- 1Sino-French Institute for Earth System Science, College of Urban and Environmental Sciences, and Laboratory for Earth Surface Processes, Peking University, Beijing, China
- 2Laboratoire des Sciences du Climat et de l’Environnement, LSCE/IPSL, CEA-CNRS-UVSQ, Université Paris-Saclay, Gif-sur-Yvette, France
Peatlands cover about 3% of the Earth’s surface and are regarded as a vital carbon (C) pool and sink. The formation of peatland is supported by continuously supplied nitrogen (N) but the sources of this N remain unclear. Here, we first review N stocks and the rate they accumulate in peatlands, then we present the sources of N, especially through biological nitrogen fixation (BNF). We found that global peatlands store 5.9–25.9 Gt N. In the past millennia, northern peatlands have a lower N accumulated rate than tropical undisturbed peatlands. BNF rate is approximately 1.9 ± 2.7 g m−2 yr−1 in northern peatlands, higher than the rate of N deposition, 0.5 ± 0.4 g m−2 yr−1. For tropical peatlands, BNF observation has hardly been reported yet and needs further investigation. This review provides a broad picture of peatland N cycling and suggests that there are large uncertainties, due to limited observations of BNF and N fluxes by inflow and outflow runoff. Therefore, we call for more efforts contributing to field observations and modelling of the N budget in peatlands.
Introduction
Peatland is a type of wetland ecosystem rich in peat, a type of soil that consists of partially decomposed organic material (Page and Baird, 2016). Although they cover only 3% of the global land surface (Yu et al., 2010), peatlands contain ∼644 Gt C, which is 21% of the global total soil organic carbon (Leifeld and Menichetti, 2018). Moreover, this carbon (C) stock is increasing at a rate of 0.14 Pg C yr−1 (Gallego-Sala et al., 2018), making peatlands an important C sink and potential C source in the context of climate change. Peat formation requires nitrogen (N) supply according to the C/N stoichiometry (C/N ratio) in organic matter (Leifeld and Menichetti, 2018), and uncertainties surrounding N hampers accurate prediction of the land C sink into the future (Wieder et al., 2015). In the past 2 decades, although more and more works related to the N cycle in peatlands at the site/regional level were reported [e.g., León and Oliván (2014); Wang et al. (2015); van Bellen et al. (2020)], the global picture for the sources of N in peatlands remains still unclear.
There are three major ways of N input into a peatland ecosystem, i.e., atmospheric deposition, biological nitrogen fixation (BNF), and N inflow through upland runoff or discharge (Limpens et al., 2006). N deposition, which includes wet and dry deposition, varies across locations. For instance, total N deposition in peatlands ranges from less than 0.2 g N m−2 yr−1 in pristine bogs in Canada (Vile et al., 2014) to more than 4 g N m−2 yr−1 in polluted regions in Europe (Aerts, 1997; Tauchnitz et al., 2010). In many studies, only bulk deposition was reported (Fenn et al., 2003), which captures mainly wet deposition with a small amount of dry deposition (Limpens et al., 2006). Although the flux of dry deposition is comparable to wet deposition (Nadim et al., 2001; Godoy et al., 2003), the dry deposition was often ignored, especially in sedge-dominated fens and wooded bogs, which may cause a large bias in peatland N budgets (Limpens et al., 2006).
BNF in peatlands can be found in free-living cyanobacteria (Granhall and Selander, 1973), quasi-symbiotic cyanobacteria associated with Sphagnum (Patova et al., 2020), methanotrophs (Larmola et al., 2014; Vile et al., 2014), actinorhizal (actinomycete-nodulated) plants (Schwintzer, 1983) and other heterotrophic bacteria (Kox et al., 2018). There are mainly two methods for measuring BNF: direct 15N2 assimilation (15N2 method) (Saiz et al., 2019) and the indirect acetylene (C2H2) reduction assay (ARA) (Hardy et al., 1968). Both methods involve incubation of samples with a certain gas, 15N2 or acetylene, respectively, followed by determination of the 15N signature in the incubated samples through mass spectrometry (15N2 method) or amount of ethylene (C2H4) reduced from acetylene in the headspace through gas chromatography (ARA) (Rousk et al., 2018; van den Elzen et al., 2020). For ARA, a conversion factor is needed to convert the moles of ethylene produced by nitrogenase enzyme activity into moles of N2 fixed. Since the high cost of the 15N2 method prohibits its usage in widespread and repeated measurements, the indirect ARA is the most common method for measuring BNF (Saiz et al., 2019). BNF rates reported in peatlands range from less than 0.1 g N m−2 yr−1 (Waughman and Bellamy, 1980; Urban and Eisenreich, 1988) to more than 2.5 g N m−2 yr−1 (Larmola et al., 2014; Vile et al., 2014).
Nutrient supply through streamflow or groundwater flow may be important for net primary productivity and peat formation of minerotrophic peatlands and some bogs (Urban and Eisenreich, 1988; Limpens et al., 2006), but data are scarce. A watershed of Marcell Bog in Minnesota, United States, received approximately 0.2 g N m−2 yr−1 input through streamflow, 50% of which was retained in the bog (Verry and Timmons, 1982). A slope mire in Germany received 0.9 ± 0.2 g N m−2 yr−1 and discharged 1.9 ± 0.3 g N m−2 yr−1 through streamflow (Tauchnitz et al., 2010), which was much less than the atmospheric deposition (4.9 ± 0.4 g N m−2 yr−1).
Thus, it is important to comb the knowns and unknowns of these three major sources into peatlands, and to understand the N cycle in peatlands. In this study, we first reviewed N stocks and N accumulation rates of global peatlands. Then we explored from literature the external sources of N in peatlands, especially via BNF. Overall, this meta-analysis provides information on N accumulation rates of peatlands and their sources, and summarizes current estimates of the N cycle in peatlands and knowledge gaps.
Methods
N Stock
Hugelius et al. (2020) reported comprehensive estimates for the N stock of northern peatland (north of 30°N) as 10 ± 7 Gt N. Due to the lack of synthesis of N stocks in tropical and southern peatlands [mainly refer to Patagonian peatlands (Yu et al., 2010)], N stocks there were derived by dividing C stocks with the mean values of C/N mass ratio. Table 1 and Table 2 summarize area, C stocks, N stocks and C/N ratios of global peatlands from literature. The C stock of tropical peatlands from Ribeiro et al. (2020) was used here. For the C/N ratio of tropical peat, we compiled a dataset of 160 records (for details of all records, see Supplementary Table S1), which consists of two types of peatlands (defined by their degradation status): natural (labeled as “undisturbed”; n = 71 records), and disturbed (drained or logged, n = 75) peatlands. For undisturbed tropical peat, the oxic, above water-table active layer of peat is approximately 10–30 cm deep (Melling et al., 2005; Girkin et al., 2020). To avoid the overrepresentation of sampling from surface soil, we divided the C/N ratios into two depth groups: surface or near surface peat (“surface peat”, n = 78) and deeper peat (“lower peat”, n = 51). Here, we simply defined surface or near surface as sampling depth less than 25 cm, to match the availability of data (Supplementary Table S1). Furthermore, tropical peatlands could also be classified by two climate types: alpine or montane, and lowland. In southern peatlands, the C stock and C/N ratio (median, in order to eliminate the impact of outliers in surface peat) were estimated from Loisel and Yu (2013a) and Knorr et al. (2015), respectively.
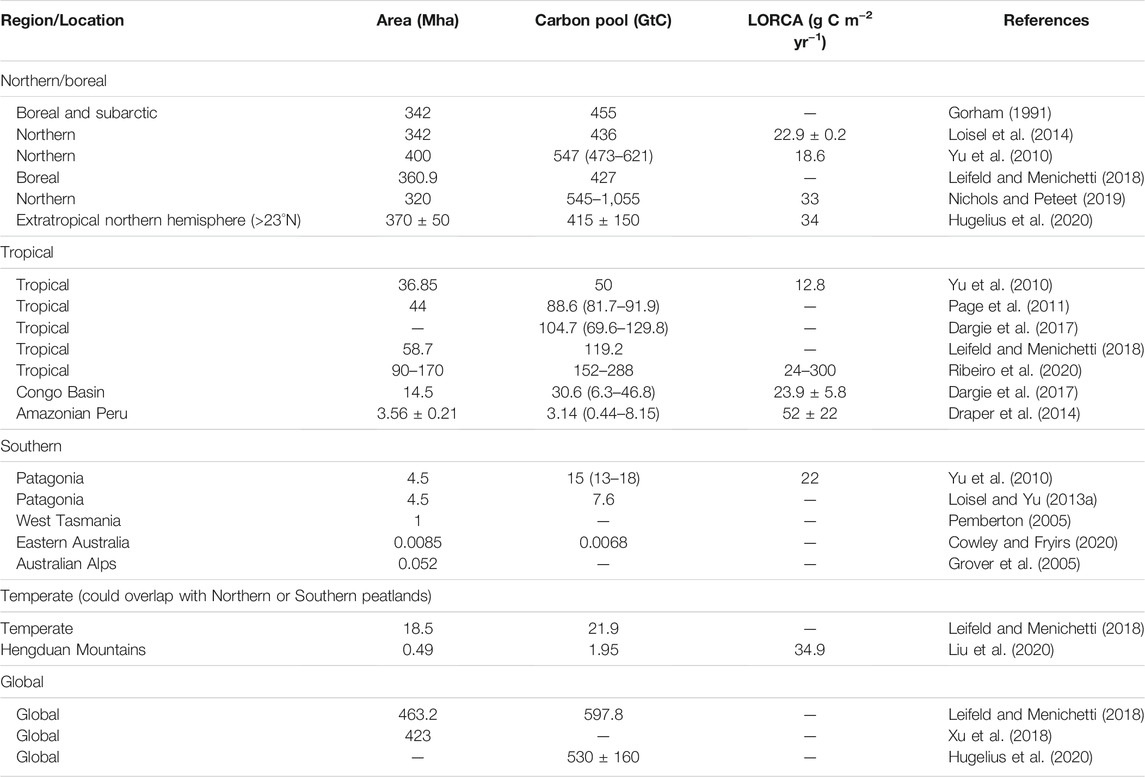
TABLE 1. Area, carbon pool and long-term (apparent) rate of carbon accumulation (LORCA) of global/regional peatlands.
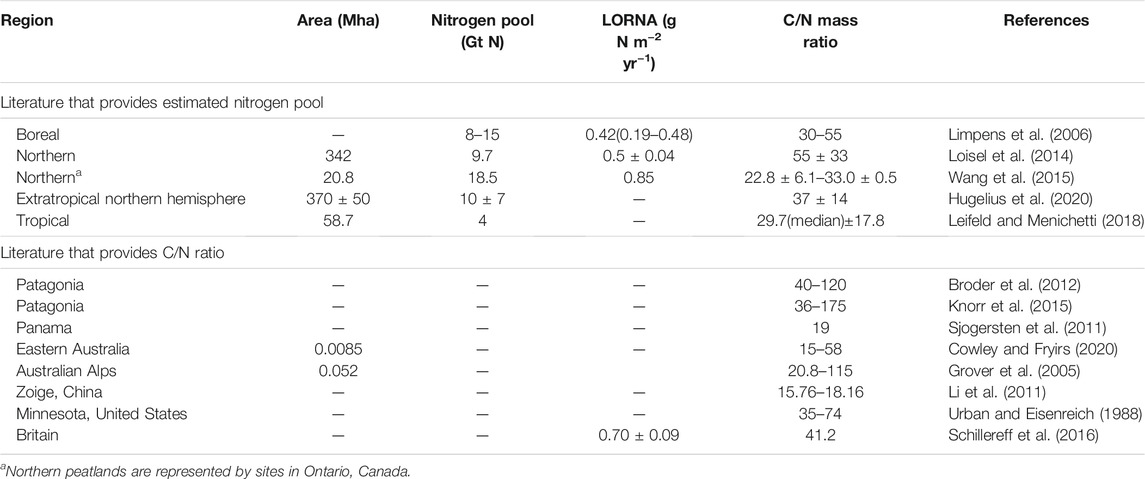
TABLE 2. Nitrogen pool, long-term (apparent) rate of nitrogen accumulation (LORNA) and the mass ratio of carbon/nitrogen of global peatlands.
Rates of N Accumulation
The long-term (apparent) rate of N accumulation (LORNA) was calculated as the cumulative N mass (g m−2) of a peat core divided by the core’s basal age (Tolonen and Turunen, 1996). In boreal peatlands, values of LORNA were collected from literature (Limpens et al., 2006; Loisel et al., 2014; Wang et al., 2015; Schillereff et al., 2016) as shown in Table 2. In tropical and southern peatlands, values of LORNA have seldom been reported, so we estimated them from the previously reported long-term carbon accumulated rate (LORCA) and C/N ratio (Supplementary Table S1). We compiled 26 records of LORCA of tropical peat, distributed in South America (n = 19), Southeast Asia (n = 6) and Africa (n = 1). Note that some records contain more than one peat core (For example, the Congo record in Africa is mean of 61 cores). Basal ages of these tropical peat cores range from >26,000 to 1,975 cal year BP.
Recent (apparent) rate of N accumulation (RERNA) was calculated as the cumulative N mass from the peat surface to a depth corresponding to a given date, divided by its age (Tolonen and Turunen, 1996; León and Oliván, 2014). In this paper, we defined “recent” as younger than 500 years since the oldest RERNA reported in literature was about 400 years old (León and Oliván, 2014). 75 records of northern peatlands were used to investigate the RERNA (Table 3). Specifically, records of RERNA in northern peatlands were divided into two groups: 1) Group A: RERNA calculated from peat core properties with the database provided by Loisel et al. (2014) (records are available via Pangaea). In this case, multiple cores taken from the same peatland were considered as independent records since these cores were not designed as replicates (Loisel et al., 2014). In total, group A consists of 19 records, distributed in Canada (n = 14), United States (n = 1), Sweden (n = 1), Scotland (n = 2) and Russia (n = 1). 2) Group B: data collected from literature other than Loisel et al. (2014) and Li et al. (2018), providing only RERNA but without information of properties of peat cores. Group B consisted of 56 records, distributed in Canada (n = 45), United States (n = 6) and Sweden (n = 5). The data from Li et al. (2018) were discussed in Recent Rate of Nitrogen Accumulation.
Biological Nitrogen Fixation
We collected information about BNF in peatlands from papers and books following the criteria as below:
Rate of BNF must have been measured in peatlands. Peatland was defined as where the surface soil layer (a minimum thickness of 30 cm) consists of at least 65% organic content (Page et al., 2011; Dargie et al., 2017). We believed in the authors’ scientific judgement on the peatlands even without definite peat properties, i.e., once they claimed their study was carried out in peatlands (including statement as mire, fen, bog or swamp), the paper was included for our meta-analysis. However, if the studies were carried out in wetland when the author(s) did not indicate the existence of peat, they were not included in this analysis. In the case where a study contained multiple sites or types of peatlands, each site or type of peatland was considered as an independent record.
In total, we obtained 21 records that reported an annual N2 fixation rate, and 8 records that reported short-term (i.e., daily or hourly) nitrogenase activities in peatlands (Table 4). Significance tests were performed with Student’s t-test. Linear correlation analyses were performed on IBM® SPSS® Statistics 26.
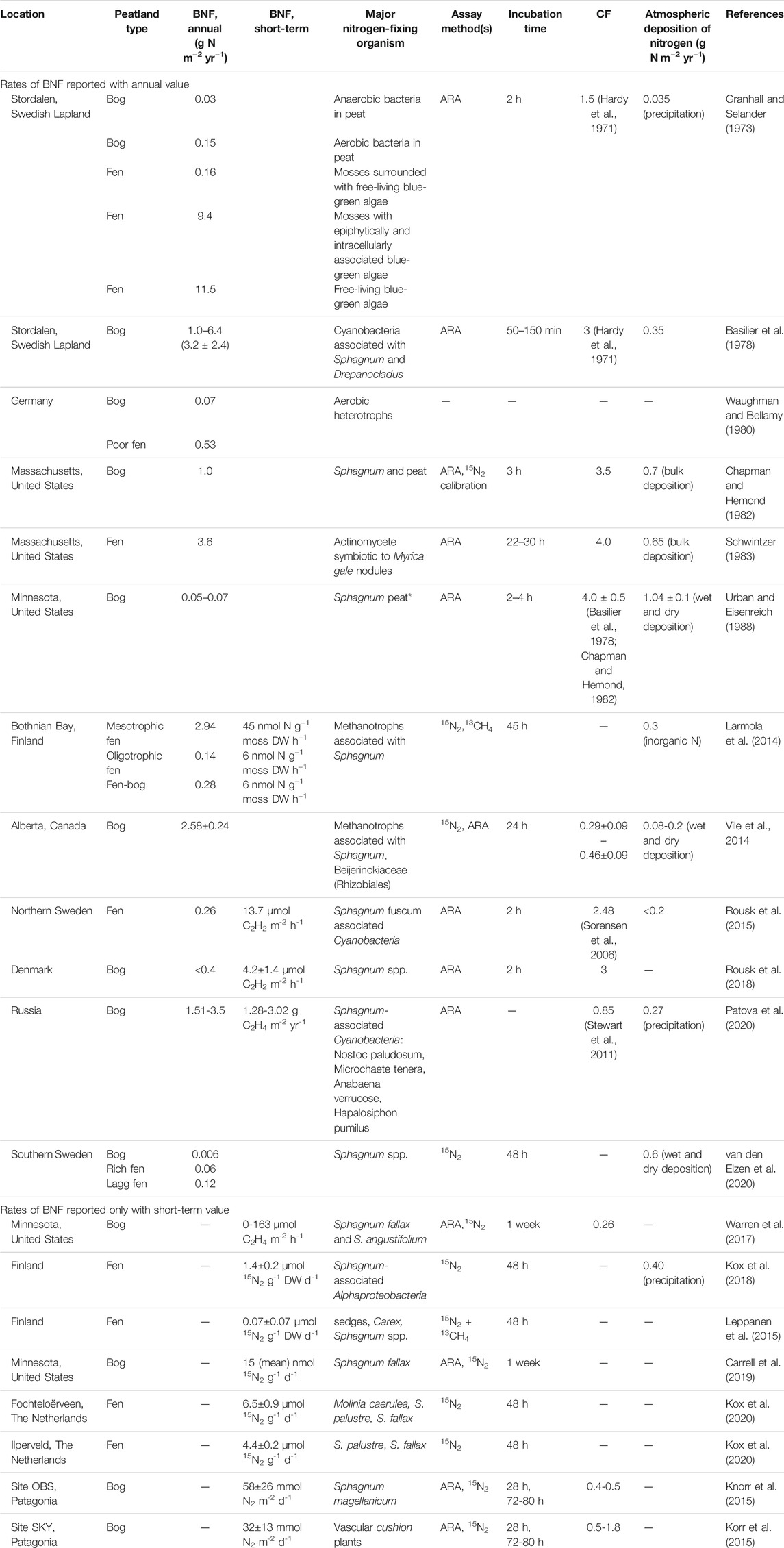
TABLE 4. Rates of biological N2 fixation (BNF) in peatlands. Conversion factor (CF) is the ratio between C2H4 produced using the ARA method and the N fixed using the 15N2 method.
In addition, climate data were derived from database CRU TS v4.04 (Harris et al., 2020). Mean temperature during the growing season and mean annual precipitation were obtained during the period of 1981–2010. The growing season was defined as early June to end of August (Vile et al., 2014; Rousk et al., 2015).
Results and Discussion
C/N Ratio of Tropical Peatland and Nitrogen Stock
Of all the 160 records of C/N ratio in tropical peatlands, 78 records belong to surface peat, 51 records lower peat, and 31 records unknown. The C/N ratio ranges from 10 to 85.6, with a mean of 35.0 ± 18.4 (±standard deviation) for all records. For all the 129 records of known depth, 60 were from natural/undisturbed peatlands and 69 were from disturbed or degraded peatlands. For undisturbed peats, the C/N ratio is 25.9 ± 8.2 for surface peat (n = 31) and 33.0 ± 20.0 for lower peat (n = 29), however, the difference between surface and lower peat is not statistically significant (p > 0.05). For disturbed peats, the C/N ratio of lower peat (57.6 ± 21.6, n = 22) is significantly higher than that of surface peat (35.3 ± 16.0; n = 47) (p < 0.01). The disturbed peatlands tend to have a lower C/N ratio in the surface peat than in the lower peat. This suggests different loss rates of C and N in the disturbed surface layer, the C loss being faster than that of N under degradation of tropical peat. In addition, although the difference between C/N ratios of surface and lower peats in undisturbed tropical peatlands is nonsignificant, they show a contrary trend compared to northern peatlands. In non-permafrost northern peatlands, C/N ratios decline with increasing depth as a result of slow, but continuous, anaerobic decay remobilizing the carbon (Malmer and Holm, 1984; Kuhry and Vitt, 1996; Sannel and Kuhry, 2009; Wang et al., 2014).
For lower peats, C/N ratios show little dependence on the sampling depth (r = -0.21, p = 0.141). This indicates that the C/N ratio in deeper layers is more or less randomly distributed along the core depth despite that the C/N ratio in surface peat, or approximately active layer, is significantly lower than the deeper, less active layer. Thus, the C/N ratio for all tropical peat weighted by depth is 42.9 ± 24.5 (n = 114, including both disturbed and undisturbed peatlands), with the provision that the surface and lower peat have average sampling depths of 7.5 and 125 cm, respectively. The C/N ratio for undisturbed peatlands (32.6 ± 19.5) is slightly higher than the previous estimate of tropical peats of 29.7 ± 17.8 (Leifeld and Menichetti, 2018) since we included more records from lower peat that has higher C/N ratio. The C/N ratio for undisturbed tropical peatlands is in range of that of northern peatlands (30–55, Limpens et al., 2006).
We also noticed that C/N ratios of alpine or montane peatlands in tropical regions (19.9 ± 8.3, n = 23) and tropical lowland peatlands (37.2 ± 18.3, n = 125) show significant difference (p < 0.001). This is probably due to the lower C content in those alpine or montane peatlands in tropical regions (p < 0.001, compared with C% in tropical lowland peatland), suggesting the materials (leaf and root litters etc.) that formed peat are different from that in tropical lowland peatlands, or it is a bias caused by the small sampling set in alpine or montane peatlands.
By simply adopting the depth-weighted mean of C/N ratio of 43 (33 ± 20 for undisturbed and 56 ± 23 for disturbed peatlands) and C stock of 152–288 Gt C (Ribeiro et al., 2020), as C stocks for disturbed and undisturbed are not available yet, we estimated at nitrogen pool of 2.7–8.7 Gt N for tropical peatlands by taking the uncertainty of C/N ratio for disturbed and undisturbed peatlands. In southern peatlands, the N stock has not been reported yet. Since the peat formation condition in Patagonia is similar to that in boreal regions (Loisel and Yu, 2013a), we adopted a C stock of 7.6 Gt C (Loisel and Yu, 2013a) and a C/N ratio of 45 (Loisel et al., 2014), and estimated the N stock of Patagonia at 0.17 Gt N. With northern peatlands storing 10 ± 7 Gt N (Hugelius et al., 2020), summing up the peatland N stocks in the above three regions (i.e., 10 ± 7, 2.7–8.7 and 0.17 Gt N, respectively), the global peatland N stock was estimated at 5.9–25.9 Gt N, which is 4–19% of global total N stocks in the top 100 cm of soil layer [i.e., 133–140 Gt N (Vitousek et al., 1997)].
Generally, there are two approaches used to estimate nitrogen stock in peatlands. The first approach estimates the peatland N stock by dividing the C stock with a mean value of C/N ratio (Loisel et al., 2014; Wang et al., 2015; Leifeld and Menichetti, 2018). The second approach builds a linear relationship between peat depth and N stock with known data of peat cores, with which N stock could be predicted by peat depth (Hugelius et al., 2020). Through the first approach, Loisel et al. (2014) estimated the N stock of northern peatlands at 9.7 Gt N by assuming a mean C/N ratio of 45 based on N content data of 40 peat cores. Through the same approach, Wang et al. (2015) analyzed more than 400 peat cores from Ontario, Canada, and showed that C/N ratio ranged from 22.8 ± 6.1 to 33.0 ± 0.5 (n = ∼1,600), thus they estimated northern peatlands had accumulated 18.5 Gt N since deglaciation. There were two key differences between the above two studies: Wang et al. (2015) collected information on more peat cores while the cores were distributed in a much more extended area in Loisel et al. (2014). For tropical peatlands, Leifeld and Menichetti (2018) estimated the N stock at 4 Gt N based on a median C/N ratio of 29.7 and a C stock of 119.2 Gt C.
Through the second approach, the N stock of northern peatlands was estimated at 10 ± 7 Gt N, and the large uncertainty came from the high variability of depth and total N content (Hugelius et al., 2020). There are several factors that may have large effects on N content and C/N ratio. Vegetation types that form peat are different, with Sphagnum peat having significantly lower N content and higher C/N ratio than woody and herbaceous peat (Loisel et al., 2014). Histories of peatland formation and the evolution of N content and the C/N ratio could be largely driven by climate change in Holocene (Yu, 2011; Zhao et al., 2014).
Decomposition and degradation history also affect N stock of a peatland (Anshari et al., 2010; Yu, 2012). Overall, the estimated regional N stocks still have large uncertainty because of the large spatial heterogeneity of peat C/N ratio and N stock as well as the quite limited data in both of above two approaches.
Long-Term Rate of Nitrogen Accumulation
As summarized in Table 2, the estimation of LORNA of northern peatlands ranges from 0.42 to 0.85 g N m−2 yr−1 (Limpens et al., 2006; Loisel et al., 2014; Wang et al., 2015; Schillereff et al., 2016). In tropical peatlands, the average LORCA was 41.0 ± 20.9 g C m−2 yr−1 (n = 26; Supplementary Data). By applying a depth-weighted mean of the C/N ratio as 33 for undisturbed tropical peatlands (see C/N Ratio of Tropical Peatland and Nitrogen Stock), the average LORNA of tropical undisturbed peatlands was estimated at 1.24 ± 0.63 g N m−2 yr−1. The LORNA in tropical peatlands is faster than that in northern peatlands, which could be mainly explained by the higher LORCA in tropical peatlands than northern peatlands (34 g C m−2 yr−1, Hugelius et al., 2020). In southern peatlands, the LORNA in Patagonia was estimated at 0.49 g N m−2 yr−1 by adopting a LORCA of 22 g C m−2 yr−1 (Yu et al., 2010) and an average C/N ratio of 45.
A more representative dataset of peat properties would help improve the estimation of LORNAs. For example, by estimating the time-weighted C/N ratio (55 ± 33) from 40 peat cores across North America and northern Eurasia, Loisel et al. (2014) indicated that northern peatlands had accumulated N at a rate of 0.5 ± 0.04 g N m−2 yr−1 during the Holocene. Moreover, based on the data of more than 400 peat cores from Ontario, Canada, Wang et al. (2015) obtained the mean of C/N ratio as 27, and estimated LORNA of northern peatlands at 0.85 g N m−2 yr−1 by adopting the LORCA of 23 g C m−2 yr−1 from Loisel et al. (2014). However, it is unknown whether the C/N ratio of 27 or 55 ± 33 is more representative for northern peatlands. Thus, more peat cores containing C and N content are needed to help improve both spatial and temporal resolution for the estimation of N accumulation.
Since LORNA is the average accumulation rate during the whole life-time of a peat-core, it is difficult to reflect the transient or short-term behaviors of N accumulation as well as its change with time. Figure 1 illustrates the changes of a 500-year N accumulation rate from 11,000 years ago to present, which was derived from the dataset of Loisel et al. (2014) that contains 19 peat core profiles with a high-resolution of age and N content data. Although the LORNA was estimated at 0.59 g N m−2 yr−1, the highest N accumulation rate was observed to be between 9.5 and 8 kyr BP, equivalent to 1.3 (mean) g N m−2 yr−1, and the lowest was observed to be between 3 and 2 kyr BP, equivalent to 0.32 g N m−2 yr−1. The variation of the N accumulation rate in peat cores reflected the possible peatlands’ development history—in the early stage of peatland development, peatlands were covered by more herbaceous vegetation characterized by low C/N ratio and high N content, but in the latest stage, they were shifted into Sphagnum-dominated bog characterized by high C/N ratio and low N content (Larmola et al., 2014; Loisel et al., 2014). By calculating the apparent rate of N accumulation from surface to bottom horizon of a peat profile, it was shown that, across the 19 peat cores, the apparent rate had a much higher variability in the most recent period but a lower variability during the Holocene. This pattern is possibly related to large spatial variation of N deposition and/or other N inputs across the peat cores for the most recent period.
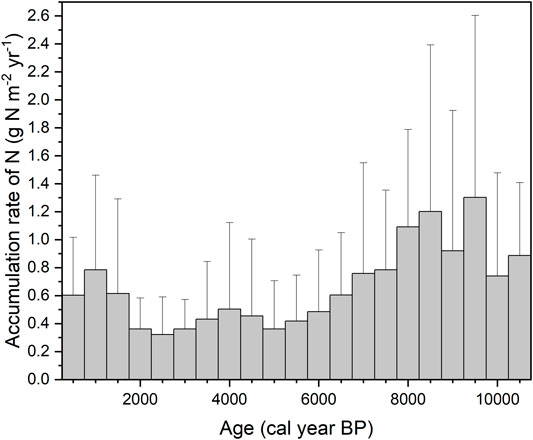
FIGURE 1. Accumulation rate of N in each 500-year bins from 10,500 to 500 cal year BP. Error bars show the standard deviations. Data are from database at https://peatlands.lehigh.edu (n = 19).
Recent Rate of Nitrogen Accumulation
Most of the RERNA data were reported in northern peatlands, only one data of RERNA was reported in southern peatlands (0.97 ± 0.68 g N m−2 yr−1 in Isla Grande de Chiloé, Chile, León and Oliván, 2014) and no data had yet been reported in tropical peatlands. Therefore, the analysis of RERNA mainly focused on that in northern peatlands, as summarized in Table 3.
In northern peatlands, RERNA of Group A was estimated at 0.6 ± 0.4 g N m−2 yr−1 (n = 19), which was 1.2 times that of LORNA (i.e., 0.5 ± 0.04 g N m−2 yr−1). In Group B, RERNA ranged from 0.94 to 2.32 g N m−2 yr−1, with a mean of 1.63 ± 0.43 g N m−2 yr−1 (n = 56) (Malmer and Holm, 1984; Urban and Eisenreich, 1988; Turunen et al., 2004; Moore et al., 2005; Vile et al., 2014; van Bellen et al., 2020). The mean of all records from Group A and Group B was 1.35 ± 0.56 g N m−2 yr−1, which was ∼2.7 times of LORNA. The largest RERNA was reported in the Zoige Plateau, China, as 13.0 ± 7.4 g N m−2 yr−1, corresponding to a rapid recent rate of C accumulation (RERCA) of 259 ± 137 g C m−2 yr−1 (Li et al., 2018). This rapid RERCA was much larger compared to other northern peatlands where RERCAs varied between 40 and 120 g C m−2 yr−1 in the recent 200 years (Turunen et al., 2004; Loisel and Yu, 2013b; van Bellen et al., 2020). Peat cores from other parts of China also showed rapid RERCAs e.g., 184∼376 g C m−2 yr−1 in the recent 100 years in Greater Khingan Range and Sanjiang Plain (Liu et al., 2019) and 124∼293 g C m−2 yr−1 in the recent 200 years in Changbai Mountains (Bao et al., 2010). Moreover, it is still unclear whether all peatlands in China accumulate C (and N as well) at such rapid rates in recent centuries, and an explanation remains to be found. Thus, as the area of Chinese peatlands accounts only a small part for northern peatlands (∼5%, Xu et al., 2018), we did not take this abnormal rate in our global upscaling of RERNA in the following discussion.
The apparent accumulation rate of N shows both high spatial and temporal variations (Figure 1, Figure 2). For example, in Group A, three out of the 19 profiles show the largest apparent accumulation rate in the most recent 500 years, six profiles in the most recent 2,000 years, and the other 10 profiles show the highest accumulation rate between 11 and 7 kyr BP (Loisel et al., 2014). This suggests that accumulated rate of N could depend on the developing periods and the locations with different input and output rates of N.
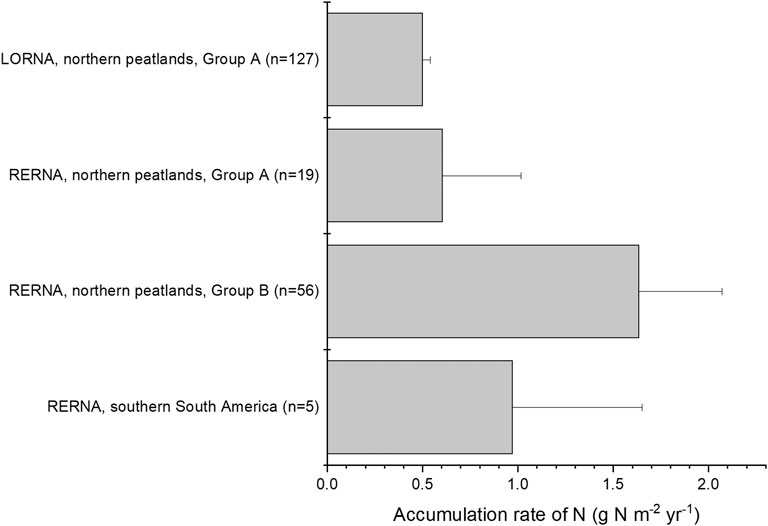
FIGURE 2. Comparison of LORNA and RERNA in peatlands. Group A (n = 127 for LORNA and n = 19 for RERNA) includes data from the database of peat cores provided by Loisel et al. (2014) and Group B (n = 56, total number of cores) includes data from other literature that did not provide properties of peat cores but only provide calculated RERNA. Error bar of LORNA of Group A shows 1 SE, while other error bars show one SD.
BNF and N Balance in Peatlands
Most of the BNF studies were performed in northern peatlands, and these available data on peatland BNF are quite limited and with large uncertainty (Table 3). Annual BNF rates were between 0.006–11.5 g N m−2 yr−1 in northern peatlands, with an arithmetic mean of 1.9 ± 2.7 g N m−2 yr−1 (n = 21, Table 3). In different types of peatland, the mean rates of BNF were 2.7 ± 3.9 g N m−2 yr−1 in fens and 0.8 ± 1.1 g N m−2 yr−1 in bogs, but the difference was non-significant (p = 0.13). Previous studies suggested that added phosphorus (P) could stimulate N fixing to meet increased N demand from plant (Larmola et al., 2014; Kox et al., 2016), and molybdenum (Mo) availability limited nitrogenase activity (Rousk and Michelsen, 2017; Warren et al., 2017). Both elements (P and Mo) should be better supplied in fens than in bogs and, indeed, a mesotrophic fen had more BNF than any other stage of peatland development of the same succession in Finland (Larmola et al., 2014). However, our results reflect large uncertainty in peatland BNF.
Despite that BNF rate was reported to be positively correlated with temperature in previous studies (Basilier et al., 1978; Schwintzer, 1983; Urban and Eisenreich, 1988), we found a weak correlation between annual BNF rate and the mean growing season temperature in northern peatlands (Figure 3A, r = −0.26, p = 0.276). Moreover, we also observed a weak and non-significant correlation between annual BNF rate and mean annual precipitation (Figure 3B, r = −0.20, p = 0.423). Our results are consistent with a recent review which found that the correlations between BNF and annual temperature as well as precipitation were weak across the globe (Davies-Barnard and Friedlingstein, 2020). But it should be noticed that our result did not take tropical peatlands into account due to the lack of data. In Long-Term Rate of Nitrogen Accumulation, the LORNA in tropical peatlands was approximately 2 times that in northern peatlands; thus, we suggest that the BNF in tropical peatlands might be much higher than that in northern peatlands, and more observations are needed to determine this point.
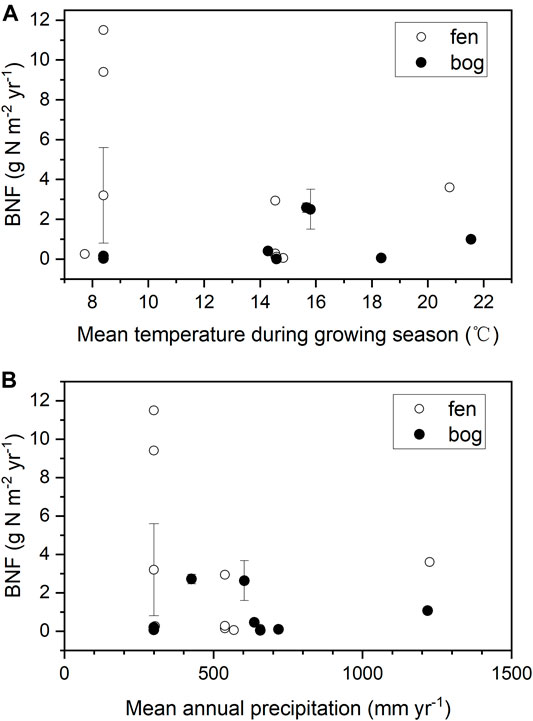
FIGURE 3. BNF plotted against (A) mean temperature during growing season (June-August, °C), and (B) annual precipitation (mm yr−1). Climate data are mean between years of 1981–2010. Error bars show one SD.
The method used to determine the BNF rate might introduce large uncertainty (Flett et al., 1975; Knorr et al., 2015; Saiz et al., 2019). The most common method, ARA, is based on the versatility of the nitrogenase enzyme: its preference for performing a reaction of reducing C2H2 to C2H4 is higher than that of reducing N2 (Schollhorn and Burris, 1967). Based on electron transfer equivalents, a theoretical conversion factor (CF) of 4:1 (moles of C2H4 produced per mole of N2 fixed) was considered in vitro and 3:1 in vivo (Hardy et al., 1971). These theoretical CFs were used in many studies (Basilier et al., 1978; Markham, 2009; Rousk et al., 2018). However, ARA could either underestimate or overestimate nitrogenase activity (Flett et al., 1975; Witty, 1979; Vile et al., 2014). For example, C2H2 inhibits the nitrogenase activity of N2-fixing methanotrophs, by inhibiting methane oxidation (King, 1996) which provides ATP needed in the reduction of C2H2 (Hardy et al., 1968). N2-fixing methanotrophs also transform C2H2 into compounds undetectable for ARA, thus making ARA unreliable (Flett et al., 1975). Overestimation happens when the amount of endogenously produced ethylene is comparable to the ethylene produced by nitrogenase where BNF rates are less than 1 g N ha−1 d−1 (Witty, 1979). Early studies indicated the bias of CF from theoretical values and suggested site-specific calibration of the CF (Granhall and Selander, 1973; Schwintzer, 1983). Recent studies obtained CF ranging from 0.26 to 1.8 by calibration with the 15N2 method (Vile et al., 2014; Knorr et al., 2015; Warren et al., 2017). However, an elaborate study found that CF was highly variable—from 0.001 to 5.363—across different Sphagnum species, site scales and over time, indicating accurate calibration may not be practical in peatlands (Saiz et al., 2019).
Generally, BNF is performed by microbes, mainly rhizobium in many ecosystems (Cleveland et al., 1999). However, legumes are usually rare in peatland ecosystems (Schwintzer, 1983; Vitt, 2006; Borken et al., 2016; Laine et al., 2021) and the main N2-fixers in peatlands remain controversial. Early studies reported that the nitrogen-fixing organisms in peatlands were cyanobacteria for Sphagnum and actinomycete symbionts for dwarf shrubs (Basilier et al., 1978; Schwintzer, 1983). However, recent studies suggested that methanotrophs introduced most BNF in early stage of peatland development (Larmola et al., 2014; Vile et al., 2014; Rousk et al., 2015). By quantifying the genetic expression of 16S rRNA and nitrogenase-encoding nifH, Vile et al. (2014) showed that BNF rates were mainly originated from methanotrophs rather than cyanobacteria in pristine bogs in boreal Alberta, Canada. Leppanen et al. (2015) confirmed that most of nifH sequences were assigned to the class Alphaproteobacteria (82%), and only a few were assigned to Cyanobacteria (5%). However, elevated CH4 concentration did not enhance BNF (Leppanen et al., 2015), indicating that BNF may not be solely controlled by methanotroph activity. Kox et al. (2018) had similar conclusion that most of the 16S rRNA genes were assigned to Alphaproteobacteria, and only 0.1% were bona fide methane-oxidizing taxa and addition of methane did not simulate incorporation of 15N-nitrogen into biomass whereas oxygen depletion increased the activity of the nitrogen-fixing community. Another candidate of N-fixing organisms is Azospirillum, which co-exists with methanotrophic bacteria (Doroshenko et al., 2007) and symbiotic bacteria (i.e., Frankia (Actinomycetaceae) and Beijerinckiaceae (Rhizobiales)) (HussDanell, 1997; Huguet et al., 2001; Vile et al., 2014; Borken et al., 2016). Nevertheless, the activity of N2-fixing organisms could be limited by other nutrients, especially phosphorus, despite sufficient C supply (Limpens et al., 2004; Larmola et al., 2014; Ho and Bodelier, 2015). Therefore, more efforts are needed to understand the mechanisms and contributions of different N-fixing organisms on BNF in peatlands.
Since BNF rates in boreal peatlands are weakly correlated with temperature, precipitation and nutrient condition (ombrotrophic bog or minerotrophic fen), the BNF could not be predicted from these factors. The method of BNF measurement and the knowledge of N2-fixing microbes also decrease the accuracy of determination of BNF rates. Based on what we know, we simply assessed the BNF rate of global peatlands. The annual BNF of northern peatlands was estimated at 6.5 Tg N yr−1, by adopting a mean rate of 1.9 g N m−2 yr−1 from measurements in boreal peatlands, and an area of 342 Mha of northern peatlands (Hugelius et al., 2020). The BNF of global peatlands was estimated at 8.0 Tg N yr−1 by adopting the same rate as that in northern peatlands, and applying a global peatland area of 423 Mha (Xu et al., 2018). This number accounts for ∼14% of the pre-industrial BNF rate of global terrestrial ecosystems (58 Tg N yr−1) (Vitousek et al., 2013), and is at the same order of reactive N produced by lightning (5 ± 3 Tg N yr−1) (Schumann and Huntrieser, 2007).
In addition to N inputs as discussed above, the N budget of peatlands also includes several major N outputs such as denitrification and runoff/discharge (Limpens et al., 2006). Denitrification involves a series of complicated biochemical processes where microbes convert nitrate (NO3−) and nitrite (NO2−) to nitric oxide (NO), nitrous oxide (N2O) and dinitrogen (N2) in gas form (Seitzinger et al., 2006; Groffman and Peter, 2012). Denitrification is difficult to quantify because of the challenge of distinguishing its end product N2 from ambient atmospheric N2, and the lack of a suitable method for upscaling the site scale measurements to regional scale due to high spatial and temporal variability (van Groenigen et al., 2015). Limpens et al. (2006) suggested a denitrification (in the form of emission of N2O) rate of 0.2 g N m−2 yr−1 by reviewing previous studies. Hill et al. (2016) reported a denitrification rate of <0.1 g N m−2 yr−1 in central peats in a bog and a fen in Minnesota, United States, and a denitrification rate of 0.36 g N m−2 yr−1 in the lag/transition part of the bog. However, Wray and Bayley (2007) reported much larger denitrification rates of 11 g N m−2 yr−1 in marshes and 24 g N m−2 yr−1 in fens in Alberta, Canada. N outflow through runoff was estimated at 0.3 (0.15–0.63) g N m−2 yr−1 (Limpens et al., 2006), but this flux is highly site-dependent, ranging from 0.01 g N m−2 yr−1 in boreal oligotrophic bogs (Hill et al., 2016) to 1.9 ± 0.3 g N m−2 yr−1 in a slope mire in Germany (Tauchnitz et al., 2010). Overall, the outputs of N are highly variant and uncertain, and limited observations critically limit global estimation.
For peatlands with measured annual BNF rates (Table 4), the mean of atmospheric N deposition was 0.5 ± 0.4 g N m−2 yr−1 (n = 11), the mean of BNF was 1.9 ± 2.7 g N m−2 yr−1 (n = 21), and average RERNA of northern peatlands was 1.35 ± 0.56 g N m−2 yr−1 (n = 80; Recent Rate of Nitrogen Accumulation). Assuming inflow and outflow of boreal oligotrophic bogs are negligible (Moore and Bubier, 2020), BNF and atmospheric deposition are the largest and second largest sources of N, which account for ∼75 and 25%, respectively, of the total input. Denitrification, the only N output to balance the N budget, is 1.0 g N m−2 yr−1. A conceptual diagram (Figure 4) shows the N cycle with large uncertainties based on literature reports of peatland N budget.
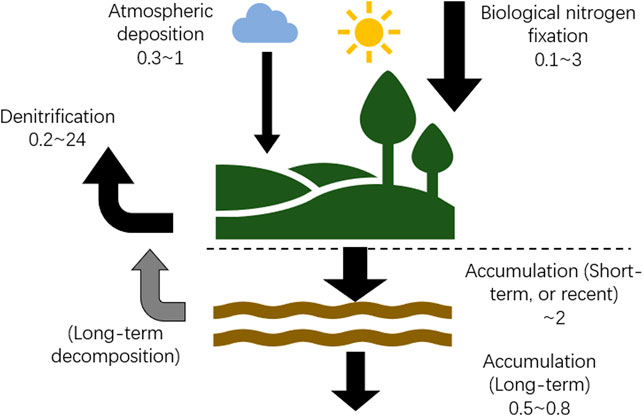
FIGURE 4. Schematic diagram of N inputs and outputs in a boreal bog. Rates are summarized from literature. Unit of rates is g N m−2 yr−1.
Conclusion
Peatlands have accumulated large amount of nitrogen in the past thousands of years. The large uncertainty of estimates of N stock and its accumulation rate in global peatlands is due to the large spatial and temporal variation of the C/N ratio and C accumulation rate in peats. For the sources of the large N stock in peatlands, our synthesis suggests that BNF may have the largest contribution to N input of peatlands, at least larger than atmospheric N deposition at the millennial timescale.
In this study, our findings are based on limited observations. There are still many unknowns in the N cycle of peatlands. The most pressing issue is that the great difference between the LORNA and RERNA indicates that most of N retained in peatland in a short-term timescale would not enter the long-term N stock. It is known that decomposition continues for a very long time in the C accumulation dynamics of peatlands (Young et al., 2019). However, we do not know in which form, how much and through which biogeochemical processes this N is released. It also remains unclear how N, if released, might affect adjacent ecosystems. Applying the 15N2 assimilation method instead of ARA would help improve the accuracy of BNF rate measurements. Nutrient supply through groundwater has long been ignored, but this absent part, along with streamflow, may help explain the “missing N” in peatlands. More field and modeling studies are both needed to improve our understanding of the N cycle in peatlands.
Data Availability Statement
The original contributions presented in the study are included in the article/Supplementary Material, further inquiries can be directed to the corresponding author.
Author Contributions
SP designed the study. TY performed the analysis and created all the tables and figures. All authors contributed the writing of the manuscript.
Funding
This study was supported by the National Natural Science Foundation of China (Grant Number 41830643).
Conflict of Interest
The authors declare that the research was conducted in the absence of any commercial or financial relationships that could be construed as a potential conflict of interest.
Publisher’s Note
All claims expressed in this article are solely those of the authors and do not necessarily represent those of their affiliated organizations, or those of the publisher, the editors and the reviewers. Any product that may be evaluated in this article, or claim that may be made by its manufacturer, is not guaranteed or endorsed by the publisher.
Acknowledgments
We acknowledge the community shared the data of peat cores (https://peatlands.lehigh.edu/).
Supplementary Material
The Supplementary Material for this article can be found online at: https://www.frontiersin.org/articles/10.3389/feart.2022.670867/full#supplementary-material
References
Aerts, R. (1997). Atmospheric Nitrogen Deposition Affects Potential Denitrification and N2O Emission from Peat Soils in the Netherlands. Soil Biol. Biochem. 29 (7), 1153–1156. doi:10.1016/s0038-0717(96)00308-2
Anshari, G. Z., Afifudin, M., Nuriman, M., Gusmayanti, E., Arianie, L., Susana, R., et al. (2010). Drainage and Land Use Impacts on Changes in Selected Peat Properties and Peat Degradation in West Kalimantan Province, Indonesia. Biogeosciences 7 (11), 3403–3419. doi:10.5194/bg-7-3403-2010
Bao, K., Yu, X., Jia, L., and Wang, G. (2010). Recent Carbon Accumulation in Changbai Mountain Peatlands, Northeast China. Mountain Res. Dev. 30 (1), 33–41. doi:10.1659/mrd-journal-d-09-00054.1
Basilier, K., Granhall, U., Stenström, T.-A., and Stenstrom, T.-A. (1978). Nitrogen Fixation in Wet Minerotrophic Moss Communities of a Subarctic Mire. Oikos 31 (2), 236–246. doi:10.2307/3543568
Borken, W., Horn, M. A., Geimer, S., Aguilar, N. A. B., and Knorr, K.-H. (2016). Associative Nitrogen Fixation in Nodules of the conifer Lepidothamnus Fonkii (Podocarpaceae) Inhabiting Ombrotrophic Bogs in Southern Patagonia. Sci. Rep. 6, 8. doi:10.1038/srep39072
Broder, T., Blodau, C., Biester, H., and Knorr, K. H. (2012). Peat Decomposition Records in Three Pristine Ombrotrophic Bogs in Southern Patagonia. Biogeosciences 9 (4), 1479–1491. doi:10.5194/bg-9-1479-2012
Carrell, A. A., Kolton, M., Glass, J. B., Pelletier, D. A., Warren, M. J., Kostka, J. E., et al. (2019). Experimental Warming Alters the Community Composition, Diversity, and N 2 Fixation Activity of Peat moss ( Sphagnum Fallax ) Microbiomes. Glob. Change Biol. 25 (9), 2993–3004. doi:10.1111/gcb.14715
Chapman, R. R., and Hemond, H. F. (1982). Dinitrogen Fixation by Surface Peat and Sphagnum in an Ombrotrophic Bog. Can. J. Bot. 60 (5), 538–543. doi:10.1139/b82-072
Cleveland, C. C., Townsend, A. R., Schimel, D. S., Fisher, H., Howarth, R. W., Hedin, L. O., et al. (1999). Global Patterns of Terrestrial Biological Nitrogen (N2) Fixation in Natural Ecosystems. Glob. Biogeochem. Cycles 13 (2), 623–645. doi:10.1029/1999gb900014
Cowley, K. L., and Fryirs, K. A. (2020). Forgotten Peatlands of Eastern Australia: An Unaccounted Carbon Capture and Storage System. Science of the Total Environment 720, 8. doi:10.1016/j.scitotenv.2020.139067
Dargie, G. C., Lewis, S. L., Lawson, I. T., Mitchard, E. T. A., Page, S. E., Bocko, Y. E., et al. (2017). Age, Extent and Carbon Storage of the central Congo Basin Peatland Complex. Nature 542(7639), 86, 90. doi:10.1038/nature21048
Davies-Barnard, T., and Friedlingstein, P. (2020). The Global Distribution of Biological Nitrogen Fixation in Terrestrial Natural Ecosystems. Glob. Biogeochem. Cycles 34 (3), 17. doi:10.1029/2019gb006387
Doroshenko, E. V., Boulygina, E. S., Spiridonova, E. M., Tourova, T. P., and Kravchenko, I. K. (2007). Isolation and Characterization of Nitrogen-Fixing Bacteria of the Genus Azospirillum from the Soil of a Sphagnum Peat Bog. Microbiology 76 (1), 93–101. doi:10.1134/s0026261707010134
Draper, F. C., Roucoux, K. H., Lawson, I. T., Mitchard, E. T. A., Coronado, E. N. H., Lahteenoja, O., et al. (2014). The Distribution and Amount of Carbon in the Largest Peatland Complex in Amazonia. Environmental Research Letters 9 (12), 12. doi:10.1088/1748-9326/9/12/124017
Fenn, M. E., Haeuber, R., Tonnesen, G. S., Baron, J. S., Grossman-Clarke, S., Hope, D., et al. (2003). Nitrogen Emissions, Deposition, and Monitoring in the Western United States. Bioscience 53 (4), 391–403. doi:10.1641/0006-3568(2003)053[0391:nedami]2.0.co;2
Flett, R. J., Rudd, J. W. M., and Hamilton, R. D. (1975). Acetylene Reduction Assays for Nitrogen Fixation Freshwaters: a Note of Caution. Appl.environ.microbiol. 29, 580–583. doi:10.1128/am.29.5.580-583.1975
Gallego-Sala, A. V., Charman, D. J., Brewer, S., Page, S. E., Prentice, I. C., Friedlingstein, P., et al. (2018). Latitudinal Limits to the Predicted Increase of the Peatland Carbon Sink with Warming. Nat. Clim. Change 8 (10), 907. doi:10.1038/s41558-018-0271-1
Girkin, N. T., Lopes dos Santos, R. A., Vane, C. H., Ostle, N., Turner, B. L., and Sjögersten, S. (2020). Peat Properties, Dominant Vegetation Type and Microbial Community Structure in a Tropical Peatland. Wetlands 40 (5), 1367–1377. doi:10.1007/s13157-020-01287-4
Godoy, R., Paulino, L., Oyarzún, C., and Boeckx, P. (2003). ATMOSPHERIC N DEPOSITION IN CENTRAL AND SOUTHERN CHILE: AN OVERVIEW. Gayana Bot. 60 (1), 47–53. doi:10.4067/s0717-66432003000100008
Gorham, E. (1991). Northern Peatlands - Role in the Carbon-Cycle and Probable Responses to Climatic Warming. Ecological Applications 1 (2), 182–195. doi:10.2307/1941811
Granhall, U., and Selander, H. (1973). Nitrogen Fixation in a Subarctic Mire. Oikos 24 (1), 8–15. doi:10.2307/3543247
Groffman, P. M., and Peter, M. (2012). Terrestrial Denitrification: Challenges and Opportunities. Ecol. Process. 1 (1), 1. doi:10.1186/2192-1709-1-11
Grover, S. P. P., McKenzie, B. M., Baldock, J. A., and Papst, W. A. (2005). Chemical Characterisation of Bog Peat and Dried Peat of the Australian Alps. Australian Journal of Soil Research 43 (8), 963–971. doi:10.1071/sr04014
Hardy, R. W. F., Holsten, R. D., Jackson, E. K., and Burns, R. C. (1968). The Acetylene-Ethylene Assay for N2 Fixation: Laboratory and Field Evaluation. Plant Physiol. 43 (8), 1185–1207. doi:10.1104/pp.43.8.1185
Hardy, R. W. F., Burns, R. C., Holsten, R. D., Hebert, R. R., and Jackson, E. K. (1971). BIOLOGICAL NITROGEN FIXATION - KEY TO WORLD PROTEIN. Plant and Soil 35, 561–590. doi:10.1007/bf02661879
Harris, I., Osborn, T. J., Jones, P., and Lister, D. (2020). Version 4 of the CRU TS Monthly High-Resolution Gridded Multivariate Climate Dataset. Sci. Data 7 (1), 109. doi:10.1038/s41597-020-0453-3
Hill, B. H., Jicha, T. M., Lehto, L. L. P., Elonen, C. M., Sebestyen, S. D., and Kolka, R. K. (2016). Comparisons of Soil Nitrogen Mass Balances for an Ombrotrophic Bog and a Minerotrophic Fen in Northern Minnesota. Sci. Total Environ. 550, 880–892. doi:10.1016/j.scitotenv.2016.01.178
Ho, A., and Bodelier, P. L. (2015). Diazotrophic Methanotrophs in Peatlands: the Missing Link. Plant Soil 389 (1-2), 419–423. doi:10.1007/s11104-015-2393-9
Hugelius, G., Loisel, J., Chadburn, S., Jackson, R. B., Jones, M., MacDonald, G., et al. (2020). Large Stocks of Peatland Carbon and Nitrogen Are Vulnerable to Permafrost Thaw. Proc. Natl. Acad. Sci. USA 117 (34), 20438–20446. doi:10.1073/pnas.1916387117
Huguet, V., Batzli, J. M., Zimpfer, J. F., Normand, P., Dawson, J. O., and Fernandez, M. P. (2001). Diversity and Specificity of Frankia Strains in Nodules of Sympatric Myrica Gale, Alnus Incana , and Shepherdia canadensis Determined by Rrs Gene Polymorphism. Appl. Environ. Microbiol. 67 (5), 2116–2122. doi:10.1128/aem.67.5.2116-2122.2001
Huss-danell, K. (1997). Actinorhizal Symbioses and Their N 2 Fixation. New Phytol. 136 (3), 375–405. doi:10.1046/j.1469-8137.1997.00755.x
King, G. M. (1996). In Situ analyses of Methane Oxidation Associated with the Roots and Rhizomes of a Bur Reed, Sparganium Eurycarpum, in a Maine Wetland. Appl. Environ. Microbiol. 62 (12), 4548–4555. doi:10.1128/aem.62.12.4548-4555.1996
Knorr, K.-H., Horn, M. A., and Borken, W. (2015). Significant Nonsymbiotic Nitrogen Fixation in Patagonian Ombrotrophic Bogs. Glob. Change Biol. 21 (6), 2357–2365. doi:10.1111/gcb.12849
Kox, M. A. R., Lüke, C., Fritz, C., van den Elzen, E., van Alen, T., Op den Camp, H. J. M., et al. (2016). Effects of Nitrogen Fertilization on Diazotrophic Activity of Microorganisms Associated with Sphagnum Magellanicum. Plant Soil 406 (1-2), 83–100. doi:10.1007/s11104-016-2851-z
Kox, M. A. R., Aalto, S. L., Penttilä, T., Ettwig, K. F., Jetten, M. S. M., and van Kessel, M. A. H. J. (2018). The Influence of Oxygen and Methane on Nitrogen Fixation in Subarctic Sphagnum Mosses. AMB Express 8, 9. doi:10.1186/s13568-018-0607-2
Kox, M. A. R., Kop, L. F. M., van den Elzen, E., van Alen, T. A., Lamers, L. P. M., van Kessel, M., et al. (2020). Functional Redundancy of the Methane-Oxidising and Nitrogen-Fixing Microbial Community Associated with Sphagnum Fallax and Sphagnum Palustre in Two Dutch Fens. Mires and Peat 26, 15. doi:10.19189/MaP.2019.SNPG.StA.1885
Kuhry, P., and Vitt, D. H. (1996). Fossil Carbon/nitrogen Ratios as a Measure of Peat Decomposition. Ecology 77 (1), 271–275. doi:10.2307/2265676
Laine, A. M., Lindholm, T., Nilsson, M., Kutznetsov, O., Jassey, V. E. J., and Tuittila, E. S. (2021). Functional Diversity and Trait Composition of Vascular Plant and Sphagnum moss Communities during Peatland Succession across Land Uplift Regions. J. Ecol. 109 (4), 1774–1789. doi:10.1111/1365-2745.13601
Larmola, T., Leppanen, S. M., Tuittila, E.-S., Aarva, M., Merila, P., Fritze, H., et al. (2014). Methanotrophy Induces Nitrogen Fixation during Peatland Development. Proc. Natl. Acad. Sci. 111 (2), 734–739. doi:10.1073/pnas.1314284111
Leifeld, J., and Menichetti, L. (2018). The Underappreciated Potential of Peatlands in Global Climate Change Mitigation Strategies. Nat. Commun. 9, 7. doi:10.1038/s41467-018-03406-6
León, C. A., and Oliván, G. (2014). Recent rates of carbon and nitrogen accumulation in peatlands of Isla Grande de Chiloé-Chile. Rev. Chil. de Hist. Nat. 87. doi:10.1186/s40693-014-0026-y
Leppänen, S. M., Rissanen, A. J., and Tiirola, M. (2015). Nitrogen Fixation in Sphagnum Mosses Is Affected by moss Species and Water Table Level. Plant Soil 389 (1-2), 185–196. doi:10.1007/s11104-014-2356-6
Li, C., Huang, Y., Guo, H., Cui, L., and Li, W. (2018). Draining Effects on Recent Accumulation Rates of C and N in Zoige Alpine Peatland in the Tibetan Plateau. Water 10 (5), 576. doi:10.3390/w10050576
Li, L., Gao, J.-Q., Lei, G.-C., Lu, c., Suo, L.-E., et al. (2011). Distribution Patterns of Soil Organic Carbon and Total Nitrogen in Zoige Peat Land With Different Ground Water Table. Shengtaixue Zazhi 30 (11), 2449–2455.
Limpens, J., Berendse, F., and Klees, H. (2004). How Phosphorus Availability Affects the Impact of Nitrogen Deposition on Sphagnum and Vascular Plants in Bogs. Ecosystems 7 (8), 793–804. doi:10.1007/s10021-004-0274-9
Limpens, J., Heijmans, M. M. P. D., and Berendse, F. (2006). “The Nitrogen Cycle in Boreal Peatlands,” in Boreal Peatland Ecosystems. Editors R. K. Wieder, and D. H. Vitt, 195–230.
Liu, H., Yu, Z., Han, D., Gao, C., Yu, X., and Wang, G. (2019). Temperature Influence on Peatland Carbon Accumulation over the Last century in Northeast China. Clim. Dyn. 53 (3-4), 2161–2173. doi:10.1007/s00382-019-04813-1
Liu, L. J., Chen, H., Yu, Z. C., Zhu, D., He, Y. X., Liu, J. L., et al. (2020). Peatland Development and Carbon Dynamics Since the Last Glacial Maximum in the Hengduan Mountains Region. Catena 190, 9. doi:10.1016/j.catena.2020.104525
Loisel, J., Yu, Z., Beilman, D. W., Camill, P., Alm, J., Amesbury, M. J., et al. (2014). A Database and Synthesis of Northern Peatland Soil Properties and Holocene Carbon and Nitrogen Accumulation. The Holocene 24 (9), 1028–1042. doi:10.1177/0959683614538073
Loisel, J., and Yu, Z. (2013a). Holocene Peatland Carbon Dynamics in Patagonia. Quat. Sci. Rev. 69, 125–141. doi:10.1016/j.quascirev.2013.02.023
Loisel, J., and Yu, Z. (2013b). Recent Acceleration of Carbon Accumulation in a Boreal Peatland, South central Alaska. J. Geophys. Res. Biogeosci. 118 (1), 41–53. doi:10.1029/2012jg001978
Malmer, N., and Holm, E. (1984). Variation in the C/N-Quotient of Peat in Relation to Decomposition Rate and Age Determination with 210 Pb. Oikos 43 (2), 171–182. doi:10.2307/3544766
Markham, J. H. (2009). Variation in moss-associated Nitrogen Fixation in Boreal forest Stands. Oecologia 161 (2), 353–359. doi:10.1007/s00442-009-1391-0
Melling, L., Hatano, R., and Goh, K. J. (2005). Soil CO2 Flux from Three Ecosystems in Tropical Peatland of Sarawak, Malaysia. Tellus B: Chem. Phys. Meteorology 57 (1), 1–11. doi:10.3402/tellusb.v57i1.16772
Moore, T., Blodau, C., Turunen, J., Roulet, N., and Richard, P. J. H. (2005). Patterns of Nitrogen and Sulfur Accumulation and Retention in Ombrotrophic Bogs, Eastern Canada. Glob. Change Biol 11 (2), 356–367. doi:10.1111/j.1365-2486.2004.00882.x
Moore, T. R., and Bubier, J. L. (2020). Plant and Soil Nitrogen in an Ombrotrophic Peatland, Southern Canada. Ecosystems 23 (1), 98–110. doi:10.1007/s10021-019-00390-w
Nadim, F., Trahiotis, M. M., Stapcinskaite, S., Perkins, C., Carley, R. J., Hoag, G. E., et al. (2001). Estimation of Wet, Dry and Bulk Deposition of Atmospheric Nitrogen in Connecticut. J. Environ. Monitor. 3 (6), 671–680. doi:10.1039/b107008h
Nichols, J. E., and Peteet, D. M. (2019). Rapid Expansion of Northern Peatlands and Doubled Estimate of Carbon Storage. Nature Geoscience 12 (11), 917. doi:10.1038/s41561-019-0454-z
Page, S. E., and Baird, A. J. (2016). “Peatlands and Global Change: Response and Resilience,”. Annual Review of Environment and Resources. Editors A. Gadgil, and T. P. Gadgil (Palo Alto: Annual Reviews), 41, 35–57. doi:10.1146/annurev-environ-110615-085520
Page, S. E., Rieley, J. O., and Banks, C. J. (2011). Global and Regional Importance of the Tropical Peatland Carbon Pool. Glob. Change Biol. 17 (2), 798–818. doi:10.1111/j.1365-2486.2010.02279.x
Patova, E. N., Sivkov, M. D., Goncharova, N. N., and Shubina, T. P. (2020). Associations between Nitrogen-Fixing Cyanobacteria and Sphagnum Mosses in Floodplain Bogs of the Middle Taiga (European Northeast). Theor. Appl. Ecol. (1), 117–123. doi:10.25750/1995-4301-2020-1-117-123
Pemberton, M. (2005). Australian Peatlands: a Brief Consideration of Their Origin, Distribution, Natural Values and Threats. Journal of the Royal Society of Western Australia 88, 81–89.
Ribeiro, K., Pacheco, F. S., Ferreira, J. W., Sousa-Neto, E. R., Hastie, A., Krieger Filho, G. C., et al. (2020). Tropical Peatlands and Their Contribution to the Global Carbon Cycle and Climate Change. Glob. Change Biol. 27, 489–505. doi:10.1111/gcb.15408
Rousk, K., Sorensen, P. L., Lett, S., and Michelsen, A. (2015). Across-Habitat Comparison of Diazotroph Activity in the Subarctic. Microb. Ecol. 69 (4), 778–787. doi:10.1007/s00248-014-0534-y
Rousk, K., Vestergård, M., and Christensen, S. (2018). Are Nitrous Oxide Emissions and Nitrogen Fixation Linked in Temperate Bogs? Soil Biol. Biochem. 123, 74–79. doi:10.1016/j.soilbio.2018.05.002
Rousk, K., and Michelsen, A. (2017). Ecosystem Nitrogen Fixation throughout the Snow-free Period in Subarctic Tundra: Effects of Willow and Birch Litter Addition and Warming. Glob. Change Biol. 23 (4), 1552–1563. doi:10.1111/gcb.13418
Saiz, E., Sgouridis, F., Drijfhout, F. P., and Ullah, S. (2019). Biological Nitrogen Fixation in Peatlands: Comparison between Acetylene Reduction Assay and 15N2 Assimilation Methods. Soil Biol. Biochem. 131, 157–165. doi:10.1016/j.soilbio.2019.01.011
Sannel, A. B. K., and Kuhry, P. (2009). Holocene Peat Growth and Decay Dynamics in Sub-arctic Peat Plateaus, West-central Canada. Boreas 38 (1), 13–24. doi:10.1111/j.1502-3885.2008.00048.x
Schillereff, D. N., Boyle, J. F., Toberman, H., Adams, J. L., Bryant, C. L., Chiverrell, R. C., et al. (2016). Long-term Macronutrient Stoichiometry of UK Ombrotrophic Peatlands. Sci. Total Environ. 572, 1561–1572. doi:10.1016/j.scitotenv.2016.03.180
Schollhorn, R., and Burris, R. H. (1967). Acetylene as a Competitive Inhibitor of N-2 Fixation. Proc. Natl. Acad. Sci. 58(1), 213, 216. doi:10.1073/pnas.58.1.213
Schumann, U., and Huntrieser, H. (2007). The Global Lightning-Induced Nitrogen Oxides Source. Atmos. Chem. Phys. 7 (14), 3823–3907. doi:10.5194/acp-7-3823-2007
Schwintzer, C. R. (1983). Nonsymbiotic and Symbiotic Nitrogen Fixation in a Weakly Minerotrophic Peatland. Am. J. Bot. 70 (7), 1071–1078. doi:10.1002/j.1537-2197.1983.tb07908.x
Seitzinger, S., Harrison, J. A., Böhlke, J. K., Bouwman, A. F., Lowrance, R., Peterson, B., et al. (2006). Denitrification across Landscapes and Waterscapes: A Synthesis. Ecol. Appl. 16 (6), 2064–2090. doi:10.1890/1051-0761(2006)016[2064:dalawa]2.0.co;2
Sjogersten, S., Cheesman, A. W., Lopez, O., and Turner, B. L. (2011). Biogeochemical Processes Along a Nutrient Gradient in a Tropical Ombrotrophic Peatland. Biogeochemistry 104, 147–163.
Sorensen, P. L., Jonasson, S., and Michelsen, A. (2006). Nitrogen Fixation, Denitrification, and Ecosystem Nitrogen Pools in Relation to Vegetation Development in the Subarctic. Arctic, Antarctic, Alpine Res. 38 (2), 263–272. doi:10.1657/1523-0430(2006)38[263:nfdaen]2.0.co;2
Stewart, K. J., Coxson, D., and Grogan, P. (2011). Nitrogen Inputs by Associative Cyanobacteria across a Low Arctic Tundra Landscape. Arctic, Antarctic, Alpine Res. 43 (2), 267–278. doi:10.1657/1938-4246-43.2.267
Tauchnitz, N., Meissner, R., Bernsdorf, S., and Wegener, U. (2010). Nitrogen Fluxes of a Slope Mire in the German Harz Mountains. Water Air Soil Pollut. 205 (1-4), 107–112. doi:10.1007/s11270-009-0059-z
Tolonen, T., and Turunen, J. (1996). Accumulation of Carbon in Mires of Finland and Implications for Climatic Change. Holocene 6, 171–178. doi:10.1177/095968369600600204
Turunen, J., Roulet, N. T., Moore, T. R., and Richard, P. J. H. (2004). Nitrogen Deposition and Increased Carbon Accumulation in Ombrotrophic Peatlands in Eastern Canada. Glob. Biogeochem. Cycles 18 (3), a–n. doi:10.1029/2003gb002154
Urban, N. R., and Eisenreich, S. J. (1988). NITROGEN CYCLING IN A FORESTED MINNESOTA BOG. Can. J. Bot. 66 (3), 435–449. doi:10.1139/b88-069
van Bellen, S., Shotyk, W., Magnan, G., Davies, L., Nason, T., Mullan-Boudreau, G., et al. (2020). Carbon and Nitrogen Accumulation Rates in Ombrotrophic Peatlands of central and Northern Alberta, Canada, during the Last Millennium. Biogeochemistry 151 (2-3), 251–272. doi:10.1007/s10533-020-00724-0
van den Elzen, E., Bengtsson, F., Fritz, C., Rydin, H., and Lamers, L. P. M. (2020). Variation in Symbiotic N2 Fixation Rates Among Sphagnum Mosses. Plos One 15 (2), e0228383. doi:10.1371/journal.pone.0228383
van Groenigen, J. W., Huygens, D., Boeckx, P., Kuyper, T. W., Lubbers, I. M., Rütting, T., et al. (2015). The Soil N Cycle: New Insights and Key Challenges. Soil 1 (1), 235–256. doi:10.5194/soil-1-235-2015
Verry, E. S., and Timmons, D. R. (1982). Waterborne Nutrient Flow through an Upland-Peatland Watershed in Minnesota. Ecology 63 (5), 1456–1467. doi:10.2307/1938872
Vile, M. A., Kelman Wieder, R., Živković, T., Scott, K. D., Vitt, D. H., Hartsock, J. A., et al. (2014). N2-fixation by Methanotrophs Sustains Carbon and Nitrogen Accumulation in Pristine Peatlands. Biogeochemistry 121 (2), 317–328. doi:10.1007/s10533-014-0019-6
Vitousek, P. M., Aber, J. D., Howarth, R. W., Likens, G. E., Matson, P. A., Schindler, D. W., et al. (1997). Human Alteration of the Global Nitrogen Cycle: Sources and Consequences. Ecol. Appl. 7 (3), 737–750. doi:10.1890/1051-0761(1997)007[0737:haotgn]2.0.co;2
Vitousek, P. M., Menge, D. N. L., Reed, S. C., and Cleveland, C. C. (2013). Biological Nitrogen Fixation: Rates, Patterns and Ecological Controls in Terrestrial Ecosystems. Phil. Trans. R. Soc. B 368 (1621), 20130119. doi:10.1098/rstb.2013.0119
Vitt, D. H. (2006). “Functional Characteristics and Indicators of Boreal Peatlands,” in Ecological Studies. Editors R. K. Wieder, and D. H. Vitt, 9–24.
Wang, M., Moore, T. R., Talbot, J., and Richard, P. J. H. (2014). The cascade of C:N:P Stoichiometry in an Ombrotrophic Peatland: from Plants to Peat. Environ. Res. Lett. 9 (2), 024003. doi:10.1088/1748-9326/9/2/024003
Wang, M., Moore, T. R., Talbot, J., and Riley, J. L. (2015). The Stoichiometry of Carbon and Nutrients in Peat Formation. Glob. Biogeochem. Cycles 29 (2), 113–121. doi:10.1002/2014gb005000
Warren, M. J., Lin, X., Gaby, J. C., Kretz, C. B., Kolton, M., Morton, P. L., et al. (2017). Molybdenum-Based Diazotrophy in a Sphagnum Peatland in Northern Minnesota. Appl. Environ. Microbiol. 83 (17), 14. doi:10.1128/aem.01174-17
Waughman, G. J., and Bellamy, D. J. (1980). Nitrogen Fixation and the Nitrogen Balance in Peatland Ecosystems. Ecology 61 (5), 1185–1198. doi:10.2307/1936837
Wieder, W. R., Cleveland, C. C., Smith, W. K., and Todd-Brown, K. (2015). Future Productivity and Carbon Storage Limited by Terrestrial Nutrient Availability. Nat. Geosci 8 (6), 441–444. doi:10.1038/ngeo2413
Witty, J. F. (1979). Acetylene Reduction Assay Can Overestimate Nitrogen-Fixation in Soil. Soil Biol. Biochem. 11 (2), 209–210. doi:10.1016/0038-0717(79)90103-2
Wray, H. E., and Bayley, S. E. (2007). Denitrification Rates in Marsh Fringes and Fens in Two Boreal Peatlands in Alberta, Canada. Wetlands 27 (4), 1036–1045. doi:10.1672/0277-5212(2007)27[1036:drimfa]2.0.co;2
Xu, J., Morris, P. J., Liu, J., and Holden, J. (2018). PEATMAP: Refining Estimates of Global Peatland Distribution Based on a Meta-Analysis. Catena 160, 134–140. doi:10.1016/j.catena.2017.09.010
Young, D. M., Baird, A. J., Charman, D. J., Evans, C. D., Gallego-Sala, A. V., Gill, P. J., et al. (2019). Misinterpreting Carbon Accumulation Rates in Records from Near-Surface Peat. Sci. Rep. 9, 8. doi:10.1038/s41598-019-53879-8
Yu, Z. (2011). Holocene Carbon Flux Histories of the World's Peatlands. The Holocene 21 (5), 761–774. doi:10.1177/0959683610386982
Yu, Z. C. (2012). Northern Peatland Carbon Stocks and Dynamics: a Review. Biogeosciences 9 (10), 4071–4085. doi:10.5194/bg-9-4071-2012
Yu, Z., Loisel, J., Brosseau, D. P., Beilman, D. W., and Hunt, S. J. (2010). Global Peatland Dynamics since the Last Glacial Maximum. Geophys. Res. Lett. 37, a–n. doi:10.1029/2010gl043584
Keywords: peatland, BNF, nitrogen fixation, nitrogen accumulation, nitrogen stock, nitrogen cycle
Citation: Yin T, Feng M, Qiu C and Peng S (2022) Biological Nitrogen Fixation and Nitrogen Accumulation in Peatlands. Front. Earth Sci. 10:670867. doi: 10.3389/feart.2022.670867
Received: 22 February 2021; Accepted: 14 January 2022;
Published: 17 February 2022.
Edited by:
Annalea Lohila, University of Helsinki, FinlandReviewed by:
Zicheng Yu, Lehigh University, United StatesPertti Juhani Martikainen, University of Eastern Finland, Finland
Copyright © 2022 Yin, Feng, Qiu and Peng. This is an open-access article distributed under the terms of the Creative Commons Attribution License (CC BY). The use, distribution or reproduction in other forums is permitted, provided the original author(s) and the copyright owner(s) are credited and that the original publication in this journal is cited, in accordance with accepted academic practice. No use, distribution or reproduction is permitted which does not comply with these terms.
*Correspondence: Shushi Peng, c3BlbmdAcGt1LmVkdS5jbg==