- 1Instituto de Geografía, Pontificia Universidad Católica de Chile, Santiago, Chile
- 2Núcleo Milenio Paleoclima, Santiago, Chile
- 3Estación Patagonia de Investigaciones Interdisciplinarias UC, Pontificia Universidad Católica de Chile, Santiago, Chile
- 4Departamento de Geología, Universidad de Chile, Santiago, Chile
- 5Lamont-Doherty Earth Observatory, Columbia University, Palisades, NY, United States
- 6Gaia-Antártica, Universidad de Magallanes, Punta Arenas, Chile
- 7Departamento de Geofísica, Universidad de Chile, Santiago, Chile
- 8Department of Geography and Environment, University of Western Ontario, London, ON, Canada
- 9Department of Earth and Environmental Sciences, Columbia University, New York, NY, United States
The causes underlying Holocene glacier fluctuations remain elusive, despite decades of research efforts. Cosmogenic nuclide dating has allowed systematic study and thus improved knowledge of glacier-climate dynamics during this time frame, in part by filling in geographical gaps in both hemispheres. Here we present a new comprehensive Holocene moraine chronology from Mt. San Lorenzo (47°S) in central Patagonia, Southern Hemisphere. Twenty-four new 10Be ages, together with three published ages, indicate that the Río Tranquilo glacier approached its Holocene maximum position sometime, or possibly on multiple occasions, between 9,860 ± 180 and 6,730 ± 130 years. This event(s) was followed by a sequence of slightly smaller advances at 5,750 ± 220, 4,290 ± 100 (?), 3,490 ± 140, 1,440 ± 60, between 670 ± 20 and 430 ± 20, and at 390 ± 10 years ago. The Tranquilo record documents centennial to millennial-scale glacier advances throughout the Holocene, and is consistent with recent glacier chronologies from central and southern Patagonia. This pattern correlates well with that of multiple moraine-building events with slightly decreasing net extent, as is observed at other sites in the Southern Hemisphere (i.e., Patagonia, New Zealand and Antarctic Peninsula) throughout the early, middle and late Holocene. This is in stark contrast to the typical Holocene mountain glacier pattern in the Northern Hemisphere, as documented in the European Alps, Scandinavia and Canada, where small glaciers in the early-to-mid Holocene gave way to more-extensive glacier advances during the late Holocene, culminating in the Little Ice Age expansion. We posit that this past asymmetry between the Southern and Northern hemisphere glacier patterns is due to natural forcing that has been recently overwhelmed by anthropogenic greenhouse gas driven warming, which is causing interhemispherically synchronized glacier retreat unprecedented during the Holocene.
Introduction
Following an early Holocene glacier minimum, most of the glacierised areas around the world experienced a phase of renewed glacier advances, a phenomenon commonly known as Neoglaciation (Porter and Denton 1967). Since their recognition by the middle of the last century, numerous studies have attempted to decipher the causes underlying these glacier fluctuations (e.g., Grove 2004) by comparing the sequence and temporal phasing of advances in disparate regions of the world with terrestrial or extraterrestrial climate-forcing mechanisms (e.g., Denton and Karlen 1973; Bond et al., 2001; Denton and Broecker 2008). Such an understanding is crucial, as it may provide key insights into the mechanisms governing abrupt climate changes and any corresponding cryospheric response. However, further advances in these efforts have been limited by the lack of a well-distributed global network of high-resolution glacier chronologies.
In the case of Patagonia (South America between 40° and 54°S), several chronological models of Holocene glacier fluctuations have been proposed (Mercer 1982; Clapperton and Sugden 1988; Aniya 1995; Aniya 2013). These models—relaying predominantly on radiocarbon dating—differ significantly from each other and more recent studies, and make difficult any effort to understand the recent glacial history of southern South America. The apparent differences between chronological models can be attributed to: i) the reliance on minimum (maximum)-limiting ages, which tend to underestimate (overestimate) the true age of the landforms; ii) the incompleteness of the chronologies due to the limited availability of datable organic matter to conduct radiocarbon analysis; and iii) the fragmentary nature of the surficial glacial record (i.e., due to erosional censoring typical for glaciers, few sites are able to preserve the evidence of every glacier advance that occurred in the area) (Glasser et al., 2005; Strelin et al., 2011; Reynhout et al., 2019). As a consequence, significant gaps remain in our knowledge of centennial- and millennial-scale glacier responses under natural climate variability.
Advances in surface exposure dating techniques, involving both sample processing (e.g., low process blanks) and AMS measurements, have opened new horizons for the development of high-resolution Holocene glacier chronologies based on the analysis of low 10Be/9Be ratios (Schaefer et al., 2009) (e.g., ∼1014−15 in Table 1). These improvements, combined with the establishment of a Patagonian 10Be production rate (Kaplan et al., 2011), have recently allowed the emergence of a new generation of comprehensive moraine chronologies from southern South America spanning much or all of the Holocene (i.e., Strelin et al., 2014; Kaplan et al., 2016; Reynhout et al., 2019; García et al., 2020). These 10Be chronologies have been for the most part, developed within a narrow latitudinal band at around 49–51°S in southern Patagonia, and they indicate that in this locale glaciers underwent a series of progressively less extensive advances throughout most of the Holocene.
Recently published glacier chronologies, moreover, show promising consistency with pollen-inferred changes in the intensity of the Southern Westerly Winds (SWW) (Moreno et al., 2018). Modern SWW intensity is positively correlated with annual precipitation, and negatively correlated with summer temperatures throughout western Patagonia (see Figures 5, 8 in Garreaud et al., 2013). Accordingly, strong westerlies are associated with the cold and wet conditions ideal for glacier expansion; weaker westerlies, on the other hand, are associated with warmer and drier conditions, promoting glacier withdrawal. Based on the apparent relationship with past Westerlies behavior, it has been hypothesized that Holocene glacier fluctuations in southern Patagonia were paced by centennial-scale temperature and precipitation anomalies associated with changes in the SWW intensity (Moreno et al., 2018; Reynhout et al., 2019). To test and validate this hypothesis across the whole of Patagonia requires expanding the spatial network of study sites, to assess the regional representativeness of emerging 10Be chronologies.
Here, we set out to refine the structure and timing of Holocene glacier fluctuations of southern South America by developing a new comprehensive 10Be moraine chronology in the Río Tranquilo valley, on the northern flank of Mt. San Lorenzo (47°35′ S; 72°19′ W). Located roughly in central Patagonia, Mt. San Lorenzo is located to the east of a tectonic depression that breaks the Andes (and separates the Patagonian Ice Fields), and despite being more than 160 km from the Pacific Ocean, it is the first major orographic barrier to the moisture-laden SWW (Mendelová et al., 2020b). Consequently, Mt. San Lorenzo is ideally located to track the position of the Westerlies and assess their role in pacing Holocene glacier fluctuations in Patagonia (Moreno et al., 2018; Reynhout et al., 2019).
Study Area
Mt. San Lorenzo (47°35′S, 72° 21′W) is an isolated granodiorite massif astride the border between Chile and Argentina, 80 km to the east of the topographic divide of the Andes (Figure 1). Mt. San Lorenzo exhibits a transitional maritime to continental climate, with a wide annual thermal amplitude and a narrow range of mean monthly precipitation (Falaschi et al., 2019). At 3,706 m a.s.l., Mt. San Lorenzo is one of the most extensively glacierized mountains in the region, with an ice-covered area of ca. 139 km2. For the years 2005/2008, Falaschi et al. (2013) estimated the snowline to be ∼1,700–1,750 m a.s.l. on the western sector of the massif and ∼1,800 m asl on the eastern side. This study focuses on the Rio Tranquilo valley, a north-south oriented valley on the northern flank of Mt. San Lorenzo. The headwall of this valley is home to 16 small glaciers <5 km2 which collectively cover an estimated area of 15 km2 (Falaschi et al., 2013).
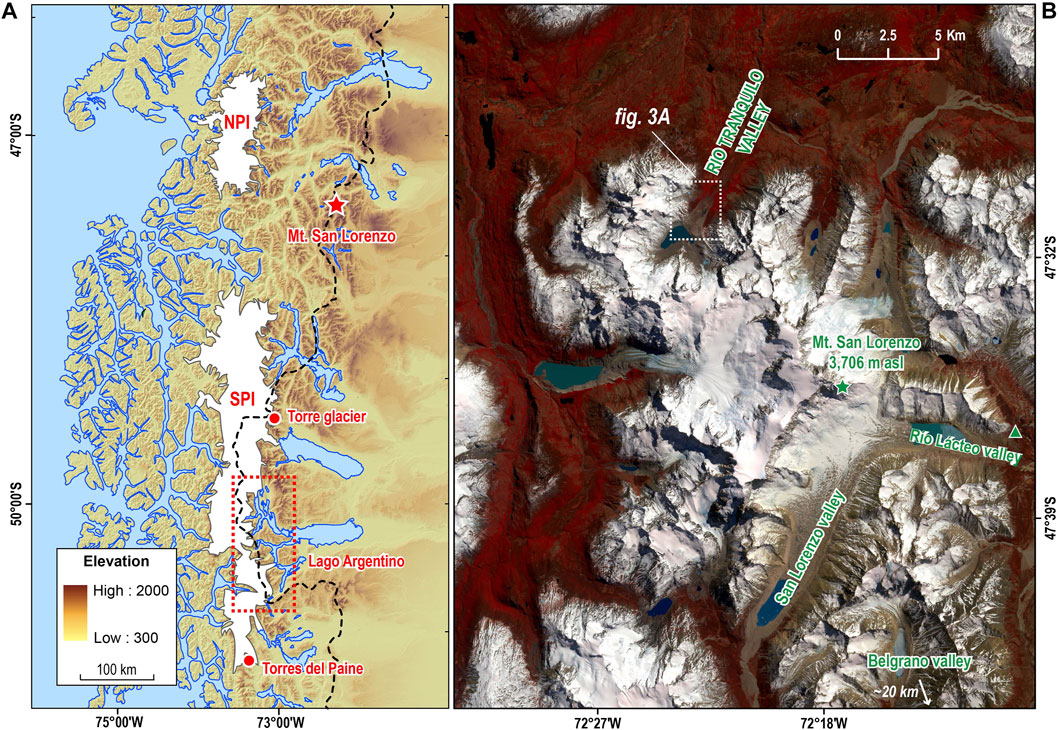
FIGURE 1. Geographic setting of this and prior studies. (A) Regional overview of the Patagonian Icefields and surrounding area. ALOS PALSAR composition (m asl) NPI, Northern Patagonian Icefield; SPI, Southern Patagonia Icefield. (B) Río Tranquilo valley, Mt. San Lorenzo. Sentinel 2 false color image (Infrared, 2016) showing the entire San Lorenzo massif and all the glaciers draining from its headwalls. Green star indicates Mt. San Lorenzo summit. Green triangle shows the approximated location of the available Holocene radiocarbon date (Mercer, 1968). Other sites discussed in the text are also shown.
Previous Studies
Nearly all studies that have reconstructed the extent and timing of glacier events on Mt. San Lorenzo have focused on the last glacial-interglacial transition (Wenzens 2005; Glasser et al., 2012; Horta et al., 2017; Davies et al., 2018; Sagredo et al., 2018; Martin et al., 2019; Mendelová et al., 2020a; Mendelová et al., 2020b). Among these studies, Sagredo et al. (2018) addressed the deglacial history specifically of the Tranquilo valley, where they identified major glacier advances during the Antarctic Cold Reversal (ACR; 13,680 ± 460 and 13,490 ± 430 years), followed by a minor stabilization during the Younger Dryas (YD; sometime in between 12,330 ± 410 and 11,460 ± 390 years). These findings are consistent with Mendelová et al. (2020a), who found glacier advances at 13,100 ± 600 and 12,400 ± 300 to the east, in the Belgrano area.
Studies regarding the Holocene glacier history of Mt. San Lorenzo are scarcer, and the available information mostly comprises minimum 14C ages, and other bracketing estimates produced by dendrochronology and lichenometry. Mercer (1968) obtained a minimum age of 5,210 ± 344 cal year BP for the most extensive Holocene glacier advance of the Río Lácteo glacier (Figure 1), based on a radiocarbon date of a tree stump buried in a moraine-dammed lagoon. This chronology was extended by Garibotti and Villalba (2017) who estimated the ages of the entire moraine sequence of the Río Lácteo valley using lichenometry, identifying glacier advances at 5,250 ± 360 years, 2,180 ± 50 years, 1,490 ± 20 years, and two advances during the last hundred years. Using the same approach, Garibotti and Villalba (2017) identified advances of the San Lorenzo glacier at 5,750 ± 430 years, 3,880 ± 180 years, 2,470 ± 70 years, as well as several glacier advances/stabilizations during the last few hundreds of years.
In the Tranquilo valley, using dendrochronology, Aravena (2007) dated a group of moraines in the upper section of the valley. Based on the oldest tree growing atop the ridges, he concluded that the entire sequence of moraines was deposited around or before AD 1670. However, the author also acknowledged the possibility that these moraines could be much older, arguing that their age estimate may be limited by the maximum biological ages that can be attained by Nothofagus pumilio (targeted species). Later on, Sagredo et al. (2017) partially confirmed this hypothesis by obtaining three coherent 10Be ages of 5,450 ± 100 years, 5,640 ± 110 years and 5,800 ± 150 years (recalculated here) from a single ridge in the middle of the same moraine sequence study by Aravena (2007).
Materials and Methods
To constrain the age of former Holocene glacier fluctuations in the Tranquilo valley, we mapped and directly dated seven moraine crests using 10Be surface exposure dating. Detailed geomorphological mapping built upon previous work by Sagredo et al. (2018). In addition to field observations, we used analyses of satellite imagery from Google Earth (2021 Cnes/SPOT, ∼15 m spatial resolution), aerial photographs (GEOTEC 1:70,000) and digital elevation models (ALOS Palsar). For exposure dating, we collected samples (∼1 kg) from boulders embedded in or resting on stable positions atop the moraine ridges in the upper section of the Tranquilo valley. We selected tall boulders (preferably >1 m) that did not show evidence of post-depositional movement, surface erosion, or exhumation. Samples were taken from the upper ∼5 cm of the boulder surface using a hammer and chisel, and the blast method of Kelly (2003). We recorded topographic skylines, as well as surficial strike and dip measurements, using a handheld transit and clinometer. We measured the coordinates and altitude of samples using a handheld commercial GPS (WGS84) with an assumed uncertainty of <10 m. Table 1 provides geographical and analytical data of the samples. Quartz isolation and beryllium extraction were performed at the Cosmogenic Nuclide Laboratory at the Lamont-Doherty Earth Observatory (LDEO), Columbia University (NY, United States), following Schaefer et al. (2009). 10Be/Be isotope ratios were measured at the Center for Accelerator Mass Spectrometry at Lawrence Livermore National Laboratory (CAMS LLNL). We used version 3 of the CRONUS-Earth online calculator (Balco et al., 2008), along with a regional nuclide production rate developed at Lago Argentino, Patagonia (50°S; Kaplan et al., 2011), assuming zero erosion. Ages discussed here are based on the time dependent version of Stone/Lal’s scaling protocol scheme (Lm) (Lal 1991; Stone 2000); however, the choice of scheme does not impact our main conclusions (Table 2). We report individual 10Be ages with 1σ analytical uncertainty. We calculate the arithmetic mean ages for moraines and present them with the standard error of the mean and a 3% propagated production rate error (Peltier et al., 2021). Given the sampled boulders were sitting atop the moraines, we assume they were likely deposited at the end of moraine construction episodes; therefore, we interpret the 10Be dates as most likely representing close minimum ages for the culmination of a glacier advance.10Be ages from previous studies were recalculated following the same procedures as above. Previously published radiocarbon ages were recalibrated (cal yr BP) using the SHCal 20 curve (Hogg et al., 2020) in OxCal v. 4.4 (Ramsey 2009).
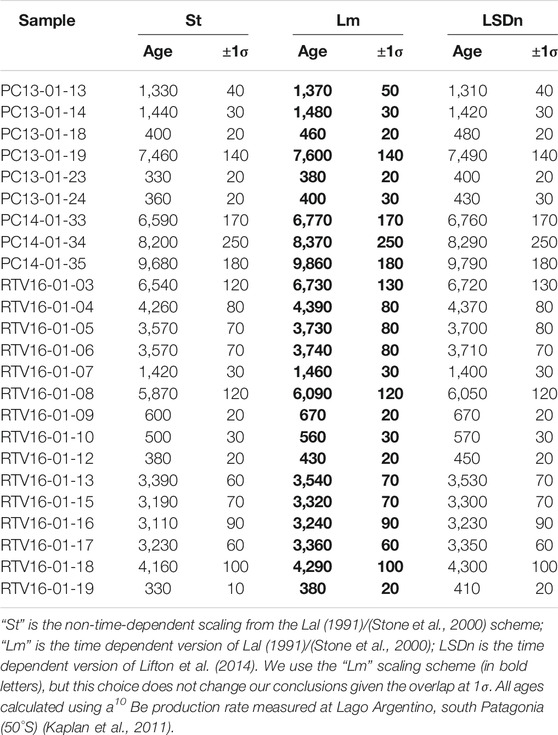
TABLE 2. All the new 10Be were ages calculated using CRONUS v. 3 (Balco et al., 2008) and are reported with 1σ internal (analytical) error.
Results
Sagredo et al. (2018) mapped and dated five late-glacial moraines (dubbed RT1-RT5) in the lower reaches of the Tranquilo valley. Upstream, on both sides of the valley, between five and seven well-defined lateral moraine crests were identified (RT6 moraines) (Figure 2). RT6 moraine ridges crosscut each other in multiple places documenting readvances of the glacier front (Figures 3, 4). Given the frontal sections of all of the RT6 moraines are not preserved, we cannot precisely determine the maximum glacier extent during these events; however, based on the geometry and slope of the lateral ridges, we infer that the Río Tranquilo glacier reached an extent of between 6 and 7 km from the headwall. Poorly-preserved moraine/glacial drift was found beyond (i.e., distal) RT6, while inward (proximal) of RT6 remnants of small, degraded ridges are occasionally preserved (Sagredo et al., 2017) (Figure 2). To avoid error associated with post-depositional disturbances of these poorly-preserved landforms, our chronology focuses on the relatively intact RT6 moraine ridges. Here, we present 24 new ages, which constrain the history of glacier advances in the Tranquilo valley during the Holocene (Figures 3, 4; Table 2). Hereafter, when referring to the right (left) moraine or side of the valley, we always mean the true-right (true-left); in other words, the location is identified in relation to an observer looking in the direction of the ice-flow (i.e., down-valley).
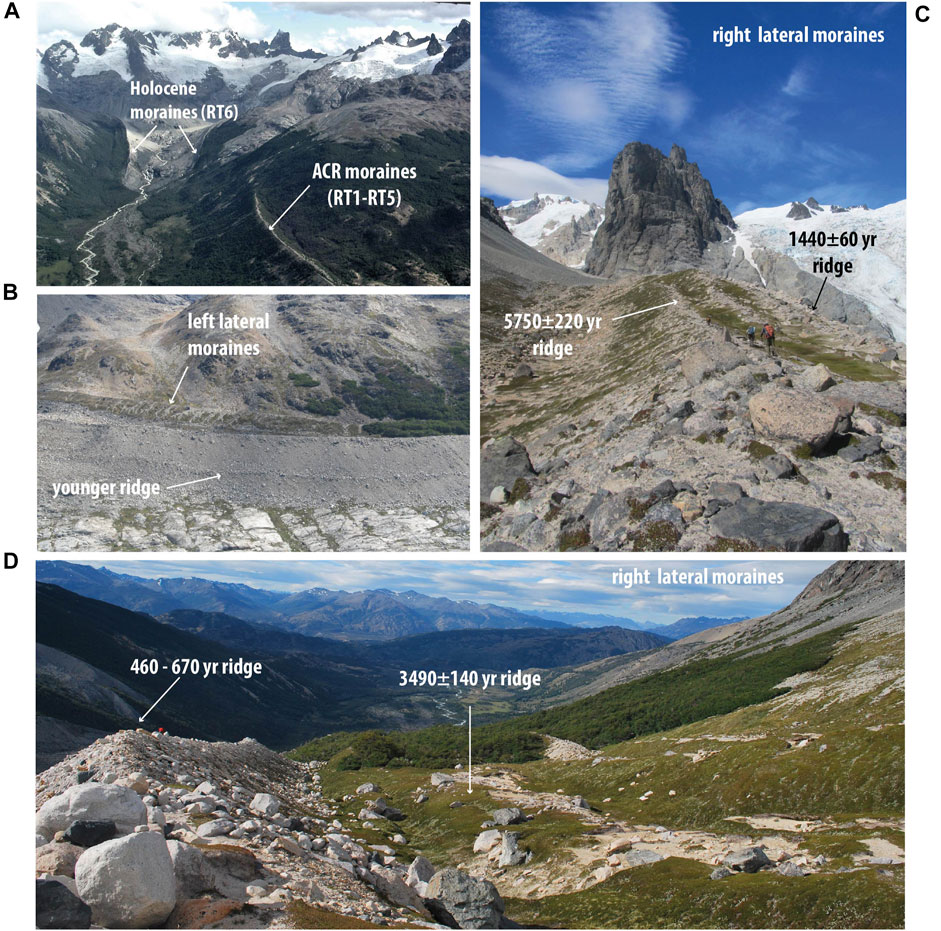
FIGURE 2. Photographs of the moraines and settings in the Río Tranquilo valley. (A) Late glacial (RT1-RT5) and Holocene (RT6) moraines. Note the difference in the magnitude between the glacier advances (Photo: Horacio Parraguez). (B) Left lateral moraines. Along the proximal slope it is possible to find remnants of younger recessional ridges. (C) Right lateral moraines (facing upstream). In this sector, the ∼3,500 years ridge was over-printed by the younger moraine. (D) Right lateral moraines (facing downstream). Note the difference in appearance between the younger and older ridges.
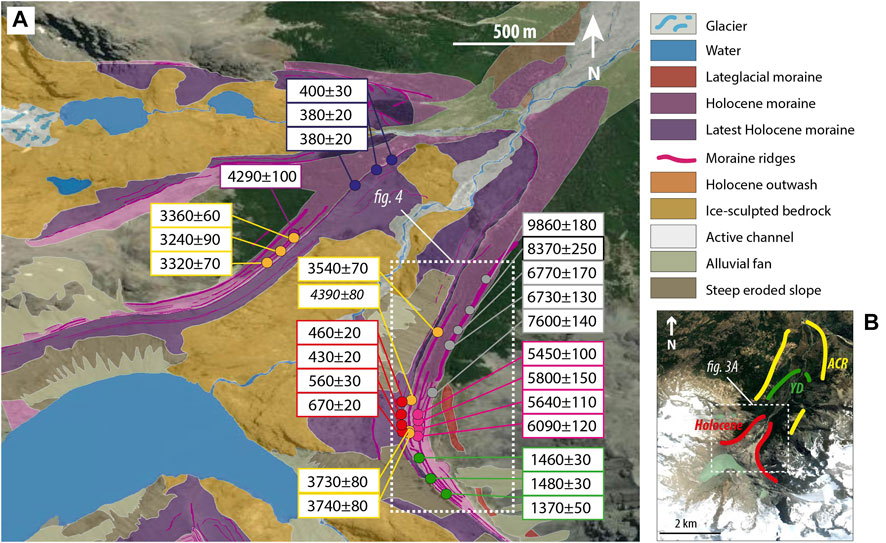
FIGURE 3. (A) Glacial geomorphologic map of the Río Tranquilo valley with new 10Be ages. Circles with the same color correspond to samples on the same moraine ridge or on correlated ridges on opposite sides of the valley. Individual 10Be ages are provided with 1σ analytical uncertainty (Table 2). One outlier is shown in italics. (B) Relative position of the Antarctic Cold Reversal (RT1-4 margins), Younger Dryas (RT5 margin) and Holocene margins (RT6) (Sagredo et al. 2017).
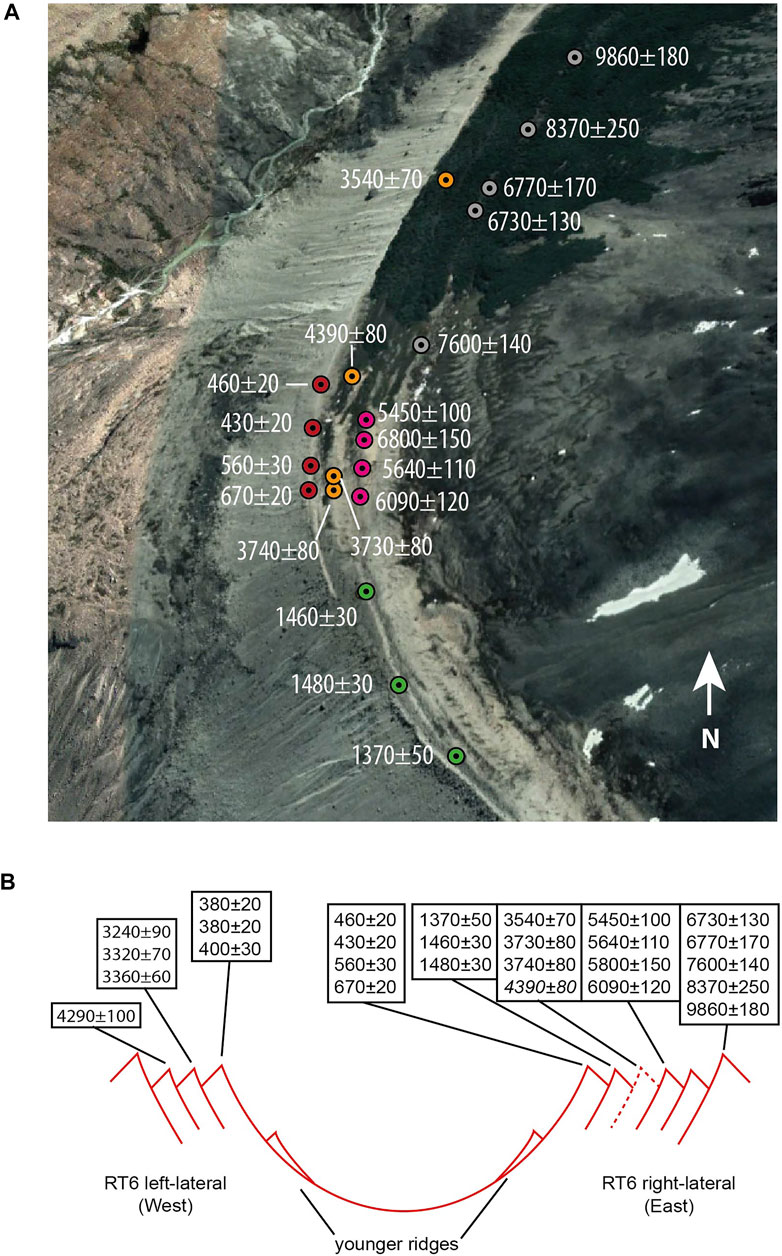
FIGURE 4. Glacier chronology of Río Tranquilo glacier. (A) 10Be ages obtained from the right lateral moraines (oblique perspective) on Google Earth image. (B) Schematic cross section of the valley. Notice that the ∼3,700 years ridge was destroyed by the ∼1,440 years advance in the upper right side of the valley (as represented by the dotted line) (Sagredo et al., 2017).
Right Lateral Moraines
The outermost RT6 ridge forms a continuous, well-defined ridge rising over 30 m in height downvalley. It gradually loses topographic relief upvalley before it disappears under the inner moraines (Figure 3). We collected five samples from boulders along the outermost RT6 ridge crest, yielding 10Be ages of 6,730 ± 130, 6,770 ± 170, 7,600 ± 140, 8,370 ± 250, and 9,860 ± 180 years.
The next prominent moraine as you move inward corresponds to the same ridges previously dated by Sagredo et al. (2017) (Previous Studies). A new sample collected from this ridge yielded an age of 6,090 ± 120 years, which is statistically indistinguishable from the existing ages. Our new date, combined with the ages in Sagredo et al. (2017) results in a mean age of 5,750 ± 220 years.
Immediately inward, we found a 5–10 m in high, sharp-crested moraine ridge that can be traced continuously for almost 1 km. We collected four samples from this ridge. Three of them yielded coherent ages of 3,540 ± 70, 3,730 ± 80 and 3,740 ± 80 years (mean of 3,670 ± 130 years). The fourth sample (RTV16-01–04) affords an age of 4,390 ± 80 years, which is excluded from the moraine mean age because it does not overlap with the three ages at 2σ.
In the upper section of the right lateral system (Figures 3, 4), the ∼3,700 years ridge has been destroyed by a glacier advance that deposited a small (2-m high) ridge that can be traced to the proximity of the present glacier margin. Three samples collected from boulders atop this ∼2-m high ridge provide ages of 1,370 ± 50, 1,460 ± 30 and 1,480 ± 30 years, with a mean age of 1,440 ± 60 years.
Finally, the innermost RT6 moraines correspond to a very sharp ridge (Figure 2) with a steep postglacially modified proximal slope that reaches the bottom of the valley. Four samples collected on this moraine yield ages ranging from 430 ± 20 to 670 ± 20 years with a mean age of 530 ± 60 years.
Left Lateral Moraines
We did not find any boulders from which to collect suitable samples for surface exposure dating on the two outermost ridges of the moraine suite on the left side of the valley. The outermost moraine dated from this area corresponds to the third-outermost crest, which rises ∼10 m above the surrounding topography and is intermittently continuous over about 1 km. A single boulder on this ridge yielded an age of 4,290 ± 100 years.
Immediately inward, we collected three samples from a continuous ∼1 km long sharp ridge, which provided ages of 3,240 ± 90, 3,320 ± 70 and 3,360 ± 60 years (mean: 3,310 ± 110 years). Finally, three samples from the steep innermost ridge provided statistically indistinguishable ages of 380 ± 20, 380 ± 20 and 400 ± 30 years (mean: 390 ± 10 years).
Discussion
The glacier chronology at Río Tranquilo shows a clear pattern of multiple progressively less-extensive advances through the Holocene. We found evidence that the Tranquilo glacier reached its maximum extent sometime - or possibly at multiple occasions - during the first half of the Holocene between 9,860 ± 180 and 6,730 ± 130 years (n = 4). Our data suggest that during this Holocene glacier maximum, the Río Tranquilo ice lobe was 30–40% smaller (less extensive) than during the ACR accounting for slight uncertainties in frontal positions. Next, Tranquilo glacier readvanced around 5,750 ± 220 years (n = 4) and 3,490 ± 140 years (n = 6). The later advance is recorded and dated on both sides of the valley by moraines with statistically indistinguishable ages of 3,670 ± 130 (right lateral) and 3,310 ± 110 years (left lateral). A single date from a left lateral ridge outside this landform suggests that Tranquilo glacier may have experienced another glacier advance in between the two advances described above, at around 4,290 ± 100 years; notably, this age is statistically indistinguishable from RTV16-01-04 (4,390 ± 80 years), which was considered an outlier specifically on the ∼3,700 years moraine (right lateral side of the valley). The next advance recorded in the area is dated to 1,440 ± 60 years (n = 3). Over the last millennium, we infer that the Tranquilo glacier advanced and lingered in an extended position between 670 ± 20 and 430 ± 20 years (n = 4) and underwent a final major advance ∼390 ± 10 years (n = 3). After, the Tranquilo glacier retreated towards the headwall, depositing the minor ridges in the process. Continuing retreat ultimately separated the Río Tranquilo glacier into smaller ice bodies, as observed at present.
The Río Tranquilo valley provides a comprehensive chronology of the glacier history of Mt. San Lorenzo and the adjacent areas. Despite methodological differences, the new 10Be chronology of Holocene glacier advances from Tranquilo valley is consistent with published chronologies (Figure 1) from the Río Lácteo (Mercer 1968; Garibotti and Villalba 2017: radiocarbon dating and lichenometry, respectively) and San Lorenzo valleys (Garibotti and Villalba 2017: lichenometry). The Tranquilo chronology encompasses most of the events recorded in these adjacent valleys, except for the glacier advances constrained by a minimum date of 2,470–2,180 years and another during the last 200 years. Additionally, the Tranquilo chronology captures an early Holocene glacier advance not recorded in these other valleys. We speculate that these differences may reflect the strengths and limitations of different dating approaches (cf., cosmogenic dating compared with lichenometry). However, immediately to the south of Mt. San Lorenzo, Wenzens 2005) identified at least three moraine ridges and based on 14C minimum-limiting ages from kettles he inferred they were deposited sometime between 9,620 ± 120 and 7,500 ± 80 cal year BP. It is unclear if the southern glaciers of Mt. San Lorenzo were tributaries of the lobe that deposited those moraines, but interestingly the inferred timeframe for their deposition overlaps with the possible deposition (n = 2 10Be ages) of the outermost moraine in Río Tranquilo valley.
At a regional level, there are remarkable similarities between the Holocene record at Mt. San Lorenzo and other 10Be (and 14C)-based chronologies to the south (Figure 5). Records from southwestern Patagonia show evidence for early Holocene advances that coincide with the range of ages captured by the outermost RT6 moraine. At Lago Argentino (49.8°–50.7°S), based on stratigraphic evidence, Strelin et al. (2014) 14C dated a glacier advance between 7,740 and 7,220 cal year BP in the Agassiz Este valley, which was less extensive than the subsequent maximum Holocene limits after 7 ka; at Torre valley (49.3°S), Reynhout et al. (2019) dated two outermost limits to 9,620 ± 340 and 6,790 ± 210 years. Although the Tranquilo record does not allow us to pinpoint the exact timing of the early Holocene events, we suggest that, collectively, these three sites offer mounting evidence to suggest that expanded glaciers were commonplace in Patagonia during the first half of the epoch. At Tranquilo and the Glacier Torre sites, the early to mid-Holocene expansions represent the Holocene glacier maximum. At Lago Argentino, on the other hand, the maximum Holocene frontal (cf., lateral) limits were reached after 7 ka and by 6 ka.
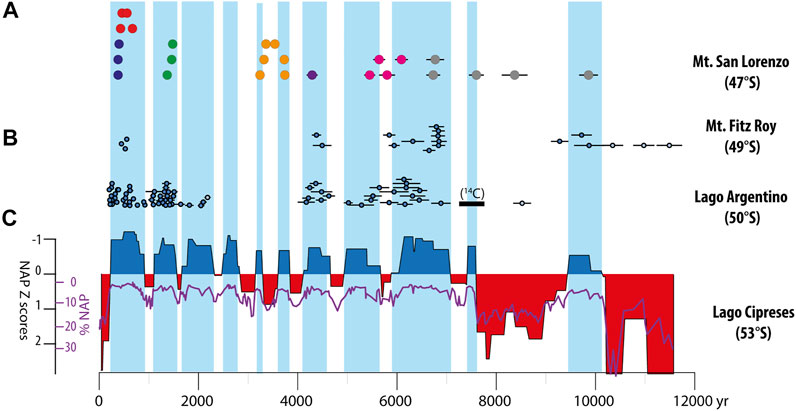
FIGURE 5. Holocene glacier 10Be chronologies from Patagonia. (A) Individual ages (±1σ) on moraine ridges in the Río Tranquillo valley; circles with the same color correspond to samples on the same moraine ridge, including correlated crests between right and left laterals on either side of the valley (same color as in Figures 3,4). For samples <4,000 years, the error is smaller than the symbol. Events are found between 9,860 ± 180 and 6,730 ± 130, at 5,750 ± 220, 4,290 ± 100 (?), 3,490 ± 140, 1,440 ± 60, between 670 ± 20 and 430 ± 20 and finally at 390 ± 10 years (B) Individual ages (±1σ) on moraine ridges in the Torre valley (Reynhout et al., 2019) and Lago Argentino basin (Strelin et al., 2014; Kaplan et al., 2016). Also, we show a glacier advance between 7.7 and 7.2 ka, detected with 14C dating of wood in a stratigraphic section (Strelin et al., 2014) but not with 10Be ages for the moraine record. (C) Cold and wet (blue) and warm and dry periods (red) as shown by regime shift detection analysis of non-arboreal pollen (NAP) record from Lago Cipreses at 51°S (Moreno et al., 2018). Negative (positive) values correspond to periods of strong (weak) and northward (southward) displaced SWW. We also included the percent of NAP (purple line). To facilitate comparisons with the glacier records, we included blue vertical shading representing the cold and wet periods.
During the middle Holocene, the Tranquilo record features glacier expansions at 5,750 ± 220, 3,490 ± 140 and 1,440 ± 60 years; within uncertainties, these events may be consistent with an advance at nearby Lago Colonia (47.3°S) at 5,055 ± 190 years (Nimick et al., 2016), and they are statistically indistinguishable from those at Lago Argentino at 5,970 ± 110 and 1,380 ± 50 years (Kaplan et al., 2016), and at Torre valley at 6,030 ± 230 years (Reynhout et al., 2019). Moreover, at Lago Argentino, three 10Be ages of 5,500 ± 200 (n = 2) and 5,700 ± 200 years on different crests within the maximum ∼6 ka Holocene limits (e.g., at Frias valley) overlap with ages in the Tranquilo record. An additional glacier advance, preliminary dated at ∼4,300 years at Tranquilo valley, is consistent with the 4,440 ± 180 years advance at Lago Argentino and the 4,440 ± 140 years advance at Torre valley.
On the other hand, Lago Argentino also preserves advances at ∼2,100–2000 and ∼1,200–1,000 years that are not present in the chronology at Tranquilo valley, although undated moraine fragments in our study area may reflect these events. We also note that the Río Tranquilo record contains a prominent glacier advance at 3,490 ± 140 years (n = 6) not found in the chronologies at either Lago Argentino or Glacier Torre; however, evidence of this advance has been inferred elsewhere in Southern Patagonia (Wenzens 1999) and Tierra del Fuego (Kuylenstierna et al., 1996; Strelin et al., 2008; Hall et al., 2019). Future efforts will help define better similarities and potential differences between the glacier histories of central and Southern Patagonia.
Finally, during the last millennium, Tranquilo glacier underwent advances between 670 ± 20 and 430 ± 20 years, and at 390 ± 10 years. Statistically indistinguishable 10Be-dated glacier advances have been documented in other sites in Patagonia: Lago Argentino basin (630–480 and 350 ± 20 years BP; Kaplan et al., 2016), Torre valley (510 ± 40 years; Reynhout et al., 2019), and Torres del Paine (51.2°S) (<600 ± 70 years BP and 340 ± 20 years BP; García et al., 2020). We acknowledge that younger glacier advances have been also documented at Lago Argentino (240 ± 10 years) and Torres del Paine (<190 years); we presume that these events correspond to the undated and poorly preserved ridges/drift inward of RT6 (Figure 2). Overall, we posit that our findings at Rio Tranquilo are strongly consistent with the onset and timing of expanded glaciers on the southern side of the SPI over the last 1,000 years.
The chronology of glacier fluctuations at the Río Tranquilo valley correlates well with changes in the strength of the SWW, as reconstructed in the pollen record at Lago Cipreses (51°S), in southern Patagonia (Figure 5) (Moreno et al., 2018). Thus, glacier advances at Río Tranquilo occurred during inferred periods of SWW maximum strength, which are associated with negative anomalies of temperature and positive anomalies of precipitations throughout western Patagonia (Moreno et al., 2018). The lower latitudinal position of Tranquilo relative to other sites demands that westerlies must have been stronger across the entirety of southern Patagonia reaching towards the central sector. We suggest that even during the early Holocene, relatively brief northward excursions of the SWW from their modern core at 52°S could have interrupted the overall warm conditions that characterized this time slice (Moreno and León 2003; Moreno et al., 2018), triggering what were likely the most extensive alpine glacier advances of the epoch.
Thus, our findings at 47°S support the hypothesis that Holocene glacier variability was modulated by shifts in intensity and location of the westerly winds throughout Patagonia. To drive glacier advances, these changes in SWW strength must have persisted over at least the decadal/centennial time scale. Moreno et al. (2018) proposed that centennial-scale climate variability, resembling that of the Southern Annular mode (SAM) (Moreno et al., 2018), the main mode of atmospheric variability in the mid- and high-latitudes of the Southern Hemisphere (Marshall 2003), could result in changes in mean SWW circulation over Patagonia. Therefore, we posit that centennial-scale paleo-SAM-like variations provide a mechanism by which SWW circulation excursions could be sustained for long enough to drive glacier advances over the Holocene in central Patagonia.
We infer that the Río Tranquilo record captures much of the centennial/millennial Holocene glacier variability observed throughout Patagonia. It captures glacier advances coeval with those recorded by both small mountain glaciers and major outlet glaciers of the larger Southern Patagonian Icefield. The new comprehensive Holocene chronology confirms the idea that Patagonia exhibited progressively less extensive glacier advances throughout most of the Holocene (Reynhout et al., 2019). In addition, the maximum Holocene glacier extent in southern South America likely occurred sometime during the early part of the epoch. Such patterns appear robust generally south of (at least) 47°S, the location of San Lorenzo, although recently published glacier chronologies from Cordillera Darwin (55°S) suggests that the scenario may be different in the southernmost tip of the continent (e.g., Menouno et al., 2013; Hall et al., 2019; Reynhout et al., 2021).
Finally, the observed high-resolution pattern of multiple moraine building events with slightly decreasing net extent throughout the early, mid- and late Holocene, appears to be one of the most distinctive signatures of glacier behavior in the southern Hemisphere. This pattern has not only been recognized in the southern mid-latitudes including New Zealand (e.g., Putnam et al., 2012; Kaplan et al., 2013; Dowling et al., 2021), but also around the Antarctic Peninsula, at 64°S (Kaplan et al., 2020). Recently, Bakke et al. (2021) documented a trend of gradually smaller glaciers traceable back to the ACR on the island of South Georgia (54.2°S). Collectively, the pattern of generally smaller expansions in the southern latitudes contrasts with the general pattern observed in the Northern Hemisphere, where glaciers reached their maximum extent in the late Holocene (e.g., Grove, 2004; Holzhauser et al., 2005; Schimmelpfennig et al., 2014, 2021; Luckman et al., 2017; Wittmeier et al., 2020; Braumann et al., 2020, 2021). This long-term, asymmetrical Holocene glacier pattern between Hemispheres seems at odds with the global glacier response during the last decades, when anthropogenic warming has overwhelmed the natural forcing (IPCC, 2021) and appears to be driving inter-hemispherically synchronized and accelerating glacier retreat.
Data Availability Statement
The original contributions presented in the study are included in the article; further inquiries can be directed to the corresponding author.
Author Contributions
ES, SR, JA, MK, and JS contributed to conception and design of the study, and analysis of the results. ES, SR, JA, PA, MK, and BL conducted field mapping and sample collection. SR, PA, and RS conducted laboratory work. ES, MK, and SR wrote the first draft of the manuscript. All authors contributed to manuscript revision, read, and approved the submitted version.
Funding
This project was funded by the ANID Millennium Science Initiative/Millennium Nucleus Paleoclimate NCN17_079; FONDECYT 1180717, and NSF EAR-1804816 (MK and JS). We also acknowledge support from the Fulbright Visiting Scholar Program (MK) and the LDEO and NASA GISS Climate Center (MK). SR was a recipient of a CONICYT-PCHA Beca de Doctorado Nacional (year 2015).
Conflict of Interest
The authors declare that the research was conducted in the absence of any commercial or financial relationships that could be construed as a potential conflict of interest.
Publisher’s Note
All claims expressed in this article are solely those of the authors and do not necessarily represent those of their affiliated organizations, or those of the publisher, the editors and the reviewers. Any product that may be evaluated in this article, or claim that may be made by its manufacturer, is not guaranteed or endorsed by the publisher.
Acknowledgments
We thank J. Araos, I. González, F. González, J. Koch, R. Orrego, L. Soto, Rodrigo Soteres, and L. Gómez, for providing field assistance. JS also acknowledges support by the NSF (award #1853881) and the Vetlesen Foundation.
References
Aniya, M. (1995). Holocene Glacial Chronology in Patagonia: Tyndall and Upsala Glaciers. Arctic Alpine Res. 27 (4), 311–322. doi:10.2307/1552024
Aniya, M. (2013). Holocene Glaciations of Hielo Patag^|^oacute;nico (Patagonia Icefield), South America: A Brief Review. Geochem. J. 47 (2), 97–105. doi:10.2343/GEOCHEMJ.1.0171
Aravena, J. C. (2007). Reconstructing Climate Variability Using Tree Rings and Glacier Fluctuations in the Southern Chilean Andes, PhD Thesis. Ontario: University of Western Western Ontario, 236.
Bakke, J., Paasche, Ø., Schaefer, J. M., and Timmermann, A. (2021). Long-term Demise of Sub-antarctic Glaciers Modulated by the Southern Hemisphere Westerlies. Sci. Rep. 11 (1), 1–10. doi:10.1038/s41598-021-87317-5
Balco, G., Stone, J. O., Lifton, N. A., and Dunai, T. J. (2008). A Complete and Easily Accessible Means of Calculating Surface Exposure Ages or Erosion Rates from 10Be and 26Al Measurements. Quat. Geochronol. 3, 174–195. doi:10.1016/j.quageo.2007.12.001
Bond, G., Kromer, B., Beer, J., Muscheler, R., Evans, M. N., Showers, W., et al. (2001). Persistent Solar Influence on North Atlantic Climate during the Holocene. Science 294, 2130–2136. doi:10.1126/science.1065680
Braumann, S. M., Schaefer, J. M., Neuhuber, S. M., Lüthgens, C., Hidy, A. J., and Fiebig, M. (2021). Early Holocene Cold Snaps and Their Expression in the Moraine Record of the Eastern European Alps. Clim. Past 17, 2451–2479. doi:10.5194/cp-17-2451-2021
Braumann, S. M., Schaefer, J. M., Neuhuber, S. M., Reitner, J. M., Lüthgens, C., and Fiebig, M. (2020). Holocene Glacier Change in the Silvretta Massif (Austrian Alps) Constrained by a New 10Be Chronology, Historical Records and Modern Observations. Quat. Sci. Rev. 245, 106493. doi:10.1016/j.quascirev.2020.106493
Bronk Ramsey, C. (2009). Bayesian Analysis of Radiocarbon Dates. Radiocarbon 51 (1), 337–360. doi:10.1017/S0033822200033865
Clapperton, C., and Sugden, D. (1988). Holocene Glacier Fluctuations in South America and Antarctica. Quat. Sci. Rev. 7, 185–198. doi:10.1016/0277-3791(88)90005-4
Davies, B. J., Thorndycraft, V. R., Fabel, D., and Martin, J. R. V. (2018). Asynchronous Glacier Dynamics during the Antarctic Cold Reversal in central Patagonia. Quat. Sci. Rev. 200, 287–312. doi:10.1016/j.quascirev.2018.09.025
Denton, G. H., and Broecker, W. S. (2008). Wobbly Ocean Conveyor Circulation during the Holocene? Quat. Sci. Rev. 27, 1939–1950. doi:10.1016/j.quascirev.2008.08.008
Denton, G. H., and Karlén, W. (1973). Holocene Climatic Variations-Their Pattern and Possible Cause. Quat. Res. 3, 155–205. doi:10.1016/0033-5894(73)90040-9
Dowling, L., Eaves, S., Norton, K., Mackintosh, A., Anderson, B., Hidy, A., et al. (2021). Local Summer Insolation and Greenhouse Gas Forcing Drove Warming and Glacier Retreat in New Zealand during the Holocene. Quat. Sci. Rev. 266, 107068. doi:10.1016/j.quascirev.2021.107068
Falaschi, D., Bravo, C., Masiokas, M., Villalba, R., and Rivera, A. (2013). First Glacier Inventory and Recent Changes in Glacier Area in the Monte San Lorenzo Region (47°S), Southern Patagonian Andes, South America. Arctic, Antarctic, Alpine Res. 45 (1), 19–28. doi:10.1657/1938-4246-45.1.19
Falaschi, D., Lenzano, M. G., Villalba, R., Bolch, T., Rivera, A., and Lo Vecchio, A. (2019). Six Decades (1958-2018) of Geodetic Glacier Mass Balance in Monte San Lorenzo, Patagonian Andes. Front. Earth Sci. 7, 326. doi:10.3389/feart.2019.00326
García, J.-L., Hall, B. L., Kaplan, M. R., Gómez, G. A., De Pol-Holz, R., García, V. J., et al. (2020). 14C and 10Be dated Late Holocene fluctuations of Patagonian glaciers in Torres del Paine (Chile, 51°S) and connections to Antarctic climate change. Quat. Sci. Rev. 246, 106541. doi:10.1016/j.quascirev.2020.106541
Garibotti, I. A., and Villalba, R. (2017). Colonization of Mid- and Late-Holocene Moraines by Lichens and Trees in the Magellanic Sub-antarctic Province. Polar Biol. 40 (9), 1739–1753. doi:10.1007/s00300-017-2096-1
Garreaud, R., Lopez, P., Minvielle, M., and Rojas, M. (2013). Large-scale Control on the Patagonian Climate. J. Clim. 26 (1), 215–230. doi:10.1175/JCLI-D-12-00001.1
Glasser, N. F., Harrison, S., Schnabel, C., Fabel, D., and Jansson, K. N. (2012). Younger Dryas and Early Holocene Age Glacier Advances in Patagonia. Quat. Sci. Rev. 58, 7–17. doi:10.1016/j.quascirev.2012.10.011
Glasser, N. F., Jansson, K. N., Harrison, S., and Rivera, A. (2005). Geomorphological Evidence for Variations of the North Patagonian Icefield during the Holocene. Geomorphology 71 (3-4), 263–277. doi:10.1016/j.geomorph.2005.02.003
Grove, J. M. (1988). Little Ice Ages: Ancient and Modern. 2nd ed. Oxfordshire, England, UK: Routledge. doi:10.4324/9780203505205
Hall, B. L., Lowell, T. V., Bromley, G. R. M., Denton, G. H., and Putnam, A. E. (2019). Holocene Glacier Fluctuations on the Northern Flank of Cordillera Darwin, Southernmost South America. Quat. Sci. Rev. 222, 105904. doi:10.1016/j.quascirev.2019.105904
Hogg, A. G., Heaton, T. J., Hua, Q., Palmer, J. G., Turney, C. S., Southon, J., et al. (2020). SHCal20 Southern Hemisphere Calibration, 0-55,000 Years Cal BP. Radiocarbon 62 (4), 759–778. doi:10.1017/RDC.2020.59
Holzhauser, H., Magny, M., and Zumbuühl, H. J. (2005). Glacier and lake-level Variations in West-central Europe over the Last 3500 Years. The Holocene 15 (6), 789–801. doi:10.1191/0959683605hl853ra
Horta, L. R., Georgieff, S. M., Aschero, C. A., and Goñi, R. A. (2017). Paleolacustrine Records from Late Pleistocene - Holocene in the Perito Moreno National Park, Argentinian Patagonian Andes. Quat. Int. 436, 8–15. doi:10.1016/j.quaint.2017.01.001
Kaplan, M. R., Schaefer, J. M., Denton, G. H., Doughty, A. M., Barrell, D. J. A., Chinn, T. J. H., et al. (2013). The Anatomy of Long-Term Warming since 15 Ka in New Zealand Based on Net Glacier Snowline Rise. Geology 41 (8), 887–890. doi:10.1130/G34288.1
Kaplan, M. R., Schaefer, J. M., Strelin, J. A., Denton, G. H., Anderson, R. F., Vandergoes, M. J., et al. (2016). Patagonian and Southern South Atlantic View of Holocene Climate. Quat. Sci. Rev. 141, 112–125. doi:10.1016/j.quascirev.2016.03.014
Kaplan, M. R., Strelin, J. A., Schaefer, J. M., Denton, G. H., Finkel, R. C., Schwartz, R., et al. (2011). In-situ Cosmogenic 10Be Production Rate at Lago Argentino, Patagonia: Implications for Late-Glacial Climate Chronology. Earth Planet. Sci. Lett. 309, 21–32. doi:10.1016/j.epsl.2011.06.018
Kaplan, M. R., Strelin, J. A., Schaefer, J. M., Peltier, C., Martini, M. A., Flores, E., et al. (2020). Holocene Glacier Behavior Around the Northern Antarctic Peninsula and Possible Causes. Earth Planet. Sci. Lett. 534, 116077. doi:10.1016/j.epsl.2020.116077
Kelly, M. A. (2003).The Late Wurmian Age in the Western Swiss Alps - Last Glacial Maximum (LGM) Ice-Surface Reconstruction and 10Be Dating of Late-Glacial Features. Bern, Switzerland: University of Bern, 105. PhD dissertation.
Kuylenstierna, J. L., Rosqvist, G. C., and Holmlund, P. (1996). Late-Holocene glacier variations in the Cordillera Darwin, Tierra del Fuego, Chile. The Holocene 6 (3), 353–358. doi:10.1177/095968369600600310
Lal, D. (1991). Cosmic ray Labeling of Erosion Surfaces: In Situ Nuclide Production Rates and Erosion Models. Earth Planet. Sci. Lett. 104, 424–439. doi:10.1016/0012-821X(91)90220-C
Lifton, N., Sato, T., and Dunai, T. (2014). Scaling In Situ Cosmogenic Nuclide Production Rates Using Analytical Approximations to Atmospheric Cosmic-Ray Fluxes. Earth Planet. Sci. Lett. 386, 149–160. doi:10.1016/j.epsl.2013.10.052
Luckman, B. H., Masiokas, M. H., and Nicolussi, K. (2017). Neoglacial History of Robson Glacier, British Columbia. Can. J. Earth Sci. 54 (11), 1153–1164. doi:10.1139/cjes-2016-0187
Marshall, G. J. (2003). Trends in the Southern Annular Mode from Observations and Reanalyses. J. Clim. 16 (24), 4134–4143. doi:10.1175/1520-0442(2003)016<4134:titsam>2.0.co;2
Martin, J. R. V., Davies, B. J., and Thorndycraft, V. R. (2019). Glacier Dynamics during a Phase of Late Quaternary Warming in Patagonia Reconstructed from Sediment-Landform Associations. Geomorphology 337, 111–133. doi:10.1016/j.geomorph.2019.03.007
Mendelová, M., Hein, A. S., Rodés, Á., Smedley, R. K., and Xu, S. (2020a). Glacier Expansion in central Patagonia during the Antarctic Cold Reversal Followed by Retreat and Stabilisation during the Younger Dryas. Quat. Sci. Rev. 227, 106047. doi:10.1016/j.quascirev.2019.106047I
Mendelová, M., Hein, A. S., Rodés, Á., and Xu, S. (2020b). Extensive Mountain Glaciation in central Patagonia during Marine Isotope Stage 5. Quat. Sci. Rev. 227, 105996. doi:10.1016/j.quascirev.2019.105996
Menounos, B., Clague, J. J., Osborn, G., Davis, P. T., Ponce, F., Goehring, B., et al. (2013). Latest Pleistocene and Holocene glacier fluctuations in southernmost Tierra del Fuego, Argentina. Quat. Sci. Rev. 77, 70–79. doi:10.1016/j.quascirev.2013.07.008
Mercer, J. H. (1968). Variations of Some Patagonian Glaciers since the Late-Glacial. Am. J. Sci. 266, 91–109. doi:10.2475/ajs.266.2.91
Mercer, M. (1982). Transition Metal Chlorofluorides. J. Fluorine Chem. 21, 35–40. doi:10.1016/s0022-1139(00)85381-6
Moreno, P. I., and León, A. L. (2003). Abrupt Vegetation Changes during the Last Glacial to Holocene Transition in Mid-latitude South America. J. Quat. Sci. 18, 787–800. doi:10.1002/jqs.801
Moreno, P. I., Vilanova, I., Villa-Martínez, R., Dunbar, R. B., Mucciarone, D. A., Kaplan, M. R., et al. (2018). Onset and Evolution of Southern Annular Mode-like Changes at Centennial Timescale. Sci. Rep. 8 (1), 3458. doi:10.1038/s41598-018-21836-6
Nimick, D. A., McGrath, D., Mahan, S. A., Friesen, B. A., and Leidich, J. (2016). Latest Pleistocene and Holocene Glacial Events in the Colonia valley, Northern Patagonia Icefield, Southern Chile. J. Quat. Sci. 31 (6), 551–564. doi:10.1002/jqs.2847
Nishiizumi, K., Imamura, M., Caffee, M. W., Southon, J. R., Finkel, R. C., and McAninch, J. (2007). Absolute Calibration of 10Be AMS Standards. Nucl. Instr. Methods Phys. Res. Section B: Beam Interactions Mater. Atoms 258, 403–413. doi:10.1016/j.nimb.2007.01.297
Peltier, C., Kaplan, M. R., Birkel, S. D., Soteres, R. L., Sagredo, E. A., Aravena, J. C., et al. (2021). The Large MIS 4 and Long MIS 2 Glacier Maxima on the Southern Tip of South America. Quat. Sci. Rev. 262, 106858. doi:10.1016/j.quascirev.2021.106858
Porter, S. C., and Denton, G. H. (1967). Chronology of Neoglaciation in the North American Cordillera. Am. J. Sci. 265, 177–210. doi:10.2475/ajs.265.3.177
Putnam, A. E., Schaefer, J. M., Denton, G. H., Barrell, D. J. A., Finkel, R. C., Andersen, B. G., et al. (2012). Regional Climate Control of Glaciers in New Zealand and Europe during the Pre-industrial Holocene. Nat. Geosci 5 (9), 627–630. doi:10.1038/ngeo1548
Reynhout, S. A., Kaplan, M. R., Sagredo, E. A., Aravena, J. C., Soteres, R. L., Schwartz, R., et al. (2021). Holocene Glacier History of Northeastern Cordillera Darwin, Southernmost South America (55°S). Quat. Res., 1–16. doi:10.1017/qua.2021.45
Reynhout, S. A., Sagredo, E. A., Kaplan, M. R., Aravena, J. C., Martini, M. A., Moreno, P. I., et al. (2019). Holocene Glacier Fluctuations in Patagonia Are Modulated by Summer Insolation Intensity and Paced by Southern Annular Mode-like Variability. Quat. Sci. Rev. 220, 178–187. doi:10.1016/j.quascirev.2019.05.029
Sagredo, E. A., Kaplan, M. R., Araya, P. S., Lowell, T. V., Aravena, J. C., Moreno, P. I., et al. (2018). Trans-pacific Glacial Response to the Antarctic Cold Reversal in the Southern Mid-latitudes. Quat. Sci. Rev. 188, 160–166. doi:10.1016/j.quascirev.2018.01.011
Sagredo, E. A., Lowell, T. V., Kelly, M. A., Rupper, S., Aravena, J. C., Ward, D. J., et al. (2017). Equilibrium Line Altitudes along the Andes during the Last Millennium: Paleoclimatic Implications. The Holocene 27 (7), 1019–1033. doi:10.1177/0959683616678458
Schaefer, J. M., Denton, G. H., Kaplan, M., Putnam, A., Finkel, R. C., Barrell, D. J. A., et al. (2009). High-Frequency Holocene Glacier Fluctuations in New Zealand Differ from the Northern Signature. Science 324, 622–625. doi:10.1126/science.1169312
Schimmelpfennig, I., Schaefer, J., Lamp, J., Godard, V., Schwartz, R., Bard, E., et al. (2021). Glacier Response to Holocene Warmth Inferred from In Situ 10Be and 14C Bedrock Analyses in Steingletscher's Forefield (central Swiss Alps). Clim. Past Discuss., 1–27. doi:10.5194/cp-2021-110
Schimmelpfennig, I., Schaefer, J. M., Akçar, N., Koffman, T., Ivy-Ochs, S., Schwartz, R., et al. (2014). A Chronology of Holocene and Little Ice Age Glacier Culminations of the Steingletscher, Central Alps, Switzerland, Based on High-Sensitivity Beryllium-10 Moraine Dating. Earth Planet. Sci. Lett. 393, 220–230. doi:10.1016/j.epsl.2014.02.046
Stone, J. O. (2000). Air Pressure and Cosmogenic Isotope Production. J. Geophys. Res. 105, 23753–23759. doi:10.1029/2000JB900181
Strelin, J. A., Denton, G. H., Vandergoes, M. J., Ninnemann, U. S., and Putnam, A. E. (2011). Radiocarbon Chronology of the Late-Glacial Puerto Bandera Moraines, Southern Patagonian Icefield, Argentina. Quat. Sci. Rev. 30, 2551–2569. doi:10.1016/j.quascirev.2011.05.004
Strelin, J. A., Kaplan, M. R., Vandergoes, M. J., Denton, G. H., and Schaefer, J. M. (2014). Holocene Glacier History of the Lago Argentino basin, Southern Patagonian Icefield. Quat. Sci. Rev. 101, 124–145. doi:10.1016/j.quascirev.2011.05.00410.1016/j.quascirev.2014.06.026
Strelin, J., Casassa, G., Rosqvist, G., and Holmlund, P. (2008). Holocene glaciations in the Ema Glacier valley, Monte Sarmiento Massif, Tierra del Fuego. Palaeogeogr. Palaeoclimatol. Palaeoecol. 260, 299–314. doi:10.1016/j.palaeo.2007.12.002
IPCC (2021). in Climate Change 2021: The Physical Science Basis. Contribution of Working Group I to the Sixth Assessment Report of the Intergovernmental Panel on Climate Change. Editors V. Masson-Delmotte, P. Zhai, A. Pirani, S. L. Connors, C. Péan, S. Bergeret al. (Cambridge UK: Cambridge University Press). In press.
Wenzens, G. (1999). Fluctuations of Outlet and Valley Glaciers in the Southern Andes (Argentina) during the Past 13,000 Years. Quat. Res. 51, 238–247. doi:10.1006/qres.1999.2043
Wenzens, G. (2005). Glacier Advances East of the Southern Andes between the Last Glacial Maximum and 5,000 BP Compared with lake Terraces of the Endorrheic Lago Cardiel (49° S, Patagonia, Argentina). NF: Zeitschrift für Geomorphologie, 433–454. doi:10.1127/zfg/49/2005/433
Keywords: Patagonia, Holocene, glacier fluctuations, 10Be dating, Southern Annular Mode, Neoglaciation, Paleoclimate, South America
Citation: Sagredo EA, Reynhout SA, Kaplan MR, Aravena JC, Araya PS, Luckman BH, Schwartz R and Schaefer JM (2021) Holocene History of Río Tranquilo Glacier, Monte San Lorenzo (47°S), Central Patagonia. Front. Earth Sci. 9:813433. doi: 10.3389/feart.2021.813433
Received: 11 November 2021; Accepted: 07 December 2021;
Published: 22 December 2021.
Edited by:
Daniel Nývlt, Masaryk University, CzechiaReviewed by:
Stephen J. A. Jennings, Masaryk University, CzechiaStephen John Roberts, British Antarctic Survey (BAS), United Kingdom
Copyright © 2021 Sagredo, Reynhout, Kaplan, Aravena, Araya, Luckman, Schwartz and Schaefer. This is an open-access article distributed under the terms of the Creative Commons Attribution License (CC BY). The use, distribution or reproduction in other forums is permitted, provided the original author(s) and the copyright owner(s) are credited and that the original publication in this journal is cited, in accordance with accepted academic practice. No use, distribution or reproduction is permitted which does not comply with these terms.
*Correspondence: Esteban A. Sagredo, ZXNhZ3JlZG9AdWMuY2w=
†These authors share first authorship