- 1Institute of Volcanology and Seismology FEB RAS, Petropavlovsk-Kamchatsky, Russia
- 2Institute of Experimental Mineralogy RAS, Chernogolovka, Russia
- 3Helmholtz-Zentrum Dresden-Rossendorf, Helmholtz Institute Freiberg for Resource Technology, Freiberg, Germany
Platinum-group elements (PGE) and gold are a promising tool to assess the processes of mantle melting beneath the subduction zones. However, fractionation processes in magmas inevitably overwrite the initial metal budgets of magmas, making constraints on the melting processes inconclusive. Moreover, little is still known about the geochemical behavior of a particular metal in a single arc magmatic system, from mantle melting towards magma solidification. Here we compare noble metals in lavas from several eruptions of the Tolbachik volcano (Kamchatka arc) to better understand the effects of magma differentiation, estimate primary melt compositions and make constraints on the mantle melting. We show that Ir, Ru, Rh and, to a lesser extent, Pt are compatible during magmatic differentiation. The pronounced incompatible behavior of Cu and Pd, observed in Tolbachik magmas, rules out the significant influence of sulfide melts on the early magmatic evolution in this particular case. Gold is also incompatible during magmatic differentiation; however, its systematics can be affected by the inferred gold recycling in the plumbing system of Tolbachik. Although the Tolbachik lavas show only slightly higher PGE fractionation than in MORB, a notable negative Ru anomaly (higher Pt/Ru and Ir/Ru) is observed. We attribute this to be a result of greater oxidation in the subarc mantle (by 1–4 log units), which promotes crystallization of Ru-bearing phases such as Fe3+-rich Cr-spinel and laurite. The estimated Pd contents for the parental melt of the Tolbachik lavas approaches 6.5 ppb. This is several times higher than reported MORB values (1.5 ± 0.5 ppb), suggesting the enrichment of Pd in the mantle wedge. Our results highlight the influence of the subduction-related processes and mantle wedge refertilization on the noble metal budgets of arc magmas.
1 Introduction
Our understanding of subduction zone structures and evolution is based on a complex array of geological, geochemical, and geophysical data. Numerous studies on the behavior of large-ion lithophile (LILE) and high-field-strength (HFSE) elements (e.g., K, Rb and Nb, REE, respectively) have significantly contributed to our comprehension of the process of mantle melting in the presence of subduction-derived fluids and/or hydrous melts (Tatsumi and Eggins, 1995; Zheng, 2019). Another potentially powerful tool in assessing the processes beneath the subduction zones is the systematics of noble metals (i.e., platinum-group elements (PGE) and gold; e.g., Kepezhinskas et al., 2002; Dale et al., 2012; Siegrist et al., 2019; Siegrist et al., 2021). However, current availability of noble metals data in arc rocks has been insufficient for using them as geochemical tracers of mantle melting and associated arc magma generation.
Noble metals have dualistic behavior and can be either chalcophile or highly siderophile depending on the conditions. In sulfide-saturated systems, PGE and Au have strong affinities to sulfide liquids and minerals (e.g., Mungall and Brenan, 2014), whereas in sulfide-undersaturated conditions they tend to form native phases (e.g., Kamenetsky et al., 2015). Under certain circumstances, some PGE (Ru, Ir and Rh) can be incorporated into the Cr-spinel lattice (Locmelis et al., 2011; Park et al., 2012; Park et al., 2017). Although they are geochemically similar, PGE can fractionate from one another, allowing their use as geochemical proxies.
Noble metal budgets in primary arc magmas are initially controlled by the bulk metal contents of the mantle wedge and subduction-related components, as well as the element host phase (e.g., sulfide or native metals), oxygen fugacity and the degree of partial melting (Fleet and Stone, 1991; Fleet et al., 1991; Kepezhinskas et al., 2002; Dale et al., 2012; Siegrist et al., 2019; Siegrist et al., 2021). Subsequent evolution of PGE and Au contents in ascending magmas is largely dependent on magma differentiation processes and parameters, such as the solubilities of metals in silicate melts (Borisov and Palme, 2000; Borisov, 2005), silicate-sulfide partitioning (e.g., Mungall and Brenan, 2014), and partitioning of noble metals into various crystallizing phases (i.e., metal nuggets, sulfides or Cr-spinel) and immiscible sulfide liquids (Dale et al., 2012; Park et al., 2012; Park et al., 2013; Zelenski et al., 2017; Hao et al., 2021). It is crucial to eliminate the effects of magma fractionation in order to use PGE and Au for assessing mantle melting processes. Subsequently, a multiplicity of factors should be considered to assess mantle wedge melting; the most important being the possible influence of the subduction influx on melting processes. This may have a direct impact on the PGE and Au budget of a primitive melt (e.g., Dale et al., 2009; Dale et al., 2012), thus affecting the geochemistry of all subsequent evolved members of a magmatic series.
In summary, our study aims to rule out the effects of magma fractionation in order to assess noble metal budgets of a primitive magma and make further constraints of mantle wedge properties and mantle melting processes. Tolbachik volcano, Kamchatka, comprises the perfect example for such a study. It has been extensively studied with regards to PGE and Au contents and mineralogy (Zelenski et al., 2016; Zelenski et al., 2017), making it one of the most well-studied in terms of noble metals geochemistry arc volcanic systems in the world. Noble metals from Tolbachik have been documented both in sulfide globules, and as native Au-Ag alloys, the latter being a result of magma differentiation and assimilation of crustal components (Zelenski et al., 2016). Furthermore, the Tolbachik volcano has also been widely studied with respect to its mantle source melting (subduction influx, degree of partial melting, etc.) and differentiation processes (Flerov et al., 1984; Portnyagin et al., 2007; Churikova et al., 2015a; Churikova et al., 2015b; Portnyagin et al., 2015; Mironov and Portnyagin, 2018), providing a framework for the interpretation of new data. Therefore, it provides a great opportunity to investigate the fate of PGE and Au within a single, well-defined arc magmatic system from the mantle wedge to its volcanic vent.
2 Geological Background
The Kurile-Kamchatka volcanic arc is one of the most active volcanic areas on Earth (Figure 1A), stretching for almost 2,000 km northeast of the Japanese Islands. The Tolbachik volcanic massif belongs to the Klyuchevskoy volcanic group (Figures 1B,C), located at the northern end of the Kurile-Kamchatka arc. It comprises the Ostry Tolbachik volcano (extinct), Plosky Tolbachik volcano (active), and a series of monogenic cones associated with two fissure zones extending ∼50 km southwards and ∼14 km northwards from Plosky Tolbachik (Figure 1C). The volcanic field related to the monogenic cones covers an area of 875 km2 (Churikova et al., 2015b). Rocks of Tolbachik are represented by low-Mg basalts, and to a lesser extent by high-Mg basalts (Churikova et al., 2015b). The most evolved varieties belong to basaltic trachyandesites (Volynets et al., 2015). The volcano is characterized by high productivity; the volume of eruptive products in dense rock equivalent estimated at 2 km3 in three eruptions over the past 80 years (e.g., Zelenski et al., 2018).
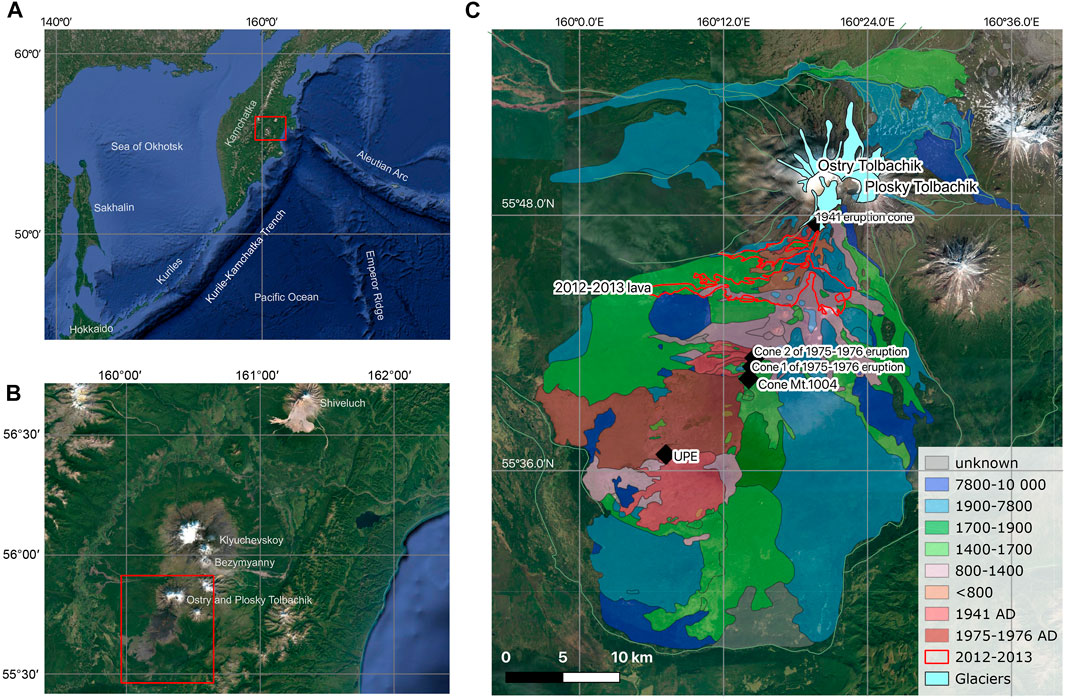
FIGURE 1. Maps of Kamchatka and adjacent areas (A), Klyuchevkoy group (B) and the Tolbachik monogenic lava field showing individual vents and lava flows (C). The map was compiled by Braitseva et al. (1984) based on tephrochronological dating of lava flows and regional marker tephra layers, and improved by Churikova et al. (2015a). Satellite images are from google.com/maps.
3 Samples and Analytical Techniques
Samples for this study were collected from the 1941 eruptive cone (n = 2), cones of the Great Tolbachik Fissure Eruption of 1975-76 (GTFE, n = 11 + 1 duplicate), the lava field of the 2012–13 eruption (n = 1), and two pre-historic sites: Cone Mt. 1004 (n = 12 + 3 duplicates) and the Unnamed Prehistoric Eruption (UPE, n = 2). Samples were ground to powder at the Geoscience Laboratories at the Ontario Geological Survey (Canada) with the usage of agate mills in order to avoid contamination, which is possible if Fe-, Ni- and Cr-alloy-based mills are used. Major and trace element contents were analyzed by XRF and ICP-MS, respectively, in the Geoscience Laboratories. Gold and PGE contents were analyzed using NiS fire assay preconcentration followed by ICP–MS (Geoscience Laboratories, Sudbury, ON, Canada). Analysis of blanks provided values not exceeding 0.02 ppb for Pd and smaller values for other PGE and Au, thus being several orders of magnitude lower than the respective concentrations of the studied samples. Several international standards were used, including UMT-1, OREAS 681, OREAS 682 and TDB-1. The latter is of special importance, as its PGE and Au contents are of the same order of magnitude as the studied samples. Table 1 compares the results of TDB-1 analysis at the Geoscience Laboratories, Sudbury with the certified values and analysis of the same standard by isotope dilution previously published. Further details are provided in the Supplementary Package 1 “Blanks and standards”.
4 Results
4.1 Major and Trace Elements
The most Mg-rich samples are from the Cone Mt. 1004 olivine-phyric lavas (Figures 2A,B), which are characterized by MgO contents lying within 9.2–9.5 wt.% (Figure 3). Two samples from the 1941 eruption (lava and scoria) are almost identical in MgO content (8.7 wt.%), as well as in all other chemical components. One sample from the GTFE and one from the Unnamed Prehistoric Eruption (UPE) also are high-Mg. Low-Mg lavas (Figures 2C,D) and scoria were collected from the GTFE and 2012–2013 eruption. Their MgO contents range from 4.0 to 5.1 wt.%, however they have significantly higher Al2O3, Na2O, K2O, and TiO2 contents than the high-Mg basalts (Figure 3). One sample from each of the 1975 eruption scoria and UPE have K2O contents significantly lower than those of equally primitive samples (Figures 3E,F,H). Iron contents remain almost constant across the whole compositional range, similar to the primitive melt composition calculated by Portnyagin et al. (2015). Mg# (MgO/(MgO + FeO), mol%) ranges from 0.58 to 0.63 for high-Mg basalts and from 0.42 to 0.55 for low-Mg lavas (Table 2).
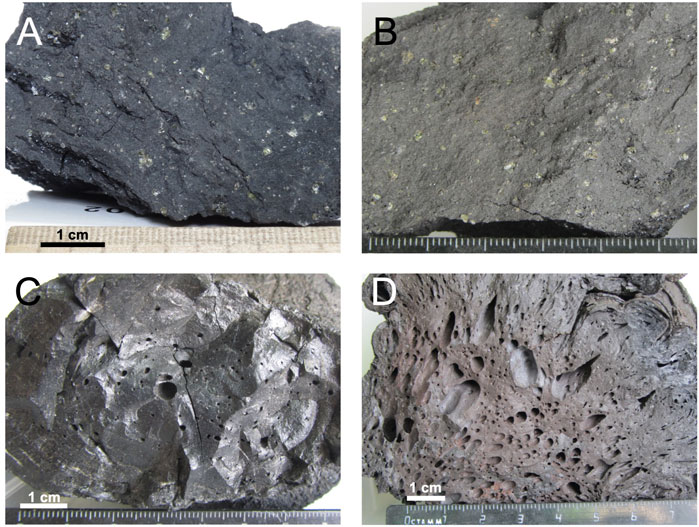
FIGURE 2. Olivine-phyric high-Mg basalts of Cone Mt. 1004 (A, B) and plagioclase-phyric low-Mg basalts of the 2012–2013 eruption (C, D).
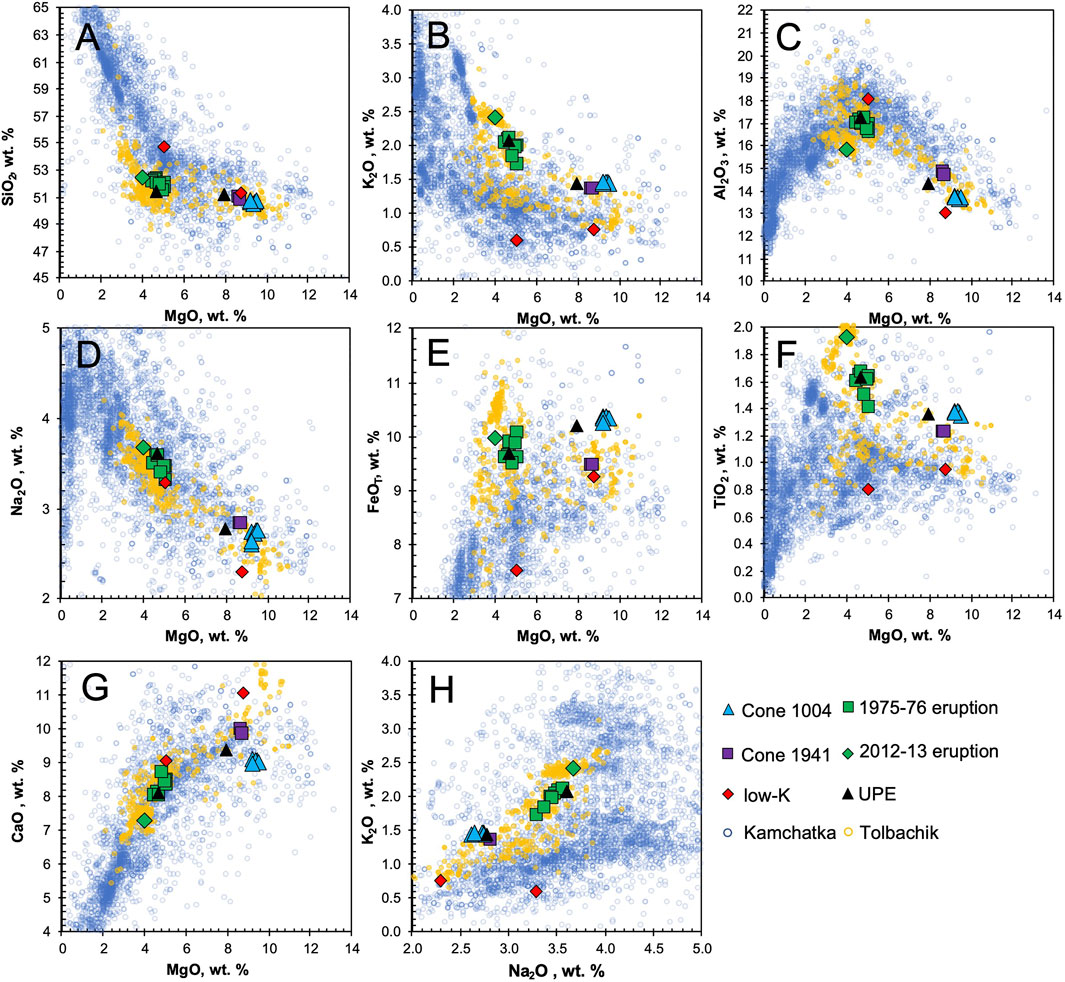
FIGURE 3. Major element contents in rocks from the Kurile-Kamchatka arc, previously published Tolbachik analyses and new results. Data from GeoRoc (http://georoc.mpch-mainz.gwdg.de/).
4.2 Noble Metals
The concentrations of platinum-group elements (PGE) in Tolbachik lavas are summarized in Table 2. Iridium is enriched in high-Mg basalts (Figure 4A). Similar behavior is observed for Ru (Figure 4B); however, the difference between high-Mg and low-Mg rocks is much less pronounced. Rhodium also defies this trend showing significantly higher concentrations in high-Mg rocks (Figure 4C).
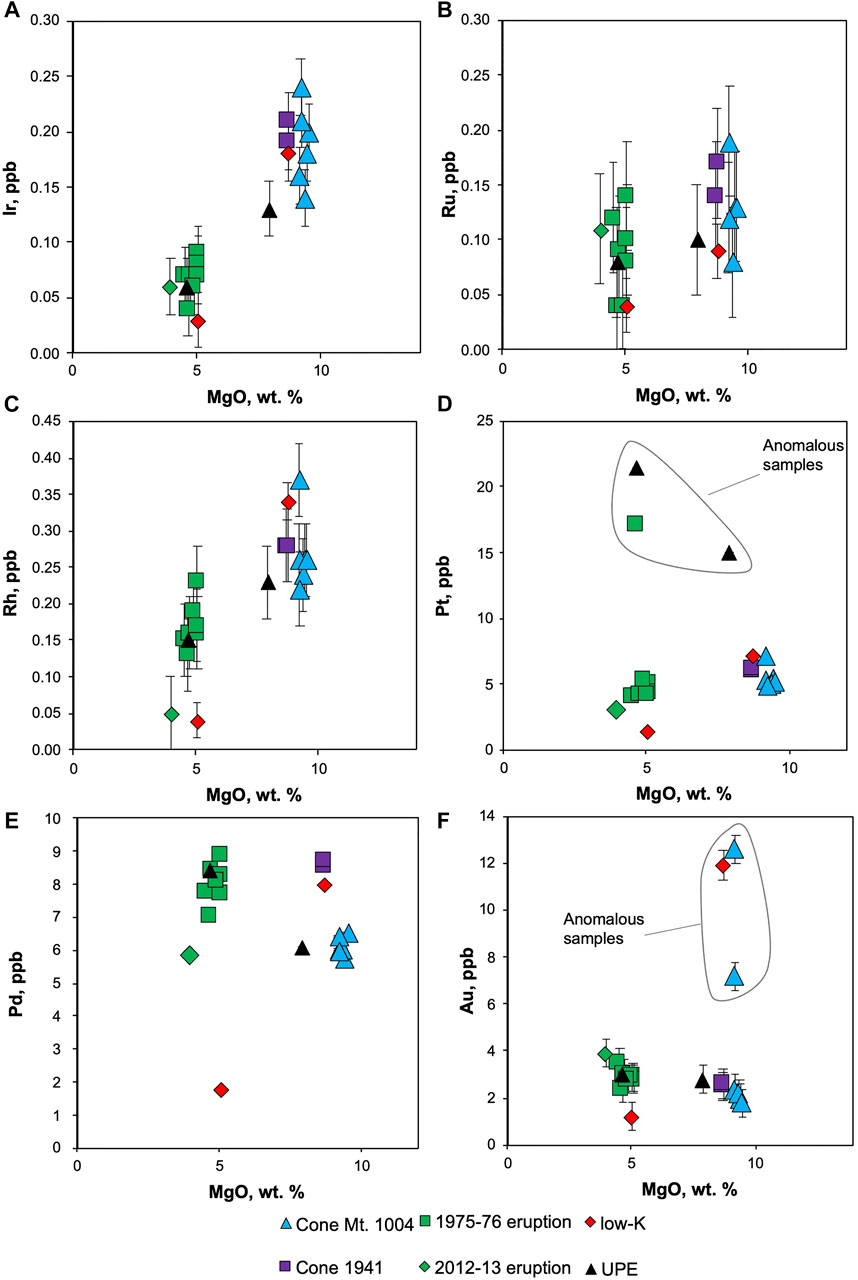
FIGURE 4. Platinum-group element variation across different MgO contents for Tolbachik lavas. Error bars are provided for Ir, Ru, Rh and Au; for Pt and Pd they are smaller than the size of a symbol.
Platinum is only slightly enriched in high-Mg basalts (with the exception of three anomalous samples; Figure 4D). These anomalous samples are high- and low-Mg basalt from the UPE (21.5 and 15 ppb Pt, respectively), and low-Mg basalt from the GTFE 1975-76 (17.1 and 21.5 ppb Pt for sample and duplicate).
Palladium is enriched in low-Mg rocks (Figure 4E). High-Mg samples from the 1941 eruption and one of the samples from GTFE have high Pd contents of 8.0–8.5 ppb, whereas other high-Mg basalts have moderate Pd contents (5.7–6.5 ppb). In general, low-Mg basalts are enriched in Pd relative to high-Mg basalts. Gold distribution closely mimics that of Pd (Figure 4F), with significantly higher concentrations in low-Mg (2.8–3.9 ppb) relative to high-Mg samples (1.8–2.6 ppb). Several samples, two from Cone Mt. 1004 and one of the 1975-76 eruption, are anomalously Au-rich and have not been considered in the calculations.
5 Discussion
5.1 Are the Platinum-Group Elements Data Representative? The Effects of Platinum-Group Elements-Bearing “Nuggets”
To determine whether PGE and Au contents are representative of the sampled lava flow, 13 samples and 3 duplicates from Cone Mt. 1004 were analyzed. The results are uniform and have relatively low standard deviations for all elements except Au (Table 3), indicating homogenous distribution of PGE-bearing phases.
Nevertheless, six samples in the full dataset (n = 32) showed anomalous enrichment in one noble metal. Gold-rich samples are exclusively from high-Mg basalts, either the 1941 eruption or GTFE, whereas Pt-rich samples are either from the UPE or GTFE. This can be attributed to the “nugget effect” (heterogeneous distribution of anomalously large grains of a particular metal) or occur as a result of a specific process that may have enriched the melt. The duplicate of the UPE Pt-rich sample also yielded anomalous Pt contents. Given the established homogenous Pt distribution in samples from Cone Mt. 1004, this suggests that, for some samples, high metal contents may be an intrinsic property of the magma and cannot be ascribed to the presence of nuggets (Figure 4D).
A plausible explanation for Pt enrichment is the presence of sulfide blebs which have been reported for high-Mg basalts of the 1941 eruption and Cone Mt. 1004 (Zelenski et al., 2017). However, these blebs show a perfect Pt-Pd correlation, whereas the studied samples are enriched exclusively in Pt (Figure 5). Alternatively, such enrichment could be attributed to the ability of Pt to form inclusions within Cr-spinel (Finnigan et al., 2008; Kamenetsky et al., 2015) or as separate nuggets (Park et al., 2013). Selective enrichment of Pt over Pd may be explained in terms of their relative solubilities in silicate melts, e.g. at a given oxygen fugacity, Pd solubility is more than two orders of magnitude higher than Pt (Borisov and Palme, 2000). Therefore, Pd is retained in the melt, whereas Pt precipitates as Pd-free alloys.
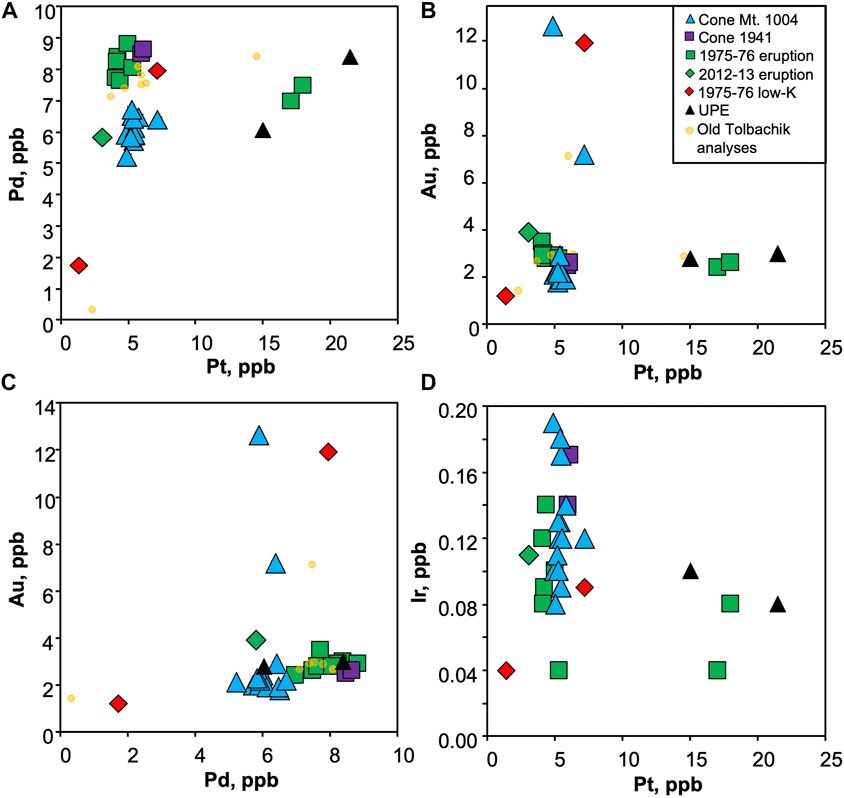
FIGURE 5. Interelement PGE and Au variation for the Tolbachik volcano. Note Au and Pt anomalies, uncorrelated with concentrations of any other element analyzed.
Despite working well for Pt, this phenomenon cannot explain the presence of gold nuggets. Gold solubility in basaltic melts is far too high, restricting its direct precipitation at a wide range of temperatures and fO2. For example, the Au solubility estimated by Borisov and Palme (2000) ranges from 40 ppb for QFM-2 at 1,200°C to 5,000 ppb for QFM+2 at 1,350°C, or even higher if sulfide complexation is considered (Botcharnikov et al., 2010). The estimated fO2 for Tolbachik lavas is QFM+1 to +2, based on chromian spinel compositions (Kamenetsky et al., 2017; Nekrylov et al., 2018) and olivine-sulfide pairs (Zelenski et al., 2018) At this fO2, Au solubility might have approached 400 ppb. However, this is two orders of magnitude higher than the most enriched samples (13.3 ppb), making direct gold precipitation from Tolbachik magmas unrealistic. Therefore, the idea of Au enrichment as a result of crustal assimilation, discussed in Zelenski et al. (2016), appears to be the most plausible explanation.
5.2 Behavior of Platinum-Group Elements During Arc Magma Evolution
Iridium, Ru and Rh show compatible behavior, reflected in their lower contents within more evolved Al-rich basalts. Similar trends have been observed in various volcanic arcs (Figures 6A,B), and can be explained by the capturing of these elements either as admixtures in Cr-spinel (Park et al., 2012; Park et al., 2017), or as nuggets (Kamenetsky et al., 2015). Their accumulation in Cr-spinel is attributed to a redox related response to mineral growth or re-equilibration with the melt surrounding Cr-spinel crystals (Finnigan et al., 2008). Local reduction within the mineral–melt interfacial region occurs as a consequence of the selective uptake of trivalent over divalent Cr and Fe from the melt by spinel (Finnigan et al., 2008).
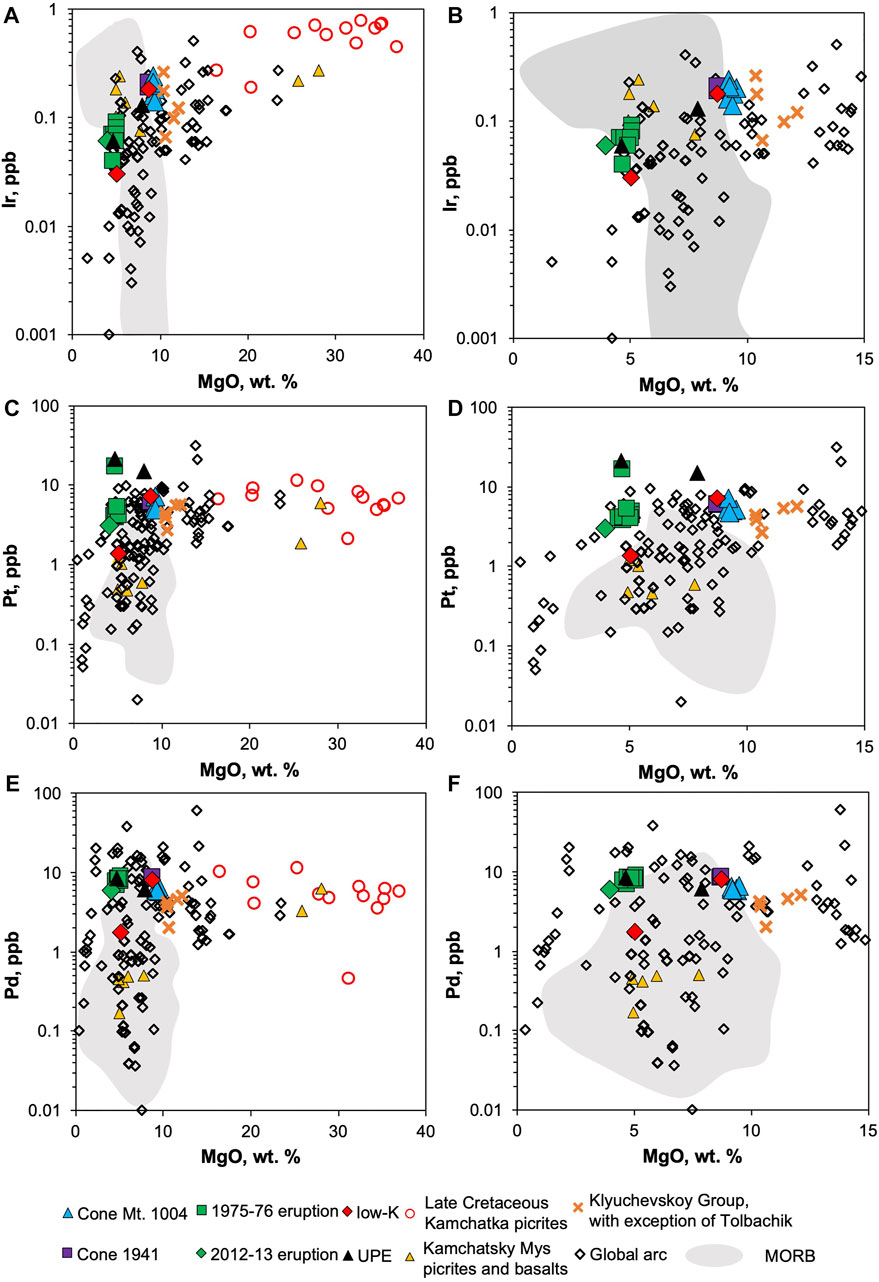
FIGURE 6. Platinum-group element variation across different MgO contents for arc lavas and MORB. Data for the Klyuchevskoy Group—(Nekrylov et al., 2018, in press), late Cretaceous Kamchatka picrites—(Kutyrev et al., 2021), Kamchatsky Mys (Savelyev et al., 2018a; Savelyev et al., 2018b), global arc—(Ely and Neal, 2003; Setiabudi et al., 2007; Dale et al., 2008; Dale et al., 2012; Park et al., 2012), MORB—(Rehkämper et al., 1999; Peucker-Ehrenbrink et al., 2003; Jenner and O’Neill, 2012; Kelley et al., 2013; Yang et al., 2014; Reekie et al., 2019; Hao et al., 2021).
Both Pt and Pd show no correlation with MgO from primitive compositions, studied here and from other magmatic arcs, down to ∼9 wt.% MgO (Figures 6C–F). Their behavior, however, becomes variable for evolved compositions from global arcs, where Pt and Pd contents often drop drastically, whereas, in Tolbachik, their concentrations remain stable as MgO decreases progressively, indicating a lack of sulfide saturation for the latter, at least up to 4 wt.% MgO. In contrast, Pd contents of MORB lavas with less than ∼9.5 wt.% MgO vary drastically (Dale et al., 2012; Hao et al., 2021). This suggests that MORB and some arc melts achieved sulfide saturation at ∼9–9.5 wt.% MgO, producing Pd and Pt depletion, and that other arc melts either did not reach sulfide saturation, or that sulfide saturation did not affect the metal budget.
However, the Tolbachik samples reveal that low-Mg lavas bear slightly higher amounts of Pd than high-Mg lavas (Figure 4E). This indicates incompatible Pd behavior and may be evidence against sulfide saturation during the Tolbachik magmas evolution. Although previous studies reported sulfide-silicate immiscibility in Tolbachik lavas (Zelenski et al., 2018), this process was limited to a small amount of melt and did not significantly affect the overall PGE budget. Moreover, sulfide precipitation itself is not sufficient to affect the PGE budget; rather, the extraction/removal of newly formed sulfide (liquid or crystalline) from a melt is more crucial.
Slight Pt enrichment in high-Mg lavas suggests it behaves compatibly during magma evolution from 10 down to 4 wt.% MgO (Figures 4D, 6C,D). This does not support sulfide saturation as the cause for Pt fractionation, because Pd contents would also be expected to decrease. Nevertheless, the lack of significant Pt–MgO correlations is evident across a range of compositions (9–37 wt.% MgO), i.e., rocks which are likely cumulates of olivine and chromian spinel. This implies that in spite of evidence for Pt-Fe alloy coprecipitation with chromian spinel (Finnigan et al., 2008; Kamenetsky et al., 2015; Arguin et al., 2016), their fractionation does not contribute significantly to the Pt budget of arc magmas in general.
5.3 Platinum Group Element Contents in Parental Melts
The understanding of the PGE budget in parental melts is hindered by fractionation processes. The plurality of factors involved in the formation of particular lava makes precise evaluation of the primitive melt composition almost impossible. Nevertheless, a rough estimation is possible for at least some elements. Due to the lack of significant sulfide saturation, Pd contents show the following: 1) moderate enrichment from ∼9 to ∼5 wt.% MgO in Tolbachik lavas, and 2) no correlation across the range between 37 and 9 wt.% MgO (Figures 6E,F). This long flat trend suggests that during evolution from the assumed parental melt (∼14 wt.% MgO Portnyagin et al., 2015) to the high-Mg basalt, Pd contents did not change significantly, and thus the mean concentration of 6.5 ppb is close to that of primitive mantle-derived melts. For Pt, such an estimate is more complex as it behaves slightly incompatibly. However, other arc rocks show minimal effects Pt contents decrease towards Mg-poor compositions (Figure 4D). Therefore, mean Pt contents in high-Mg basalts (5.8 ppb, ignoring three anomalous samples) may be used as a lower estimate of initial Pt abundances in the Tolbachik primitive melt.
Iridium, unlike Pt and Pd, shows a broad correlation with MgO due to strong compatibility with chromian spinel (Figure 4A). The parental melt of the studied lavas should thus be significantly enriched in Ir. Statistical analysis of previously published data of arc rocks provides an adequate MgO–Ir linear correlation with R2 = 0.82. This allows for the extrapolation of mean Ir contents in low- and high-Mg lavas to 14 wt.% MgO, which results in 0.29 ppb Ir. Normalization of estimated Ir, Pt and Pd contents in the parental melt to primitive mantle values (Lyubetskaya and Korenaga, 2007) yields 0.1, 1.3 and 1.8 ppb, respectively, and a PdN/IrN (“N” stands for primitive mantle normalization) ratio of 20. This indicates that the fractionated pattern of the Tolbachik lavas is not only the result of fractional crystallization, but also inherited from the mantle-derived melt.
5.4 Comparison Between Arc and MORB Primitive Magmas
Mechanisms of metal transfer from mantle to melt are variable in subduction and oceanic rift environments (e.g., Dale et al., 2012; Mungall and Brenan, 2014; Park et al., 2017). The main PGE hosts in the mantle are sulfides (Keays et al., 1981; Kepezhinskas et al., 2002; Barnes et al., 2015) and alloys (e.g., Rehkämper et al., 1999). Oxidizing conditions in the sub-arc mantle during magma generation should result in complete sulfide consumption. Therefore, Pd, and to a lesser extent Pt, partition into the melt, whereas Ir, Os and Ru are retained in the source residue (Dale et al., 2012). Thus, a comparison between PGE contents in primitive arc and MORB melts may provide insights into the role of the subduction flux on the PGE budget.
Palladium can fractionate from Ir during common magma differentiation processes, including the formation of immiscible sulfide melts and the partitioning of Ir in Cr-spinel (Barnes et al., 1985; Park et al., 2012; Barnes et al., 2015). It has been shown, however, that the fractionated patterns of arc rocks are partly inherited from their mantle source or produced during partial melting (Park et al., 2017). This is also supported by the estimated PdN/IrN value for the Tolbachik primitive magmas (20). The difference in PdN/IrN between MORB and arc rocks across the range of 8.5–12.0 wt.% MgO is not significant, yielding 35.8 and 53.4, respectively (Figures 7, 8A). This similarity is also observed for PtN/PdN (Figure 8B). The principal difference between arc and MORB is related to Ru. For example, ratios of PtN/RuN (Figure 8A) or IrN/RuN (Figure 8B) are much greater in arc rocks.
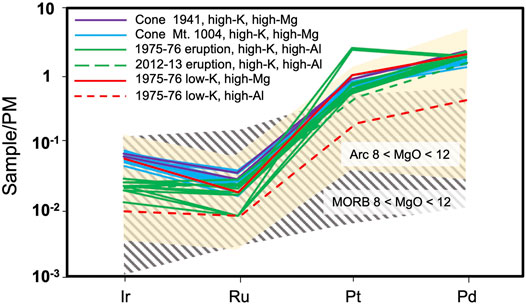
FIGURE 7. Primitive mantle-normalized PGE patterns of the Tolbachik lavas compared to arc and MORB rocks. Only samples with MgO contents between 8 and 12 wt.% are displayed. Same references as Figure 8. Values normalized following Lyubetskaya and Korenaga (2007).
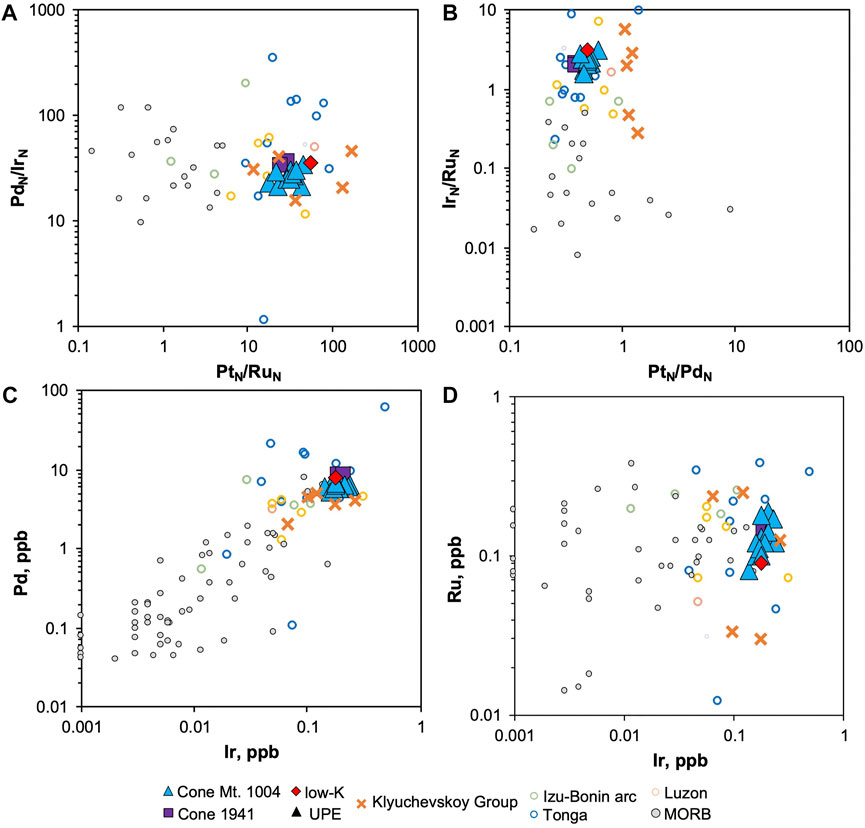
FIGURE 8. Element ratios and contents of the Tolbachik lavas compared to arc and MORB. Only samples with MgO content of 8–12 wt.% are displayed. Data for the Klyuchevskoy Group—(Nekrylov et al., 2018, in press), Izu-Bonin arc—(Dale et al., 2008), Luzon arc—(Setiabudi et al., 2007), Tonga arc—(Dale et al., 2012), MORB—(Rehkämper et al., 1999; Peucker-Ehrenbrink et al., 2003; Jenner and O’Neill, 2012; Kelley et al., 2013; Yang et al., 2014; Reekie et al., 2019; Hao et al., 2021). Values normalized following Lyubetskaya and Korenaga (2007).
The differences in individual element contents are more prominent than their ratios (Figures 8C,D). For instance, the average Pd content in MORB samples containing MgO from 8.5 to 14 wt.% is 0.4 ppb, which is ∼15 times less than the corresponding arc values (6.4 ppb). Such a drastic difference evokes the idea that fractionation processes may in fact produce low Pd concentrations in MORB. However, Hao et al. (2021) calculated primitive MORB Pd content to be 1.5 ± 0.5 ppb, which is significantly lower than our estimates of Pd content in the primitive Tolbachik melt (∼6.5 ppb). This implies that unfractionated primitive arc melts are enriched in Pd relative to MORB.
The differences between the primitive or near-primitive arc and MORB melts are summarized as the following:
1. Palladium contents are significantly higher in primitive arc magmas than those reported for parental MORB melts.
2. Iridium, Rh and Pt contents have not been evaluated for the parental MORB melt; however, comparing high-Mg arc and MORB analyses reveals that the former are systematically enriched in these elements.
3. Ruthenium contents of near-primitive arc and MORB melts are similar.
5.5 Mantle Source Controls on the Behavior of Platinum-Group Elements in Primitive Arc Melts
Factors influencing PGE contents in primitive arc and MORB melts are summarized in Figure 9. Each process, from partial melting of a MORB-type mantle (depletion) to subsequent alteration in subduction zones (accompanied by potential refertilization), and melt-mantle reactions, precede fractionation processes en-route to, and following emplacement at, the Earth’s surface or crust.
5.5.1 Mantle Depletion
It is supposed that prior to melting in supra-subduction environments, peridotites of the mantle wedge may have experienced repeated melting beneath spreading ridges. The degree of depletion may vary depending on the number of melting cycles. For example, the mantle wedge beneath some parts of the modern Kamchatka arc is considered to be moderately depleted relative to depleted MORB mantle (DMM) (e.g., Portnyagin et al., 2007). Accounting for the preceding DMM depletion is especially important in the case of an intra-oceanic arc with active back-arc basins (e.g., Pearce and Parkinson, 1993). As near-primitive MORB rocks show fractioned PGE patterns (Figure 7), it is reasonable to expect that the mantle source must be depleted in Pd and Pt relative to other PGEs. Moreover, due to extremely high sulfide-silicate partition coefficients for PGE, the presence of even minute quantities of residual sulfide in the source region produces melts strongly depleted in PGE (“all or nothing” behavior, Barnes et al., 2015; Waterton et al., 2021). Indeed, most studied abyssal peridotites show depletion in Pt and Pd, whereas there is only a limited record of fertile oceanic mantle rocks (Prichard H. M. et al., 1996). However, this contradicts the Pt and Pd enrichment observed for near-primitive arc rocks, implying that there must be a process that suppresses the effects of mantle depletion.
5.5.2 Refertilization Prior to Subduction
There is evidence for abyssal peridotite refertilization caused by incremental melts en-route to the surface. Harzburgites enriched in sulfides were reported in the Kane Fracture Zone in the Middle-Atlantic Ridge (Luguet et al., 2003). These rocks display elevated (Pd/Ir)N ratios, which positively correlate with sulfide modal abundances. In addition, Pt contents were not significantly affected by refertilization.
5.5.3 Subduction Influx: Refertilization
Mantle peridotites may be refertilized in the supra-subduction environment by slab-derived melts and fluids. For instance, Prichard et al. (1996) concluded that the supra-subduction Shetland peridotites, which are enriched in Pt and Pd, were refertilized by boninitic melts, based on comparisons with Pt- and Pd-poor Oman and Troodos peridotites. Mantle wedge xenoliths in subduction zones can be variably enriched in Pt and Pd (McInnes et al., 1999; Kepezhinskas et al., 2002). However, Pt and Pd may be depleted in mafic oceanic crust that has been metamorphosed under conditions similar to those of subduction zones, inferring that these elements may be transferred to the mantle wedge via slab-derived fluids (Dale et al., 2009).
5.5.4 Subduction Influx: Increase of fO2
It is problematic to explain the elevated PGE budget in arc magmas simply as a consequence of more oxidized conditions during mantle wedge melting, as the arc magma source is 1–4 log units more oxidized than MORB (e.g., Evans et al., 2012), which leads to sulfide destruction. However, prior to sulfide exhaustion, a hypothetical melt would be PGE-depleted due to extreme partitioning of PGE into residual sulfide. After sulfide breakdown, Pd is removed by the melt (as a result of extremely high Pd solubility in silicate melts, Borisov and Palme, 2000; Borisov, 2005), whereas all other PGE may remain in the residue as alloy phases. This may adequately explain Pd abundances in MORB (Mungall and Brenan, 2014), but does not account for the difference between Pd contents in arc and MORB melts, nor for the fractionation of Pt from IPGE and Rh. Several factors have been suggested to resolve the latter problem.
Base-metal sulfides (BMS) in the mantle are composed of monosulfide solid solution (MSS) and/or intermediate solid solution (ISS) (Luguet et al., 2003; Bockrath et al., 2004a; Luguet et al., 2004). BMS melt incongruently producing Cu-Ni-rich sulfide liquid, whereas residual MSS becomes more Fe-rich. Partitioning of PGE between MSS and sulfide liquid varies considerably; from compatible to highly incompatible, in the following sequence Ir ≈ Ru >> Pt > Pd (Li et al., 1996; Mungall et al., 2005). Hence, Pt and Pd fractionate from other PGE via the exhaustion of sulfide liquid in the mantle and leaving residual MSS (Dale et al., 2012). Though this mechanism can explain fractionation in general, it still cannot account for the elevated Pd and Pt concentrations in primitive arc melts relative to primitive MORB (Figures 6, 7C), as well as for their approximately similar Pd/Ir ratios (Figure 8A).
Some PGE may be retained in phases other than sulfides and alloys, such as Ru which has been measured up to 500 ppb in Cr-spinel (Locmelis et al., 2011). Moreover, Ir, Ru and Rh content in Cr-spinel can reach 101, 185 and 95 ppb, respectively, showing a positive association only in Fe3+-rich arc spinels (Park et al., 2017). As the arc mantle is significantly more oxidized than MORB (e.g., Evans et al., 2012), its spinel is expected to be Fe3+-rich and host significant amounts of Ir, Ru and Rh. O’Driscoll and González Jiménez, 2016 argued that since Cr-spinel is an accessory mineral comprising 1-2% of mantle peridotite, its contribution to the PGE budget should be negligible. However, given the presence of ∼200 ppb Ru in arc Cr-spinel (Park et al., 2017), the mantle may contain up to 3.7 ppb Ru, which approaches primitive mantle estimates (5 ppb, Lyubetskaya and Korenaga, 2007). As the partition coefficient between Cr-spinel and melt is higher for Ru than for Ir and Rh (Park et al., 2017), this process can adequately account for the positive Ru-anomalies typical for arc magmas (Figure 7).
Another mineral that can be responsible for the Ru anomaly is laurite RuS2 (Dale et al., 2012; Nekrylov et al., 2018, in press). This sulfide can precipitate from sulfide-undersaturated basaltic melts (Bockrath et al., 2004b), which suggests that it can possibly act as a residual phase in the mantle. This is also supported by the mineral assemblage of ophiolitic ultramafics, in which laurite is one of the most abundant minerals (Lorand and Ceuleneer, 1989; Garuti et al., 1999; Tolstykh et al., 2009; Prichard et al., 2017; Zaccarini et al., 2018). However, the question of why laurite retains Ru in subduction environments, and not in spreading centers, remains. A possible explanation is that laurite can only form in sulfide-undersaturated conditions, hence high fO2 (otherwise, MSS will retain Ru, Bockrath et al., 2004b). This scenario corresponds to subduction settings, implying that mantle residue following arc melting must be enriched in laurite and have a positive Ru anomaly, thus mirroring PGE distribution in arc rocks. Indeed, such anomalies have been reported, including within xenoliths of the Kharchinsky volcano (75 km north of Tolbachik, Siegrist et al., 2021). Evidently, the formation of laurite at the expense of MSS contradicts previously suggested explanations of IPGE/PPGE fractionation.
PGE contents are also invariably affected by the stability of alloy phases during mantle melting following sulfide exhaustion. Solubilities of PGE have been established in artificial silicate melts (Borisov and Palme, 2000), however these may be significantly different in natural magmas. Nonetheless, the general rule is clear: PGE solubility increases gradually with temperature and drastically with fO2. The temperature of relatively dry mantle melting in spreading ridges is higher than those of fluid-assisted subduction conditions, which should lead to more efficient alloy dissolution in the former. However, this difference appears to be negligible compared to the effects of higher fO2 of arc settings. Consequently, this indicates that PGE-bearing alloys should more easily transfer into the melt during subduction-related melting.
In summary, fractionation of PPGE from IPGE can be satisfactorily explained by a combination of processes and factors, including IPGE-rich MSS retention, variable PGE solubility after sulfide exhaustion, and/or IPGE partitioning within the lattice of Cr-spinel in the arc mantle. Furthermore, elevated Pd contents in arc rocks should be directly inherited from the mantle source, which, in turn, must be enriched by preceding refertilization processes.
6 Conclusion
1. The incompatible behavior of both Cu and Pd in magmas within the compositional range of 4–10 wt.% MgO implies a limited influence of sulfide-silicate immiscibility on the PGE budget. As the Tolbachik magmas are oxidized (QFM + 1–2), typical for arc magmas, sulfide saturation was limited to restricted portions of melt and periods of time, and thus did not allow sulfide accumulation and extraction of noble metals from the melt. Previously reported sulfide globules, preserved in olivine phenocrysts, are the products of such limited sulfide saturation.
2. Gold enrichment, observed for several samples, is not accompanied by correspondingly elevated PGE concentrations, and thus cannot be a result of sulfide accumulation. Instead, gold anomalies are interpreted in terms of previously supposed gold recycling in the plumbing system of Tolbachik.
3. The Tolbachik lavas and arc rocks show higher degrees of fractionation than MORB; however, the difference is moderate (PdN/IrN is 53.4 and 35.8, respectively). The only prominent dissimilarity is the negative Ru anomaly in arc rocks. This is due to the subarc mantle being 1 to 4 log units more oxidized than MORB, leading to the crystallization of Fe3+-rich Cr-spinel and laurite, which are both known for hosting Ru.
4. Palladium contents in the parental melt of Tolbachik are close to 6.5 ppb, which is four times higher than reported MORB values (1.5 ± 0.5 ppb). An influx of Pd during refertilization processes of the subarc mantle may be responsible for Pd enrichment of arc melts.
Data Availability Statement
The original contributions presented in the study are included in the article/Supplementary Material, further inquiries can be directed to the corresponding author.
Author Contributions
AK, MZ, and VK conceived the idea of the study and organized fieldwork on the Tolbachik volcano with a help of DS and NN. Samples were processed by AK, MZ, and DS. All co-authors contributed to the discussion, conclusions, and a final draft for review.
Funding
This work was supported by the Russian Science Foundation Grant #21-17-00122.
Conflict of Interest
The authors declare that the research was conducted in the absence of any commercial or financial relationships that could be construed as a potential conflict of interest.
Publisher’s Note
All claims expressed in this article are solely those of the authors and do not necessarily represent those of their affiliated organizations, or those of the publisher, the editors and the reviewers. Any product that may be evaluated in this article, or claim that may be made by its manufacturer, is not guaranteed or endorsed by the publisher.
Acknowledgments
Editor Philipp A. Brandl is acknowledged for the efficient handling of the manuscript. Constructive comments by John Mavrogenes and Ilya Bindeman helped to greatly improve this manuscript and refine modelling. The reference data on various volcanic rocks compositions was assembled using the GEOROC database (Geochemistry of Rocks of the Oceans and Continents).
Supplementary Material
The Supplementary Material for this article can be found online at: https://www.frontiersin.org/articles/10.3389/feart.2021.791465/full#supplementary-material
References
Arguin, J.-P., Page, P., Barnes, S.-J., Yu, S.-Y., and Song, S.-Y. (2016). The Effect of Chromite Crystallization on the Distribution of Osmium, Iridium, Ruthenium and Rhodium in Picritic Magmas: an Example from the Emeishan Large Igneous Province, Southwestern China. J. Petrol. 57 (5), 1019–1048. doi:10.1093/petrology/egw033
Barnes, S. J., Mungall, J. E., and Maier, W. D. (2015). Platinum Group Elements in Mantle Melts and Mantle Samples. Lithos 232, 395–417. doi:10.1016/j.lithos.2015.07.007
Barnes, S. J., Naldrett, A. J., and Gorton, M. P. (1985). The Origin of the Fractionation of Platinum-Group Elements in Terrestial Magmas. Chem. Geology. 53 (53), 303–323. doi:10.1016/0009-2541(85)90076-2
Bockrath, C., Ballhaus, C., and Holzheid, A. (2004a). Fractionation of the Platinum-Group Elements during Mantle Melting. Science 305, 1951–1953. doi:10.1126/science.1100160
Bockrath, C., Ballhaus, C., and Holzheid, A. (2004b). Stabilities of Laurite RuS2 and Monosulfide Liquid Solution at Magmatic Temperature. Chem. Geology. 208 (1-4), 265–271. doi:10.1016/j.chemgeo.2004.04.016
Borisov, A. A. (2005). Crystallization and Stability of Noble Metal Alloys in the Magmatic Process. Geology. Ore Deposits 47 (6), 469–475.
Borisov, A., and Palme, H. (2000). Solubilities of noble Metals in Fe-Containing Silicate Melts as Derived from Experiments in Fe-free Systems. Am. Mineral. 85 (11-12), 1665–1673. doi:10.2138/am-2000-11-1209
Botcharnikov, R. E., Linnen, R. L., Wilke, M., Holtz, F., Jugo, P. J., and Berndt, J. (2010). High Gold Concentrations in Sulphide-Bearing Magma under Oxidizing Conditions. Nat. Geosci 4 (2), 112–115. doi:10.1038/ngeo1042
Braitseva, O. A., Melekestsev, I. V., Flerov, G. B., Ponomareva, V. V., and Sulerzhitsky, L. D. (1984). “Holocene Volcanism of the Tolbachik Regional Zone of Cinder Cones,” in Greate Tolbachik Fissure Eruption, Kamchatka, 1975-1976. Editor S. A. Fedotov (Moscow: Nauka), 177–209.
Churikova, T. G., Gordeychik, B. N., Edwards, B. R., Ponomareva, V. V., and Zelenin, E. A. (2015a). The Tolbachik Volcanic Massif: A Review of the Petrology, Volcanology and Eruption History Prior to the 2012-2013 Eruption. J. Volcanology Geothermal Res. 307, 3–21. doi:10.1016/j.jvolgeores.2015.10.016
Churikova, T. G., Gordeychik, B. N., Iwamori, H., Nakamura, H., Ishizuka, O., Nishizawa, T., et al. (2015b). Petrological and Geochemical Evolution of the Tolbachik Volcanic Massif, Kamchatka, Russia. J. Volcanology Geothermal Res. 307, 156–181. doi:10.1016/j.jvolgeores.2015.10.026
Dale, C. W., Burton, K. W., Greenwood, R. C., Gannoun, A., Wade, J., Wood, B. J., et al. (2012). Late Accretion on the Earliest Planetesimals Revealed by the Highly Siderophile Elements. Science 336 (6077), 72–75. doi:10.1126/science.1214967
Dale, C. W., Burton, K. W., Pearson, D. G., Gannoun, A., Alard, O., Argles, T. W., et al. (2009). Highly Siderophile Element Behaviour Accompanying Subduction of Oceanic Crust: Whole Rock and mineral-scale Insights from a High-Pressure Terrain. Geochimica et Cosmochimica Acta 73 (5), 1394–1416. doi:10.1016/j.gca.2008.11.036
Dale, C. W., Luguet, A., Macpherson, C. G., Pearson, D. G., and Hickey-Vargas, R. (2008). Extreme Platinum-Group Element Fractionation and Variable Os Isotope Compositions in Philippine Sea Plate Basalts: Tracing Mantle Source Heterogeneity. Chem. Geology. 248 (3-4), 213–238. doi:10.1016/j.chemgeo.2007.11.007
Ely, J. C., and Neal, C. R. (2003). Using Platinum-Group Elements to Investigate the Origin of the Ontong Java Plateau, SW Pacific. Chem. Geology. 196 (1-4), 235–257. doi:10.1016/s0009-2541(02)00415-1
Evans, K. A., Elburg, M. A., and Kamenetsky, V. S. (2012). Oxidation State of Subarc Mantle. Geology 40 (9), 783–786. doi:10.1130/G33037.1
Finnigan, C. S., Brenan, J. M., Mungall, J. E., and McDonough, W. F. (2008). Experiments and Models Bearing on the Role of Chromite as a Collector of Platinum Group Minerals by Local Reduction. J. Petrol. 49 (9), 1647–1665. doi:10.1093/petrology/egn041
Fleet, M. E., and Stone, W. E. (1991). Partitioning of Platinum-Group Elements in the Fe-Ni-S System and Their Fractionation in Nature. Geochimica et Cosmochimica Acta 55, 245–253. doi:10.1016/0016-7037(91)90415-2
Fleet, M. E., Tronnes, R. G., and Stone, W. E. (1991). Partitioning of Platinum Group Elements in the Fe-O-S System to 11 GPa and Their Fractionation in the Mantle and Meteorites. J. Geophys. Res. 96 (B13), 21949–21958. doi:10.1029/91jb02172
Flerov, G. B., Andreev, V. N., Budnikov, V. A., and Tsyurupa, A. I. (1984). “Petrology of the Eruption Products,” in Great Tolbachik Fissure Eruption, Kamchatka, 1975–1976. Editor S. A. Fedotov (Moscow: Nauka), 223–284.
Garuti, G., Zaccarini, F., Moloshag, V., and Alimov, V. (1999). Platinum-group Minerals as Indicators of Sulfur Fugacity in Ophiolitic Upper Mantle; an Example from Chromitites of the Ray-Iz Ultramafic Complex, Polar Urals, Russia. Can. Mineral. 37 (5), 1099–1115.
Govindaraju, K. (1994). Compilation of Working Values and Sample Description for 383 Geostandards. Geostandards Newsletter. J. Geostand. Geoanal., 18–158. doi:10.1046/j.1365-2494.1998.53202081.x-i1
Hao, H., Campbell, I. H., Arculus, R. J., and Perfit, M. R. (2021). Using Precious Metal Probes to Quantify Mid-ocean ridge Magmatic Processes. Earth Planet. Sci. Lett. 553, 116603. doi:10.1016/j.epsl.2020.116603
Jenner, F. E., and O’Neill, H. S. C. (2012). Analysis of 60 Elements in 616 Ocean Floor Basaltic Glasses. Geochem. Geophys. Geosyst. 13 (1), a–n. doi:10.1029/2011GC004009
Kamenetsky, V. S., Park, J.-W., Mungall, J. E., Pushkarev, E. V., Ivanov, A. V., Kamenetsky, M. B., et al. (2015). Crystallization of Platinum-Group Minerals from Silicate Melts: Evidence from Cr-Spinel-Hosted Inclusions in Volcanic Rocks. Geology 43 (10), 903–906. doi:10.1130/G37052.1
Kamenetsky, V. S., Zelenski, M., Gurenko, A., Portnyagin, M., Ehrig, K., Kamenetsky, M., et al. (2017). Silicate-sulfide Liquid Immiscibility in Modern Arc basalt (Tolbachik Volcano, Kamchatka): Part II. Composition, Liquidus Assemblage and Fractionation of the Silicate Melt. Chem. Geology. 471 (February), 92–110. doi:10.1016/j.chemgeo.2017.09.019
Keays, R. R., Sewell, D. K. B., and Mitchell, R. H. (1981). Platinum and Palladium Minerals in Upper Mantle-Derived Lherzolites. Nature 294, 646–648. doi:10.1038/294646a0
Kelley, K. A., Kingsley, R., and Schilling, J.-G. (2013). Composition of Plume-Influenced Mid-ocean ridge Lavas and Glasses from the Mid-Atlantic Ridge, East Pacific Rise, Galápagos Spreading Center, and Gulf of Aden. Geochem. Geophys. Geosyst. 14 (1), 223–242. doi:10.1002/ggge.20049
Kepezhinskas, P., Defant, M. J., and Widom, E. (2002). Abundance and Distribution of PGE and Au in the Island-Arc Mantle: Implications for Sub-arc Metasomatism. Lithos 60 (3-4), 113–128. doi:10.1016/S0024-4937(01)00073-1
Kutyrev, A. V., Kamenetsky, V. S., Park, J.-W., Maas, R., Demonterova, E. I., Antsiferova, T. N., et al. (2021). Primitive High-K Intraoceanic Arc Magmas of Eastern Kamchatka: Implications for Paleo-Pacific Tectonics and Magmatism in the Cretaceous. Earth-Science Rev. 220, 103703–103722. doi:10.1016/j.earscirev.2021.103703
Li, C., Barnes, S.-J., Makovicky, E., Rose-Hansen, J., and Makovicky, M. (1996). Partitioning of Nickel, Copper, Iridium, Rhenium, Platinum, and Palladium between Monosulfide Solid Solution and Sulfide Liquid: Effects of Composition and Temperature. Geochimica et Cosmochimica Acta 60 (7), 1231–1238. doi:10.1016/0016-7037(96)00009-9
Locmelis, M., Pearson, N. J., Barnes, S. J., and Fiorentini, M. L. (2011). Ruthenium in Komatiitic Chromite. Geochimica et Cosmochimica Acta 75 (13), 3645–3661. doi:10.1016/j.gca.2011.03.041
Lorand, J. P., and Ceuleneer, G. (1989). Silicate and Base-Metal Sulfide Inclusions in Chromites from the Maqsad Area (Oman Ophiolite, Gulf of Oman): A Model for Entrapment. Lithos 22 (3), 173–190. doi:10.1016/0024-4937(89)90054-6
Luguet, A., Lorand, J.-P., Alard, O., and Cottin, J.-Y. (2004). A Multi-Technique Study of Platinum Group Element Systematic in Some Ligurian Ophiolitic Peridotites, Italy. Chem. Geology. 208 (1-4), 175–194. doi:10.1016/j.chemgeo.2004.04.011
Luguet, A., Lorand, J.-P., and Seyler, M. (2003). Sulfide Petrology and Highly Siderophile Element Geochemistry of Abyssal Peridotites: a Coupled Study of Samples from the Kane Fracture Zone (45°W 23°20N, MARK Area, Atlantic Ocean). Geochimica et Cosmochimica Acta 67 (8), 1553–1570. doi:10.1016/s0016-7037(02)01133-x
Lyubetskaya, T., and Korenaga, J. (2007). Chemical Composition of Earth's Primitive Mantle and its Variance: 1. Method and Results. J. Geophys. Res. 112 (3), 1–21. doi:10.1029/2005JB004223
McInnes, B. I. A., McBride, J. S., Evans, N. J., Lambert, D. D., and Andrew, A. S. (1999). Osmium Isotope Constraints on Ore Metal Recycling in Subduction Zones. Science 286 (5439), 512–516. doi:10.1126/science.286.5439.512
Meisel, T., and Moser, J. (2004). Reference Materials for Geochemical PGE Analysis: New Analytical Data for Ru, Rh, Pd, Os, Ir, Pt, and Re by Isotope Dilution ICP-MS in 11 Geological Reference Materials. Chem. Geol. 208, 319–338. doi:10.1016/j.chemgeo.2004.04.019
Mironov, N. L., and Portnyagin, M. V. (2018). Coupling of Redox Conditions of Mantle Melting and Copper and Sulfur Contents in Primary Magmas of the Tolbachinsky Dol (Kamchatka) and Juan de Fuca Ridge (Pacific Ocean). Petrology 26, 145–166. doi:10.1134/S0869591118020030
Mungall, E., Andrews, D. R. A., Cabri, L. J., Sylvester, P. J., and Tubrett, M. (2005). Partitioning of Cu, Ni, Au, and Platinum-Group Elements between Monosulfide Solid Solution and Sulfide Melt under Controlled Oxygen and Sulfur Fugacities. Geochimica et Cosmochimica Acta 69 (17), 4349–4360. doi:10.1016/j.gca.2004.11.025
Mungall, J. E., and Brenan, J. M. (2014). Partitioning of Platinum-Group Elements and Au between Sulfide Liquid and basalt and the Origins of Mantle-Crust Fractionation of the Chalcophile Elements. Geochimica et Cosmochimica Acta 125, 265–289. doi:10.1016/j.gca.2013.10.002
Nekrylov, N., Portnyagin, M. V., Kamenetsky, V. S., Mironov, N. L., Churikova, T. G., Plechov, P. Y., et al. (2018). Chromium Spinel in Late Quaternary Volcanic Rocks from Kamchatka: Implications for Spatial Compositional Variability of Subarc Mantle and its Oxidation State. Lithos 322, 212–224. doi:10.1016/j.lithos.2018.10.011
O’Driscoll, B., and González-Jiménez, J. M. (2016). Petrogenesis of the Platinum-Group Minerals. Rev. Mineralogy Geochem. 81 (1), 489–578. doi:10.2138/rmg.2016.81.09
Park, J.-W., Campbell, I. H., and Arculus, R. J. (2013). Platinum-alloy and Sulfur Saturation in an Arc-Related basalt to Rhyolite Suite: Evidence from the Pual Ridge Lavas, the Eastern Manus Basin. Geochimica et Cosmochimica Acta 101, 76–95. doi:10.1016/j.gca.2012.10.001
Park, J.-W., Campbell, I. H., and Eggins, S. M. (2012). Enrichment of Rh, Ru, Ir and Os in Cr Spinels from Oxidized Magmas: Evidence from the Ambae Volcano, Vanuatu. Geochimica et Cosmochimica Acta 78, 28–50. doi:10.1016/j.gca.2011.11.018
Park, J.-W., Kamenetsky, V., Campbell, I., Park, G., Hanski, E., and Pushkarev, E. (2017). Empirical Constraints on Partitioning of Platinum Group Elements between Cr-Spinel and Primitive Terrestrial Magmas. Geochimica et Cosmochimica Acta 216, 393–416. doi:10.1016/j.gca.2017.05.039
Pearce, J. A., and Parkinson, I. J. (1993). Trace Element Models for Mantle Melting: Application to Volcanic Arc Petrogenesis. Geol. Soc. Lond. Spec. Publications 76 (1), 373–403. doi:10.1144/gsl.Sp.1993.076.01.19
Peucker-Ehrenbrink, B., Bach, W., Hart, S. R., Blusztajn, J. S., and Abbruzzese, T. (2003). Rhenium-osmium Isotope Systematics and Platinum Group Element Concentrations in Oceanic Crust from DSDP/ODP Sites 504 and 417/418. Geochem. Geophys. Geosyst. 4 (7), 1–28. doi:10.1029/2002GC000414
Plessen, H.-G., and Erzinger, J. (1998). Determination of the Platinum-Group Elements and Gold in Twenty Rock Reference Materials by Inductively Coupled Plasma-Mass Spectrometry (ICP-MS) After Pre-Concentration by Nickel Sulfide Fire Assay. Geostandarts Newsletter 22, 187–194. doi:10.1111/j.1751-908X.1998.tb00691.x
Portnyagin, M., Bindeman, I., Hoernle, K., and Hauff, F. (2007). “Geochemistry of Primitive Lavas of the Central Kamchatka Depression: Magma Generation at the Edge of the Pacific Plate,” in Volcanism and Subduction: The Kamchatka Region. Editors J. Eichelberger, E. Gordeev, P. Izbekov, M. Kasahara, and J. Lees (Washington D.C.: American Geophysical Union), 203–244. doi:10.1029/172gm16
Portnyagin, M., Duggen, S., Hauff, F., Mironov, N., Bindeman, I., Thirlwall, M., et al. (2015). Geochemistry of the Late Holocene Rocks from the Tolbachik Volcanic Field, Kamchatka: Quantitative Modelling of Subduction-Related Open Magmatic Systems. J. Volcanology Geothermal Res. 307, 133–155. doi:10.1016/j.jvolgeores.2015.08.015
Prichard, H. M., Barnes, S. J., Dale, C. W., Godel, B., Fisher, P. C., and Nowell, G. M. (2017). Paragenesis of Multiple Platinum-Group mineral Populations in Shetland Ophiolite Chromitite: 3D X-ray Tomography and In Situ Os Isotopes. Geochimica et Cosmochimica Acta 216, 314–334. doi:10.1016/j.gca.2017.03.035
Prichard, H. M., Lord, R. A., and Neary, C. R. (1996a). A Model to Explain the Occurrence of Platinum- and Palladium-Rich Ophiolite Complexes. J. Geol. Soc. 153 (2), 323–328. doi:10.1144/gsjgs.153.2.0323
Prichard, H. M., Puchelt, H., Eckhardt, J.-D., and Fisher, P. C. (1996b). Platinum-group Element Concentrations in Mafic and Ultramafic Lithologies Drilled from Hess Deep. Proc. Ocean Drilling Program Scientific Results 147, 77–90. doi:10.2973/odp.proc.sr.147.004.1996
Reekie, C. D. J., Jenner, F. E., Smythe, D. J., Hauri, E. H., Bullock, E. S., and Williams, H. M. (2019). Sulfide Resorption during Crustal Ascent and Degassing of Oceanic Plateau Basalts. Nat. Commun. 10 (1), 1–11. doi:10.1038/s41467-018-08001-3
Rehkämper, M., Halliday, A. N., Fitton, J. G., Lee, D. C., Wienke, M., and Arndt, N. (1999). Ir, Ru, Pt, and Pd in Basalts and Komatiites: New Constraints for the Geochemical Behavior of the Platinum-Group Elements in the Mantle. Geochimica et Cosmochimica Acta 63, 3915–3934. doi:10.1016/S0016-7037(99)00219-7
Savard, D., Barnes, S.-J., and Meisel, T. (2010). Comparison Between Nickel-Sulfur Fire Assay Te Co-Precipitation and Isotope Dilution With High-Pressure Asher Acid Digestion for the Determination of Platinum-Group Elements, Rhenium and Gold. Geostand. Geoanalytical Res. 34, 281–291. doi:10.1111/j.1751-908X.2010.00090.x
Savelyev, D. P., Kamenetsky, V. S., Danyushevsky, L. V., Botcharnikov, R. E., Kamenetsky, M. B., Park, J.-W., et al. (2018a). Immiscible Sulfide Melts in Primitive Oceanic Magmas: Evidence and Implications from Picrite Lavas (Eastern Kamchatka, Russia). Am. Mineral. 103 (6), 886–898. doi:10.2138/am-2018-6352
Savelyev, D. P., Palesskii, S. V., and Portnyagin, M. V. (2018b). The Source of Platinum Group Elements in Basalts of the Ophiolite Complex of the Kamchatsky Mys Peninsula (Eastern Kamchatka). Russ. Geology. Geophys. 59 (12), 1592–1602. doi:10.1016/j.rgg.2018.12.005
Setiabudi, B. T., Campbell, I. H., Martin, C. E., and Allen, C. M. (2007). Platinum Group Element Geochemistry of Andesite Intrusions of the Kelian Region, East Kalimantan, Indonesia: Implications of Gold Depletion in the Intrusions Associated with the Kelian Gold deposit. Econ. Geology. 102 (1), 95–108. doi:10.2113/gsecongeo.102.1.95
Siegrist, M., Yogodzinski, G., Bizimis, M., Fournelle, J., Churikova, T., Dektor, C., et al. (2019). Fragments of Metasomatized Forearc: Origin and Implications of Mafic and Ultramafic Xenoliths from Kharchinsky Volcano, Kamchatka. Geochem. Geophys. Geosyst. 20 (9), 4426–4456. doi:10.1029/2019gc008478
Siegrist, M., Yogodzinski, G. M., and Bizimis, M. (2021). Origins of Os-Isotope and Platinum-Group Element Compositions of Metasomatized Peridotite and Cumulate Pyroxenite Xenoliths from Kharchinsky Volcano, Kamchatka. Geochimica et Cosmochimica Acta 299, 130–150. doi:10.1016/j.gca.2021.01.045
Tolstykh, N., Sidorov, E., and Kozlov, A. (2009). Platinum-group Minerals from the Olkhovaya-1 Placers Related to the Karaginsky Ophiolite Complex, Kamchatskiy Mys peninsula, Russia. Can. Mineral. 47 (5), 1057–1074. doi:10.3749/canmin.47.5.1057
Volynets, A. O., Edwards, B. R., Melnikov, D., Yakushev, A., and Griboedova, I. (2015). Monitoring of the Volcanic Rock Compositions during the 2012-2013 Fissure Eruption at Tolbachik Volcano, Kamchatka. J. Volcanology Geothermal Res. 307, 120–132. doi:10.1016/j.jvolgeores.2015.07.014
Waterton, P., Mungall, J., and Pearson, D. G. (2021). The Komatiite-Mantle Platinum-Group Element Paradox. Geochimica et Cosmochimica Acta 313, 214–242. doi:10.1016/j.gca.2021.07.037
Yang, A. Y., Zhou, M.-F., Zhao, P., Deng, X.-G., Qi, L., and Xu, J.-F. (2014). Chalcophile Elemental Compositions of MORBs from the Ultraslow-Spreading Southwest Indian Ridge and Controls of Lithospheric Structure on S-Saturated Differentiation. Chem. Geology. 382, 1–13. doi:10.1016/j.chemgeo.2014.05.019
Zaccarini, F., Garuti, G., Pushkarev, E., and Thalhammer, O. (2018). Origin of Platinum Group Minerals (PGM) Inclusions in Chromite Deposits of the Urals. Minerals 8 (9), 379. doi:10.3390/min8090379
Zelenski, M., Kamenetsky, V. S., and Hedenquist, J. (2016). Gold Recycling and Enrichment beneath Volcanoes: A Case Study of Tolbachik, Kamchatka. Earth Planet. Sci. Lett. 437, 35–46. doi:10.1016/j.epsl.2015.12.034
Zelenski, M., Kamenetsky, V. S., Mavrogenes, J. A., Danyushevsky, L. V., Matveev, D., and Gurenko, A. A. (2017). Platinum-group Elements and Gold in Sulfide Melts from Modern Arc basalt (Tolbachik Volcano, Kamchatka). Lithos 290-291, 172–188. doi:10.1016/j.lithos.2017.08.012
Zelenski, M., Kamenet, V. S., Mavrogenes, J. A., Gurenko, A. A., and Danyushevsky, . V. (2018). Silicate-sulfide Liquid Immiscibility in Modern Arc basalt (Tolbachik Volcano, Kamchatka): Part I. Occurrence and Compositions of Sulfide Melts. Chem. Geology. 478 (April 2017), 102–111. doi:10.1016/j.chemgeo.2017.09.013
Keywords: basalt, island arc and continental margin arc environment, subduction, platinum, PGE, gold, sulfide, immiscibility
Citation: Kutyrev A, Zelenski M, Nekrylov N, Savelyev D, Kontonikas-Charos A and Kamenetsky VS (2021) Noble Metals in Arc Basaltic Magmas Worldwide: A Case Study of Modern and Pre-Historic Lavas of the Tolbachik Volcano, Kamchatka. Front. Earth Sci. 9:791465. doi: 10.3389/feart.2021.791465
Received: 08 October 2021; Accepted: 17 November 2021;
Published: 02 December 2021.
Edited by:
Philipp A. Brandl, Helmholtz Association of German Research Centres (HZ), GermanyReviewed by:
John Mavrogenes, Australian National University, AustraliaIlya Bindeman, University of Oregon, United States
Copyright © 2021 Kutyrev, Zelenski, Nekrylov, Savelyev, Kontonikas-Charos and Kamenetsky. This is an open-access article distributed under the terms of the Creative Commons Attribution License (CC BY). The use, distribution or reproduction in other forums is permitted, provided the original author(s) and the copyright owner(s) are credited and that the original publication in this journal is cited, in accordance with accepted academic practice. No use, distribution or reproduction is permitted which does not comply with these terms.
*Correspondence: Anton Kutyrev, YW50b24udi5rdXR5cmV2QGdtYWlsLmNvbQ==