Possible Tsunami-Induced Sediment Transport From Coral Reef to Deep Sea Through Submarine Canyons on the Southern Ryukyu Forearc, Japan
- 1Research Institute of Geology and Geoinformation, Geological Survey of Japan, National Institute of Advanced Industrial Science and Technology (AIST), Tsukuba, Japan
- 2Research Institute of Marine Geodynamics, Japan Agency for Marine-Earth Science and Technology (JAMSTEC), Yokosuka, Japan
- 3Japan NUS Co., Ltd., Tokyo, Japan
- 4Japan Oil, Gas and Metals National Corporation (JOGMEC), Chiba, Japan
Submarine canyons are efficient sediment transport pathways from shallow marine areas to deep sea. Along active margins, large tsunamis are a trigger for sediment transport to deep sea. However, sediment transport through submarine canyons by such extreme wave events in the carbonate depositional environments has not been fully understood. Large tsunamis have repeatedly struck the coral reef islands of the southern Ryukyu Islands and have transported large boulders composed of coral from the reef to shore. In this study, we examined sediment cores collected near the mouths of submarine canyons and basin floor on the southern Ryukyu arc’s forearc. The presence of coarse calciturbidites containing coral, molluskan fragments, and coral reef benthic foraminifera was limited on the submarine fan formed at the mouth of the reef-connected and shelf-incised submarine canyon. In cores collected near the mouth of shelf-incised submarine canyons with no reef connection and slope-confined canyons, no coarse calciturbidite is observed. Few calciturbidites were found in more downslope cores, implying that most calcareous grains derived from shallow marine areas were deposited on this fan. Depositional intervals of turbidites were calculated to be several hundred–a few thousand years, which agree with the recurrence intervals of large tsunamis estimated from onshore tsunami deposits, based on radiocarbon dates in hemipelagic mud intervals. No temporal change in the depositional intervals of calciturbidites in the cores from the submarine fan since the last glacial maximum. Therefore, the tsunamis may be an important mechanism for surface sediment reworking in coral reefs, and shelf-incised and reef-connected submarine canyons can play an important role in the efficient transport of shallow marine calcareous grains to the deep sea.
Introduction
Submarine canyons have been recognized as preferential sediment transport pathways from continental margins to deep sea (e.g., Gardner, 1989; Xu et al., 2002, 2010; Palanques et al., 2005; Liu et al., 2016). Erosion and deposition by sediment gravity flows are the main overarching processes in submarine canyons (e.g., Pickering and Hiscott, 2016). However, modern submarine canyons in carbonate settings have been poorly understood because most submarine canyon studies focused on the siliciclastic depositional environments (Harris and Whiteway, 2011). Sediment production in carbonate settings is highest when the carbonate platform is flooded during high sea-level; sediment is commonly produced in excess of the available accommodation on the platform, and excess sediment is exported to the deep-sea (Mullins, 1983; Eberli, 1991). This differs from the siliciclastic system, in which the maximum export to the deep-sea commonly occurs during the low sea-level stands. Further, Eberli (1991) showed that sediment export from carbonate platform rims, either along a volcanic island or along a continental shelf differs from that from pure carbonate platforms. Several studies on submarine canyons and their role in sediment transport in pure carbonate and mixed carbonate-siliciclastic depositional settings along passive margins such as Bahamas, Great Barrier Reef and Mozambique Channel have been conducted (Grammer and Ginsberg, 1992; Jorry et al., 2010, 2020; Mulder et al., 2012a, b, 2017; Webster et al., 2012; Puga-Bernabéu et al., 2014; Wunsch et al., 2017; Tournadour et al., 2017; Counts et al., 2018, 2021). However studies on carbonate sediment export to the deep-sea, from small reefs surrounding a small island along an active margin are still limited. More acquisition of knowledge is therefore necessary to better understand the role of submarine canyons in source-to-sink in carbonate environments.
Tsunamis have been repeatedly generated by interplate mega-earthquakes along active plate margins. Large earthquakes and tsunamis have enough potential to disturb sea floor, remobilize surface sediments and form submarine earthquake- and tsunami-induced event deposits (e.g., Ikehara and Usami, 2018). Recent studies have suggested that tsunami-induced sediment resuspension and tsunami backwash can transport shallow marine sediments to the deep sea (Feldens et al., 2009; Ikehara et al., 2021a). Tsunami-induced turbidity currents by the 2011 Tohoku-oki earthquake were observed by instrumental records at two sites off Sanriku upper slope (Arai et al., 2013) and tsunami-induced turbidites were recognized on the outer shelf–upper slope (Ikehara et al., 2014, 2021b; Usami et al., 2017) along the Japan Trench. In the tropical-subtropical coastal zones, large tsunamis transported meter-sized coral boulders and calcareous coral reef sediments onshore (e.g., Goto et al., 2010; Fujiwara et al., 2020). Therefore, tsunamis can significantly disturb the seafloor and remobilize coral reef sediments. Notwithstanding a few studies in ancient environments (e.g., Puga-Bernabéu et al., 2007), our knowledge on tsunami-induced sediment transport from the shelf to deep sea, especially in carbonate settings, is insufficient because of the limited number of post-tsunami offshore surveys. Composition of carbonate sediments in the southern Ryukyu arc show zonal distribution with water depths (e.g., Tsuji, 1993), providing a unique opportunity to trace the sediment transport from a shallow marine reef (source) to deep sea (sink).
The southern Ryukyu arc is located in a subtropical region, and small islands in the arc are surrounded by coral reefs (Hori, 1977; Hori and Kayanne, 2000). Large tsunamis have repeatedly struck these islands with 500–1,000 years intervals (Kawana and Nakata, 1994; Ando et al., 2018; Fujita et al., 2020) from studies of onshore sandy tsunami deposits and tsunami boulders, although Araoka et al. (2013) inferred 150–400 years intervals during the last 2,400 years. The latest tsunami was the Meiwa tsunami in 1771, which had run-up heights of 30 m and approximately 12,000 deaths (Goto et al., 2010). Kitamura et al. (2018) estimated that the sizes of the two tsunamis that occurred before the 1771 event were comparable to the 1771 tsunami. However, because of the limited distribution of onshore tsunami deposits, long-term evaluation on tsunami recurrence are not available. There are several submarine canyons along the forearc slope of the southern Ryukyu. The heads of some canyons are near the coral reef around the southern Ryukyu islands. Ujiié et al. (1997) indicated a frequent occurrence of turbidites, which include coral reef species of benthic foraminifera in a core collected from a submarine fan in a pioneer study on sedimentation on the forearc slope. This suggests that this slope is a potential area for the detailed analysis of the source-to-sink sediment transport related to the tectonic events, such as large tsunamis and earthquakes, and for examination of response of sediment export from coral reef to the deep-sea to sea-level variations in this carbonate setting.
In this study, we examine sediment cores collected from the forearc slope to the basin area, off the southern Ryukyu arc, determine the spatiotemporal distribution pattern of calciturbidites in these cores, and discuss the source-to-sink movement of the carbonate sediment and the role of the submarine canyons in the transport of coral reef sediments to deep sea.
Southern Ryukyu Arc
The Ryukyu subduction zone extends >1,200 km from Kyushu to Taiwan (Figure 1). At the southern Ryukyu Trench, the Philippine Sea plate subducts northwestward beneath the Eurasian plate at a rate of >10 cm/yr (Taylor and Goodliffe, 2004; Argus et al., 2011). However, only two great interplate earthquakes (Mw > 8) have occurred along the Ryukyu Trench in the last 300 years; the 1771 Yaeyama earthquake at the southern Ryukyu and the 1911 earthquake off Kikai Island at the central Ryukyu (Usami, 2003; Ando et al., 2009), implying that overall plate coupling is weak (Peterson and Seno, 1984). Recent geodetic, geophysical, and seismological observations, such as the occurrence of slow slip events, low- and very-low-frequency earthquakes, and small repeating earthquakes, which support the weak coupling, suggest fluids are present at the plate boundary (Heki and Kataoka, 2008; Igarashi, 2010; Ando et al., 2012; Nishimura, 2014; Nakamura and Sunagawa, 2015; Arai et al., 2016). The Meiwa tsunami in 1771 was generated by either a tsunami earthquake, which assumed a slip on the plate boundary along a trench (Nakamura, 2009) or a submarine landslide induced by an intraplate earthquake (Imamura et al., 2001, 2008; Miyazawa et al., 2012). Another possible source is splay faults branching from the plate boundary (Hsu et al., 2013; Arai et al., 2016). Recently, Okamura et al. (2018) proposed an accretionary prism collapse as a new hypothesis for giant tsunami generation. However, an exact mechanism to produce such a large tsunami in 1771 remains controversial.
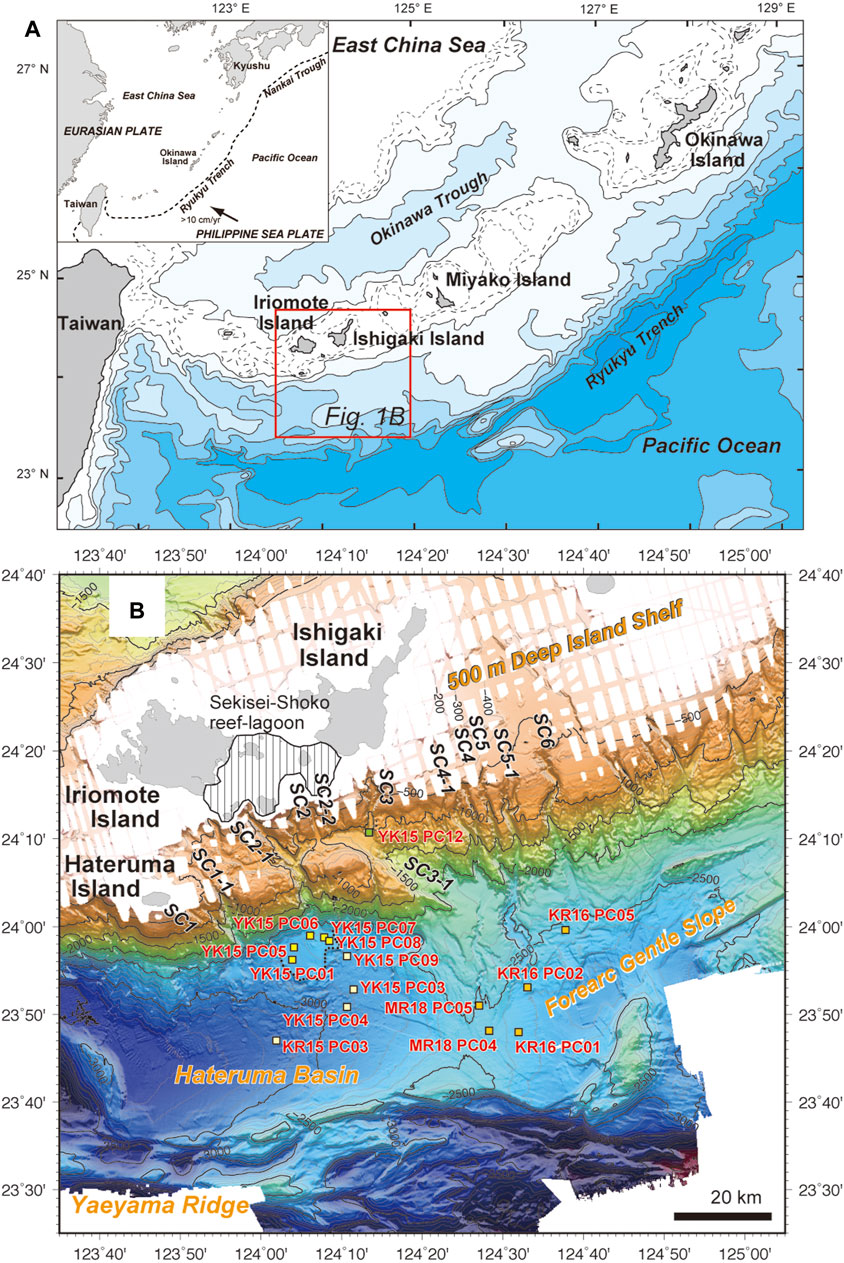
FIGURE 1. Location (A) and bathymetry (B) of the study area with piston coring locations. SC means submarine canyon listed in Supplementary Table S4.
The southern Ryukyu Islands have a subtropical climate, and fringing coral reefs have developed around the islands. The development of modern coral reefs in the southern Ryukyu Islands began around 10–11 ka (Hori and Kayanne, 2000). The Sekisei-Shoko reef-lagoon between Ishigaki and Iriomote islands (Figure 1) is the largest reef in Japan. The Ryukyu arc extends NE-SW and changes direction southeast of Ishigaki Island to ESE-WNW direction (Figure 1). Trench-parallel right-lateral tectonic movements due to the oblique subduction along the Ryukyu Trench has developed a complex geomorphic structure in this forearc area (Lallemand et al., 1999). The southern Ryukyu forearc has a narrow shelf, a wide forearc basin, a deep and flat trench floor, separated by steep slopes (Figure 1). A wide terrace with a water depth of 200–500 m, which is called a “500 m deep island shelf” (Ujiié, 1980), is found between Iriomote and Miyako islands. A steep slope exists between the wide terrace and forearc basins and gentle slope. The water depth of the base of the steep slope is shallower to the east (∼2,200 m), than to the west (∼2,500–2,800 m) of ∼124°25′E. Many submarine canyons and gullies occur in the slope. At least six major submarine canyons (SC1 to SC6 from west to east) incise the slope between 123°50′E and 124°40′E. In some canyons, a major canyon diverges into a few branches, which are called as SC*-1 to SC*-2 from west to east (Figure 1 and Supplementary Table S4). Several forearc basins occur between Taiwan and Ishigaki Island with ESE-WNW direction. The Hateruma Basin, with its water depth of 3,400–3,500 m, is the easternmost forearc basin located to the south of Iriomote Island. The northern margin of the Hateruma Basin is a steep slope with small gullies and submarine canyons (Figure 1B). The direction of the forearc slope changes at ∼124°25′E from ESE-WNW to E-W in the west to NE-SW in the east according to the bending of the Ryukyu arc. No forearc basin exists eastward of the arc-bending point, but a wide forearc gentle slope, which tilts southward, occurs. The Yaeyama Ridge is a topographic high that extends the E-W direction along the offshore margin of the forearc basins.
Materials and Methods
We obtained 15 sediment cores from the forearc of the southern Ryukyu Islands during YK15-01 (YK15) cruise of R/V Yokosuka, KR15-18 (KR15) and KR16-E06 (KR16) cruises of R/V Kairei and MR18-01C (MR18) cruise of R/V Mirai of JAMSTEC (Figure 1 and Supplementary Table S1) using a piston corer; 5 cores (cores YK15 PC01, PC05, PC06, PC07, and PC08) from a submarine fan near the mouth of SC2 (Kuroshima submarine canyon), 4 cores (cores YK15 PC03, PC04, PC09, and KR15 PC03) from the forearc (Hateruma) basin floor [note; core KR15 PC03 near the mouth of SC1 (Hateruma submarine canyon)], 1 core (core YK15 PC12) at the mouth of SC3 (Shiraho submarine canyon), 2 cores (cores MR18 PC04 and PC05) at the mouth of SC4, 2 cores (cores KR16 PC01 and PC02) at the mouth of SC5 (Nishi-Ishigaki submarine canyon) and 1 core (core KR16 PC05) at the mouth of SC6 (Higashi-Ishigaki submarine canyon). Cores ranging in length from ∼1.8 to 5.0 m were recovered (Supplementary Table S1). The cores were cut vertically and one-half of the cut surface was visually logged. Sediment colors were determined using Revised Standard Soil Color Charts (Oyama and Takehara, 1967). To observe detailed sedimentary structures, two-dimensional (2D) soft-X radiographs were taken from 1 cm-thick slab samples. The other half of the cores was sampled continuously using a standard paleomagnetic cube (7 cm3). The magnetic susceptibility of samples was measured using a KLY-4 magnetic susceptibility meter (Agico Co.). Accelerator mass spectrometry radiocarbon age-determinations using single species (Globigerinoides sacculifer) or mixed planktonic foraminifera in hemipelagic mud (bioturbated silt) intervals were conducted for cores YK15 PC01 (10 horizons), PC05 (14 horizons), PC07 (12 horizons), PC08 (15 horizons), PC09 (4 horizons), KR15 PC03 (10 horizons) and KR16 PC02 (8 horizons) (Figure 2 and Supplementary Table S2). Radiocarbon dating was conducted at Beta Analytics Co. Ltd. Obtained radiocarbon ages are calibrated to calendar ages using Calib8.20 calibration program with Marine 20 calibration curve (Heaton et al., 2020) and local marine reservoir value of 35 ± 25 years, which was obtained for coral on Ishigaki Island (Hideshima et al., 2001). Benthic foraminiferal assemblage analysis was also conducted at the selected intervals both from calcareous sand layers and hemipelagic mud of cores YK15 PC01 (11 calcareous sands and 4 hemipelagic muds) and PC08 (10 calcareous sands and 6 hemipelagic muds) (Supplementary Table S3) at Palynosurvey Co. Ltd. Samples were washed gently through a 63-µm sieve. The residues were observed under stereoscopic microscopy to perform planktonic foraminiferal tests as well as count and identify approximately 200 individuals of benthic foraminifera.
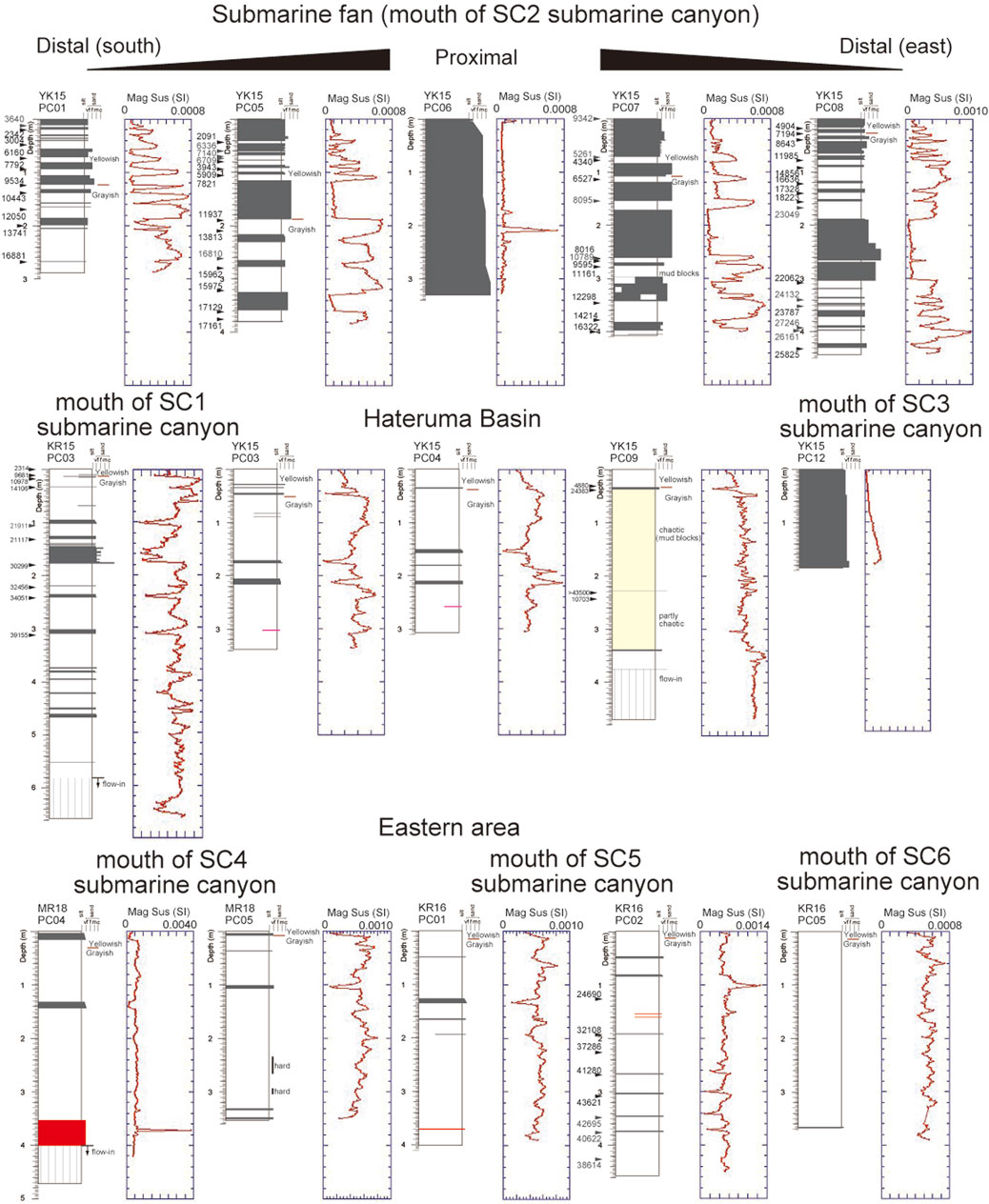
FIGURE 2. Log section, magnetic susceptibility profile, and radiocarbon dates of each core. Lithology: calcareous sand–coarse silt (gray), bioturbated silt (white), chaotic mud (yellow), and tephra (red).
Bathymetric surveys were conducted during cruises YK15-01 of R/V Yokosuka, KR15-18 and KR16-E01 of R/V Kairei and MR18-01C of R/V Mirai. Bathymetric data were collected using multibeam echosounder SeaBeam 2012 and Kongsberg EM 122 at 12 kHz frequency. Seafloor bathymetry obtained during the cruises was reported by Kanamatsu et al. (2020). We also used the existing bathymetric data collected during the GH17 and GH18 cruises by the Geological Survey of Japan (GSJ), AIST.
Results
Seafloor Morphology of the Slope and Submarine Canyons
The slope between Ishigaki Island (∼124°40′E) and Iriomote Island (∼123°50′E) is steep and its average gradient ranges from 5° to 14°. On this slope, at least six major submarine canyons can be found (Figure 1 and Supplementary Table S4). A small N-S trending topographic high occurs offshore of Ishigaki Island at ∼124°25′E, where the southern Ryukyu arc bends, and the morphology of the slope is different between the east and west of the topographic high. To the east of the topographic high, the upper slope (500–2,200 m in water depth) has an average gradient of 5°–7° with some canyons. Gentle slope (2,200–2,700 m in water depth and 2°–3° in average gradient) with a few canyons exist at the foot of the steep slope, and a wide and gentle slope with its water depth of 2,500–2,800 m exists offshore. To the west of the topographic high, a steep slope and a wide forearc basin (Hateruma Basin) are prominent features. The steep slope has its base at 2,500–2,800 m in water depth and an average gradient of ∼12°–14°. No gentle slope exists at the northern slope of the eastern part of Hateruma Basin, but a gentle slope exists between the foot-of-steep slope and the forearc basin floor at the western part. A submarine fan with a water depth of 2,600–2,800 m can be found at the mouth of SC2 submarine canyon south of Iriomote Island. The surface of the fan is smooth and gently slopes toward the SW. Kanamatsu et al. (2020) indicated the occurrence of submarine landslides and scattered blocks of a few km in length in the eastern margin of the fan.
Many submarine canyons and gullies incise the steep slopes. Submarine canyons have low sinuosity and incise the slope ∼200–600 m (Supplementary Table S4). Submarine canyons in the east are slope-confined canyons (Puga-Bernabéu et al., 2011), which do not breach the shelf-break. Canyon heads locate at ∼500–550 m in water depths near the edge of “500 m deep island shelf” (Ujiié, 1980). Canyon heads become shallower toward the west and canyon incises the shelf break (∼140 m) south of Ishigaki Island (SC3 canyon). Canyon heads locate at ∼50–60 m in the south of Sekisei-Shoko reef-lagoon (SC2-1 (Aragusukushima submarine canyon) and SC2 canyons). Canyons in the west (SC2-1 and SC2 canyons) are partly blocked by reefs and classified as shelf-incised and partly reef-blocked canyons (sensu Puga-Bernabéu et al., 2011). The westernmost two canyons (SC1 and SC1-1 canyons) are slope-confined canyons, whose heads locate at ∼200–300 m. Reflecting the steeper upper slope of the western side and the occurrence of the gentle lower slope at the foot-of-steep-slope of the eastern area, the submarine canyons are longer in the eastern area than in the western area. However, SC3 canyon is short and open to a small basin at the middle of forearc slope. Extension of the submarine canyon (SC3-1 canyon) incises the lower part of the slope. The longest submarine canyon (SC4) occurs at the eastern slope of the topographic high and has ∼50 km in length. The submarine canyons at the steep slope are narrow with 0.8–2 km in widths but relatively wider and flatter floor in the western canyons (SC2-1, SC2, SC2-2, and SC3). SC5 canyon has incised the lower slope larger than those of SC4 and SC6 canyons.
Sediment Core Lithology, Sedimentary Structures and Sediment Compositions
All collected cores are mainly composed of yellowish-gray–grayish olive slightly calcareous and bioturbated silt intercalated with yellowish-orange calcareous very fine to coarse sand layers (Figure 2). Bioturbated silt of the uppermost part of all cores is yellowish gray, but those of the other parts are grayish olive. The thickness of the calcareous sand layers ranges from a few cm to >300 cm. Most sand layer exhibits parallel- or cross-lamination with a sharp basal contact and a fining-upward grading. Sandy grains are mainly composed of calcareous bioclasts such as fragments of molluskan shells, corals, bryozoa, and benthic and planktonic foraminifera. Preservation of benthic foraminifera is moderate, being slightly better in bioturbated silts and fine calcareous sand layers than in coarse calcareous sand layers (Supplementary Table S3). Benthic foraminiferal assemblages of sandy layers of cores YK15 PC01 and PC08 contain shallow-water species (S) of Inoue (1980a, b), which mainly live in shallow water <200 m water depth, such as Quinqueloculina spp., Textularia spp., Miliolinella spp., and Glabratella spp., and coral reef species (C) of Fujita (2013), such as Acervulina spp., Calcarina spp., Amphisorus sp., Operculina spp., and Dendritina striata, and are different from those of bioturbated silt, which contains the middle and deep-water species (M-D) of Inoue (1980a, b), such as Globocassidulina subglobosa, Globocassidulina spp., Bolivina decussata, Cassidulina carinata, and Gyroidina spp. (Figure 3 and Supplementary Table S3). Coral reef species occur in a higher percentage in calcareous sand layers of medium–coarse sand size than in calcareous sand layers of fine sand size. The calcareous sand have a composition in agreement with a shallow marine and coral reef origin (Figure 3 and Supplementary Table S3). The sedimentary structures show that the calcareous sand layers are calciturbidites (division Tb–Tc of Bouma, 1962) of shallow marine origin. The upper boundary of each sand layer is sharp and no or little turbidite mud without bioturbation occurs above the calcareous sand layer. On the contrary, the bioturbated nature and benthic foraminiferal assemblages of the similar water depths to the coring sites in slightly calcareous silt show that the bioturbated silt is hemipelagic mud deposited at the coring place.
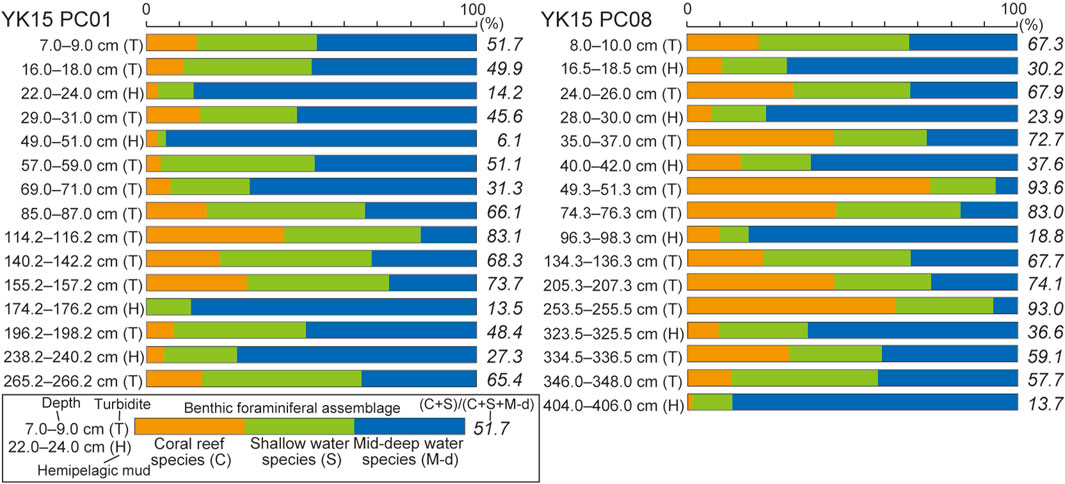
FIGURE 3. Ratios of coral reef–shallow water–mid-deep water benthic foraminiferal species in calciturbidite (T) and hemipelagic muds (H) of cores YK15 PC01 and PC08.
The frequent occurrence of calcareous sand layers (calciturbidites) is characteristic of cores from the submarine fan (cores YK15 PC01, PC05, PC07 and PC08). The frequency and thickness of sand layers in the upper part of cores YK15 PC01 and PC05 is higher than those in the lower part, whereas those in the upper part of core YK15 PC08 is lower than those in the lower part (Figure 2). Instead, frequency is low in the Hateruma Basin floor cores in cores YK15 PC03, 04, PC09, and KR15 PC03, and in the forearc gentle slope cores (cores KR16 PC01, PC02, PC05, MR18 PC04, and PC05) (Figure 2). Sands are coarser and layers are thicker in the submarine fan cores than in the forearc basin and gentle slope cores. Thick calcareous sand with a fining-upward grain-size trend from coarse sand at the base to very fine sand at the top occurs in core YK15 PC06 from SC2 canyon mouth on the submarine fan (Figure 2). A similar thick calcareous sand layer with a fining-upward grain size trend occurs in core YK15 PC12 from SC3 canyon mouth of the upper slope (Figure 2).
Magnetic Susceptibility
Values of the measured volumetric magnetic susceptibility range from ×0.3 E-04 to 1.3 × E-03 (Figure 2). Calcareous sand layers have magnetic susceptibility of <∼2 × E-04 and show negative peaks, indicating lower content of magnetic minerals than bioturbated silt (magnetic susceptibility of >∼4 × E-04) despite their coarser grain size (Figure 2). Therefore, it is easy to distinguish calcareous sand layers from the background (hemipelagic) bioturbated silt.
Radiocarbon Dates
In seven cores, 73 radiocarbon dates were obtained: cores YK15 PC01 (10 horizons), PC05 (14 horizons), PC07 (12 horizons), PC08 (15 horizons), PC09 (4 horizons), KR15 PC03 (10 horizons) and KR16 PC02 (8 horizons) (Figure 2 and Supplementary Table S2). Although some overturns in age are probably due to a mixture of reworked, older foraminifera from the calcareous sand layers by benthic organisms, the obtained radiocarbon dates generally become older with increasing sub-bottom depths. Assuming no erosion at the base of the sand layer and constant sedimentation within hemipelagic mud (bioturbated silt), the averaged sedimentation rates of hemipelagic mud are calculated as ∼10 cm/ky (6.8 cm/ky for core YK15 PC07 to 11.8 cm/ky for core YK15 PC01) throughout the study area. Recurrence intervals of calcareous sand layers are calculated as 46–2,857 years (average: 1,042 years) for core YK15 PC01, 126–3,572 years (1,556 years) for core YK15 PC05, 1,608–4,760 years (2,850 years) for core YK15 PC07, 73–3,180 years (1,169 years) for core YK15 PC08, 86–5,517 years (2,103 years) for core KR15 PC03 and 1745–11851 years (4,987 years) for core KR16 PC02, respectively (Figure 4 and Supplementary Table S5).
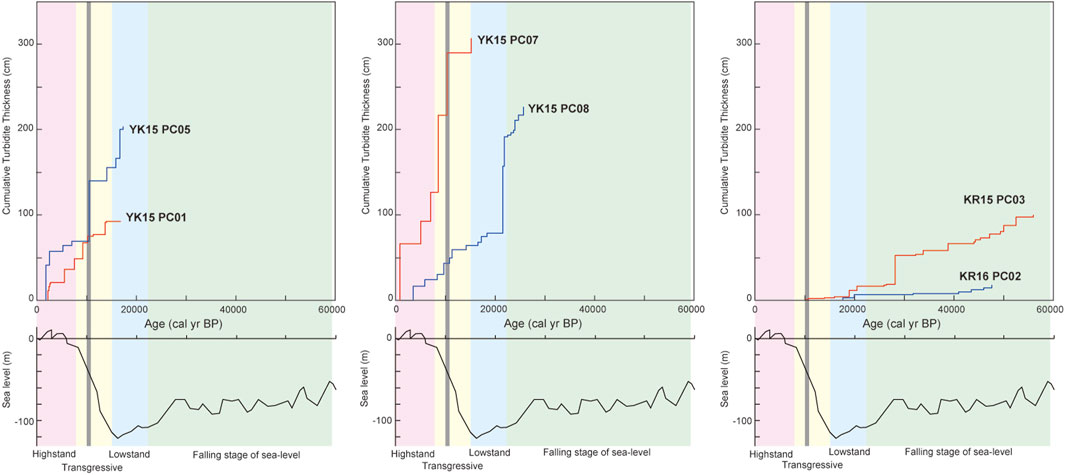
FIGURE 4. Turbidite accumulation in cores YK15 PC01 and PC05 on the southern part of submarine fan at mouth of SC2 (Kuroshima) submarine canyon, in cores YK15 PC07 and PC08 on the eastern part of submarine fan, in core KR15 PC03 near the mouth of SC1 (Hateruma) submarine canyon and in core KR16 PC02 near the mouth of SC4 submarine canyon. Sea-level curve and periods of depositional stages (highstand shown in pink, transgressive in yellow, lowstand in light blue and falling sea-level stage in yellowish green) in the East China Sea shelf are after Saito et al. (1998). Gray band indicates onset of the development of the Holocene coral reefs in the southern Ryukyu islands (Hori and Kayanne, 2000).
Discussion
Connectivity of Submarine Canyon Heads With Coral Reefs and Sediment Core Lithology
Sediment core lithology around submarine canyon mouths of the southern Ryukyu forearc varies depending on the connectivity of submarine canyon heads with coral reefs. In pure carbonate and mixed carbonate–siliciclastic settings, sediment exports from shallow carbonate platforms and reefs to the deep-sea is controlled by the carbonate and clastic sediment productivity, type of canyon, and connectivity of shelf channel with the submarine canyon (Mullins, 1983; Eberli, 1991; Jorry et al., 2010, 2020; Puga-Bernabéu et al., 2011, 2014; Counts et al., 2018, 2021). First, we will examine the relationship between sediment core lithology and the type and connectivity of submarine canyons. In the eastern part of the study area, where the submarine canyons are slope-confined and the canyon heads lie at ∼500–550-m water depths far from modern coral reef distribution (Figure 1), only several thin (a few cm order) and fine-grained (very fine sand–coarse silt-sized) calciturbidites occur in the cores (Figure 2). These sand layers are mainly composed of fine-carbonate grains such as planktonic foraminifera, and lack large bioclasts such as coral, bryozoa, and molluskan (bivalve) fragments. On the contrary, sediment cores from a submarine fan at mouth of SC2 canyon, which is shelf-incised and partly reef-blocked, in the western part of the study area contain several thick (several cm–m scale) and coarse-grained (fine sand–coarse sand-sized) calciturbidites (Figures 2, 5A,5B). In the Great Barrier Reef, the shelf-connection type of submarine canyons influences the type of sedimentary gravity flow deposits (Puga-Bernabéu et al., 2011, 2014). Although SC2-1 and SC2 canyons belong to shelf-incised and partly reef-blocked canyon, sedimentary dynamics are more complicated owing to the multiple sediment sources, no shelf channels connected to the land in the Sekisei-Shoko lagoon, and low clastic sediment supply from nearby small islands may contribute to form carbonate deposits at the mouth of these canyons. The frequency and thickness of calciturbidites in core KR15 PC03 collected near SC1 canyon mouth is larger than in cores collected from the eastern forearc gentle slope (cores KR16 PC01, PC02, PC05, MR18 PC04, and PC05) but smaller than in cores from the submarine fan (cores YK15 PC01, PC05, PC07, and PC08) (Figure 2). SC1 canyon head is located near the Hateruma Island coral reef based on a bathymetric map “Hateruma Sima” (Japan Coast Guard, 1988). This suggests that distance between canyon head and sediment source (coral reef) plays some roles on sediment transport to deep-sea through submarine canyon.
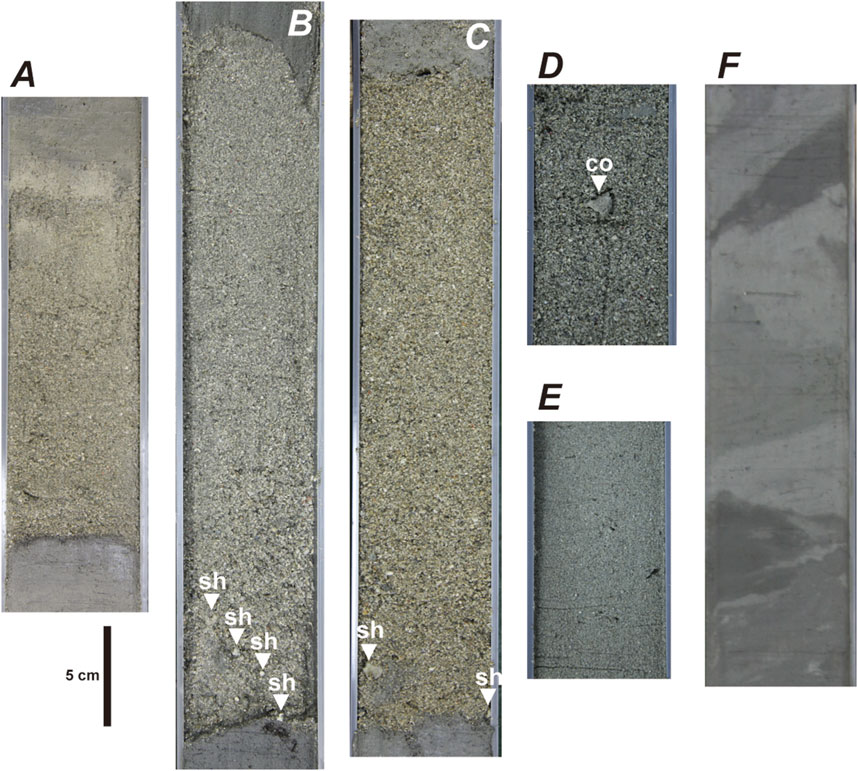
FIGURE 5. Typical examples of calciturbidite (A–E) and chaotic mass-transported deposit facies (F) in the obtained cores. (A) fining-upward calcareous medium–very fine sand with a sharp base (core YK15 PC01 at 100.2–126.2 cm), (B) fining-upward calcareous coarse–fine sand with a sharp base (core YK15 PC08 at 267.5–305.5 cm), (C) fining-upward calcareous medium–coarse sand with shell fragments (core YK15 PC07 at 117.5–154.5 cm), (D) calcareous medium–coarse sand with a coral fragment (core YK15 PC06 at 286.5–299.5 cm), (E) calcareous very fine sand (core YK15 PC12 at 132.2–145.2 cm), (F) varicolored chaotic silt (core YK15 PC09 at 287.3–312.3 cm). sh: shell fragment, co: coral fragment.
Next, we will examine the relationship between composition of calciturbidites and the connectivity of submarine canyon heads with coral reefs. In the western part of the study area, molluskan (bivalve and gastropoda) and bryozoa fragments and benthic foraminifera are common (Figures 3, 5) whereas coral fragments rarely occur in coarse–medium sand-sized calciturbidites (Figure 5) in the cores collected from the submarine fan at SC2 canyon mouth. Molluskan fragments and benthic foraminifera are also found in fine–medium sand-sized calciturbidites (Figure 5). Benthic foraminiferal assemblages in calciturbidites consist mainly of coral reef (C) and shallow-water (S) species with a low percentage of middle-deep-water (M-D) species (>60% in (C + S)/(C + S + M-D) ratio). A larger number of coral reef species is observed in the coarse–medium sand-sized calciturbidites than in the very fine–fine sand-sized calciturbidites (Figure 3). Ujiié et al. (1997) also reported the occurrence of coral reef benthic foraminifera in calciturbidites in the Holocene sequence of a core collected from the same fan. These benthic foraminiferal assemblages indicate that these calciturbidites are shallow water-origin, and the coral reef is the most likely source of coarse calciturbidites. Instead, very fine–fine calcareous sands originate from shallow-water areas including the coral reefs. In the eastern part of the study area, no intercalation of coarse calciturbidites and a few fine calciturbidites occur in cores MR18 PC04 and PC05, KR16 PC01, PC02, and PC05 at the mouths of submarine canyons (SC4, SC5, and SC6) without connection to the coral reef. Although no precise report on the distribution and characteristics of surface sediments near the heads of submarine canyons exist, Tsuji (1993) showed a wide distribution of planktonic foraminiferal sand and mud at the water depths of 200–900 m around Miyako Island. Planktonic foraminiferal tests in surface sediments are the most likely origin of fine calciturbidite sands. Even in the western part, the sediment lithology of cores YK15 PC06 and PC12 at SC2 and SC3 submarine canyon mouths, respectively, suggest that the distance from the submarine canyon head and coral reef edge is a factor for the transport of coarse-grained coral reef materials to deep sea through the submarine canyon. The closer location of submarine canyon head to the coral reef for core YK15 PC06 [0.6 km at SC2-1, ∼1 km at SC2-2, and 1.4 km at SC2 based on the bathymetric map “Southern Part of Isigaki Sima” (Japan Coast Guard, 1992)] than for core YK15 PC12 [2.6 km at SC3] may be related to coarser grain-size nature (Figure 2) with many coral and molluskan bioclasts of core YK15 PC06 (Figure 5D) than core YK15 PC12 (Figure 5E). Therefore, the connectivity of submarine canyon heads with coral reefs is a possible factor for thick deposition of calcareous bioclasts at the deep-sea floor and efficient sediment transport from shallow- to deep sea through submarine canyons in the carbonate depositional system.
Reflecting such differences in frequency and thickness of calciturbidites transported through submarine canyons between the eastern and western parts of the study area, topography near the submarine canyon mouth is also different. No fan and lobe topography is observed near the two eastern submarine canyon (SC5 and SC6) mouths where a few thin and fine-grained calciturbidites are obtained (Figure 1). Only a distinct fan-shaped relief is found at the mouth of SC2 canyon where many thick and coarse-grained calciturbidites are obtained (Figure 2). Smooth surface of this fan shows an active deposition of sediments on the fan. Larger widths in the western submarine canyons (SC2-1, SC2, SC2-2, and SC3) than those in the eastern canyons (SC4-1, SC4, SC5, SC5-1, and SC6) may relate to larger coarse sediment transport through canyons and to larger lateral erosion of canyon walls in the western canyons than in the eastern canyons.
Temporal Change in the Frequency of Calciturbidites
Carbonate production on carbonate platform tops and sediment exports from platforms to surrounding deep seas by plumes and gravity flows have been influenced by late Quaternary large scale oscillations of sea level (e.g., Mullins 1983; Droxler and Schlager, 1985; Eberli, 1991; Jorry et al., 2010, 2020; Webster et al., 2012; Counts et al., 2021). In many studies, a general correlation has been found between an increase in calciturbidite frequency and periods of sea-level highstand (Droxler and Schlager, 1985; Jorry et al., 2010, 2020; Webster et al., 2012; Wunsch et al., 2017; Counts et al., 2018, 2021). However, the exact occurrence patterns of calciturbidites are variables, reflecting the bank-top water depths (the timing of the flooding of bank-top), type of submarine canyon, and shelf-edge morphology (Jorry et al., 2010; Puga-Bernabéu et al., 2014). Interestingly, the frequency of deposition, thickness, and grain size of calciturbidites in the four cores from the submarine fan (cores YK15 PC01, PC05, PC07 and PC08; Figures 2, 4) do not show a relationship with glacial-interglacial variability. For example, during the sea-level highstand, the frequency of calciturbidites is highest in cores YK15 PC01 and PC05 but lowest in core YK15 PC08 and is almost even in core YK15 PC07. This suggests that another control on coral reef sediment exports other than carbonate production exist in this carbonate system. Moreover, the frequency of calciturbidites is high before the last glacial maximum (LGM) and low in the Holocene in cores from the eastern part (core KR16 PC02) and Hateruma Basin (core KR15 PC03) (Figures 2, 4 and Supplementary Table S5). No and only three thin (<1 cm thick) Holocene calciturbidite occurs in cores KR16 PC02 and KR15 PC03, respectively. In core KR15 PC03 two and three calciturbidites are formed in early transgressive stage and in the lowest sea-level stand (LGM) with their recurrence intervals of ∼1,300–3,300 years. The frequency of calciturbidites (∼550–5,500 years intervals) in core KR15 PC03 is slightly high in the falling sea-level stage before the LGM. A high occurrence of calciturbidites is also found in the lower part of Hateruma Basin cores (cores YK15 PC03 and PC04), although there is no radiocarbon date in the two cores. A similar decrease of calciturbidite frequency in the LGM was reported from the Gulf of Papua, western Caribbean Sea, Bahamas (Jorry et al., 2010), and Mozambique Channel (Jorry et al., 2020). Lack of the Holocene turbidite in the eastern part and Hateruma Basin cores may reflect core top disturbance in the recovery of the piston cores and low sedimentation rates. Sea-level oscillation is a likely control on down-slope sediment transport along the forearc slope in the southern Ryukyu, except at the submarine fan. However, it is necessary to obtain many radiocarbon ages to examine the relationship between calciturbidite frequency and sea-level state in these basin floor cores.
The development of modern coral reefs in water depths shallower than 50–55 m in the middle–southern Ryukyu Islands began around 10–11 ka (Hori and Kayanne, 2000). Five to ten calciturbidites are found on the submarine fan after the development of modern coral reefs (Figure 4 and Supplementary Table S5). Coral reefs could form during the lowest-sea-level glacial period in southern Ryukyu (Sasaki et al., 2006). Although there is no report on the glacial coral reefs around the study area, the occurrence of coral reef materials in calciturbidites of the LGM to last deglaciation sequence in core YK15 PC08 show that mound-like (Webster et al., 2004; Sasaki et al., 2006) or small shelf-edge reef (Webster et al., 2018) had been formed near the submarine canyon head throughout the LGM to the last deglaciation.
Deposition of Coral Reef–Shallow-Water Origin Carbonate Sand on a Submarine Fan
The calciturbidites become thinner and finer-grained from proximal to distal locations on the submarine fan (Figure 2). The submarine fan has a radial in shape and has a sand-dominated character (Shanmugam and Moiola, 1988; Pickering and Hiscott, 2016). The thickest (>3 m) and coarsest (coarse–fine sand) turbidite occurs at SC2 canyon mouth in core YK15 PC06 (Figure 2). Sand thickness and grain size decrease quickly toward distally to a few tens cm in thickness and very fine–fine sand in cores YK15 PC01 and PC08, through several tens cm and medium–fine sand in cores YK15 PC05 and PC07. This shows that coarse-grained sand transported from the coral reef through the submarine canyon has been deposited near the canyon mouth according to rapidly decreasing flow velocity. A narrow topographic high at the submarine canyon mouth (Figure 1) may reflect such rapid deposition of coarse-grained materials on the upper fan.
Although a similar spatial change in sand thickness and grain size is found both toward east and south, temporal depositional patterns of calciturbidites on the eastern and southern parts of the fan are different. In the lower part of cores YK15 PC07 and PC08 on the eastern part of the fan, calciturbidites are thicker and coarser than those in the upper part of the cores (Figure 2). Contrarily, on the southern part of the fan (cores YK15 PC01 and PC05), calciturbidites are more frequent, coarser, and thicker in the upper part than in the lower part (Figure 2). Ujiié et al. (1997) reported the occurrence of 10 calciturbidites during the last ∼15 ky in a core collected near core YK15 PC01, and relatively low frequency before ∼10 ka. This trend is similar to our record in core YK15 PC01. This shows that the turbidite depocenter shifted on the submarine fan, at ∼11 cal ky BP (Figures 2, 4).
Almost no coarse calciturbidite occurs in cores collected from the forearc basin floor (cores YK15 PC03, PC04, and PC09) apart from core KR16 PC03, which was collected near SC1 canyon mouth. This means that most coarse calcareous materials transported from coral reefs through the submarine canyon have been deposited on the submarine fan. Therefore, canyon-connected submarine fan plays an important role in the deposition (sink) of coarse-grained materials from the coral reef. The rapid and thick accumulation of sediments on the fan at the foot of the slope can cause instability of the seafloor. Kanamatsu et al. (2020) reported the presence of a submarine landslide at the margin of the submarine fan. They also discussed the age of the submarine landslide as ∼7 ky BP in conventional radiocarbon date from thin-sandy layer (turbidite) in core YK15 PC03 based on the radiocarbon ages at the correlative horizon of the sand layer in core YK15 PC13. Interestingly, these thin calciturbidites with very fine–fine sand are traceable among the sediment cores collected from the Hateruma Basin floor near the horizon of sediment color change from yellowish to grayish (Figure 2). Although newly obtained radiocarbon dates in this study show that the sediment color change occurred at ∼7–9 cal ky BP (Figure 2), this turbidite deposition might be a basin-wide event in the Hateruma Basin. Another evidence on submarine landslide near the margin of the fan is the occurrence of a varicolored chaotic mud layer (mass-transported deposit; MTD), which occurred just below the sediment color change in core YK15 PC09 (Figures 2, 5F). Newly obtained radiocarbon dates in this core suggest that the MTD deposited before ∼5 cal ky BP and after ∼11 cal ky BP (Figure 2 and Supplementary Table S2). Secondary gravitational sediment transport from the fan to the peripheral basin floor may contribute to sediment dispersal of calcareous sediments in the deep-sea environments.
Repeated Transport of Coral Reef Calcareous Grains to a Submarine Fan
Transport of calcareous sand from the coral reef to deep sea through SC2-1 and SC2 submarine canyons has repeatedly occurred (Figures 2, 4). Large earthquakes and tsunamis are possible mechanisms for the transport of shallow marine sediments to the deep-sea (e.g., Feldens et al., 2009, 2012; Ikehara and Usami, 2018; Ikehara et al., 2021b). Especially in coral reefs, large tsunamis have enough potential to transport coral reef materials to both onshore (Goto et al., 2010; Kitamura et al., 2018; Fujita et al., 2020) and offshore (Feldens et al., 2009, 2012; Paris et al., 2010; Sukuna-Schwartz et al., 2015). Recurrence intervals of calciturbidite depositions in cores YK15 PC01, PC05, PC07, and PC08 are calculated as several hundred–a few thousand years (average: 1,388 years) (Figure 4 and Supplementary Table S5). The intervals are likely concordant with those (500–1,000 years) of large tsunamis as reconstructed from coral boulders and onshore tsunami deposits in the southern Ryukyu Islands (Kawana and Nakata, 1994; Ando et al., 2018; Kitamura et al., 2018; Fujita et al., 2020), and slightly larger than those (150–400 years) from coral boulders on Ishigaki Island (Araoka et al., 2013). Remobilization of coral reef sediments and collapse of reef slopes by strong earthquake ground shaking might be another mechanism for the transport of coral reef sediments to the deep-sea. Although there is no evidence on strong shaking, such as soft-sediment deformations in our cores, the possibility of the association between that large earthquakes and down-slope sediment transport in the study area cannot be denied. Intervals of ∼50–300 years may be too small as recurrence intervals of large tsunamis. The erosion of surficial hemipelagic mud during calciturbidite deposition is a likely mechanism to shorten the intervals. Another explanation is sediment transport by large typhoons. Typhoon-induced hyperpycnal flows and megarips may transport shallow marine materials to deep-sea (Liu et al., 2016; Porcile et al., 2020). Although no large hinterland and large river is expected in the small islands of the southern Ryukyu arc, extreme waves by large typhoon may remobilize coral reef calcareous sediments to deep-sea through reef-connected submarine canyons. However, the coincidence in the recurrence intervals suggests that a large tsunami is likely to trigger the mechanism of sediment transport from the coral reef to the deep sea through a reef-connected submarine canyon in southern Ryukyu. However, longer intervals in our turbidite records than those of onshore records from tsunami deposits and tsunami boulders show that not all large tsunami events have been recorded as turbidites in a core. The shift of turbidite depocenter may influence such an incomplete record in a core, because no turbidite might deposit when the depocenter moved far from the coring site. Moreover, no direct evidence on the 1771 Meiwa tsunami-induced calciturbidite is found in our cores. This may be due to core top disturbance during the piston corer recovery and thin surface sediment cover after the 1771 Meiwa tsunami because of the low background sedimentation rate of hemipelagic mud. Some studies suggested that submarine landslides play an essential role in generating large tsunamis in this region (Imamura et al., 2001, 2008; Miyazawa et al., 2012; Okamura et al., 2018). Based on the detailed bathymetry of the forearc slope, Kanamatsu et al. (2020) stated that there is no large submarine landslide along the forearc slope, except for a submarine landslide at the submarine fan’s margin. Based on our core data, there is a possibility that the submarine landslide was related to the deposition of a thin turbidite distributed basinwide, as discussed above. However, no other direct evidence to connect submarine landslides and turbidite depositions exists at present. In addition, we do not have any sediment data from lower slope and trench floor to determine the submarine landslides at the lower slope, as suggested by Okamura et al. (2018). We need further study to clarify the relationship between submarine landslides and turbidite depositions.
Conclusion
Bathymetry and sediment core data along the southern Ryukyu forearc indicate that coral reef-connected submarine canyons play an important role in the delivery of coarse calcareous sand from shallow water to the deep sea. The connection between the submarine canyon head with the coral reef (source) is a crucial factor for the efficiency of coarse sediment transport. The volume and frequency of coarse sediment transport through the submarine canyon decrease with the increase in the distance between the coral reef and the canyon head. Coarse calcareous sediment transported through the coral reef-connected submarine canyon were deposited as calciturbidites near the submarine canyon’s mouth, forming a submarine fan (sink) at the toe of the slope. Depocenters of calciturbidites on the fan has been shifted at ∼11 cal ky BP. The calciturbidites’ recurrence intervals (several hundred–a few thousand years) show that a large tsunami is the most likely trigger mechanism for sediment transport of coarse calcareous sands from the coral reef to the deep sea through submarine canyons. The lack of temporal changes in the recurrence intervals of the calciturbidites in the cores from the submarine fan since the LGM implies that tectonic-driven trigger mechanisms, such as large tsunamis, are a more important factor than climatic-controlled sediment production and supply from the coral reef, in this carbonate source-to-sink system in the southern Ryukyu forearc. This also suggests that, where the tsunami-induced turbidity currents could occur repeatedly, the tsunamis may contribute to maintain submarine canyons.
Data Availability Statement
The original contributions presented in the study are included in the article/Supplementary Material, further inquiries can be directed to the corresponding author.
Author Contributions
KI and TK projected the study and the survey cruises. KI and KU conducted sedimentological analysis. TK performed paleomagnetic and rock magnetic measurements. KI drafted the manuscript. All authors contributed to the data acquisitions and have read and approved the final manuscript.
Funding
This work was funded as part of the “Research Project for Compound Disaster Mitigation on the Great Earthquakes and Tsunamis around the Nankai Trough Region” by the Ministry of Education, Culture, Sports, Science and Technology of Japan.
Conflict of Interest
KU is employed by Japan NUS Co. Ltd.
The remaining authors declare that the research was conducted in the absence of any commercial or financial relationships that could be construed as a potential conflict of interest.
Publisher’s Note
All claims expressed in this article are solely those of the authors and do not necessarily represent those of their affiliated organizations, or those of the publisher, the editors and the reviewers. Any product that may be evaluated in this article, or claim that may be made by its manufacturer, is not guaranteed or endorsed by the publisher.
Acknowledgments
We thank the captains, crews, marine technicians and onboard scientists of the R/Vs Yokosuka (YK15-01), Kairei (KR15-18 and KR16-E01), and Mirai (MR18-01) for their efforts and supports in data collection. We also thank Dr. A. Misawa of the GSJ, AIST for providing us bathymetric data obtained by the survey cruises conducted by the GSJ, AIST, for Figure 1, and Dr. K. Arai of the GSJ, AIST for discussion on the glacial coral reefs in the southern Ryukyu Islands and carbonate depositional systems. We thank Dr. Á. Puga-Bernabéu and an anonymous reviewer for their constructive comments, and Enago for the English language review.
Supplementary Material
The Supplementary Material for this article can be found online at: https://www.frontiersin.org/articles/10.3389/feart.2022.753583/full#supplementary-material
References
Ando, M., Kitamura, A., Tu, Y., Ohashi, Y., Imai, T., Nakamura, M., et al. (2018). Source of High Tsunamis along the Southernmost Ryukyu Trench Inferred from Tsunami Stratigraphy. Tectonophysics 722, 265–276. doi:10.1016/j.tecto.2017.11.007
Ando, M., Nakamura, M., Matsumoto, T., Furukawa, M., Tadokoro, K., and Furumoto, M. (2009). Is the Ryukyu Subduction Zone in Japan Coupled or Decoupled? -The Necessity of Seafloor Crustal Deformation Observation. Earth Planet Sp. 61, 1031–1039. doi:10.1186/BF03352954
Ando, M., Tu, Y., Kumagai, H., Yamanaka, Y., and Lin, C.-H. (2012). Very Low Frequency Earthquakes along the Ryukyu Subduction Zone. Geophys. Res. Lett. 39, L04303. doi:10.1029/2011GL050559
Arai, K., Naruse, H., Miura, R., Kawamura, K., Hino, R., Ito, Y., et al. (2013). Tsunami-generated Turbidity Current of the 2011 Tohoku-Oki Earthquake. Geology 41, 1195–1198. doi:10.1130/G34777.1
Arai, R., Takahashi, T., Kodaira, S., Kaiho, Y., Nakanishi, A., Fujie, G., et al. (2016). Structure of the Tsunamigenic Plate Boundary and Low-Frequency Earthquakes in the Southern Ryukyu Trench. Nat. Commun. 7, 12255. doi:10.1038/ncomms12255
Araoka, D., Yokoyama, Y., Suzuki, A., Goto, K., Miyagi, K., Miyazawa, K., et al. (2013). Tsunami Recurrence Revealed by Porites Coral Boulders in the Southern Ryukyu Islands, Japan. Geology 41, 919–922. doi:10.1130/G34415.1
Argus, D. F., Gordon, R. G., and DeMets, C. (2011). Geologically Current Motion of 56 Plates Relative to the No-Net-Rotation Reference Frame. Geochem. Geophys. Geosyst. 12, Q1101. doi:10.1029/2011GC003751
Counts, J. W., Jorry, S. J., Vazquez Riveiros, N., Jouet, G., Giraudeau, J., Cheron, S., et al. (2019). A Late Quaternary record of highstand shedding from an isolated carbonate platform (Juan de Nova, southern Indian Ocean). Depositional Rec. 5, 540–557. doi:10.1002/dep2.57
Counts, J. W., Jorry, S. J., Vazquez-Riveiros, N., Amy, L. A., Dennielou, E., and Jouet, G. (2021). Sedimentology and Distribution of Late Quaternary Calciturbidites and Calcidebrites in the Mozambique Channel (Southwest Indian Ocean). Facies 67, 17. doi:10.1007/s10347-021-00624-1
Droxler, A. W., and Schlager, W. (1985). Glacial versus Interglacial Sedimentation Rates and Turbidite Frequency in the Bahamas. Geology 13, 799–802. doi:10.1130/0091-7613(1985)13<799:gvisra>2.0.co;2
Eberli, G. P. (1991). “Calcareous Turbidites and Their Relationship to Sea-Level Fluctuations and Tectonism,” in Cycles and Events in Stratigraphy. Editors G. Einsele, W. Ricken, and A. Seilacher (Springer-Verlag), 340–359.
Feldens, P., Schwarzer, K., Sakuna, D., Szczuciński, W., and Sompongchaiyakul, P. (2012). Sediment Distribution on the Inner Continental Shelf off Khao Lak (Thailand) after the 2004 Indian Ocean Tsunami. Earth Planet Sp. 64, 875–887. doi:10.5047/eps.2011.09.001
Feldens, P., Schwarzer, K., Szczucinski, W., Stattegger, K., Sakuna, D., and Somgpongchaiyakul, P. (2009). Impact of 2004 Tsunami on Seafloor Morphology and Offshore Sediments, Pakarang Cape, Thailand. Pol. J. Environ. Stud. 18, 63–68.
Fujita, K. (2013). Ecology of Large Benthic Foraminifers; a Review. Nihon Sangosho Gakkaishi 15, 57–77. doi:10.3755/jcrs.15.57
Fujita, R., Goto, K., Iryu, Y., and Abe, T. (2020). Millennial Paleotsunami History at Minna Island, Southern Ryukyu Islands, Japan. Prog. Earth Planet Sci. 7, 53. doi:10.1186/s40645-020-00365-9
Fujiwara, O., Goto, K., Ando, R., and Garrett, E. (2020). Paleotsunami Research along the Nankai Trough and Ryukyu Trench Subduction Zones - Current Achievements and Future Challenges. Earth-Science Rev. 210, 103333. doi:10.1016/j.earscirev.2020.103333
Gardner, W. D. (1989). Baltimore Canyon as a Modern Conduit of Sediment to the Deep Sea. Deep Sea Res. Part A. Oceanogr. Res. Pap. 36, 323–358. doi:10.1016/0198-0149(89)90041-1
Goto, K., Kawana, T., and Imamura, F. (2010). Historical and Geological Evidence of Boulders Deposited by Tsunamis, Southern Ryukyu Islands, Japan. Earth-Science Rev. 102, 77–99. doi:10.1016/j.earscirev.2010.06.005
Grammer, G. M., and Ginsburg, R. N. (1992). Highstand versus Lowstand Deposition on Carbonate Platform Margins: Insight from Quaternary Foreslopes in the Bahamas. Mar. Geol. 103, 125–136. doi:10.1016/0025-3227(92)90012-7
Harris, P. T., and Whiteway, T. (2011). Global Distribution of Large Submarine Canyons: Geomorphic Differences between Active and Passive Continental Margins. Mar. Geol. 285, 69–86. doi:10.1016/j.margeo.2011.05.008
Heaton, T. J., Köhler, P., Butzin, M., Bard, E., Reimer, R. W., Austin, W. E. N., et al. (2020). Marine20-The Marine Radiocarbon Age Calibration Curve (0-55,000 Cal BP). Radiocarbon 62, 779–820. doi:10.1017/RDC.2020.68
Heki, K., and Kataoka, T. (2008). On the Biannually Repeating Slow-Slip Events at the Ryukyu Trench, Southwestern Japan. J. Geophys. Res. 113, B11402. doi:10.1029/2008JB005739
Hideshima, S., Matsumoto, E., Abe, O., and Kitagawa, H. (2001). Northwest Pacific Marine Reservoir Correction Estimated from Annually Banded Coral from Ishigaki Island, Southern Japan. Radiocarbon 43, 473–476. doi:10.1017/s0033822200038352
Hori, K., and Kayanne, H. (2000). Submarine Morphology of the Island Shelf off the Middle and South Ryukyu Islands, Southwest Japan. Geogr. Rev. Jpn. Ser. A, Chirigaku Hyoron 73, 161–181. (in Japanese with Eng. Abstract). doi:10.4157/grj1984a.73.3_161
Hori, N. (1977). A Morphometrical Study on the Geographical Distribution of Coral Reefs. Geogr. Rep. Tokyo Metrop. Univ. 12, 1–75.
Hsu, S.-K., Yeh, Y.-C., Sibuet, J.-C., Doo, W.-B., and Tsai, C.-H. (2013). A Mega-Splay Fault System and Tsunami Hazard in the Southern Ryukyu Subduction Zone. Earth Planet. Sci. Lett. 362, 99–107. doi:10.1016/j.epsl.2012.11.053
Igarashi, T. (2010). Spatial Changes of Inter-plate Coupling Inferred from Sequences of Small Repeating Earthquakes in Japan. Geophys. Res. Lett. 37, L20304. doi:10.1029/2010GL044609
Ikehara, K., Irino, T., and Saito, Y. (2021a). The 2011 Tohoku-Oki Tsunami-Induced Sediment Remobilization on the Sendai Shelf, Japan, from a Comparison of Pre- and Post-tsunami Surface Sediments. Sci. Rep. 11, 7864. doi:10.1038/s41598-021-87152-8
Ikehara, K., Irino, T., Usami, K., Jenkins, R., Omura, A., and Ashi, J. (2014). Possible Submarine Tsunami Deposits on the Outer Shelf of Sendai Bay, Japan Resulting from the 2011 Earthquake and Tsunami off the Pacific Coast of Tohoku. Mar. Geol. 358, 120–127. doi:10.1016/j.margeo.2014.11.0004
Ikehara, K., Usami, K., Irino, T., Omura, A., Jenkins, R. G., and Ashi, J. (2021b). Characteristics and Distribution of the Event Deposits Induced by the 2011 Tohoku-Oki Earthquake and Tsunami Offshore of Sanriku and Sendai, Japan. Sediment. Geol. 411, 105791. doi:10.1016/j.sedgeo.2020.105791
Ikehara, K., and Usami, K. (2018). Submarine Earthquake- and Tsunami-Induced Event Deposits. Synth. Engl. Ed. 11, 13–23. doi:10.5571/syntheng.11.1_13
Imamura, F., Goto, K., and Ohkubo, S. (2008). A Numerical Model for the Transport of a Boulder by Tsunami. J. Geophys. Res. 113. doi:10.1029/2007JC004170
Imamura, F., Yoshida, Y., and Moore, A. (2001). Numerical Study of the 1771 Meiwa Tsunami at Ishigaki Island, Okinawa and the Movement of the Tsunami Stones. Proc. Coast. Eng. Jpn. Soc. Civ. Eng. 48, 346–350. (in Japanese with English abstract). doi:10.2208/proce1989.48.346
Inoue, Y. (1980a). Distribution of Recent Benthic Foraminifera in the Adjacent Seas of Japan (Part 1). Spec. Rep. Tech. Lab. Jpn. Pet.Explor. Co. 41-1, 1–114.
Inoue, Y. (1980b). Distribution of Recent Benthic Foraminifera in the Adjacent Seas of Japan (Part 2). Spec. Rep. Tech. Lab. Jpn. Pet.Explor. Co. 41-2, 1–307.
Jorry, S. J., Droxler, A. W., and Francis, J. M. (2010). Deepwater Carbonate Deposition in Response to Re-flooding of Carbonate Bank and Atoll-Tops at Glacial Terminations. Quat. Sci. Rev. 29, 2010–2026. doi:10.1016/j.quascirev.2010.04.016
Jorry, S. J., Jouet, G., Edinger, E. N., Toucanne, S., Counts, J. W., Miramontes, E., et al. (2020). From Platform Top to Adjacent Deep Sea: New Source-To-Sink Insights into Carbonate Sediment Production and Transfer in the SW Indian Ocean (Glorieuses Archipelago). Mar. Geol. 423, 106144. doi:10.1016/j.margeo.2020.106144
Kanamatsu, T., Ikehara, K., and Misawa, A. (2020). “Seafloor Morphology and Sediment Magnetic Fabric in a Putative 1771 Meiwa Tsunami Source Region in the Southern Ryukyu Islands, SW Japan,”. Editors Y. Dilek, Y. Ogawa, and Y. Okubo. Bath; Japan Coast Guard, 1988 and 1992 b Tokyo. Geol. Soc. London Spec. Publ), 501, 289–299. doi:10.1144/SP501-2019-94
Kawana, T., and Nakata, T. (1994). Timing of Late Holocene Tsunamis Originated Around the Southern Ryukyu Islands, Japan, Deduced from Coralline Tsunami Deposits. J. Geogr. (Chigaku Zasshi) 103, 352–376. (in Japanese with English abstract). doi:10.5026/jgeography.103.4_352
Kitamura, A., Ito, M., Ikuta, R., and Ikeda, M. (2018). Using Molluscan Assemblages from Paleotsunami Deposits to Evaluate the Influence of Topography on the Magnitude of Late Holocene Mega-Tsunamis on Ishigaki Island, Japan. Prog. Earth Planet Sci. 5, 41. doi:10.1186/s40645-018-0200-y
Lallemand, S., Liu, C.-S., Dominguez, S., Schnürle, P., and Malavieille, J. (1999). Trench-parallel Stretching and Folding of Forearc Basins and Lateral Migration of the Accretionary Wedge in the Southern Ryukyus: A Case of Strain Partition Caused by Oblique Convergence. Tectonics 18, 231–247. doi:10.1029/1998TC900011
Liu, J. T., Hsu, R. T., Hung, J.-J., Chang, Y.-P., Wang, Y.-H., Rendle-Bühring, R. H., et al. (2016). From the Highest to the Deepest: The Gaoping River-Gaoping Submarine Canyon Dispersal System. Earth-Science Rev. 153, 274–300. doi:10.1016/j.earscirev.2015.10.012
Miyazawa, K., Goto, K., and Imamura, F. (2012). “Re-evaluation of the 1771 Meiwa Tsunami Source Model, Southern Ryukyu Islands, Japan,” in Submarine Mass Movements and Their Consequences. (Springer), 497–506. Dordrecht: doi:10.1007/978-94-007-2162-3_44
Mulder, T., Ducassou, E., Eberli, G. P., Hanquiez, V., Gonthier, E., Kindler, P., et al. (2012a). New Insights into the Morphology and Sedimentary Processes along the Western Slope of Great Bahama Bank. Geology 40, 603–606. doi:10.1130/g32972.1
Mulder, T., Ducassou, E., Gillet, H., Hanquiez, V., Tournadour, E., Combes, J., et al. (2012b). Canyon Morphology on a Modern Carbonate Slope of the Bahamas: Evidence of Regional Tectonic Tilting. Geology 40, 771–774. doi:10.1130/g33327.1
Mulder, T., Joumes, M., Hanquiez, V., Gillet, H., Reijmer, J. J. G., Tournadour, E., et al. (2017). Carbonate Slope Morphology Revealing Sediment Transfer from Bank-To-Slope (Little Bahama Bank, Bahamas). Mar. Petroleum Geol. 83, 26–34. doi:10.1016/j.marpetgeo.2017.03.002
Mullins, H. T. (1983). Comments and Reply on 'Eustatic Control of Turbidites and Winnowed Turbidites'. Geol 11, 57–58. doi:10.1130/0091-7613(1983)11<57:caroec>2.0.co;2
Nakamura, M. (2009). Fault Model of the 1771 Yaeyama Earthquake along the Ryukyu Trench Estimated from the Devastating Tsunami. Geophys. Res. Lett. 36, L19307. doi:10.1029/2009GL039730
Nakamura, M., and Sunagawa, N. (2015). Activation of Very Low Frequency Earthquakes by Slow Slip Events in the Ryukyu Trench. Geophys. Res. Lett. 42, 1076–1082. doi:10.1002/2014GL062929
Nishimura, T. (2014). Short-term Slow Slip Events along the Ryukyu Trench, Southwestern Japan, Observed by Continuous GNSS. Prog. Earth Planet. Sci. 1, 22. doi:10.1186/s40645-014-0022-5
Okamura, Y., Nishizawa, A., Fujii, Y., and Yanagisawa, H. (2018). Accretionary Prism Collapse: a New Hypothesis on the Source of the 1771 Giant Tsunami in the Ryukyu Arc, SW Japan. Sci. Rep. 8, 13620. doi:10.1038/s41598-018-31956-8
Oyama, M., and Takehara, H. (1967). Revised Standard Soil Color Charts. Tokyo: Japan Color Enterprise.
Palanques, A., El Khatab, M., Puig, P., Masqué, P., Sánchez-Cabeza, J. A., and Isla, E. (2005). Downward Particle Fluxes in the Guadiaro Submarine Canyon Depositional System (North-western Alboran Sea), a River Flood Dominated System. Mar. Geol. 220, 23–40. doi:10.1016/j.margeo.2005.07.004
Paris, R., Fournier, J., Poizot, E., Etienne, S., Morin, J., Lavigne, F., et al. (2010). Boulder and Fine Sediment Transport and Deposition by the 2004 Tsunami in Lhok Nga (Western Banda Aceh, Sumatra, Indonesia): A Coupled Offshore-Onshore Model. Mar. Geol. 268, 43–54. doi:10.1016/j.margeo.2009.10.011
Peterson, E. T., and Seno, T. (1984). Factors Affecting Seismic Moment Release Rates in Subduction Zones. J. Geophys. Res. 89, 10233–10248. doi:10.1029/JB089iB12p10233
Pickering, K. T., and Hiscott, R. N. (2016). Deep Marine Systems, Processes, Deposits, Environments, Tectonics and Sedimentation. Oxford: AGU and Wiley, 657p.
Porcile, G., Bolla Pittaluga, M., Frascati, A., and Sequeiros, O. E. (2020). Typhoon-induced Megarips as Triggers of Turbidity Currents Offshore Tropical River Deltas. Commun. Earth Environ. 1, 2. doi:10.1038/s43247-020-0002-1
Puga-Bernabéu, Á., Webster, J. M., Beaman, R. J., Reimer, P. J., and Renema, W. (2014). Filling the Gap: A 60 Ky Record of Mixed Carbonate-Siliciclastic Turbidite Deposition from the Great Barrier Reef. Mar. Pet.Geol. 50, 40–50. doi:10.1016/jmarpetgeo.2013.11.009
Puga-Bernabéu, Á., Webster, J. M., Beaman, R. J., and Guilbaud, V. (2011). Morphology and Controls on the Evolution of a Mixed Carbonate-Siliciclastic Submarine Canyon System, Great Barrier Reef Margin, North-Eastern Australia. Mar. Geol. 289, 100–116. doi:10.1016/j.margeo.2011.09.013
Puga-Bernabéu, Á., Martín, J. M., and Braga, J. C. (2007). Tsunami-related Deposits in Temperate Carbonate Ramps, Sorbas Basin, Southern Spain. Sed. Geol. 199, 107–127. doi:10.1016/j.sedgeo.2007.01.20
Saito, Y., Katayama, H., Ikehara, K., Kato, Y., Matsumoto, E., Oguri, K., et al. (1998). Transgressive and Highstand Systems Tracts and Post-glacial Transgression, the East China Sea. Sediment. Geol. 122, 217–232. doi:10.1016/s0037-0738(98)00107-9
Sakuna-Schwartz, D., Feldens, P., Schwarzer, K., Khokiattiwong, S., and Stattegger, K. (2015). Internal Structure of Event Layers Preserved on the Andaman Sea Continental Shelf, Thailand: Tsunami vs. Storm and Flash-Flood Deposits. Nat. Hazards Earth Syst. Sci. 15, 1181–1199. doi:10.5194/nhess-15-1181-2015
Sasaki, K., Omura, A., Miwa, T., Tsuji, Y., Matsuda, H., Nakamori, T., et al. (2006). 230Th/234U and14C Dating of a Lowstand Coral Reef beneath the Insular Shelf off Irabu Island, Ryukyus, Southwestern Japan. Isl. Arc 15, 455–467. doi:10.1111/j.1440-1738.2006.00541.x
Shanmugam, G., and Moiola, R. J. (1988). Submarine Fans: Characteristics, Models, Classification, and Reservoir Potential. Earth-Science Rev. 24, 383–428. doi:10.1016/0012-8252(88)90064-5
Taylor, B., and Goodliffe, A. M. (2004). The West Philippine Basin and the Initiation of Subduction, Revisited. Geophys. Res. Lett. 31, L12602. doi:10.1029/2004GL020136
Tournadour, E., Mulder, T., Borgomano, J., Gillet, H., Chabaud, L., Ducassou, E., et al. (2017). Submarine Canyon Morphologies and Evolution in Modern Carbonate Settings: The Northern Slope of Little Bahama Bank, Bahamas. Mar. Geol. 391, 76–97. doi:10.1016/j.margeo.2017.07.014
Tsuji, Y. (1993). Tide Influenced High Energy Environments and Rhodolith-Associated Carbonate Deposition on the Outer Shelf and Slope off the Miyako Islands, Southern Ryukyu Island Arc, Japan. Mar. Geol. 113, 255–271. doi:10.1016/0025-3227(93)90021-m
Ujiie, H., Nakamura, T., Miyamoto, Y., Park, J.-O., Hyun, S., and Oyakawa, T. (1997). Holocene Turbidite Cores from the Southern Ryukyu Trench Slope: Suggestions of Periodic Earthquakes. Jour. Geol. Soc. Jpn. 103, 590–603. doi:10.5575/geosoc.103.590
Ujiié, H. (1980). Significance of “500 M Deep Island Shelf” Surrounding the Southern Ryukyu Island Arc for its Quaternary Geological History. Quat. Res. (Daiyonki-Kenkyu) 18, 209–219.
Usami, K., Ikehara, K., Jenkins, R. G., and Ashi, J. (2017). Benthic Foraminiferal Evidence of Deep-Sea Sediment Transport by the 2011 Tohoku-Oki Earthquake and Tsunami. Mar. Geol. 384, 214–224. doi:10.1016/j.margeo.2016.04.001
Usami, T. (2003). New Comprehensive Summary of Historical Destructive Earthquakes in Japan. Tokyo: Univ. Tokyo Press, 605p.
Webster, J. M., Beaman, R. J., Puga-Bernabéu, Á., Ludman, D., Renema, W., Wust, R. A. J., et al. (2012). Late Pleistocene History of Turbidite Sedimentation in a Submarine Canyon off the Northern Great Barrier Reef, Australia. Palaeogeogr. Palaeoclimatol. Palaeoecol. 331-332, 75–89. doi:10.1016/j.palaeo.2012.02.034
Webster, J. M., Braga, J. C., Humblet, M., Potts, D. C., Iryu, Y., Yokoyama, Y., et al. (2018). Response of the Great Barrier Reef to Sea-Level and Environmental Changes over the Past 30,000 Years. Nat. Geosci. 11, 426–432. doi:10.1038/s41561-018-0127-3
Webster, J. M., Clague, D. A., Riker-Coleman, K., Gallup, C., Braga, J. C., Potts, D., et al. (2004). Drowning of the −150 M Reef off Hawaii: A Casualty of Global Meltwater Pulse 1A? Geology 32, 249–252. doi:10.1130/G20170.1
Wunsch, M., Betzler, C., Lindhorst, S., Lüdmann, T., and Eberli, G. P. (2017). Sedimentary Dynamics along Carbonate Slopes (Bahamas Archipelago). Sedimentology 64, 631–657. doi:10.1111/sed.12317
Xu, J. P., Noble, M., Eittreim, S. L., Rosenfeld, L. K., Schwing, F. B., and Pilskaln, C. H. (2002). Distribution and Transport of Suspended Particulate Matter in Monterey Canyon, California. Mar. Geol. 181, 215–234. doi:10.1016/s0025-3227(01)00268-7
Keywords: turbidite, coral reef, tsunami, submarine canyon, submarine fan, Ryukyu islands
Citation: Ikehara K, Kanamatsu T and Usami K (2022) Possible Tsunami-Induced Sediment Transport From Coral Reef to Deep Sea Through Submarine Canyons on the Southern Ryukyu Forearc, Japan. Front. Earth Sci. 10:753583. doi: 10.3389/feart.2022.753583
Received: 05 August 2021; Accepted: 20 April 2022;
Published: 11 May 2022.
Edited by:
Fabiano Gamberi, National Research Council (CNR), ItalyReviewed by:
Susanne Pohler, University of Graz, AustriaÁngel Puga-Bernabéu, University of Granada, Spain
Copyright © 2022 Ikehara, Kanamatsu and Usami. This is an open-access article distributed under the terms of the Creative Commons Attribution License (CC BY). The use, distribution or reproduction in other forums is permitted, provided the original author(s) and the copyright owner(s) are credited and that the original publication in this journal is cited, in accordance with accepted academic practice. No use, distribution or reproduction is permitted which does not comply with these terms.
*Correspondence: Ken Ikehara, k-ikehara@aist.go.jp