Spatially and Temporally Resolved Monitoring of Glacial Lake Changes in Alps During the Recent Two Decades
- 1School of Geographical Sciences, Nanjing University of Information Science and Technology, Nanjing, China
- 2Key Laboratory of Watershed Geographic Sciences, Nanjing Institute of Geography and Limnology, Chinese Academy of Sciences, Nanjing, China
Climate warming is intensifying the melting of glaciers and the growth of glacial lakes in the Alps, which has a profound impact on the management of water resources and high-mountain hydropower in this region. However, the research on the spatial distribution and temporal evolution of the Alps glacial lakes of various types still lacks a holistic view. In this study, we developed an inventory of Alps glacial lakes of different types and then obtained the annual areas of these lakes from 2000 to 2019 using JRC Global Surface Water and Global Land Analysis and Discovery data at a resolution of 30 m. A total of 498 glacial lakes (>0.01 km2) with the net area of 33.77 ± 6.94 km2 were identified in the Alps in 2019 and are mainly distributed in the western and central Alps. These Alps glacial lakes, with the area ranging 0.01–1.59 km2, are generally dominated by small-sized ones. The comparison of lakes of different types indicated that ice-uncontacted lakes are dominant in number and area, accounting for 59.4 and 58.4%, respectively. In terms of the elevation distribution, almost half of the lakes are concentrated at the altitude of 2,250–2,750 m (a.s.l.). Meanwhile, the mean altitude of small glacial lakes is higher than that of large lakes. The distribution of ice-contacted lakes and supraglacial lakes were more concentrated, and the mean altitude was higher. During the study period, the number, area, and water volume of glacial lakes were increasing, but the expansion varied between different periods. The changing trends of the glacial lake area and volume were consistent and presents in three stages, as the glacial lake expanded rapidly in the first 5 years and in the last 7 years and remained relatively stable between 2005 and 2012. The number and area of glacier-fed lakes increased rapidly, while the non-glacier-fed lakes were relatively stable. The area change rate of supraglacial lakes was the largest (+47%). This study provides a spatially-complete and temporally-consecutive picture of glacial lake changes in the Alps and can be greatly helpful for future research on climate-glacier-lake interactions, glacial lake outburst floods, and freshwater resources in this region.
Introduction
With global warming, the temperature rising is particularly obvious in high-altitude regions, and glaciers show accelerated retreat or thinning (Zemp et al., 2006; Fischer et al., 2015; Zemp et al., 2019). Persistently glacier retreat and meltwater supply promotes the circulation of surface water and increases the abundance of glacial lake (Yao et al., 2018). As the source of precious water resources and mountain flood in high mountain regions, glacial lakes are not only the carrier of environmental information, but also the indicator of climate change (Che et al., 2014). At the same time, glacial lakes promote the melting of alpine glaciers and landscape evolution and acts vital role in surface water cycle and glacier disaster evolution effect (Song et al., 2016; Song et al., 2017). On the one hand, the glacial lake retains considerable glacier meltwater, a feature which plays a temporary role in storing freshwater resources and maintaining sea level (Wang et al., 2020). On the other hand, in view of the global expansion of glacial lakes and the instability of many glacial lakes (Shugar et al., 2020), their unexpected outburst will lead to catastrophic floods which seriously threaten downstream residents, infrastructure, and regional ecological environment security. Therefore, the consecutive monitoring of glacial lake changes is particularly important and urgently needed (Allen et al., 2019; Dubey and Goyal, 2020; Veh et al., 2020).
Given the rapid development of remote sensing technology, varieties of high-resolution satellites and sensors have shown outstanding performance for the identification, extraction, and monitoring of glacial lakes (Petrov et al., 2017; Begam and Sen, 2019). For example, the near five-decade Landsat archive launched by NASA has become the main data source for monitoring glacial lake dynamics. On the basis of remote sensing data, many scholars or institutions have combined research needs to perform glacial lake cataloging, especially for High Mountain Asia (HMA) (Chen et al., 2020; Wang et al., 2020). For example, remote sensing imagery has been employed to map glacial lake inventories for regions of Bhutan (Veettil et al., 2016), Nepal (Khadka et al., 2018), China (Nie et al., 2017), and Patagonia (Wilson et al., 2018) and provides an effective way to reveal the characteristics of glacial lake temporal and spatial changes and understand the response of glacial lakes to climate change, as well as offer a data source for subsequent glacial lake water volume estimation and outburst floods research.
So far, the researches on Alps glacial lakes are sporadic and regional (Buckel et al., 2018), few studies explore the spatial distribution and dense time-series changes of glacial lakes for the entire Alps area, and this shortage leads to the lack of detailed understanding of the changes of glacial lakes in this region. Recent glacier studies indicate a rapid retreat of Alps glaciers (–39 km2 a−1) and ice thickness variations (–0.5 m a−1 to –0.9 m a−1) (Huss, 2012; Zekollari et al., 2018; Sommer et al., 2020). Rapidly melt of Alps glaciers may cause substantial changes in glacial lakes during the past several decades. Besides, lakes connected to glaciers provide positively feedback on the glacier system, resulting in changes in mass balance, and enhanced melting (Song et al., 2017). In order to deepen the understanding of the impact of glacial lakes on mountain environment and the response to glacier change, detailed data on glacial lake evolution and their spatially and temporally heterogeneous changing characteristics are needed. Here, we aim to develop an inventory of Alps glacial lakes with the maximum inundation distribution at 30 m resolution and further characterize the annual variations of glacial lakes from 2000 to 2019. The specific objectives of this research are as follows: 1) to map the glacial lake inventory of annual time series in the Alps region over the past 20 years to fill the gap in a complete glacial lake inventory of the entire region; 2) to reveal the heterogeneous changes of glacial lakes of different types, scales, and elevation distributions in the Alps; 3) to provide open access to the resulting inventory data set so as to offer geographic information on glacial lakes and improve awareness of the Alps glacial lakes.
Study Area and Data
Study Area
The Alps is the highest mountain range in Europe and is located in southern Europe and known as the “European water tower” (Figure 1). The Alps accounts for only 11% of Europe’s total area, but provides more than 90% of the water supply of Europe, especially in arid regions and in summer (Vanham, 2012). The mountain ranges arc in shape and extend from west to east, starting from the Mediterranean coast near Nice in southeast France in the West and ending in the Vienna basin in Austria in the East (Paul et al., 2020). The mountain ranges are 1,200 km long and 130–260 km wide, with a mean altitude of approximately 3,000 m and a total area of up to 220000 km2 (Auer et al., 2007; Zemp et al., 2015). The highest peak is Mont Blanc (4,808 m).
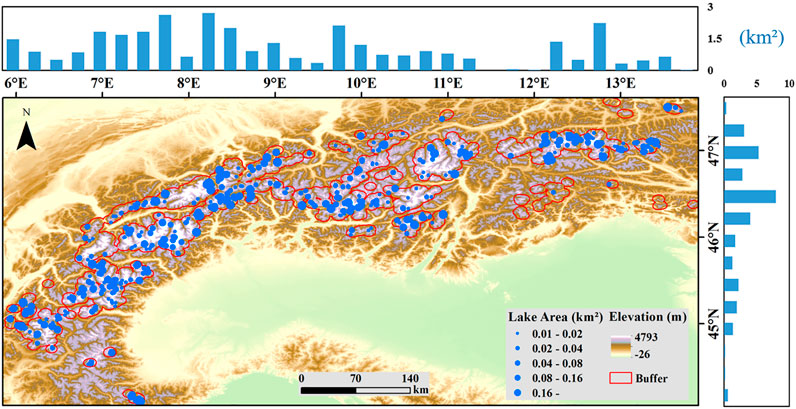
FIGURE 1. Spatial distribution of Alps glacial lakes in 2019. The upper and right panels show the statistics of and glacial lake area within the 5 km buffer of glacier terminus at 0.25° latitude/longitude summaries.
Located between temperate and subtropical latitudes, the Alps is the boundary between the temperate continental humid climate of central Europe and the summer dry climate of the subtropical southern Europe. The peak is cold all year round, and the annual mean temperature is 0°C at the elevation of 2000 m, and the annual precipitation is up to 1,200–2000 mm (Frei et al., 2003). The annual precipitation in the edge area varies significantly from that in the interior of the mountains. The elevation of 3,000 m is the maximum precipitation zone, and the annual precipitation in the high mountains exceeds 2,500 mm (Vanham, 2012; Ács et al., 2018). The area is home to more than 1,000 modern glaciers covering a total area of 3,600 km2. These glaciers were mostly shrinking and thinning rapidly, and substantial glacial meltwater provide continuous supply for the development of glacial lakes (Paul et al., 2020).
Data Source
We developed our glacial lake inventory of the Alps according to the JRC Global Surface Water (GSW) and the Global Land Analysis & Discovery (GLAD) data stored in the Google Earth Engine (GEE, https://earthengine.google.com/) cloud computing platform for free to global users. The GSW is a spatial and temporal distribution map of the global surface water generated by Pekel et al. (2016) using 4,185,439 scenes of Landsat images acquired between 1984 and 2019 and provides spatial raster data on the water extent and variations. Similar to the GSW data set, the GLAD data (https://www.glad.umd.edu/dataset/global-surface-water-dynamics) is generated by Pickens et al. (2020) using a classification tree to categorize the land and water in all Landsat scenes from 1999 to 2020 and perform a time-series analysis to produce maps that characterize inter-annual and intra-annual open surface water dynamics. The spatial resolution of both datasets is 30 m. In this study, the annual data layers of the GSW (JRC Yearly Water Classification History, v1.2) as the primary data source were used to map the annual areas of glacial lakes in the Alps. To ensure the accuracy of the glacial lake inventory, we use GLAD annual data as supplementary information for manually inspecting and editing the mapping results from the GSW.
In addition, the Shuttle Radar Topography Mission (SRTM V3) digital elevation data provided by NASA JPL (http://gdex.cr.usgs.gov/gdex/) with a spatial resolution of up to 1 arc-second are used to extract the elevation of the glacial lakes. RGI 6.0 glacier data (https://www.glims.org/RGI/) are utilized to determine the buffer range of glacial lake data distribution.
Methods
Glacial Lake Area Extraction
At present, the extraction methods of glacial lakes are mainly divided into two categories: automatic mapping and manual visual interpretation. Automatic extraction algorithms have the advantages of being relatively efficient and less labor-consuming, but manual visual interpretation has higher accuracy and ease of implementation (Huang et al., 2018); moreover, the latter is widely used in different regions for delineating the extent of glacial lakes (Wang et al., 2013a; Zhang et al., 2015; Wang et al., 2020). The public release of the GSW and GLAD data provide a great convenience for extracting information on glacial lakes. However, the dataset maps all surface water bodies of different types, e.g., lakes, rivers, floodplains, and paddy water bodies. Therefore, many non-lake water bodies were excluded from glacial lakes in the study area. Manual inspection can also largely reduce influences of mountain shadows and exclude other types of water bodies (such as rivers, and tectonic lakes).
The research on glacial lake has been carried out over a long period, but a consensus on the remote sensing-based specific standards of glacial lake categorization is yet to be reached. Researchers typically combine their research purposes and the geographical conditions of the study area to establish some thresholds to differentiate the glacial lakes from other natural or artificial lakes (Li et al., 2020). Prior studies emphasize the relationship between glacial lakes and glaciers and suggest that lakes within 3, 5, or 10 km from the margin of glaciers are regarded as glacial lakes (Wang et al., 2013b; Worni et al., 2013; Veh et al., 2018). Others define glacial lakes by setting altitude thresholds and other parameters (Raj and Kumar, 2016; Wang et al., 2017). The extraction of glacial lakes based on altitude threshold is not universal, and the approach of establishing distance buffer is still a common method to extract and assist glacial lake recognition. According to the visual judgement of numerous glacial lake cases on the geographical location of Alps glaciers and surrounding lakes, we determine that it is reasonable to map glacial lakes within the distance of 5 km from the glacier termini. We also conducted a procedure of quality control on the mistaken or missed glacial lakes around the buffering distance by manual inspection. In addition, the glacial lake inventory aims to provide basic data for the management of freshwater resources in alpine regions and the research of glacial lake outburst flood (GLOFs), glacial lakes with an area of more than 0.01 km2 are considered to be a kind of lakes that pose a threat to downstream facilities when dam break occurs (Worni et al., 2013; Veh et al., 2020)). Hence, we established a 5-km buffer of distance to upstream glaciers and set a threshold of 0.01 km2 as the minimum area of Alps glacial lakes to exclude other water bodies in the region. In other words, we defined glacial lake as natural lake formed by glaciation, with an area of greater than 0.01 km2 within 5 km from the terminal of the glacier and then employ the high-resolution Google Earth imagery to assist in the manual identification for improved accuracy of the glacial lake inventory.
To estimate the long-term change rates of area time series, a simple and robust strategy is to calculate the annual change rate by referring to the observed values in starting year and ending year. Specifically, the area change rate of the glacial lake in each period is obtained via the difference between the starting and ending lake area divided by the number of years between them, and the corresponding standard error is obtained by combining the annual glacial lake area with the multi-year average area.
Glacial Lake Classification
Prior studies categorize glacial lakes according to their physical characteristics, including the glacial lake size, the geometrical relationship with modern glaciers, the geographical location of the lake, and whether or not the lake is being fed by glacial meltwater (Lizong et al., 2011; Song and Sheng, 2016; Yao et al., 2018). Different researchers classify glacial lakes according to their research aims or focuses. The common approaches can be summarized as two systems. One method is implemented on the basis of the relationship between the glacial lake and glacier. For example, to examine the inter-annual variation characteristics of glacial lakes in HMA, Chen et al. (2020) classified glacial lakes into four categories: 1) pro-glacial lake, 2) supraglacial lake, 3) unconnected glacial lake, and 4) ice-marginal lake. The other method is in accordance with the formation mechanism of glacial lakes (Wilson et al., 2018), which enables them to be divided into 1) glacial erosion lakes, 2) moraine-dammed lakes, and 3) ice-blocked lake, among others. Evaluating the formation mechanism of glacial lakes on the basis of remote sensing images only is generally difficult. The classification system of glacial lakes used in this study is developed according to the relationship between glacial lakes and glaciers, as this mechanism reflects the important role that glaciers play in the formation of glacial lakes. Wang et al. (2020) provides a practical reference for our classification.
In this research, we divided glacial lakes into four categories: 1) glacier-fed lakes (GFL, lakes fed by modern glacier meltwater) which were further divided into 1) ice-uncontacted lakes (IUL, lakes not in contact with glaciers but fed by glacier meltwater), 2) ice-contacted lakes (ICL, lakes that are in close contact with a glacier terminal), and 3) supraglacial lakes (SGL, lakes developed on glacier surfaces); and 2) non-glacier-fed lakes (NGFL, lakes are formed due to the historical glacial movements and are not fed by modern glacial meltwater). To obtain reliable classification results, the glacial lakes are manually distinguished by using high-resolution imagery from Google and Bing and the glacier data of RGI 6.0 (Pfeffer et al., 2014).
Uncertainty Estimation
Given the impact of remote sensing image quality (such as spatial resolution, mountain shadow, cloud cover, and water extraction algorithm, etc.,), the interpreters’ prior knowledge, and the definition of glacial lake, errors inevitably occur when extracting glacial lake information (Zhang et al., 2015; Li et al., 2020). In this study, we used a semiautomatic method to map the glacial lake inventory, and every lake was manually validated and edited. However, no appropriate and quantifiable uncertainty estimation exists for the error caused by artificial correction process and the definition of glacial lake. To improve the credibility of the inventory, recheck it is necessary. Previous studies have shown that the mixed pixel is the key factor of error sources (Mixed pixel refers to the existence of different types of ground objects in a pixel, mainly at the boundary of ground types. The existence of mixed pixels is one of the main factors affecting the recognition and classification accuracy) (Hanshaw and Bookhagen, 2014). In other words, the error is mainly caused by the spatial resolution of the image (Wang et al., 2020). Using the buffer zone of a half-pixel distance outside the boundary is optimal to evaluate the uncertainty (Fujita et al., 2009; Salerno et al., 2012). Therefore, the uncertainty of a single lake area within 1 standard deviation (1σ) can be expressed as (Hanshaw and Bookhagen, 2014):
where
Results and Discussions
Characteristics of the Spatial Distribution of Glacial Lakes in the Alps
A total of 498 glacial lakes (>0.01 km2) were identified in 2019 by using remote sensing data products and cover a net area of 33.77 ± 6.94 km2. Glacial lakes are widely distributed across the Alps, and several of the largest glacial lakes (>1 km2) in the study area are mainly concentrated in the western and central regions (Figure 1). On the whole, the Alps are dominated by small glacial lakes, and all glacial lakes are in the size range of 0.01–1.59 km2. Glacial lakes less than 0.1 km2 account for 86.3% of all the glacial lakes of the inventory in number, but their area is only 41.8% of the total. Only 68 glacial lakes exceed 0.1 km2, yet they account for 58.2% of the total area. Therefore, although many small glacial lakes exist in the Alps, the total area of glacial lakes in the region is still dominated by relatively large lakes.
Figure 2 presents the spatial distribution of glacial lakes of different types. GFL are dominant in number and area, and NGFL are in the minority. Specifically, the number of IUL take the leading position, accounting for approximately 59.4%. NGFL, ICL and SGL account for 20.4, 11.4 and 8.8%, respectively. In terms of area, the four types (IUL, NGFL, ICL, and SGL) of glacial lakes account for 58.4, 12.6, 20.4, 8.6, and 12.6%, respectively. Compared with other lakes, ICL have larger sizes, probably because they are connected with the glacier termini and can evolve with the retreating of glaciers. Compared with ICL, SGL do not exist all year round and their area fluctuation is evident.
The elevation bins by the step of 250 m were defined to analyze the dependence of glacial lake distribution on elevation. Figure 3 shows the distribution of the Alps’ glacial lakes in different elevation zones. As the altitude increases, the quantity and area of glacial lakes are approximately normally distributed. The altitudes of all glacial lakes mapped in 2019 ranged from 1,060 m a.s.l. (above sea level) to 3,133 m a.s.l., of which 72% are distributed at the altitude belt of 2,250–2,750 m a.s.l., with a mean of 2,410 m. The lakes between 2000 and 2,500 m also have the largest proportion in area, accounting for 70.3%. Further analysis reveals that in different elevation bins, the distribution of glacial lakes at different sizes is also uneven and vary greatly (Figure 3C). Glacial lakes predominantly range from 2000 m to 2,750 m, and most are small lakes (≤0.04 km2), with the larger lakes distributed in relatively low altitude regions (Song and Sheng, 2016) mainly because the land is flat in the area with low altitude, thereby providing a good water storage environment for some large NGFL and a few IUL.
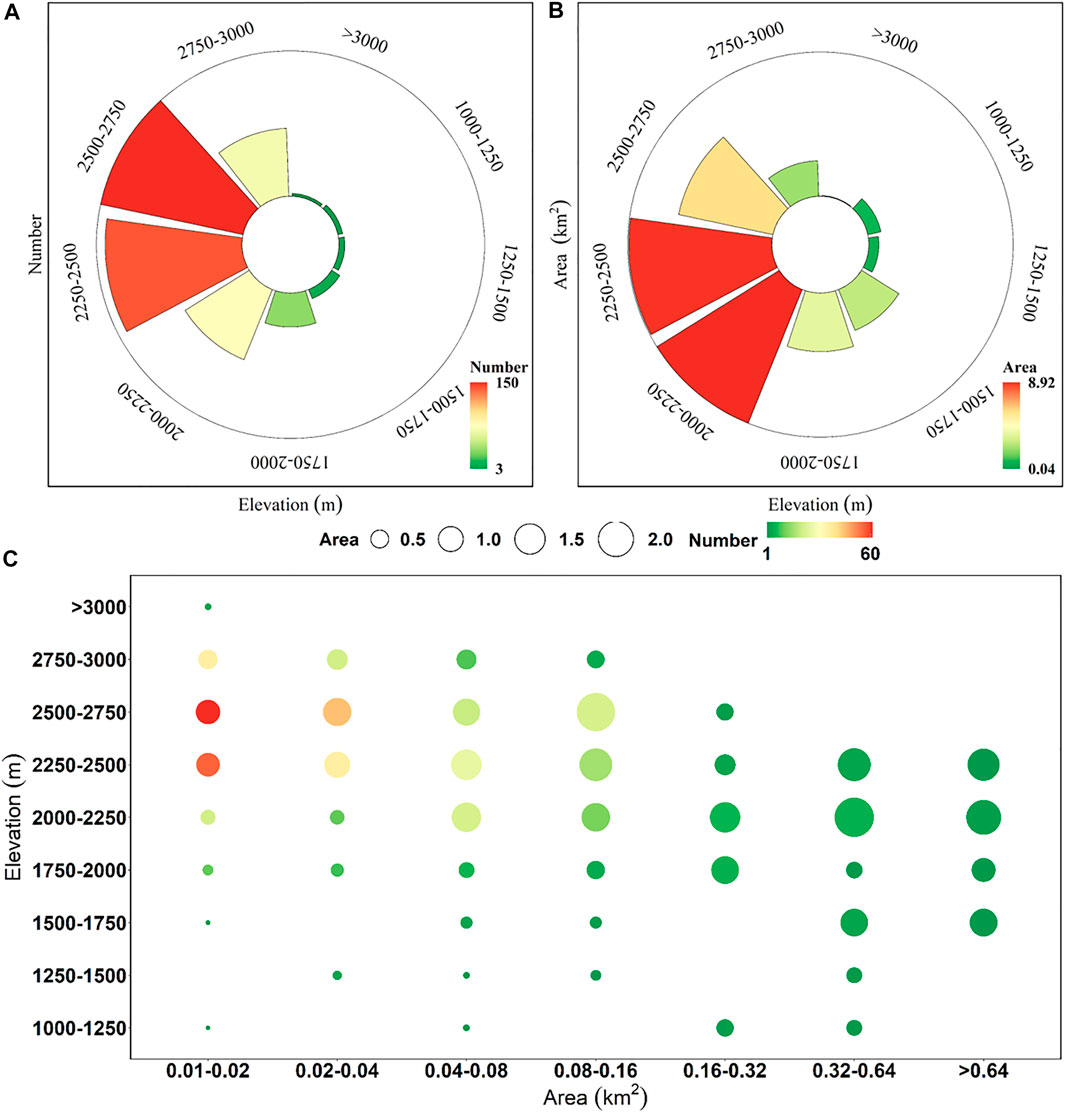
FIGURE 3. Number (A) and area (B) of glacial lakes grouped into different elevation bins, (C) the distribution of glacial lakes at different scales in 2019.
In addition, the distribution of glacial lakes varies with the types of glacial lakes given the influence of glacial movement and action (Figure 4). Among the lakes, ICL and SGL are closely related to glaciers and therefore have a higher and more concentrated elevation distribution, with a mean elevation of approximately 2,600 m a.s.l. However, IUL and NGFL have more discrete elevation distributions, with lower mean elevations (2,400 m and 2,100 m, respectively). NGFL are particularly prominent as products of pre-glacial movement.
Number and Area Changes of Glacial Lakes in the Alps
We further obtained the annual glacial lake areas (>0.01 km2) in the Alps from 2000 to 2019. During that period, the number and area of glacial lakes present an ascending tendency (Figure 5), yet the increasing rates varied between different periods. There were 485 and 498 glacial lakes in 2000 and 2019, respectively. The total area of the glacial lakes in the study region increased from 29.78 ± 6.48 km2 in 2000 to 33.77 ± 6.94 km2 in 2019 and expanded by approximately 3.99 ± 0.46 km2 (by 13%). During the sub-period of 2000–2004, the quantity and total area of glacial lakes presented relatively more rapid increases, with an increase of 1.74 ± 0.21 km2 (5.8%) and an annual growth rate of 0.44 ± 0.07 km2 a−1. During 2005–2012, the number and area of glacial lakes were relatively stable. From 2013 to 2019, the number of glacial lakes changed gradually and the area increased rapidly, increasing by 1.68 ± 0.20 km2 (5.2%) with an annual growth rate of 0.28 ± 0.05 km2 a−1.
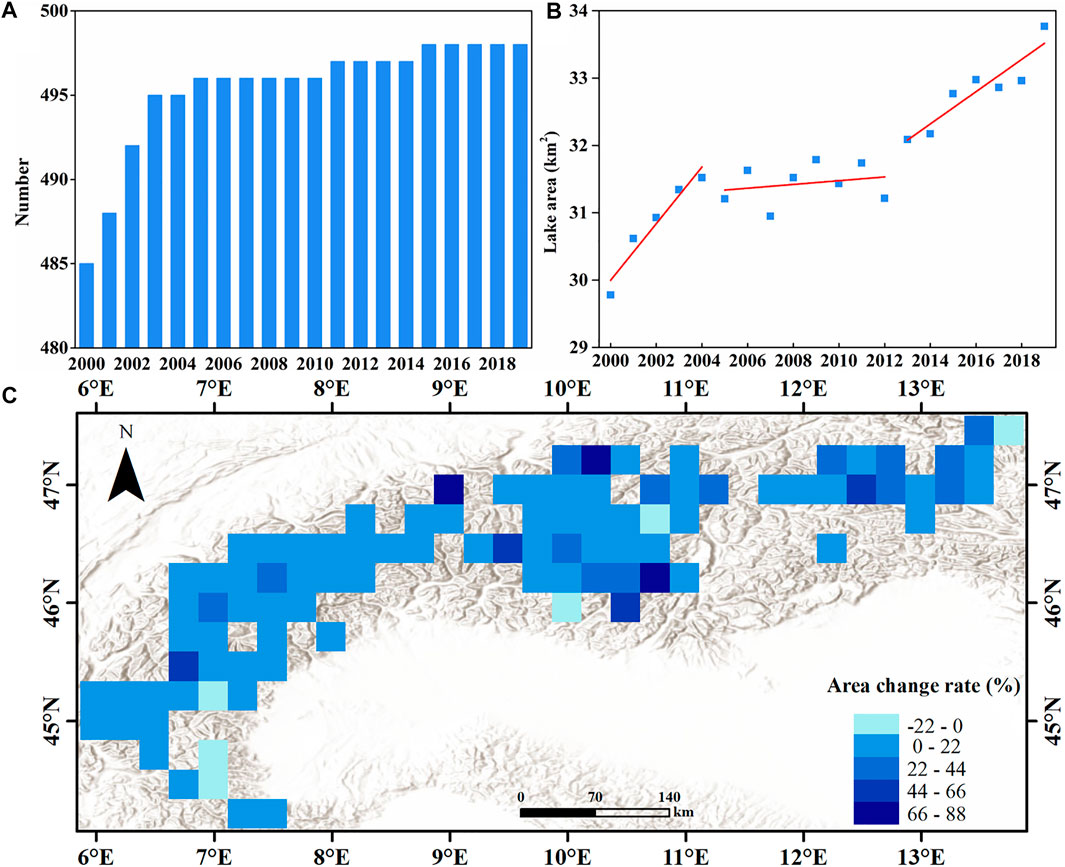
FIGURE 5. Changes in the number (A) and area (B) of glacial lakes in the Alps from 2000 to 2019 and percent area change, (C) per 2.5° latitude and longitude bins with 2.5° latitude/longitude summaries.
Overall, the majority of glacial regions experienced glacial lake growth during the study period (Shugar et al., 2020), particularly in the central Alps. However, some separate districts recorded a decrease in glacial lake area (light-blue grid cell in Figure 5C) and were mainly affected by some large NGFL.
Globally, the number of available lake inventories has gradually increased recently, the glacial lake inventory from individual basin (Kumar et al., 2020) to long-time series and global scale (Shugar et al., 2020) deepens the understanding of the evolution differences of glacial lakes in different regions, so as to better constrain and simulate the hydrological response of glaciers to climate change (Wood et al., 2021). we discover that, compared with those of other regions with glacial lake distributions such as the Andes (the total area of glacial lake increased by 14% from 2000 to 2016, excluding Lake Argentino, Lake Viedma, Lake O'Higgins/San Martín) (Wilson et al., 2018) and Nepal (increased by approximately 17% from 1997 to 2017) (Haritashya et al., 2018), the change rate of Alps’ glacial lakes in the recent years seems to be relatively slow. However, compared with Alaska (Shugar et al., 2020) and Himalayas (increased by 5% from 2000 to 2015) (Nie et al., 2017), the change rate seems to be relatively fast (Due to the difference of glacial lake identification and statistical years, the actual results may be a little biased.).
Changes in the Water Volume of Glacial Lakes
The “real” water volume of a glacial lake can be calculated by combining the water level and shoreline data and accurate lake bathymetry. However, most glacial lakes are located in harsh environments and remote areas, making the field measurements of glacial lake depths difficult and infeasible. Therefore, the empirical model fitted by the relationship of glacial lake area-depth-volume is a commonly-used method for estimating the glacial lake water volume on regional scale. The water volume estimation model (Eq. 3) of glacial lakes proposed by Cook and Quincey, (2015) has strong applicability to the Alps.
Where V and A represent the water volume and area of each lake, respectively. Substantial retreat and downwasting of glaciers have occurred in the Alps since the Little Ice Age due to global warming and is expected to exacerbate. Accelerated glacier shrinkage has been reported over the past few decades. In the first 5 years of the 21st Century, the mass-change rate was close to −1 m w.e.a−1 (Huss, 2012); from 2000 to 2012, it was –0.71 m w.e.a−1 (Sommer et al., 2020). The glacier mass change rate decreased to –0.5 m w.e.a−1 from 2006 to 2016 (Zemp et al., 2019). Then, the glacier mass entered a period of rapid retreat (Zekollari et al., 2018; Carrivick et al., 2020), this outcome is consistent with research findings which show several years of record-breaking volume loss and glacier retreat after 2012 (2015 and 2018) (Zekollari et al., 2020). Referring to the glacier mass changes reported recently suggest that the changing tendency of glacial lakes in the Alps is generally consistent with the temporal patterns of negative glacier mass balance. The volume change of a glacial lake was similar to its area change and generally behaves in a three-stage evolution trajectory (Figure 6). During the study period, lake volumes increased from 0.57 to 0.66 km3 mainly in the two sub-periods of 2000–2004 and 2013–2019, with growth rates of 5 and 8%, respectively. From 2005 to 2012, the total water volume of glacial lakes was relatively stable.
Heterogeneous Changes of Glacial Lakes by Different Types
Differences in the area changes of the four types of glacial lakes were further examined as illustrated in Figure 7. During the study period, the number and area of GFL increased rapidly because of the influence of glacier retreating and glacial meltwater supply, while that of NGFL were relatively stable (Figure 7). The number of GFL increased from 384 in 2000 to 397 in 2019, and their area expanded from 25.85 ± 5.39 km2 in 2000 to 29.52 ± 5.81 km2 in 2019, with an increase of 3.67 km2 (+14.2%). By contrast, the differences in the numbers (+0) and area (+0.32 km2, +8%) of NGFL during 2000–2019 varied slightly given the absence of the influence of modern glaciation.
For the past 2 decades, SGL were closely related to glacier changes and were vulnerable to glacier ablation and precipitation given their small sizes. As a result, they have the highest rate of change in area (+47%), as well as large inter-annual fluctuations in area. Except for the case with SGL, the glacier terminus retreats faster and the glacial meltwater increases due to the rising temperature, thereby providing a favorable condition for the expansions of IUL and ICL (their area growth percentages were 11 and 12%, respectively). A feedback relationship also occurs between glacial lakes and glaciers. First, as the product of glacier movement, glacial lakes receive energy and materials transported by glaciers and can highly respond to the changes of glaciers. Second, the expansion of glacial lakes connected with glaciers accelerated the melting of glaciers while also indirectly promoting the appearance of new and higher elevation glacial lakes (mainly ICL) at the end of the glacier and the conversion between glacial lakes (transformation from SGL to ICL or ICL to IUL).
Comparison of Inventoried Glacial Lakes With Previous Study
We compared our glacial lake inventory with that of Buckel et al. (2018) for the same period over the approximately same extent. Our inventory includes 84 glacial lakes (≥0.02 km2) with a total area of 6.33 ± 1.28 km2, while the artificially interpreted inventory of Buckel et al. (2018) contains 105 glacial lakes (≥0.02 km2) with a total area of 6.26 km2. The differences of glacial lakes between these two inventories are 21 and 0.07 km2 in the total number and area, respectively. Some of reasons for the missed glacial lakes in our inventory are the interference of some not-so-great observations (shade or snow), identification criteria for glacial lakes, and a few glacial lakes that have dried up. The major reason for the shortage of glacial lake inventory is the difference of extraction methods. Different from our glacial lake inventory, Buckel mapped Austria glacial lakes by manual digitization using multi- source remote sensing image, such as Google Earth, Esri World Imagery, and Orthofoto Österreich.
In short, the semi-automatic method shows unprecedented advantages in glacial lake extraction efficiency. However, in terms of extraction accuracy, it is slightly insufficient compared with the manual digitization method. It cannot be ignored that the integrity and availability of data sources fundamentally affect the extraction of glacial lakes. Lake mapping using satellite images and orthophotos from multiple sources from different years is also a vital channel to map a complete glacial lake inventory.
Conclusion
Glacial lakes in the alpine regions are highly sensitive to glacier mass evolution and climate change. However, the research on the spatial distribution and temporal evolution of various types of glacial lakes in the Alps still lacks a holistic view. Through a semi-automatic approach, we used remote sensing products (the GSW and GLAD) to map annual glacial lakes and compiled an inventory of these lakes in the Alps. Our results show that 498 glacial lakes (>0.01 km2) were identified in 2019 using remote sensing data within the study area, covering a total area of 33.77 ± 6.94 km2. The glacial lakes are widely distributed and concentrated mainly in the western and central regions of the Alps. On the whole, the Alps are dominated by small glacial lakes in number, with all glacial lakes in the size range of 0.01–1.59 km2. The differences among different types of glacial lakes are also significant. Glacier-fed lakes are dominant in both number and total area, and non-glacier-fed lakes are in the minority. Among all the lakes, ice-uncontacted lakes occupy the leading position in number and area, accounting for 59.4 and 58.4%, respectively. In terms of the elevation distribution, almost half of the lakes are concentrated between 2,250 and 2,750 m above sea level. Meanwhile, the mean elevation of the small glacial lakes is higher, and the larger lakes are distributed in relatively low altitude regions. The distributions of ice-contacted lakes and supraglacial lakes were more concentrated, and their mean elevations were higher. However, ice-uncontacted lakes and non-glacier-fed lakes have more discrete altitude distributions with lower mean elevations.
Referring to the glacier mass changes reported recently indicate that the changing tendency of glacial lakes in Alps is generally consistent with the temporal patterns of negative glacier mass balance. Glacier retreating and additional meltwater supply could play a leading role in the area expansion of most glacial lakes. During the study period, the quantity and area of glacial lakes show an increasing tendency, while the expansion varied between different periods. The area shows a three-stage trend whereby the glacial lake expansion is fast in the first 5 years and the last 7 years (with an annual growth rate of 0.44 ± 0.07 km2 a−1 and 0.28 ± 0.05 km2 a−1) and remained relatively stable between 2005 and 2012. The water volume and area of the glacial lake in the region are similar and reveal a consistent trend of change.
The number and area of glacier-fed lakes increased rapidly during the study period because of the influence of glacier retreat and glacial meltwater supply, while those of non-glacier-fed lakes were relatively stable. For the past 2 decades, the area change rate of supraglacial lakes is the largest percentage (+47%). Given the rapid melting of glaciers, several glacial lakes are undergoing irreversible changes.
In summary, this study attempted to produce a complete glacial lake inventory in the Alps and examined the dense time-series of lake area changes linked to glacier retreating through detailed classification of glacial lakes. This work is expected to provide basic data and information for cryospheric hydrology research, water resource utilization and management, and glacier hazard assessment in the Alps.
Data Availability Statement
The original contributions presented in the study are included in the article/Supplementary Material, further inquiries can be directed to the corresponding author.
Author Contributions
JM and CS led the writing of the manuscript. YW made valuable comments on the manuscript. All co-authors contributed to the manuscript.
Funding
This work was partly funded by the Second Tibetan Plateau Scientific Expedition and Research (2019QZKK0202), the National Key Research and Development Program of China (Grant No. 2019YFA0607101, 2018YFD0900804, 2018YFD1100101), the Thousand Young Talents Program in China (Grant No. Y7QR011001), the Strategic Priority Research Program of the Chinese Academy of Sciences (Grant No. XDA23100102), and the National Natural Science Foundation of China (No. 41971403, 41801321).
Conflict of Interest
The authors declare that the research was conducted in the absence of any commercial or financial relationships that could be construed as a potential conflict of interest.
Publisher’s Note
All claims expressed in this article are solely those of the authors and do not necessarily represent those of their affiliated organizations, or those of the publisher, the editors and the reviewers. Any product that may be evaluated in this article, or claim that may be made by its manufacturer, is not guaranteed or endorsed by the publisher.
Acknowledgments
We are grateful to the satellite data providers: USGS for Landsat, JPL/NASA for SRTM, and EARTHDATA for RGI. We are also grateful to YW for numerous fruitful discussions, advice, and comments on the present article. Finally, we are grateful to the scientific editor and the reviewers for their careful review of the article used to improve the study.
Supplementary Material
The Supplementary Material for this article can be found online at: https://www.frontiersin.org/articles/10.3389/feart.2021.723386/full#supplementary-material
References
Ács, F., Takács, D., Breuer, H., and Skarbit, N. (2018). Climate and Climate Change in the Austrian-Swiss Region of the European Alps During the Twentieth Century According to Feddema. Theor. Appl. Climatol. 133, 899–910. doi:10.1007/s00704-017-2230-6
Allen, S. K., Zhang, G., Wang, W., Yao, T., and Bolch, T. (2019). Potentially Dangerous Glacial Lakes Across the Tibetan Plateau Revealed Using a Large-Scale Automated Assessment Approach. Sci. Bull. 64, 435–445. doi:10.1016/j.scib.2019.03.011
Auer, I., Böhm, R., Jurkovic, A., Lipa, W., Orlik, A., Potzmann, R., et al. (2007). HISTALP-Historical Instrumental Climatological Surface Time Series of the Greater Alpine Region. Int. J. Climatol. 27, 17–46. doi:10.1002/joc.1377
Begam, S., and Sen, D. (2019). Mapping of Moraine Dammed Glacial Lakes and Assessment of Their Areal Changes in the Central and Eastern Himalayas Using Satellite Data. J. Mt. Sci. 16, 77–94. doi:10.1007/s11629-018-5023-1
Buckel, J., Otto, J. C., Prasicek, G., and Keuschnig, M. (2018). Glacial Lakes in Austria - Distribution and Formation Since the Little Ice Age. Glob. Planet. Change. 164, 39–51. doi:10.1016/j.gloplacha.2018.03.003
Carrivick, J. L., James, W. H. M., Grimes, M., Sutherland, J. L., and Lorrey, A. M. (2020). Ice Thickness and Volume Changes across the Southern Alps, New Zealand, from the Little Ice Age to Present. Sci. Rep. 10, 13392. doi:10.1038/s41598-020-70276-8
Che, T., Xiao, L., and Liou, Y.-A. (2014). Changes in Glaciers and Glacial Lakes and the Identification of Dangerous Glacial Lakes in the Pumqu River Basin, Xizang (Tibet). Adv. Meteorology. 2014, 1–8. doi:10.1155/2014/903709
Chen, F., Zhang, M., Guo, H., Allen, S., Kargel, J. S., Haritashya, U. K., et al. (2020). Annual 30-Meter Dataset for Glacial Lakes in High Mountain Asia From 2008 to 2017. Earth Syst. Sci. Data. 13, 741–766. doi:10.5194/essd-2020-57
Cook, S. J., and Quincey, D. J. (2015). Estimating the Volume of Alpine Glacial Lakes. Earth Surf. Dynam. 3, 559–575. doi:10.5194/esurf-3-559-2015
Dubey, S., and Goyal, M. K. (2020). Glacial Lake Outburst Flood Hazard, Downstream Impact, and Risk over the Indian Himalayas. Water Resour. Res. 56, e2019WR026533. doi:10.1029/2019wr026533
Fischer, M., Huss, M., and Hoelzle, M. (2015). Surface Elevation and Mass Changes of All Swiss Glaciers 1980-2010. The Cryosphere. 9, 525–540. doi:10.5194/tc-9-525-2015
Frei, C., Christensen, J. H., Déqué, M., Jacob, D., Jones, R. G., and Vidale, P. L. (2003). Daily Precipitation Statistics in Regional Climate Models: Evaluation and Intercomparison for the European Alps. J. Geophys. Res. 108, a–n. doi:10.1029/2002JD002287
Fujita, K., Sakai, A., Nuimura, T., Yamaguchi, S., and Sharma, R. R. (2009). Recent Changes in Imja Glacial Lake and its Damming Moraine in the Nepal Himalaya Revealed by In Situ Surveys and Multi-Temporal ASTER Imagery. Environ. Res. Lett. 4, 045205. doi:10.1088/1748-9326/4/4/045205
Hanshaw, M. N., and Bookhagen, B. (2014). Glacial Areas, lake Areas, and Snow Lines From 1975 to 2012: Status of the Cordillera Vilcanota, Including the Quelccaya Ice Cap, Northern central Andes, Peru. The Cryosphere. 8, 359–376. doi:10.5194/tc-8-359-2014
Haritashya, U., Kargel, J., Shugar, D., Leonard, G., Strattman, K., Watson, C., et al. (2018). Evolution and Controls of Large Glacial Lakes in the Nepal Himalaya. Remote Sensing. 10, 798. doi:10.3390/rs10050798
Huang, C., Chen, Y., Zhang, S., and Wu, J. (2018). Detecting, Extracting, and Monitoring Surface Water From Space Using Optical Sensors: A Review. Rev. Geophys. 56, 333–360. doi:10.1029/2018rg000598
Huss, M. (2012). Extrapolating Glacier Mass Balance to the Mountain-Range Scale: the European Alps 1900-2100. The Cryosphere. 6, 713–727. doi:10.5194/tc-6-713-2012
Khadka, N., Zhang, G., and Thakuri, S. (2018). Glacial Lakes in the Nepal Himalaya: Inventory and Decadal Dynamics (1977-2017). Remote Sensing. 10, 1913. doi:10.3390/rs10121913
Kumar, R., Bahuguna, I. M., Ali, S. N., and Singh, R. (2020). Lake Inventory and Evolution of Glacial Lakes in the Nubra-Shyok Basin of Karakoram Range. Earth Syst. Environ. 4, 57–70. doi:10.1007/s41748-019-00129-6
Li, D., Shangguan, D., and Anjum, M. N. (2020). Glacial Lake Inventory Derived From Landsat 8 OLI in 2016-2018 in China-Pakistan Economic Corridor. Inter. J. Geo-Info. 9, 294. doi:10.3390/ijgi9050294
Lizong, W., Xin, L., Shiyin, L., Mool, P. K., Joshi, S., and Bajracharya, S. (2011). “Remote Sensing Based Glacial Lake Inventory in the Hindu Kush-Himalaya Region,” in Paper presented at the 2011 International Conference on Remote Sensing, Environment and Transportation Engineering.
Nie, Y., Sheng, Y., Liu, Q., Liu, L., Liu, S., Zhang, Y., et al. (2017). A Regional-Scale Assessment of Himalayan Glacial Lake Changes Using Satellite Observations From 1990 to 2015. Remote Sensing Environ. 189, 1–13. doi:10.1016/j.rse.2016.11.008
Paul, F., Rastner, P., Azzoni, R. S., Diolaiuti, G., Fugazza, D., Le Bris, R., et al. (2020). Glacier Shrinkage in the Alps Continues Unabated as Revealed by a New Glacier Inventory From Sentinel-2. Earth Syst. Sci. Data. 12, 1805–1821. doi:10.5194/essd-12-1805-2020
Pekel, J.-F., Cottam, A., Gorelick, N., and Belward, A. S. (2016). High-Resolution Mapping of Global Surface Water and its Long-Term Changes. Nature. 540, 418–422. doi:10.1038/nature20584
Petrov, M. A., Sabitov, T. Y., Tomashevskaya, I. G., Glazirin, G. E., Chernomorets, S. S., Savernyuk, E. A., et al. (2017). Glacial Lake Inventory and Lake Outburst Potential in Uzbekistan. Sci. Total Environ. 592, 228–242. doi:10.1016/j.scitotenv.2017.03.068
Pfeffer, W. T., Arendt, A. A., Arendt, A., Bolch, T., Cogley, J. G., Gardner, A. S., et al. (2014). The Randolph Glacier Inventory: a Globally Complete Inventory of Glaciers. J. Glaciol. 60, 537–552. doi:10.3189/2014JoG13J176
Pickens, A. H., Hansen, M. C., Hancher, M., Stehman, S. V., Tyukavina, A., Potapov, P., et al. (2020). Mapping and Sampling to Characterize Global Inland Water Dynamics from 1999 to 2018 With Full Landsat Time-Series. Remote Sensing Environ. 243, 111792. doi:10.1016/j.rse.2020.111792
Raj, K. B. G., and Kumar, K. V. (2016). Inventory of Glacial Lakes and its Evolution in Uttarakhand Himalaya Using Time Series Satellite Data. J. Indian Soc. Remote Sens. 44, 959–976. doi:10.1007/s12524-016-0560-y
Salerno, F., Thakuri, S., D’Agata, C., Smiraglia, C., Manfredi, E. C., Viviano, G., et al. (2012). Glacial Lake Distribution in the Mount Everest Region: Uncertainty of Measurement and Conditions of Formation. Glob. Planet. Change. 92-93, 30–39. doi:10.1016/j.gloplacha.2012.04.001
Shugar, D. H., Burr, A., Haritashya, U. K., Kargel, J. S., Watson, C. S., Kennedy, M. C., et al. (2020). Rapid WorldWide Growth of Glacial Lakes Since 1990. Nat. Clim. Chang. 10, 939–945. doi:10.1038/s41558-020-0855-4
Sommer, C., Malz, P., Seehaus, T. C., Lippl, S., Zemp, M., and Braun, M. H. (2020). Rapid Glacier Retreat and Downwasting Throughout the European Alps in the Early 21st Century. Nat. Commun. 11, 3209. doi:10.1038/s41467-020-16818-0
Song, C., and Sheng, Y. (2016). Contrasting Evolution Patterns Between Glacier-Fed and Non-Glacier-fed Lakes in the Tanggula Mountains and Climate Cause Analysis. Climatic Change. 135, 493–507. doi:10.1007/s10584-015-1578-9
Song, C., Sheng, Y., Ke, L., Nie, Y., and Wang, J. (2016). Glacial lake Evolution in the Southeastern Tibetan Plateau and the Cause of Rapid Expansion of Proglacial Lakes Linked to Glacial-Hydrogeomorphic Processes. J. Hydrol. 540, 504–514. doi:10.1016/j.jhydrol.2016.06.054
Song, C., Sheng, Y., Wang, J., Ke, L., Madson, A., and Nie, Y. (2017). Heterogeneous Glacial lake Changes and Links of lake Expansions to the Rapid Thinning of Adjacent Glacier Termini in the Himalayas. Geomorphology. 280, 30–38. doi:10.1016/j.geomorph.2016.12.002
Vanham, D. (2012). The Alps Under Climate Change: Implications for Water Management in Europe. J. Water Clim. Change. 3, 197–206. doi:10.2166/wcc.2012.032
Veettil, B. K., Bianchini, N., de Andrade, A. M., Bremer, U. F., Simões, J. C., and de Souza Junior, E. (2016). Glacier Changes and Related Glacial lake Expansion in the Bhutan Himalaya, 1990-2010. Reg. Environ. Change. 16, 1267–1278. doi:10.1007/s10113-015-0853-7
Veh, G., Korup, O., Roessner, S., and Walz, A. (2018). Detecting Himalayan Glacial Lake Outburst Floods From Landsat Time Series. Remote Sensing Environ. 207, 84–97. doi:10.1016/j.rse.2017.12.025
Veh, G., Korup, O., and Walz, A. (2020). Hazard From Himalayan Glacier Lake Outburst Floods. Proc. Natl. Acad. Sci. USA. 117, 907–912. doi:10.1073/pnas.1914898117
Wang, X., Chai, K., Liu, S., Wei, J., Jiang, Z., and Liu, Q. (2017). Changes of Glaciers and Glacial Lakes Implying Corridor-Barrier Effects and Climate Change in the Hengduan Shan, southeastern Tibetan Plateau. J. Glaciol. 63, 535–542. doi:10.1017/jog.2017.14
Wang, X., Ding, Y., Liu, S., Jiang, L., Wu, K., Jiang, Z., et al. (2013a). Changes of Glacial Lakes and Implications in Tian Shan, Central Asia, Based on Remote Sensing Data From 1990 to 2010. Environ. Res. Lett. 8, 044052. doi:10.1088/1748-9326/8/4/044052
Wang, X., Siegert, F., Siegert, A.-g., and Franke, J. (2013b). Glacier and Glacial Lake Changes and Their Relationship in the Context of Climate Change, Central Tibetan Plateau 1972-2010. Glob. Planet. Change. 111, 246–257. doi:10.1016/j.gloplacha.2013.09.011
Wang, X., Guo, X., Yang, C., Liu, Q., Wei, J., Zhang, Y., et al. (2020). Glacial Lake Inventory of High-Mountain Asia in 1990 and 2018 Derived From Landsat Images. Earth Syst. Sci. Data. 12, 2169–2182. doi:10.5194/essd-12-2169-2020
Wilson, R., Glasser, N. F., Reynolds, J. M., Harrison, S., Anacona, P. I., Schaefer, M., et al. (2018). Glacial Lakes of the Central and Patagonian Andes. Glob. Planet. Change. 162, 275–291. doi:10.1016/j.gloplacha.2018.01.004
Wood, J. L., Harrison, S., Wilson, R., Emmer, A., Yarleque, C., Glasser, N. F., et al. (2021). Contemporary Glacial Lakes in the Peruvian Andes. Glob. Planet. Change. 204, 103574. doi:10.1016/j.gloplacha.2021.103574
Worni, R., Huggel, C., and Stoffel, M. (2013). Glacial Lakes in the Indian Himalayas - From an Area-Wide Glacial Lake Inventory to On-Site and Modeling Based Risk Assessment of Critical Glacial Lakes. Sci. Total Environ. 468-469, S71–S84. doi:10.1016/j.scitotenv.2012.11.043
Yao, X., Liu, S., Han, L., Sun, M., and Zhao, L. (2018). Definition and Classification System of Glacial Lake for Inventory and Hazards Study. J. Geogr. Sci. 28, 193–205. doi:10.1007/s11442-018-1467-z
Zekollari, H., Huss, M., and Farinotti, D. (2018). Modelling the Future Evolution of Glaciers in the European Alps Under the EURO-CORDEX RCM Ensemble. Cryosphere Discuss. 13, 1125–1146. doi:10.5194/tc-13-1125-2019
Zekollari, H., Huss, M., and Farinotti, D. (2020). On the Imbalance and Response Time of Glaciers in the European Alps. Geophys. Res. Lett. 47, e2019GL085578. doi:10.1029/2019GL085578
Zemp, M., Frey, H., Gärtner-Roer, I., Nussbaumer, S. U., Hoelzle, M., Paul, F., et al. (2015). Historically Unprecedented Global Glacier Decline in the Early 21st century. J. Glaciol. 61, 745–762. doi:10.3189/2015JoG15J017
Zemp, M., Haeberli, W., Hoelzle, M., and Paul, F. (2006). Alpine Glaciers to Disappear Within Decades? Geophys. Res. Lett. 33, L13504. doi:10.1029/2006gl026319
Zemp, M., Huss, M., Huss, E., Eckert, N., McNabb, R., Huber, J., et al. (2019). Global Glacier Mass Changes and Their Contributions to Sea-Level Rise From 1961 to 2016. Nature. 568, 382–386. doi:10.1038/s41586-019-1071-0
Keywords: glacial lake, ALPS, glacier, climate change, remote sensing, landsat
Citation: Ma J, Song C and Wang Y (2021) Spatially and Temporally Resolved Monitoring of Glacial Lake Changes in Alps During the Recent Two Decades. Front. Earth Sci. 9:723386. doi: 10.3389/feart.2021.723386
Received: 10 June 2021; Accepted: 16 August 2021;
Published: 30 August 2021.
Edited by:
Hongjie Xie, University of Texas at San Antonio, United StatesReviewed by:
Riddhi Singh, Indian Institute of Technology Bombay, IndiaWeili Duan, Xinjiang Institute of Ecology and Geography CAS, China
Copyright © 2021 Ma, Song and Wang. This is an open-access article distributed under the terms of the Creative Commons Attribution License (CC BY). The use, distribution or reproduction in other forums is permitted, provided the original author(s) and the copyright owner(s) are credited and that the original publication in this journal is cited, in accordance with accepted academic practice. No use, distribution or reproduction is permitted which does not comply with these terms.
*Correspondence: Chunqiao Song, cqsong@niglas.ac.cn