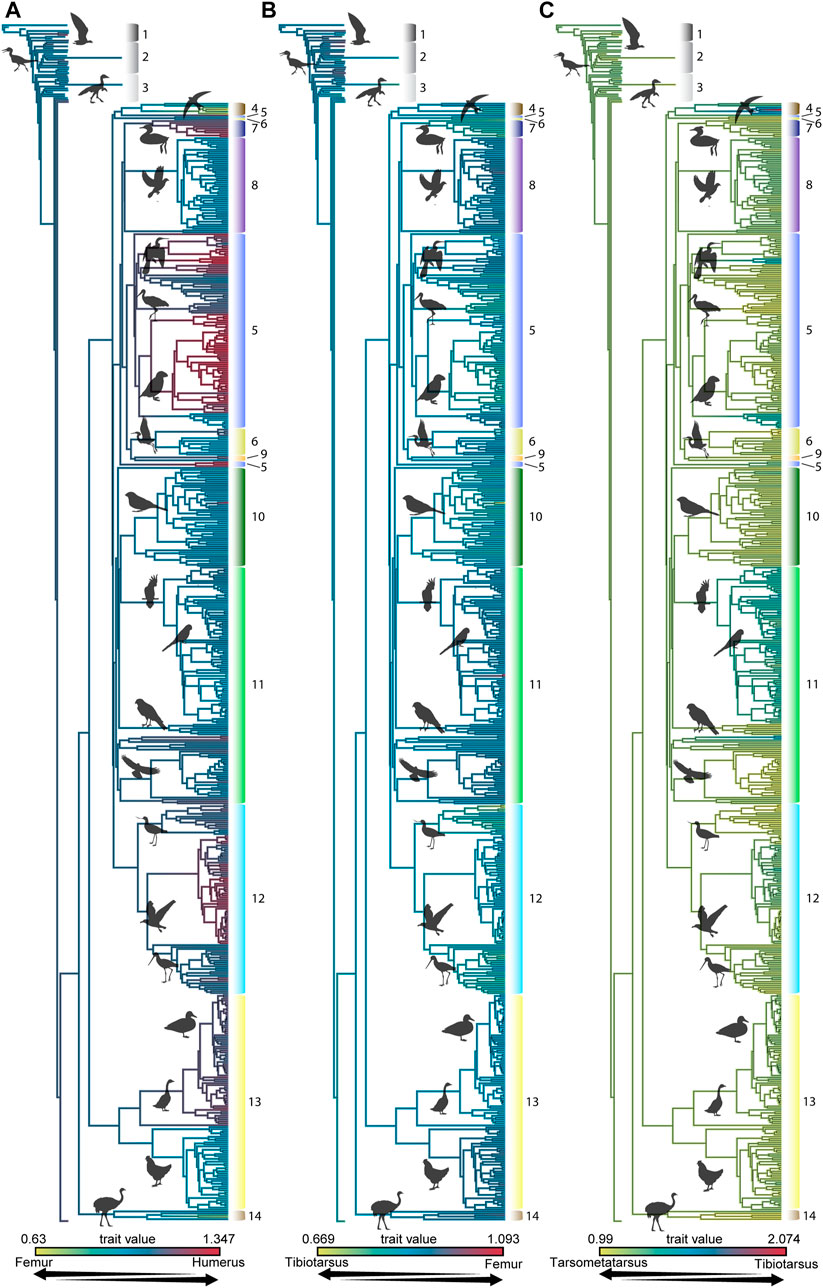
FIGURE 4. Proportions of the log10-transformed lengths of the humerus to femur (A), femur to tibiotarsus (B), and tibiotarsus to tarsometatarsus (C) mapped onto the phylogeny created for this analysis. Phylogenetic grade numbers are as follows: 1 Non-ornithothoracine taxa, 2 Enantiornithes, 3 Ornithuromorpha, 4 Strisores, 5 Ardeae, 6 Gruiformes, 7 Mirandornithes, 8 Columbimorphae, 9 Otidimorphae, 10 Passeriformes, 11 Telluraves, 12 Charadriiformes, 13 Galloanserae, 14 Palaeognathae.
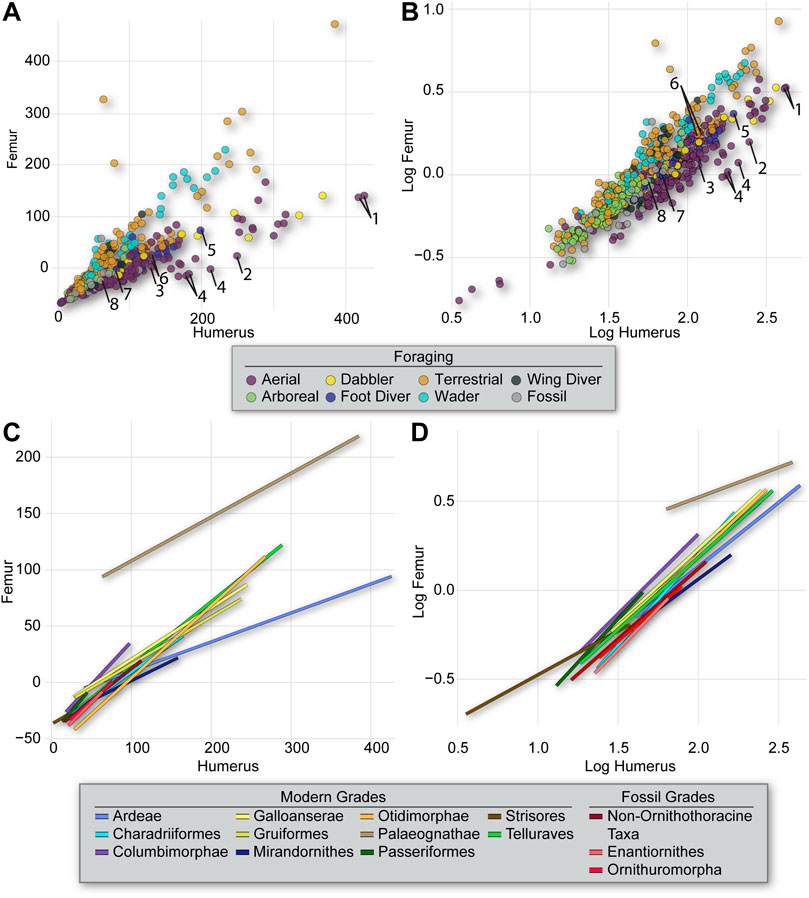
FIGURE 5. Interlimb proportions (humerus vs. femur) from all specimens coded by foraging strategy (A,B) and slopes for phylogenetic groupings (C,D) resulting from the PGLS regression of the untransformed (A,C) and log10-transformed (B,D) data. Notice clear differences among the regression slopes for the foraging groups and clades when using untransformed data (A,C) and their collapse when using log10-transformed data (B,D). Taxa are numbered as follows in (A,B): Tubenoses–1 Diomedea, 2 Phoebetria, 3 Calonectris; Frigatebird–4 Fregata; Loon–5 Gavia; Grebe–6 Aechmophorous, 7 Podiceps, 8 Rollandia.
Upper Leg Proportions and Scaling
The proportion of the femur to the tibiotarsus does not exhibit significant phylogenetic signal [K = 0.274 and 0.293, for untransformed (Figure 3) and log10-transformed (Figure 4) data, respectively], unlike the proportion of the femur to the humerus g described above. Ecomorphologically, the most extreme values of this proportional index are among very long-legged taxa with a wading foraging strategy, such as storks and herons (Ardeae), wading birds (Charadriiformes), and flamingoes (Mirandornithes) (Figure 6). The slopes for all these clades roughly align, and deviate from those for clades that forage primarily arboreally or terrestrially (Telluraves, Columbimorphae, Passeriformes) (Figure 6; Supplemental Tables S2 and S3). However, many of these differences collapse when using the log10-transformed data, indicating differences in total size are an important factor defining this allometric scaling (Figure 6). The general pattern is confirmed in three of seven of the pairwise comparisons, with aerial foragers being statistically distinct from the other foraging groups, as these taxa are more short-legged in general (Supplemental Tables S2 and S4). Arboreal taxa could be distinguished from many of the other groups (such as aerial, terrestrial, and wading groups) using raw data but not with log10-transformed data, revealing that these taxa tend to be very small in overall size (Supplemental Tables S2 and S4).
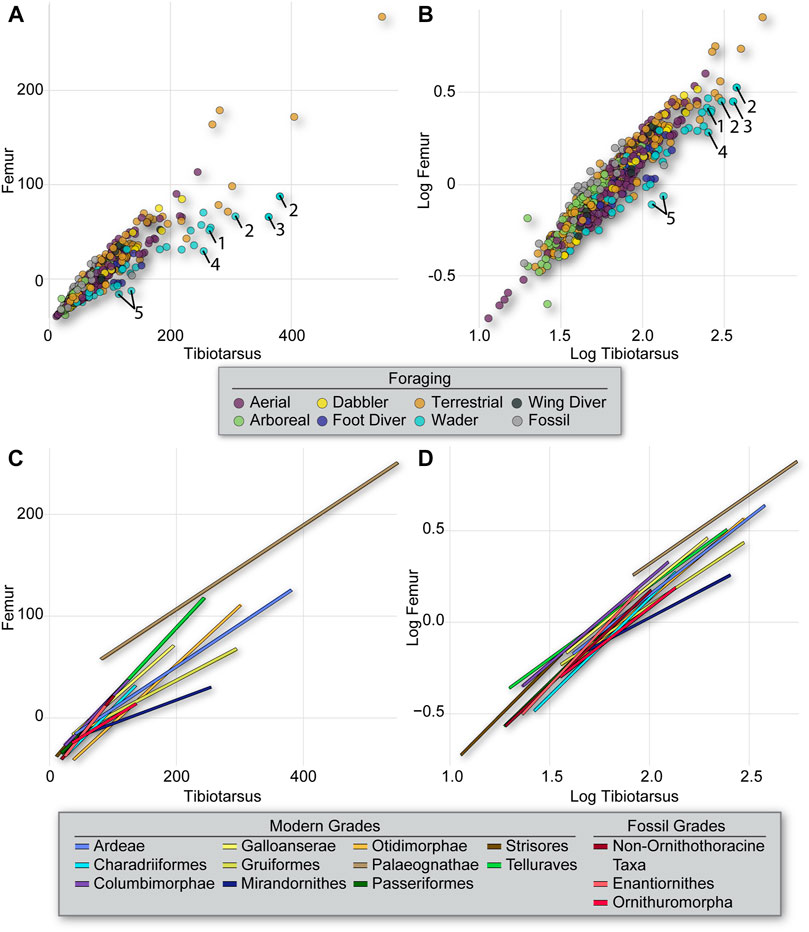
FIGURE 6. Intralimb upper-leg proportions (femur vs. tibiotarsus) from all specimens coded by foraging strategy (A,B) and slopes for phylogenetic groupings (C,D) resulting from the PGLS regression of the untransformed (A,C) and log10-transformed (B,D) data. As in Figure 5, slope differences collapse when using log10-transformed data, confounding results. Taxa are numbered as follows in (A,B): Storks–1 Ciconia, 2 Leptoptilos, 3 Epihippiorhynchus; Flamingo–4 Phoeniconaias; Stilt–5 Himantopus.
Enantiornithines exhibit significant differences in slope from many clades of modern birds and ornithuromorphs, and near significant differences from non-ornithothoracines. However, their regression linenearly overlaps that for modern passerines. This suggests stark differences in ecology among enantiornithines and ornithuromorphs in Mesozoic ecosystems, particularly during the Early Cretaceous.
Lower Leg Proportions and Scaling
The proportion of the tibiotarsus to the tarsometatarsus also exhibits a weak phylogenetic signal [K = 0.660 and 0.616 for untransformed (Figure 3) and log10-transformed (Figure 4) data, respectively]. Penguins and frigatebirds (Ardeae), parrots (Telluraves), and hummingbirds (Strisores) exhibit the most extreme values, and some long-legged taxa like ratites (Palaeognathae) or storks (Charadriiformes) exhibit extreme values in the other direction (Figure 7). As expected from the diverse phylogenetic positions of these taxa, there are practically no significant differences in scaling among the phylogenetic groups when considering the log10-transformed data, with only Strisores differing from other clades (Supplemental Tables S2 and S3). Similarly, the only statistically significant differences in slope evident from the log10-transformed data separate terrestrial and wading birds from all other foraging ecologies (i.e., dabbler, arboreal, aerial, plunge divers, and foot- and wing-propelled divers). When considering raw data, many more significant differences between clades and foraging groups emerge, as most groups exhibit idiosyncratic scaling relationships in which total size is a defining factor.
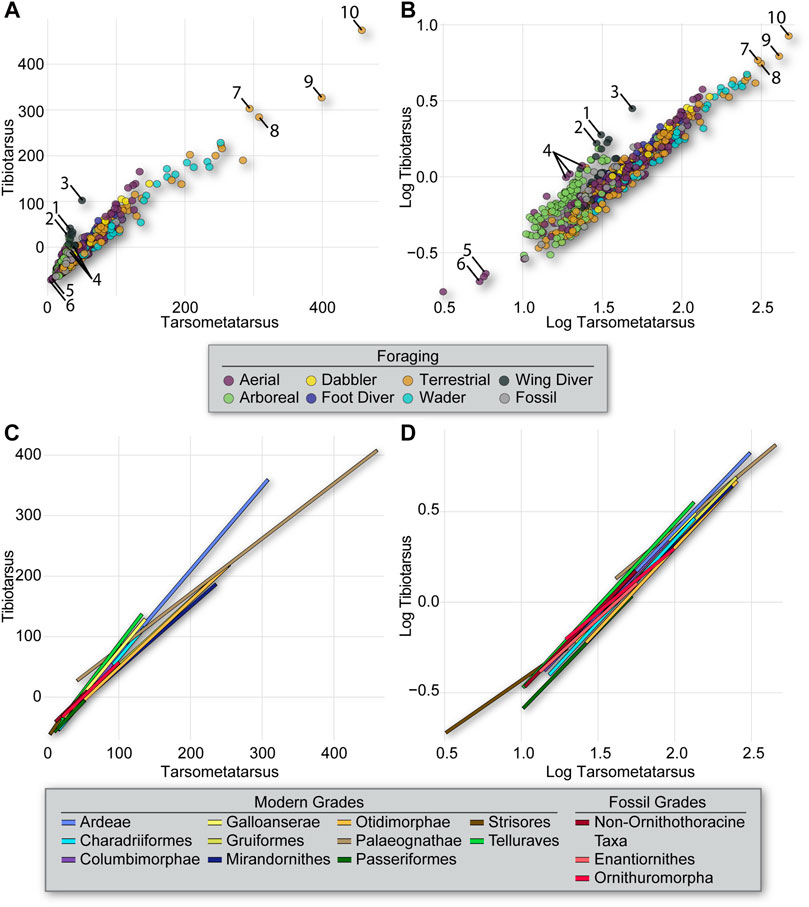
FIGURE 7. Intralimb lower-leg proportions (tibiotarsus vs tarsometatarsus) from all specimens coded by foraging strategy (A,B) and slopes for phylogenetic groupings (C,D) resulting from the PGLS regression of the untransformed (A,C) and log10-transformed (B,D) data. As in Figure 5, slope differences collapse when using log10-transformed data, confounding results. Taxa are numbered as follows in (A,B): Penguins–1 Pygoscelis, 2 Eudyptes, 3 Aptenodytes; Frigatebird–4 Fregata; Hummingbirds–5 Aphantochroa, 6 Archilochus; Storks–7 Leptoptilos, 8 Epihippiorhynchus; Ratites–9 Dromaius, 10 Struthio.
When considering the proportions of the lower limb, none of the groups of Mesozoic birds (non-ornithothoracine taxa, enantiornithines, ornithuromorphs) exhibit slopes that differ significantly from those for other groups of birds included in this analysis.
Discussion
Our results are largely consistent in general patterns of morphospace occupation among lineages and foraging groups with previous studies that included a similar range of taxa (for example, Dyke and Nudds 2009; Bell et al., 2010; Bell and Chiappe 2011; Falk et al., 2020). All these studies found most avian taxa clustered together in morphospace, regardless of ecology, with only small regions of morphospace in which specific ecological niches were isolated. The only taxa whose positions in morphospace may actually result from shared ecology are the terrestrial and wading birds, as these taxa belong to a variety of clades. Their shared morphospace may thus reflect similar selective pressures that drive elongation of the hindlimb for walking in grass or shallow water, foraging strategies that might be functionally similar. Other similarities in morphospace occupation, such as the overlap of dabbling and aerial birds, likely reflect the close relationship of the taxa in question. For example, all of the dabblers and aerial foragers that plot together outside of the common central morphospace are members of the same clade (Ardeae) (Figure 2).
As described above, our results indicate varying degrees of phylogenetic signal in inter-and intra-limb proportions (Figure 3). Proportions among pairs of elements are generally consistent within clades, and only rarely is intra-clade variation high. Several clades included in our analyses exhibit a variety of foraging strategies among their constituent taxa but little variation in element ratios. One example of this is the Charadriiformes, which are generally thought of as wading or shore birds, but actually encompass taxa with diverse foraging strategies, including wing propelled divers, aerial hunters, terrestrial birds, waders, and dabblers. Despite these differences in foraging style, the phylogenetic map of the humerus:femur ratio shows less variation than is present among the different clades considered in this study (Figure 3). An example of this is the similar (and extreme) humerus:femur proportion values seen in both the aerial foraging frigatebirds and tubenoses and the foot-propelled diving loons. These birds all belong to the Ardeae, and thus may share an elongate humerus and proportionally reduced femur as a result of their close evolutionary relationship, despite having very different ecological habits.
However, we found some scaling differences among foraging groups regardless of phylogeny. Proportional relationships between the long bones of the hindlimb (femur:tibiotarsus and tibiotarsus:tarsometatarsus) were associated with low K-values, indicating weak phylogenetic signal. For example, the upper leg proportion reveals differences among several foraging strategies when pairwise comparisons are made between foraging groups (Supplemental Table S4). Foot-propelled divers, waders, and terrestrial birds show significant differences from all other foraging strategies, but not from each other, in this proportion. When considered together, these three foraging strategies encompass a phylogenetically diverse sample of the birds included in this dataset, including members of each of the 13 clades used in this study except Strisores. This well-supported difference in scaling between birds that use their hindlimbs for foraging in some way and all other birds is intriguing. When considering the lower leg proportions, wing-propelled divers differ significantly from all other foraging groups. Wing-propelled diving birds are represented by penguins (Ardeae) and alcids (Charadriiformes) in this dataset, clades that are not particularly closely related (Figure 1).
Previous studies concur in detecting generalities about the contribution of phylogeny and ecology to variation in skeletal morphometrics in birds. However, most such investigations have been relatively inconclusive regarding the biological contributions of the variables to such patterns, or in other words, regarding the real relationships among the original variables underlying the findings. This may occur because the original variables have been transformed into logarithms in an effort to constrain large size variation (i.e., dimensionality; Reyment et al., 1984; Sokal and Rohlf 2012), and hence accommodate scale by equalizing variances (Bryant 1989). What most authors fail to recognize, however, is that such transformation introduces a systematic bias into estimates of scaling coefficients, which is very rarely acknowledged, corrected, or reported (LaBarbera 1989). This situation is especially worrying when multivariate approaches are utilized, particularly PCA, because ecological processes may lead to scaling effects that render log-transformation inappropriate, as such methods are affected by any scale-dependent variation (Bryant 1989). By analyzing (and illustrating) the real scaling patterns among the original variables, our results have demonstrated that logarithmically transformed traits hide or at least obfuscate the ecological and evolutionary circumstances that lead to the observed scaling patterns, inevitably affecting the biological and ecological information that can be gained for paleobiological inference.
Our results support the interpretation that phylogenetic history strongly covaries with ecology in Neornithes. Therefore, although limb skeletal scaling patterns vary in several respects among lineages and/or foraging groups, the strong covariation implies that morphometric data on long bones have limited utility for identification of ecological trends at macroevolutionary scales in birds. Despite this, one interesting trend that is apparent in these analyses is the divergent scaling of the femur and tibiotarsus between enantiornithines and ornithuromorphs (Figure 6 and Supplemental Table S3). Furthermore, enantiornithines do not differ significantly from non-ornithothoracine taxa with regard to this ratio, but ornithuromorphs do. This implies a deep divergence between ornithuromorph and non-ornithuromorph taxa in upper hindlimb scaling patterns. To our knowledge, this is the first time quantitative evidence for such a distinction between ornithuromorphs and other stem birds, some of which may have been sympatric, has been adduced. The vast majority of stem birds in the present dataset come from the Jehol Biota. For example, 11 enantiornithines, six ornithuromorphs, and five non-ornithothoracine taxa in the current dataset have all been reported from the Jiufotang Formation, one of the geologic units containing the Jehol Biota. Previously, qualitative studies of the Jehol birds have proposed a stark difference in ecology between enantiornithines and ornithuromorphs based primarily on inferred trophic niches, with enantiornithines purportedly specialized for arboreal niches and ornithuromorphs specialized for land-based or amphibious niches (primarily terrestrial or wading niches) (Hopson 2001; Zhou 2006; O’Connor 2012; O’Connor 2019; Field et al., 2018). The quantitative differences in the proportions of the upper hindlimb bones identified here may relate to this previously proposed niche partitioning.
Furthermore, comparison of the results obtained from untransformed and log-transformed data highlights how data transformations can obscure real scaling relationships among the original variables in an analysis; for instance, using such treated data, past studies (Gatesy and Middleton 1997; Middleton and Gatesy 2000; Hopson 2001; Benson et al., 2017; Falk et al., 2020) detected that morphometric differences adhere to phylogeny, but the use of log-transformed data obscured that proportional relationships in skeletal elements are largely phylogenetic. This, in turn, implies that 1) ecology only latently underlies morphological differences at macroevolutionary scales such as those presented in this paper and thus, 2) any ecomorphological approximation based on function needs to be performed with careful consideration of phylogenetic context. This indicates that the numerous previous studies that have relied upon multivariate methods to draw inferences regarding the foraging strategies of stem birds without the proper data and without duly considering phylogenetic context should be viewed cautiously. Such caution does not imply that ecomorphological studies have no utility in the study of Mesozoic birds; rather, it highlights the importance of using appropriate methods that take into account the phylogenetic structure of the data (i.e., Wang and Clarke 2015; Falk et al., 2020; Pigot et al., 2020). Additionally, these results highlight the variability in phylogenetic signal obtained from different combinations of variables, with the humerus:femur proportion showing a much higher phylogenetic signal than the tibiotarsus:tarsometatarsus proportion. The variation in phylogenetic signal among different limb element ratios has not been previously demonstrated. Future work utilizing different types of morphometric data may identify morphometric proxies that are less tied to phylogenetic history.
Data Availability Statement
The original contribution presented in the study are included in the article/Supplementary Material, further inquiries can be directed to the corresponding author.
Author Contributions
The division of labor for this study was as follows: AB developed the database and supervised the collection of modern bird measurements, conducted the literature review, authored the introduction and database methods section of this paper, finalized the statistical methods, results, and discussion sections of this paper, developed initial drafts of figures, and coordinated all work among co-authors; JM, GN, and SN carried out the statistical analyses; JD collected the Mesozoic birds data and conducted the preliminary statistical assessments; and LC acquired funding, assisted with overall project design, and provided specimen photographs for inclusion in the database. All authors contributed to writing and reviewing the manuscript.
Funding
This research was funded by generous donations of Gretchen Augustyn to the Dinosaur Institute of the NHMLA. SMN is funded by a FPI-UAM 2019 predoctoral grant from the Universidad Autónoma de Madrid. GN is funded by the European Union’s Horizon 2020 research and innovation program 2014–2018 under grant agreement 677774 (European Research Council (ERC) Starting Grant: TEMPO).
Conflict of Interest
The authors declare that the research was conducted in the absence of any commercial or financial relationships that could be construed as a potential conflict of interest.
Acknowledgments
The authors would like to thank the interns of Proyecto Dinosaurios, who assisted in the collection of portions of the modern bird database, and Stephanie Abramowicz, who photographed fossil specimens and contributed to the figures for this paper.
Supplementary Material
The Supplementary Material for this article can be found online at: https://www.frontiersin.org/articles/10.3389/feart.2021.663342/full#supplementary-material
References
Adams, D. C., and Otárola-Castillo, E. (2013). Geomorph: Anrpackage for the Collection and Analysis of Geometric Morphometric Shape Data. Methods Ecol. Evol. 4 (April), 393–399. doi:10.1111/2041-210X.12035
Alerstam, T., Rosén, M., Bäckman, J., Ericson, P. G. P., Hellgren, O., and Hellgren, O. (2007). Flight Speeds Among Bird Species: Allometric and Phylogenetic Effects. Plos Biol. 5 (8), e197. doi:10.1371/journal.pbio.0050197
Alvarenga, H. M. F., and Bonaparte, J. F. (1992). “A New Flightless Land Bird from the Cretaceous of Patagonia.” Natural History Museum Of Los Angeles County. Sci. Ser. 36, 51–64.
Bapst, D. W., and Wagner, P. (2019). Paleotree: An R Package for Paleontological and Phylogenetic Analyses of Evolution. Available at: https://CRAN.Rproject.org/package=paleotree.
Barbosa, A. (1993). Morphometric Variation of the Hindlimb of Waders and its Evolutionary Implications. Ardeola 40 (1), 65–75.
Bell, A., and Chiappe, L. M. (2016). A Species-Level Phylogeny of the Cretaceous Hesperornithiformes (Aves: Ornithuromorpha): Implications for Body Size Evolution Amongst the Earliest Diving Birds. J. Syst. Palaeontology 14 (3), 239–251. doi:10.1080/14772019.2015.1036141
Bell, A., and Chiappe, L. M. (2011). Statistical Approach for Inferring Ecology of Mesozoic Birds. J. Syst. Palaeontology 9 (1), 119–133. doi:10.1080/14772019.2010.525536
Bell, A. K., Chiappe, L. M., Erickson, G. M., Suzuki, S., Erickson, G. M., Suzuki, S., et al. (2010). Description and Ecologic Analysis of Hollanda Luceria, a Late Cretaceous Bird from the Gobi Desert (Mongolia). Cretaceous Res. 31 (1), 16–26. doi:10.1016/j.cretres.2009.09.001
Bell, A., Wu, Y.-H., and Chiappe, L. M. (2019). Morphometric Comparison of the Hesperornithiformes and Modern Diving Birds. Palaeogeogr. Palaeoclimatol. Palaeoecol. 513, 196–207. doi:10.1016/j.palaeo.2017.12.010
Benson, R. B. J., Starmer-Jones, E., Close, R. A., and Walsh, S. A. (2017). Comparative Analysis of Vestibular Ecomorphology in Birds. J. Anat. 231 (6), 990–1018. doi:10.1111/joa.12726
Blomberg, S. P., Garland, T., and Ives, A. R. (2003). Testing for Phylogenetic Signal in Comparative Data: Behavioral Traits Are More Labile. Evolution 57 (4), 717–745. doi:10.1111/j.0014-3820.2003.tb00285.x
Bock, W. J. (1994). Concepts and Methods in Ecomorphology. J. Biosci. 19 (4), 403–413. doi:10.1007/bf02703177
Brocklehurst, N., Paul, U., Mannion, P. D., and O’Connor, J. (2012). The Completeness of the Fossil Record of Mesozoic Birds: Implications for Early Avian Evolution. PLoS One 7 (6), e39056. doi:10.1371/journal.pone.0039056
Brusatte, S. L., O’Connor, J. K., and Jarvis, E. D. (2015). The Origin and Diversification of Birds. Curr. Biol. 25 (19), R888–R898. doi:10.1016/j.cub.2015.08.003
Bryant, E. H. (1989). Multivariate Morphometrics of Bottlenecked Populations. Evolutionary Biology of Transient Unstable Populations. NY: New York, Springer, 19–31.
Chan, N. R., Dyke, G. J., and Benton, M. J. (2012). Primary Feather Lengths May Not Be Important for Inferring the Flight Styles of Mesozoic Birds. Lethaia 46 (September), 146–153. doi:10.1111/j.1502-3931.2012.00325.x
Chiappe, L. M., and Bell., A. (2020). Mesozoic Birds. Encyclopedia of Life Sciences American Cancer Society, Wiley-Blackwell, NJ: Hoboken, American Cancer Society, 549–557. doi:10.1002/9780470015902.a0029218
Chiappe, L. M., Suzuki, S., Dyke, G. J., Watabe, M., Tsogtbaatar, K., and Barsbold, R. (2007). A New Enantiornithine Bird from the Late Cretaceous of the Gobi Desert. J. Syst. Palaeontology 5 (2), 193–208. doi:10.1017/S1477201906001969
Chiappe, L. M., and Witmer, L. M. (2002). “Osteology of the Flightless Patagopteryx Deferrariisi from the Late Cretaceous of Patagonia (Argentina),” in Mesozoic Birds: Above the Heads of Dinosaurs. Editor L. M. Chiappe (Berkeley, Califonia: University of California Press), 281À316.
Chiappe, L. M. (2018). Origin and Early Evolution. in Ornithology: Foundation, Analysis, and Application, 9.
Chiappe, L. M., Meng, Q., Serrano, F., Sigurdsen, T., Wang, M., Bell, A., et al. (2019). New Bohaiornis-like Bird from the Early Cretaceous of China: Enantiornithine Interrelationships and Flight Performance. PeerJ 7, e7846. doi:10.7717/peerj.7846
Chiappe, L. M., and Meng, Q. (2016). Birds of Stone: Chinese Avian Fossils from the Age of Dinosaurs. Baltimore, MD: JHU Press.
Chiappe, L. M., and Walker, C. A. (2002). Skeletal Morphology and Systematics of the Cretaceous Euenantiornithes (Ornithothoraces: Enantiornithes). Mesozoic Birds: Above the Heads of Dinosaurs, CA: Berkeley, University of California Press, 240–267.
Close, R. A., and Rayfield., E. J. (2012). Functional Morphometric Analysis of the Furcula in Mesozoic Birds. PLoS One 7 (5), e36664. doi:10.1371/journal.pone.0036664
Cobb, S. E., and Sellers, W. I. (2020). Inferring Lifestyle for Aves and Theropoda: A Model Based on Curvatures of Extant Avian Ungual Bones. Plos One 15 (2), e0211173. doi:10.1371/journal.pone.0211173
Collyer, M. L., and Adams., D. C. (2018). RRPP: An R Package for Fitting Linear Models to High‐dimensional Data Using Residual Randomization. Methods Ecol. Evol. 9 (7), 1772–1779. doi:10.1111/2041-210x.13029
Dyke, G. J., and Nudds, R. L. (2009). The Fossil Record and Limb Disparity of Enantiornithines, the Dominant Flying Birds of the Cretaceous. Lethaia 42 (June), 248–254. doi:10.1111/j.1502-3931.2008.00135.x
Falk, A. R., Lamsdell, J. C., and Gong, E. (2020). Principal Component Analysis of Avian Hind Limb and Foot Morphometrics and the Relationship between Ecology and Phylogeny. Paleobiology, 47, 1–23. doi:10.1017/pab.2020.39
Feduccia, A. (1993). Evidence from Claw Geometry Indicating Arboreal Habits of Archaeopteryx. Science 259 (5096), 790–793. doi:10.1126/science.259.5096.790
Felice, R. N., and Goswami., A. (2018). Developmental Origins of Mosaic Evolution in the Avian Cranium. Proc. Natl. Acad. Sci. USA. 115 (3), 555–560. doi:10.1073/pnas.1716437115
Felice, R. N., Tobias, J. A., Pigot, A. L., and Goswami, A. (2019). Dietary Niche and the Evolution of Cranial Morphology in Birds. Proc. R. Soc. B. 286, 20182677. doi:10.1098/rspb.2018.2677
Field, D. J., Benito, J., Chen, A., Jagt, J. W. M., and Ksepka, D. T. (2020). Late Cretaceous Neornithine from Europe Illuminates the Origins of Crown Birds. Nature 579 (7799), 397–401. doi:10.1038/s41586-020-2096-0
Field, D. J., Bercovici, A., Berv, J. S., Dunn, R., Fastovsky, D. E., Lyson, T. R., et al. (2018). Early Evolution of Modern Birds Structured by Global Forest Collapse at the End-Cretaceous Mass Extinction. Curr. Biol. 28 (11), 1825–1831. doi:10.1016/j.cub.2018.04.062
Fountaine, T. M. R., Benton, M. J., Dyke, G. J., and Nudds, R. L. (2005). The Quality of the Fossil Record of Mesozoic Birds. Proc. R. Soc. B. 272 (1560), 289–294. doi:10.1098/rspb.2004.2923
Gatesy, S. M., and Middleton, K. M. (1997). Bipedalism, Flight, and the Evolution of Theropod Locomotor Diversity. J. Vertebr. Paleontol. 17 (2), 308–329. doi:10.1080/02724634.1997.10010977
Glen, C. L., and Bennett, M. B. (2007). Foraging Modes of Mesozoic Birds and Non-avian Theropods. Curr. Biol. 17 (21), R911–R912. doi:10.1016/j.cub.2007.09.026
Habib, M. B., and Ruff., C. B. (2008). The Effects of Locomotion on the Structural Characteristics of Avian Limb Bones. Zoolog. J. Linn. Soc. 153 (3), 601–624. doi:10.1111/j.1096-3642.2008.00402.x
Hertel, F. (1995). Ecomorphological Indicators of Feeding Behavior in Recent and Fossil Raptors. The Auk 112 (4), 890–903. doi:10.2307/4089021
Hinic-Frlog, Sanja., and Motani, R. (2009). Relationship between Osteology and Aquatic Locomotion in Birds: Determining Modes of Locomotion in Extinct Ornithurae. J. Evol. Biol. 23 (December), 372–385. doi:10.1111/j.1420-9101.2009.01909.x
Hopson, J. A. (2001). Ecomorphology of Avian and Nonavian Theropod Phalangeal Proportions: Implications for the Arboreal versus Terrestrial Origin of Bird Flight Editors Gauthier, J., and Gall, L., in New Perspectives on the Origin and Early Evolution of Birds: Proceedings of the International Symposium in Honor of John H. Ostrom. Peabody Museum of Natural History, CT: New Haven, Yale University, 211–235.
Hou, L., Chiappe, L. M., Zhang, F., and Chuong, C.-M. (2004). New Early Cretaceous Fossil from China Documents a Novel Trophic Specialization for Mesozoic Birds. Naturwissenschaften 91 (1), 22–25. doi:10.1007/s00114-003-0489-1
Hoyo, J. d. (2007). Buteo Books: Handbook of Birds of the World. Available at: https://www.buteobooks.com/category/HBW.html.
Hu, H., O’Connor, J. K., and Zhou, Z. (2015). A New Species of Pengornithidae (Aves: Enantiornithes) from the Lower Cretaceous of China Suggests a Specialized Scansorial Habitat Previously Unknown in Early Birds. PLoS One 10 (6), e0126791. doi:10.1371/journal.pone.0126791
Hu, H., O’Connor, J. K., Wang, M., Wroe, S., and McDonald, P. G. (2020). New Anatomical Information on the Bohaiornithid Longusunguis and the Presence of a Plesiomorphic Diapsid Skull in Enantiornithes. J. Syst. Palaeontology 18 (18), 1481–1495. doi:10.1080/14772019.2020.1748133
Hui, C. A. (2002). Avian Furcula Morphology May Indicate Relationships of Flight Requirements Among Birds. J. Morphol. 251 (3), 284–293. doi:10.1002/jmor.1089
Jarvis, E. D., Mirarab, S., Aberer, A. J., Li, B., Houde, P., Li, C., et al. (2014). Whole-Genome Analyses Resolve Early Branches in the Tree of Life of Modern Birds. Science 346 (6215), 1320–1331. doi:10.1126/science.1253451
Jetz, W., Thomas, G. H., Joy, J. B., Hartmann, K., and Mooers, A. O. (2012). The Global Diversity of Birds in Space and Time. Nature 491 (7424), 444–448. doi:10.1038/nature11631
Kim, J. Y., Lockley, M. G., Seo, S. J., Kim, K. S., Kim, S. H., and Baek, K. S. (2012). A Paradise of Mesozoic Birds: The World's Richest and Most Diverse Cretaceous Bird Track Assemblage from the Early Cretaceous Haman Formation of the Gajin Tracksite, Jinju, Korea. Ichnos 19 (1–2), 28–42. doi:10.1080/10420940.2012.660414
LaBarbera, M. (1989). Analyzing Body Size as a Factor in Ecology and Evolution. Annu. Rev. Ecol. Syst. 20 (1), 97–117. doi:10.1146/annurev.es.20.110189.000525
Lim, J.-D., Zhou, Z., Martin, L. D., Baek, K.-S., and Yang, S.-Y. (2000). The Oldest Known Tracks of Web-Footed Birds from the Lower Cretaceous of South Korea. Naturwissenschaften 87 (6), 256–259. doi:10.1007/s001140050715
Liu, Di., Chiappe, L. M., Serrano, F., Habib, M., Zhang, Y., and Meng, Q. (2017). Flight Aerodynamics in Enantiornithines: Information from a New Chinese Early Cretaceous Bird. PLoS One 12 (10), e0184637. doi:10.1371/journal.pone.0184637
Lockley, M. G., and Harris, J. D. (2010). On the Trail of Early Birds: A Review of the Fossil Footprint Record of Avian Morphological and Behavioral Evolution. In Ulrich, P. K. and Willett, J. H. Trends in Ornithology Research, NY: New York, Nova Science Publishers, Inc. 1–63.
Lockley, M. G., Li, R., Harris, J. D., Matsukawa, M., and Liu, M. (2007). Earliest Zygodactyl Bird Feet: Evidence from Early Cretaceous Roadrunner-like Tracks. Naturwissenschaften 94 (8), 657–665. doi:10.1007/s00114-007-0239-x
Marsh, O. C. (1880). Odontornithes: A Monograph on the Extinct Toothed Birds of North America, Vol. 1. Washington, DC: Museum.
Mayr, G. (2016). Avian Evolution: The Fossil Record of Birds and its Paleobiological Significance. NJ: Hoboken, John Wiley & Sons. doi:10.1002/9781119020677
Mayr, G., Kaye, T. G., Pittman, M., Saitta, E. T., and Pott, C. (2020). Reanalysis of Putative Ovarian Follicles Suggests that Early Cretaceous Birds Were Feeding Not Breeding. Scientific Rep. 10 (1), 1–10. doi:10.1038/s41598-020-76078-2
Mayr, G. (2017). Pectoral Girdle Morphology of Mesozoic Birds and the Evolution of the Avian Supracoracoideus Muscle. J. Ornithology 158 (3), 859–867. doi:10.1007/s10336-017-1451-x
Middleton, K. M., and Gatesy, S. M. (2000). Theropod Forelimb Design and Evolution. Zoolog. J. Linn. Soc. 128 (2), 149–187. doi:10.1006/zjls.1998.019310.1111/j.1096-3642.2000.tb00160.x
Mitchell, J. S., and Makovicky, P. J. (2014). Low Ecological Disparity in Early Cretaceous Birds. Proc. R. Soc. B. 281 (1787), 20140608. doi:10.1098/rspb.2014.0608
Navalón, G., Bright, J. A., Marugán‐Lobón, J., and Rayfield, E. J. (2019). The Evolutionary Relationship Among Beak Shape, Mechanical Advantage, and Feeding Ecology in Modern Birds*. Evolution 73 (3), 422–435. doi:10.1111/evo.13655
Nudds, R. L., Dyke, G. J., and Rayner, J. M. V. (2007). Avian Brachial Index and Wing Kinematics: Putting Movement Back into Bones. J. Zoolog. 272 (2), 218–226. doi:10.1111/j.1469-7998.2006.00261.x
Nudds, R. L., Dyke, G. J., and Rayner, J. M. V. (2004). Forelimb Proportions and the Evolutionary Radiation of Neornithes. Proc. R. Soc. Lond. B 271 (Suppl. l_5), S324–S327. doi:10.1098/rsbl.2004.0167
O’Connor, J. K., and Chiappe., L. M. (2011). A Revision of Enantiornithine (Aves: Ornithothoraces) Skull Morphology. J. Syst. Palaeontology 9 (1), 135–157. doi:10.1080/14772019.2010.526639
O’Connor, J. K. (2019). The Trophic Habits of Early Birds. Palaeogeogr. Palaeoclimatol. Palaeoecol. 513, 178–195. doi:10.1016/j.palaeo.2018.03.006
O’Connor, J. K. (2012). A Revised Look at Liaoningornis Longidigitrus (Aves). Vertebrata PalAsiatica 50, 25–37. doi:10.1007/s10336-017-1451-x
O’Connor, J. K., Chiappe, L. M., Gao, C., and Zhao, B. (2011). Anatomy of the Early Cretaceous Enantiornithine Bird Rapaxavis Pani. Acta Palaeontologica Pol. 56 (3), 463–475. doi:10.4202/app.2010.0047
Paradis, E., and Schliep, K. (2019). Ape 5.0: An Environment for Modern Phylogenetics and Evolutionary Analyses in R. Bioinformatics 35 (3), 526–528. doi:10.1093/bioinformatics/bty633
Peters, D. S., and Görgner, E. (1992). “A Comparative Study on the Claws of Archaeopteryx,” in Papers in Avian Paleontology Honoring Pierce Brodkorb. Editor K C Campbell (CA: Los Angeles, Natural History Museum of Los Angeles County Science Series), 36, 29–37.
Pigot, A. L., Miller, E. T., Bregman, T. P., Freeman, B. G., Roll, U., Seddon, N., et al. (2020). Macroevolutionary Convergence Connects Morphological Form to Ecological Function in Birds. Nat. Ecol. Evol. 4 (2), 230–239. doi:10.1038/s41559-019-1070-4
Pike, A. V. L., and Maitland, D. P. (2004). Scaling of Bird Claws. J. Zoolog. 262 (1), 73–81. doi:10.1017/s0952836903004382
Prum, R. O., Berv, J. S., Dornburg, A., Field, D. J., Townsend, J. P., Lemmon, E. M., et al. (2015). A Comprehensive Phylogeny of Birds (Aves) Using Targeted Next-Generation DNA Sequencing. Nature 526 (7574), 569–573. doi:10.1038/nature15697
R Core Team (2020). R: A Language and Environment for Statistical Computing. Vienna, Austria: R Foundation for Statistical Computing. https://www.R-project.org.
Rambaut, A., and Drummond, A. (2015). TreeAnnotator v1.8.2: MCMC Output Analysis. http://beast.bio.ed.ac.uk.
Revell, L. J. (2012). Phytools: An R Package for Phylogenetic Comparative Biology (And Other Things). Methods Ecol. Evol. 3 (2), 217–223. doi:10.1111/j.2041-210X.2011.00169.x
Reyment, R. A., Blackith, R. E., and Campbell, N. A. (1984). Multivariate Morphometrics, Vol. 10. MA: Cambridge, Academic Press.
Richardson, F. (1942). Adaptive Modifications for Tree-Trunk Foraging in Birds. University of California Publications in Zoology. 46 (4). Available at: http://paper/Adaptive-modifications-for-tree-trunk-foraging-in-Richardson/9755cedcf873177efcc883a22360d32b71551cd2
Schneider, C. A., Rasband, W. S., and Eliceiri, K. W. (2012). NIH Image to ImageJ: 25 Years of Image Analysis. Nat. Methods 9 (7), 671–675. doi:10.1038/nmeth.2089
Serrano, F. J., and Chiappe, L. M. (2017). Aerodynamic Modelling of a Cretaceous Bird Reveals Thermal Soaring Capabilities during Early Avian Evolution. J. R. Soc. Interf. 14 (132), 20170182. doi:10.1098/rsif.2017.0182
Serrano, F. J., Palmqvist, P., Chiappe, L. M., and Sanz, J. L. (2017). Inferring Flight Parameters of Mesozoic Avians through Multivariate Analyses of Forelimb Elements in Their Living Relatives. Paleobiology 43 (1), 144–169. doi:10.1017/pab.2016.35
Sheard, C., Neate-CleggNeate-Clegg, M. H. C., Alioravainen, N., Jones, S. E. I., Vincent, C., MacGregor, H. E. A., et al. (2020). Ecological Drivers of Global Gradients in Avian Dispersal Inferred from Wing Morphology. Nat. Commun. 11 (1), 2463. doi:10.1038/s41467-020-16313-6
Tobias, J. A., Ottenburghs, J., and Pigot, A. L. (2020). Avian Diversity: Speciation, Macroevolution, and Ecological Function. Annu. Rev. Ecol. Evol. Syst. 51, 533–560. doi:10.1146/annurev-ecolsys-110218-025023
Wang, Min., and Zhou, Z. (2017). The Evolution of Birds with Implications from New Fossil Evidences. The Biology of the Avian Respiratory System. NY: New York, Springer, 1–26.
Wang, X., and Clarke, J. A. (2015). The Evolution of Avian Wing Shape and Previously Unrecognized Trends in Covert Feathering. Proc. R. Soc. B. 282 (1816), 20151935. doi:10.1098/rspb.2015.1935
Wang, X., McGowan, A. J., and Dyke, G. J. (2011). Avian Wing Proportions and Flight Styles: First Step towards Predicting the Flight Modes of Mesozoic Birds. PLOS ONE 6 (12), e28672. doi:10.1371/journal.pone.0028672
Wang, X., Cau, A., Kundrát, M., Chiappe, L. M., Ji, Q., Wang, Y., et al. (2020). A New Advanced Ornithuromorph Bird from Inner Mongolia Documents the Northernmost Geographic Distribution of the Jehol Paleornithofauna in China. Hist. Biol. 2020, 1–13. doi:10.1080/08912963.2020.1731805
Wang, X., Luis, C. M., Teng, F., and Qiang, J. (2013). Xinghaiornis Lini (Aves: Ornithothoraces) from the Early Cretaceous of Liaoning: An Example of Evolutionary Mosaic in Early Birds. Acta Geologica Sinica-English Edition 87 (3), 686–689. doi:10.1111/1755-6724.12080
Wang, Y., Wang, M., O'Connor, J. K., Wang, X., Zheng, X., and Zhang, X. (2016). A New Jehol Enantiornithine Bird with Three-Dimensional Preservation and Ovarian Follicles. J. Vertebr. Paleontol. 36 (2), e1054496. doi:10.1080/02724634.2015.1054496
Yalden, D. W. (1985). “Forelimb Function in Archaeopteryx,” in The Beginnings of Birds: Proceedings of the International Archaeopteryx Conference, Eichstatt, Germany. Editors M. K. Hecht, J. H. Ostrom, G. Viohl, and P. Wellnhofer (Eichstätt). 1984.
You, H.-l., Lamanna, M. C., Harris, J. D., and Chiappe, L. M. (2006). Jingmai O’Connor, Shu-An Ji, Jun-Chang Lü, Chong-Xi Yuan, Da-Qing Li, and Xing Zhang. A Nearly Modern Amphibious Bird from the Early Cretaceous of Northwestern China. Science 312 (5780), 1640–1643. doi:10.1126/science.1126377
Zhou, S., Zhou, Z., O’Connor, J. K., and O’Connor, (2013). Anatomy of the Basal Ornithuromorph birdArchaeorhynchus Spathulafrom the Early Cretaceous of Liaoning, China. J. Vertebr. Paleontol. 33 (1), 141–152. doi:10.1080/02724634.2012.714431
Zhou, Z. (2006). Evolutionary Radiation of the Jehol Biota: Chronological and Ecological Perspectives. Geol. J. 41 (3–4), 377–393. doi:10.1002/gj.1045
Zhou, Z., Zhang, F., and Li, Z. H. (2009). A New Basal Ornithurine Bird (Jianchangornis Microdonta Gen. et Sp. Nov.) from the Lower Cretaceous of China. Vertebrata PalAsiatica 47 (4), 299–310.
Keywords: aves, mesozoic birds, neornithes, enantiornithes, ornithuromorpha, ecology, ecomorphology, phylogeny
Citation: Bell A, Marugán-Lobón J, Navalón G, Nebreda SM, DiGuildo J and Chiappe LM (2021) Quantitative Analysis of Morphometric Data of Pre-modern Birds: Phylogenetic Versus Ecological Signal. Front. Earth Sci. 9:663342. doi: 10.3389/feart.2021.663342
Received: 02 February 2021; Accepted: 18 June 2021;
Published: 06 July 2021.
Edited by:
Corwin Sullivan, University of Alberta, CanadaReviewed by:
Federico Agnolin, Museo Argentino de Ciencias Naturales Bernardino Rivadavia, ArgentinaRaúl Orencio Gómez, University of Buenos Aires, Argentina
Copyright © 2021 Bell, Marugán-Lobón, Navalón, Nebreda, DiGuildo and Chiappe. This is an open-access article distributed under the terms of the Creative Commons Attribution License (CC BY). The use, distribution or reproduction in other forums is permitted, provided the original author(s) and the copyright owner(s) are credited and that the original publication in this journal is cited, in accordance with accepted academic practice. No use, distribution or reproduction is permitted which does not comply with these terms.
*Correspondence: Alyssa Bell, YWJlbGxAbmhtLm9yZw==