Wind Tunnel Studies on the Prevention of Particle Accumulation Onto the Bogie of High-Speed Train
- 1Key Laboratory of Mechanics on Disaster and Environment in Western China, the Ministry of Education of China, Lanzhou University, Lanzhou, China
- 2College of Civil Engineering and Mechanics, Lanzhou University, Lanzhou, China
- 3School of Information Engineering, Lanzhou City University, Lanzhou, China
- 4College of Atmospheric Sciences, Lanzhou University, Lanzhou, China
- 5School of Architecture, Civil and Environmental Engineering, Swiss Federal Institute of Technology, Lausanne, Switzerland
When high-speed train runs in the environment with a large number of airborne particles, these particles may accumulate on the bogie of the train, which will further challenge the normal operation of the bogie and reduce the riding comfort and train safety. In order to prevent particles entering into the bogie area, different protective devices around the bogie are used. However, up to present, the systematical analysis on the protective effects of diverse protective measures against different types of particles are still very limited. In this study, with the widely-used shirt boards and spoilers as protective devices, the airborne particle transport processes around the bogie were simulated in the wind tunnel Lab. Three kinds of typical particles, including artificial snow particle, wheat bran (substitute of snowflake) and sieved soil, were chosen to represent different airborne particles under real conditions. Three evolution indexes, including the relative reduction ratio of artificial snow particles, the relative particle flux reduction ratio of fine wheat bran, and the relative concentration reduction ratio of fine sieve soil particles, were used to assess their protective effects. Results showed that the combination of short-skirt board and straight triangular spoiler (i.e., in A5 case, the straight triangle spoilers installed at the front and rear ends of the train bogie, and the short-skirt boards installed on both sides of the train bogie) was the best protection with the studied three particles. The relative reduction ratio of the average snow mass in this case was 75.59%, the relative reduction ratio of the flux in the level of fine wheat bran exceeded 78%, and the relative reduction ratio of the fine soil particle concentration was more than 96%.
Introduction
When high-speed train runs in the environment with a large number of airborne particles such as dust (Tian, 2015; Wang et al., 2017) or snow (Vajda et al., 2014; Wu et al., 2019), these particles may enter the bogie area and affect the normal operation of the components inside the bogies. Especially, in cold areas with abundant snow particles, snow particle accumulation and icing on the bogie often (Liu et al., 2020) happens and then threatens the safety of trains (Knudsen et al., 1994; Shishido et al., 2014; Zhu et al., 2016; Xie et al., 2017).
In order to prevent the accumulation of particles on the bogie, solutions are usually focused on the two sub-processes of particle accumulation, including 1) particles enter the bogie area, and 2) particles contact with and stick to the surface of the bogie to form the accumulation eventually (Zhang et al., 2018). For example, Bettez (2011) prevented snow and ice to enter the bogie through sealing the coil spring device with a small gap or adding spoilers in multiple locations. Andersson (2012) improved the design of train bodies and bogies operating under the extreme cold conditions in Northern Europe with protection measures to block the accumulation of snow and ice in the sensitive areas, such as suspension systems and braking systems. Azuma et al. (2010) used the heater or air conditioner to heat the bogie end cover and bogie cover plate to effectively prevent the accumulation of snow particles, although the durability of the heating wire and the layout of the exhaust pipe of air conditioning were difficult to be satisfied. Recently, studies showed that anti-icing coating on the key components of the bogie was very effective although its cost was relatively high and life time was till short (Janjua et al., 2017).
Up to present, due to the low cost and easy operation, fairings or spoilers were often installed around the bogie to prevent or reduce the entering of particles without any major change in the layout of bogie components. For example, among the twelve suggestions of Kloow (2011) to reduce the snow accumulation at the bottom of bogie, the relatively simple and feasible method was to change the flow field around bogie through adding spoilers to reduce the snow particles entering the steering, although these methods and the corresponding optimization needed further experiments to confirm. Shishido et al. (2009) installed deflectors on the outside of bogie to change the air flow direction and prevent snow particles to enter from the bogie side. According to wind tunnel tests and numerical simulations on different-angle deflectors, it was believed that 60-degree deflector was the best choice to reduce snow accumulation on the bogie. Wang et al. (2018), Wang et al.(2019) used a discrete model to study the effects of the end wall slope of the bogie cabin, spoiler and brake shroud on the snow accumulation on the bogie and brake calipers. Gao et al. (2020a), Gao et al.(2020b), and Cai et al. (2021) simulated the anti-snow deflector with different angles installed at the bottom of the bogie to reduce the accumulation of snow on the surface of bogie. However, analysis, comparison and optimization of the protection efficiency of various measures under the same conditions has not been done systematically. Therefore, in this study, five bogie protection methods with typical particles (artificial snow particles and wheat bran, the replacement of snowflake) and dust (fine sieve soil) were investigated systematically through wind tunnel experiments for the first time.
Wind Tunnel Experiment
The Main Equipment
Experiments were conducted in the Multi-functional Environmental Wind Tunnel at Lanzhou University. The main measuring instruments, train models, auxiliary equipments and granular materials used in these experiments were shown in Figure 1.
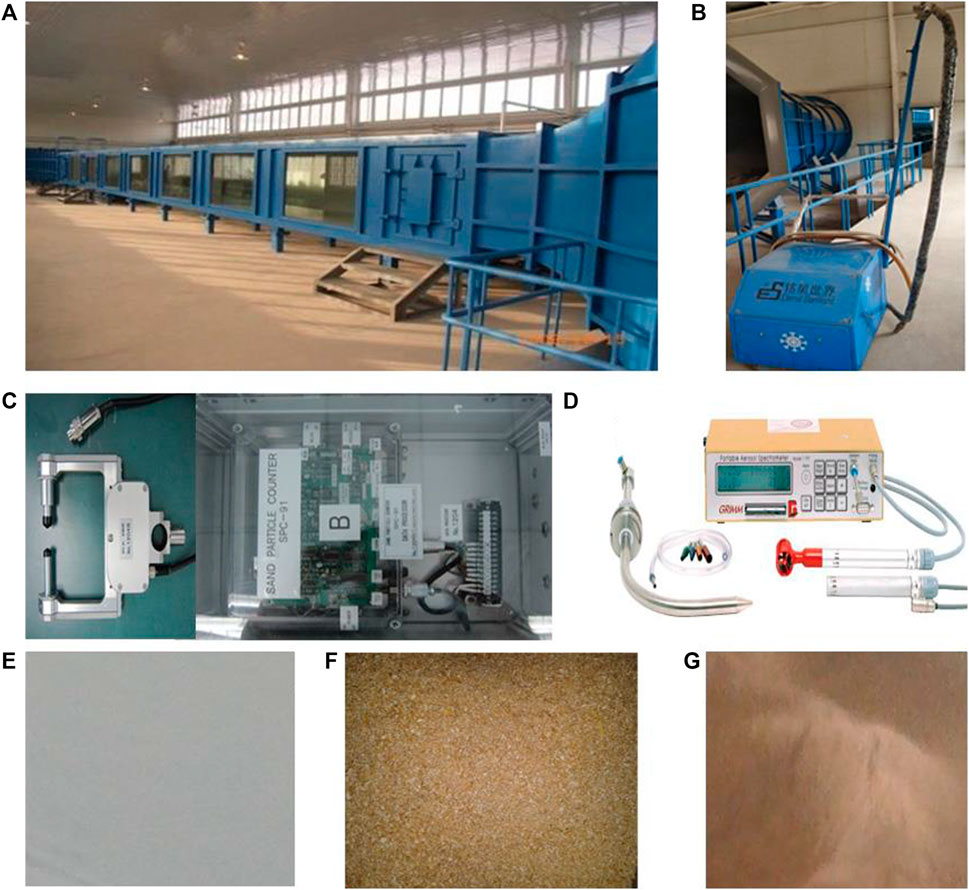
FIGURE 1. Major devices and granular materials in this study, including (A) Multi-functional Environment Wind Tunnel at Lanzhou University, (B) the moveable snowmaker, (C) prober and processer of the sand particle counter (SPC-91), (D) aerosol spectrometer, (E) artificial snow particles, (F) fine wheat bran particles, and (G) fine-sieve soil particles.
The Multi-Functional Environment Wind Tunnel at Lanzhou University
In order to simulate the boundary layer wind environment, the Multi-functional Environmental Wind Tunnel at Lanzhou University (Figure 1A) was used. The wind tunnel has a blow-down wind tunnel with four parts, including power, rectification, experiment and diffusion sections, with a total length of 85.00 m, a cave length of 55.00 m, an experiment section length of 20.00 m and a cross-sectional area of 1.3 m (width) × 1.45 m (height). The wind speed can be continuously adjusted from 3 m/s to 40 m/s (Zhang et al., 2014).
Main Measuring and Auxiliary Equipment
The Aerosol spectrometer 1.109 with a measuring frequency of 1/6 Hz from German (Figure 1D) was used to measure the concentration of particles with a size smaller than 32 μm. The light scattering principle was used to analyze the light scattering intensity signal and the particle size in real time. Typically, after the laser lights on the particle, the solid-state detector will detect the scattered light and convert the light signal into an electrical signal. After the electrical signal is amplified according to different pulse amplitudes in the register, the number of particles in a period of time or the concentration of particles can be calculated out. The Aerosol spectrometer 1.109 can continuously monitor the dust particle mass concentration in 31 particle diameter channels within 0.25∼32 μm in real time.
The Snow/Sand Particle Counter (SPC-91, Figure 1C) was from Niigata Electric Co., Ltd. Japan. The device emits a laser beam opposing to a photosensitive elements with a certain distance. When the particles pass through the region covered by the laser, the received light intensity changes and the number and size of the particle are identified and recorded. According to the particle number and size, the transport flux of particles at the measuring location can be obtained. The chosen SPC-91 can identify particles with a particle size of 50–500 μm within 38 channels.
SHD4-1.5 moveable snowmaker (Figure 1B) was used to make artificial snow. The mass of snow deposited on the bogie was weighed on a balance.
Train Models and Experiment Layout
According to reference (Sui et al., 2020; Niu et al., 2016; Allain et al., 2014), a high-speed train model with three coaches (head train, middle train and tail train) was built in the Wind Tunnel shown in Figure 2. The ratio of the model over the original CRH2 (China Railway High-speed 2) was 1/10. Blockage ratio of the model fixed in tunnel was less than 10%, which satisfied the definition of low blockage ratios given by Sui et al. (Sui et al., 2020) Each bogie was sequentially numbered from G to L along the direction of incoming flow, and number F was the front reference position of the vehicle (Figure 2C).
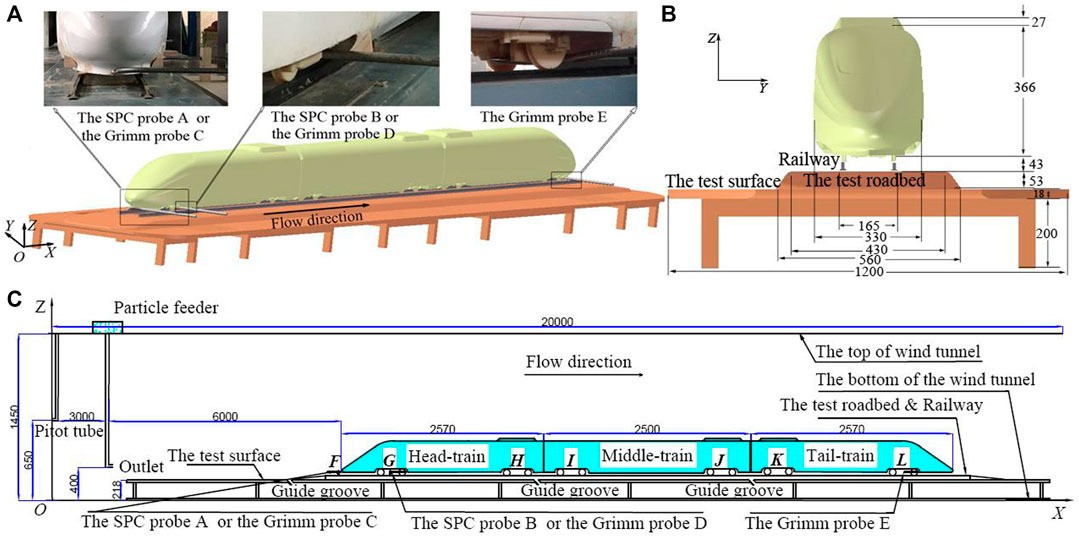
FIGURE 2. Sketch of the train model, including (A) 3 days diagram, (B) front view of YZ plane in mm, and (C) side view of XZ plane in mm (F is reference position, G, H, I, J, K and L represent the central positions of six bogie areas).
In order to descript easily, a three-dimensional Cartesian right-handed coordinate system was established with the origin of coordinate as the intersection of the wind tunnel entrance face, the wind tunnel center axis and the wind tunnel bottom face. The central axis of wind tunnel was the positive X axis along the wind direction, the XOY plane was parallel to the bottom of the wind tunnel, the Z axis direction was vertically upward, and the unit was mm.
The simulated ground (Figures 2B,C) at a height of 200 mm from the bottom of the wind tunnel was used to obtain a uniform incoming wind field. The width of the simulated ground was 1,200 mm and the thickness 18 mm. Meanwhile, the 45° guide grooves were cut in the ground to reduce the thickness of surface layer over it. The simulated roadbed and track were placed on the simulated ground. The width of upper plane of the simulated roadbed was 430 mm, the width of its lower plane was 560 mm, its height was 53 mm, and the height of simulated track was 10 mm. The height of train model was 366 mm with an outer distance between the two tracks of 165 mm (Figure 2B). The coordinates of the reference position F in front of the train were (9,000, 0, 299).
A Pitot tube (Figure 2C) was used to measure the wind speed at the center of the entrance of wind tunnel test section with a distance of 9,000 mm to the front of train. The Pitot tube was 650 mm from the bottom of the wind tunnel and 650 mm from the side wall of the wind tunnel. The coordinates of the Pitot tube measuring point were (0, 0, 650). A particle feed inlet was set at the top of the wind tunnel with a distance of 3,000 mm from the entrance. The coordinate of the feed inlet were (3,000, 0, 1,450). The incoming wind speed used in the experiment was 30 m/s.
Particles and Relative Measuring Methods
In this study, the snow outlet of a moveable snowmaker was 6,000 mm from the head train and 400 mm high from the bottom of the wind tunnel. Coordinates of the snow outlet of snowmaker were (3,000, 0, 400). After the experiment, the weight increase of the bogie of train was measured as the weight of snow accumulated.
Through the SPC-91 with Probe A fixed at the F position (reference position, Figure 2C) and Probe B fixed at the center of the G area (Figure 2C), the horizontal transport fluxes at the measured positions were obtained. The reduction ratio of the horizontal flux of the G area over that of F area was used as the basis to determine its protecting effect, in which a greater horizontal flux reduction ratio meant a better protection generated.
As shown in Figure 1D, the real-time dust concentration measurement systems (Aerosol spectrometers) were fixed at the positions of F, L and G to measure the concentration of sieved soil particles (diameter dp = 0.1∼150 μm). According to the reduction ratio of the concentration at these points, the effectiveness of protective measures was determined.
As listed in Table 1, the bulk density of fine wheat bran particles used in this study was 290 kg/m3, and that of the fine-sieve soil particles was 650 kg/m3. The physical properties of natural snow particles were adapted from reported literatures.
Protective Components
As shown in Figure 3, three protective parts, including short-skirt board (Figure 3E), oblique triangle spoiler (Figure 3A) and straight triangle spoiler (Figure 3C), were added and tested. The short-skirt board added to the two sides of train steering frame was downward extended parts of the original train skirt. In the test, the short-skirt board extended 17 mm downwards. The spoiler was installed on the plane part of the equipment cover at the front and back of bogie which was at the bottom of the train. In order to compare the protection efficiency, two different shapes of spoilers were provided. The maximum height of the two spoilers was 10 mm, and they were attached to the train equipment cover with a length of 178 mm and a width of 100 mm. The lengths of the two oblique sides of the oblique triangle spoiler (Figure 3A) were 31.60 and 70.73 mm, and the length of the oblique side of the straight triangular spoiler was 100.50 mm (Figure 3C).
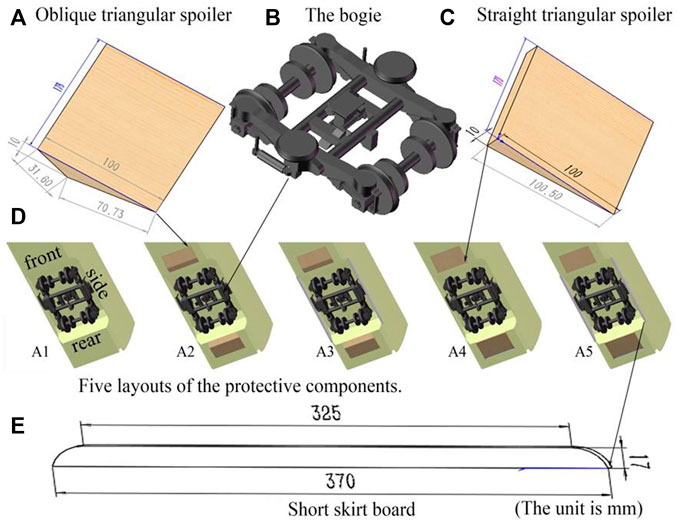
FIGURE 3. Protective components [(A) oblique triangle spoiler, (B) the bogie and (C) straight triangle spoiler, and (E) short-skirt board] and their five layout with arrows pointing to the component enlarged (D).
In the experiment, each protective component was glued to the corresponding position of the train model with plastic tape. Because the surface of the tape is smooth, it should only have little effect on the experiment and be easy to replace.
Experiment Procedures
According to different combinations of protection and spoiler measures, five cases were tested (Figures 3DA1–A5; Table 2), including the original case A1 without short-skirt board and spoiler, A2 without short-skirt board but with oblique triangular spoiler, A3 with both short-skirt board and oblique triangular spoiler, A4 without short-skirt board but with straight triangular spoiler, and A5 with both short-skirt board and straight triangular spoiler.
As shown in Figure 2, after trains were fixed along the direction of incoming flow, protective effects of artificial snow, fine wheat bran and fine-and-sieved soil were investigated in the five cases (A1-A5). Experiments were carried out as the followed:
1. The simulated ground and train models and related experimental equipment were arranged in the wind tunnel as shown in Figure 2.
2. in the wind field test, a pitot tube was used to measure the wind speed at the upwind cross section [Z∈(223, 518) mm and Y∈(-160, 160) mm] with a distance (in X direction) 500 mm away from the train head.
3. During the artificial snow accumulation measuring, when the outdoor temperature was below −6°C, the moveable snowmaker (Figure 1B) was used to make snow particles. These snow particles were fed into the wind tunnel to simulate wind blowing and snowing conditions. After the accumulation test was done within 5 min, the bogie was removed and measured on a balance to obtain its mass change. The increase of weight was the amount of accumulated snow. The A1-A5 cases were tested separately, while the average accumulated snow mass on the six bogies (G-L in Figure 2C) in each case was measured.
4. In the comparative test on particle flux of fine wheat bran, fine wheat bran was added from the feeding port as shown in Figure 2. Probes of SPC were located at F and G positions along the center line of the train, respectively. Probe A was fixed at the reference position F to measure the horizontal flux of incoming particles. Probe B was put under the bogie G of the front train at the center to measure the horizontal flux of particles around bogie G. Each measurement was repeated for 5 min, and each feed was about 4 kg.
5. In the test on fine and sieved soil concentration, through a feeding tube at the feeding port (openings on both ends of wind flow), fine and sieved soil dust particles was sent into the wind tunnel. The Grimm probe C (Figures 2A,C) at the reference position F (Figure 2C) was installed to measure the concentration of incoming particles. The Grimm probe D (Figures 2A,C) was put behind the center of the bottom on the train bogie G to measure the dust concentration in the region of bogie G. The Grimm probe E was put behind the center of the truck of the bogie L (Figures 2A,C) to measure the particle concentration around bogie L. Each test was done within 5 min and A1-A5 cases were tested separately.
6. In the data processing and analysis, the average snow mass of the bogies in A1-A5 cases, the horizontal flux of fine wheat bran and the soil concentration of the fine-and-sieved soil were all summarized to evaluate the snow-protecting effects of the tested protective components.
Results and Discussion
Wind Field Test and Reynolds Number
As shown in Figure 4; Supplementary Appendix SA1, the test was carried out in a stable flow field because the height of the train bogie model was above the position Z > 218 + 53 = 271 mm (Figure 4).
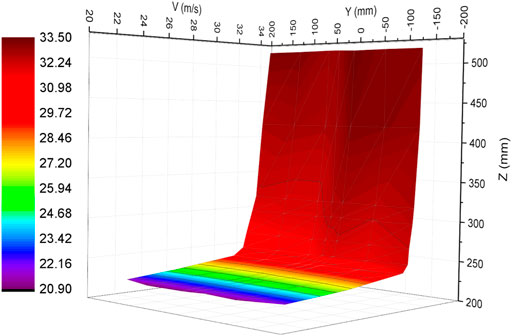
FIGURE 4. Effects of incoming wind field. V is the wind speed at the upwind cross section [Z∈(223, 518) mm and Y∈(-160, 160) mm].
According to previous reports (Kwon et al., 2001; Anderson, 2007; Cross et al., 2015; Niu et al., 2016), the Reynolds number can be calculated from the following Eq. 1 as,
where the air density ρ is 1.225 kg/m³, U is the incoming wind velocity (21–33 m/s), H is the height of the train model and used as a characteristic length, and the air viscosity coefficient μ is 1.8 × 10−5 Pa s.
In this study, the established Reynolds numbers were in the range of 5.2 × 105–8.8×105.
Tests on Snow Accumulation
The five cases were tested separately to calculate the average mass of snow accumulated on the six bogies under the test conditions, and the average snow mass was recorded as Mi for cases Ai. The average reducing ratio of snow mass in each case from that of the original case A1 (without short-skirt board and spoiler) was recorded as RMi, as,
With the original case A1 as a reference, a larger RMi meant a smaller average amount of accumulated snow in the Ai case, indicating a better measure to suppress the snow accumulation on the bogie correspondingly.
Figure 5 shows the relative reduction ratio of the average snow mass on the six bogies in the five cases. With the short-skirt boards and bottom spoilers, the average mass of accumulated snow was significantly lower than that in the original case A1 without short-skirt board and spoiler. In the case of A3 with the smallest reduction, its reduction ratio RM3 was 62.46%, indicating that the short-skirt boards and oblique triangular spoilers might have excellent protective effects. The reduction ratios of average accumulated snow mass in A5 with short-skirt boards and straight triangular spoilers, A4 without short-skirt board but with straight triangular spoilers, and A2 without short-skirt board but with oblique triangular spoilers were RM5 = 75.59%, RM4 = 69.79%, and RM2 = 67.08%, respectively. Results showed that both short-skirt boards and straight triangular spoilers could reduce the snow accumulation and icing in the bogie area effectively. Therefore, the design with changes in the position of the bogie side skirts and the front and rear cover plates of the original case could be done to reduce the snow accumulation and icing in the bogie area during the snowy days as listed in Supplementary Appendix SA2. Among these five cases, A4 case was similar to case 3 in reference (Wang et al., 2018), and its snow cover of the entire bogie was reduced by less than 69.79% due to the spoiler while that of case 3 in reference (Wang et al., 2018) was reduce by 49.34%.
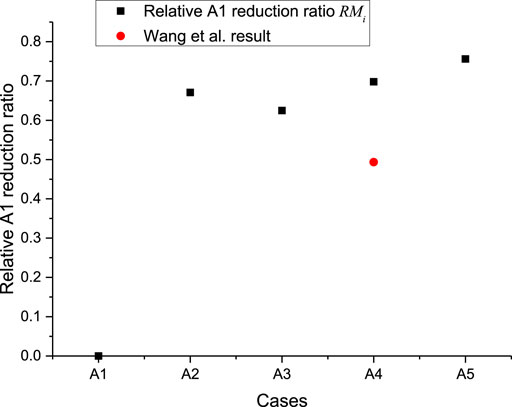
FIGURE 5. The relative reduction ratio RMi of average snow mass on the six bogies in different cases over that of the original case (A1).
Flux Test on Fine Wheat Bran
The horizontal flux measured with the SPC probe A at position F was recorded as QAi (i = 1, 2, 3, 4, 5, corresponding to the five cases). Similarly, QB was the data from the SPC probe B at position G. The horizontal flux QA of incoming particles was measured at front of head train, which was the initial particle horizontal flux state without effects of the train and protective parts. QB was the horizontal flux state of particles when the flow field was affected by lower-part cover plates and protective parts of the train. The ratio difference between these two fluxes (QA-QB) and QA was defined as the flux reduction ratio RQ (Eq. 3).
A larger RQ means that the incoming flux horizontal flux QA and the particle horizontal flux at the bottom of the bogie G QB have a larger difference, the horizontal flux QB at the bottom of the bogie G is smaller than the horizontal flux QA of incoming particles, the amount of particles entering the bogie area is relatively small, and the protective effect of the corresponding protective measures on the bogie from fine wheat bran with large particle size and low density is better.
Figure 6 shows the Probability density function (pdf) of fine bran particles (particle diameter/pdf) tested with SPC probe A in the A1 case. Because the size of fine wheat bran particles is out of the SPC test range, there was a relatively high proportion of 36–60 μm and 490–500 μm particles, but the overall particle flux was still controllable.
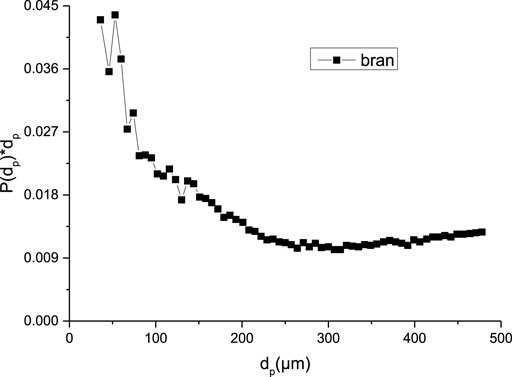
FIGURE 6. Probability density function (pdf) of fine bran particles tested with SPC Probe A in A1 case (particle diameter/pdf).
Figure 7 illustrates the relative reduction ratios of particle flux RQi (i = 1, 2, 3, 4, 5) with fine wheat bran under the five conditions (A1, A2, A3, A4 and A5). Because repeated RQi (i = 1, 2, 3, 4, 5) values of every group were relatively close, the experiments were repeatable. According to the layout of the SPC and the data processing method, a greater relative reduction ratio of flux RQi should suggest a better protection effect on the bogie from fine wheat bran with large-grain and low-density.
As shown in Figure 7, the relative reduction ratio RQ1 of the original case A1 without any protective measures did not exceed 8%, which was the lowest in the five cases. This result indicated that, without the skirt boards and bottom spoilers, the horizontal flux QA1 of incoming particles and the horizontal flux QB1 of the particles at the bottom of bogie G was very small, and there were more than 92% of incoming particles entering the bogie area through the train bottom and along the incoming flow. These results further approved that the airborne snow particles would enter the bogie area along the high-speed travelling of the train and might form accumulated snow and ice. Additionally, with the protection of skirt boards and bottom spoilers, the relative reduction ratio of flux RQi (i = 2, 3, 4, 5) was increased significantly compared to that of the original case A1. The relative flux reduction ratio of RQ2 (without short-skirt board but with oblique triangular spoilers) exceeded 34%, RQ3 (with short-skirt boards and oblique triangular spoilers) was more than 30%, RQ4 (without short-skirt board but with straight triangular spoilers) was larger than 37%, and RQ5 (with short-skirt boards and straight triangular spoilers) was more than 78%. These results showed that the protective effect in A5 with both short-skirt boards and straight triangular spoilers was the best among the five cases. Therefore, in the experiments with fine wheat bran having large particle size and low density, the combination of protective short-skirt boards and straight triangular spoilers could effectively reduce the amount of particles entering the bogie area as shown in Supplementary Appendix SA3.
Concentration Test on Fine-and-Sieved Soil Particles
In the test of sieved soil, a Grimm probe C (Figures 2A,C) was placed at the reference position F (Figures 2A,C) to measure the concentration of incoming soil particles, which was recorded as NAi (i = 1, 2, 3, 4, 5, representing the five tested cases). Another Grimm probe D was placed in the back center at the bottom of bogie G of the head train (Figures 2A,C) to measure the dust concentration entering the bottom of the bogie G (recorded as NBi). The third Grimm probe E was placed in the back center of the bogie L of the trail train (Figures 2A,C) to measure the concentration of particles entering the bottom of the bogie L (recorded as NLi). A1-A5 cases were tested separately.
The reduction ratio of particle concentration under the bogie G (NBi) over the incoming particle concentration (NAi) was calculated as,
The reduction ratio of particle concentration (NLi) under the bogie L over the incoming particle concentration (NAi) was determined as,
And the larger RNBi and RNLi indicated the better protection.
The Probability density function (pdf) of soil particles (particle diameter/pdf) tested with Grimm Probe A in the A1 case showed that 10–30 μm particles account for a relatively high proportion (Figure 8).
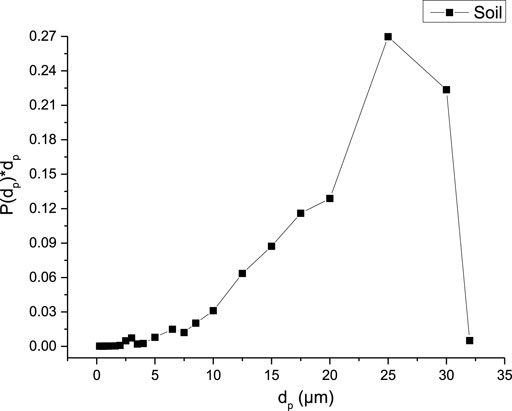
FIGURE 8. Probability density function (pdf) of fine soil particles tested with Grimm Probe A in A1 case (particle diameter/pdf).
Figure 9 shows the concentration reduction ratio of fine and sieved soil particles under bogies G and L in the five cases. A larger concentration reduction ratio suggested a better protective effect from fine and sieved soil particles with a small size and high density. The relative concentration reduction ratio in all cases exceeded 71%, suggesting that, at high wind speed of 30 m/s, only relatively limited dust particles could enter the bogie area. However, in different cases, compared to that of the original case A1 (without short-skirt board and spoiler), the relative reduction ratios of particle concentration in all cases with skirt boards and bottom spoilers [RNBi and RNLi (i = 2, 3, 4, 5)] were increased to more than 94%. At the bottom of the bogie G, the relative reduction ratio of particle concentration in case A5 (with short-skirt boards and straight triangular plates) (RNB5) was the largest. At the bottom of the bogie L, the relative concentration reduction ratio in A4 (without short-skirt board but with straight triangular spoilers) (RNL4) was the largest, followed by that in A5 (RNL5). In these tested cases, A5 had the best protection, followed by A4, which indicated that short-skirt boards and straight triangular spoilers could effectively reduce the dust particle concentration in the bogie area in the experiment with fine and sieved soil particles with small size and high density.
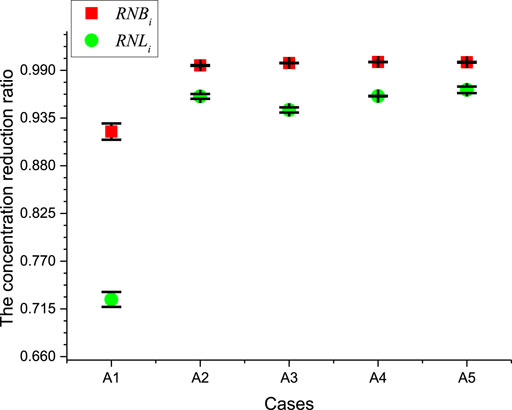
FIGURE 9. The reduction ratios of particle concentration at the bottom of bogies G and L (RNBi and RNLi) over the incoming particle concentration.
In the experiments with fine and sieved soil particles, both the relative reduction ratios of the particle concentration under the front bogie G of the head-train and the incoming particle concentration, and those under the rear bogie L of the tail-train and the incoming particle concentration, were compared. It was found that the relative concentration reduction ratios of the five cases all exceeded 71%, which verified again that relatively limited dust particles entered the bogie area at a high wind speed of 30 m/s. The relative particle concentration reduction ratio in A5 was more than 96%, and even exceeded 99% in the front bogie area of the front train. These results suggested that short-shirt boards and straight triangular spoilers could reduce the dust particle concentration in the bogie area and protect the high-speed train effectively (Supplementary Appendix SA4).
Conclusion
In this study, through wind tunnel experiments, protecting effects of the bottom protective parts of the bogie (skirt boards and spoilers) from three typical particles (snow grains, fine wheat bran and fine sieved soil particle) were studied. Based on different combinations of protective shirt boards and bottom spoilers, experiments were done within five cases, including the original case A1 (without short-skirt board and spoiler), A2 (without short-skirt board but with oblique triangular spoilers), A3 (with short-skirt boards and oblique triangular spoilers), A4 (without short-skirt board but with straight triangular spoilers), and A5 (with short-skirt boards and straight triangular spoilers). It is concluded that both skirt boards and bottom spoilers could significantly prevent the particle to enter into the bogie area. A5 had the best protection in all five tested cases with different particles. Compared with the original case A1, A5 had the average reduction ratio of accumulated snow mass of 75.59%, the relative reduction ratio of the fine wheat bran horizontal flux (RQ5) of more than 78%, the relative concentration reduction ratio of fine and sieved soil particles of more than 96%. Therefore, in the new train design, changes should be done in side skirt boards of the bogie, and the front and rear cover spoilers of the original case, thus to effectively reduce the particle accumulation in the bogie area of new trains.
Data Availability Statement
The original contributions presented in the study are included in the article/Supplementary Material, further inquiries can be directed to the corresponding author.
Author Contributions
YL: experiment design, data collection and analysis, writing; GL: experiment design and data collection; JZ: conceptualization, validation, methodology, supervision and writing.
Funding
This work is supported by the Second Tibetan Plateau Scientific Expedition and Research Program (2019QZKK020611), the Scientific and Technological Research and development plan of China Railway Urumqi Bureau Group Co., Ltd. (WLMQ-KGHZGS-HRTLGGB-2020-0031), the Major Science and Technology Project of Gansu Province (21ZD4FA010), and the Fundamental Research Funds for the Central Universities (lzujbky-2020-cd06, lzujbky-2020-pd11).
Conflict of Interest
The authors declare that the research was conducted in the absence of any commercial or financial relationships that could be construed as a potential conflict of interest.
Publisher’s Note
All claims expressed in this article are solely those of the authors and do not necessarily represent those of their affiliated organizations, or those of the publisher, the editors and the reviewers. Any product that may be evaluated in this article, or claim that may be made by its manufacturer, is not guaranteed or endorsed by the publisher.
Supplementary Material
The Supplementary Material for this article can be found online at: https://www.frontiersin.org/articles/10.3389/feart.2022.829309/full#supplementary-material
References
Allain, E., Paradot, N., and Ribourg, M. (2014). Experimental and Numerical Study of Snow Accumulation on a High-Speed Train. Int. Symp. Appl. Aerodynamics.
Andersson, E. (2012). Green Train Concept Proposal for a Scandinavian High-Speed Train: Final Report, Part B. Stockholm: KTH Railway Group.
Azuma, T., Horikawa, S., and Fujino, K. (2010). Countermeasure against Snow. JR East Technical Review 16, 31–34.
Cai, L., Lou, Z., and Li, T. (2021). Numerical Study on the Effects of Anti-snow Deflector on the Wind-Snow Flow underneath a High-Speed Train. J. Appl. Fluid Mech. 14 (1).
Cross, D., Hughes, B., Ingham, D., and Ma, L. (2015). A Validated Numerical Investigation of the Effects of High Blockage Ratio and Train and Tunnel Length upon Underground Railway Aerodynamics. J. Wind Eng. Ind. Aerodynamics 146, 195–206. doi:10.1016/j.jweia.2015.09.004
Gao, G.-j., Zhang, Y., and Wang, J.-b. (2020). Numerical and Experimental Investigation on Snow Accumulation on Bogies of High-Speed Trains. J. Cent. South. Univ. 27 (4), 1039–1053. doi:10.1007/s11771-020-4350-x
Gao, G., Chen, Q., and Zhang, J. (2020). Numerical Study on the Anti-snow Performance of Deflectors on a High-Speed Train Bogie Frame. J. Appl. Fluid Mech. 13 (5), 1735–3645.
Isyumov, N., and Mikitiuk, M. (1990). Wind Tunnel Model Tests of Snow Drifting on a Two-Level Flat Roof. J. Wind Eng. Ind. Aerodynamics 36 (1-3), 893–904. doi:10.1016/0167-6105(90)90086-r
Janjua, Z. A. A., Turnbull, B., Choy, K.-L., Pandis, C., Liu, J., Hou, X., et al. (2017). Performance and Durability Tests of Smart Icephobic Coatings to Reduce Ice Adhesion. Appl. Surf. Sci. 407, 555–564. doi:10.1016/j.apsusc.2017.02.206
Kloow, L. (2011). High-speed Train Operation in winter Climate. Stockholm: Transrail Publication BVF5KTH Railway Group Publication.
Knudsen, C., Slivsgaard, E., Rose, M., True, H., and Feldberg, R. (1994). Dynamics of a Model of a Railway Wheelset. Nonlinear Dyn. 6 (2), 215–236. doi:10.1007/bf00044986
Kumar, G., Gairola, A., and Vaid, A. (2020). Flow and Deposition Measurement of Foam Beads in a Closed Re-circulating Wind Tunnel for Snowdrift Modelling. Flow Meas. Instrumentation 72, 101687. doi:10.1016/j.flowmeasinst.2019.101687
Kuroiwa, D., Mizuno, Y., and Takeuchi, M. (1967). Micromeritical Properties of Snow. Phys. Snow Ice: Proc. 1 (2), 751–772.
Kwon, H.-b., Park, Y.-W., Lee, D.-h., and Kim, M.-S. (2001). Wind Tunnel Experiments on Korean High-Speed Trains Using Various Ground Simulation Techniques. J. Wind Eng. Ind. Aerodynamics 89 (13), 1179–1195. doi:10.1016/s0167-6105(01)00107-6
Liu, M., Wang, J., Zhu, H., Krajnovic, S., Zhang, Y., and Gao, G. (2020). A Numerical Study on Water spray from Wheel of High-Speed Train. J. Wind Eng. Ind. Aerodynamics 197, 104086. doi:10.1016/j.jweia.2019.104086
Niu, J., Liang, X., and Zhou, D. (2016). Experimental Study on the Effect of Reynolds Number on Aerodynamic Performance of High-Speed Train with and without Yaw Angle. J. Wind Eng. Ind. Aerodynamics 157, 36–46. doi:10.1016/j.jweia.2016.08.007
Shishido, M., Kurihara, Y., Takahashi, D., Kamata, Y., and Iikura, S. (2014). A Simplified Method for Estimating Outflow from the Bottom of Snowpack. QR of RTRI 55 (3), 157–163. doi:10.2219/rtriqr.55.157
Shishido, M., Nakade, K., and Ido, A. (2009). Development of Deflector to Decrease Snow-Accretion to Truck of a Vehicle (In Japanese). Rtri Rep. 23 (3), 29–34.
Sui, Y., Niu, J., and Yuan, Y. (2020). An Aerothermal Study of Influence of Blockage Ratio on a Supersonic Tube Train System. J. Therm. Sci., 1–12. doi:10.1007/s11630-020-1281-7
Tian, H. Q. (2015). Development of Research on Aerodynamics of High-Speed Rails in China (In Chinese). Strateg. Study CAE 17 (4), 30–41.
Tominaga, Y., Okaze, T., and Mochida, A. (2011). CFD Modeling of Snowdrift Around a Building: An Overview of Models and Evaluation of a New Approach. Building Environ. 46 (4), 899–910. doi:10.1016/j.buildenv.2010.10.020
Vajda, A., Tuomenvirta, H., Juga, I., Nurmi, P., Jokinen, P., and Rauhala, J. (2014). Severe Weather Affecting European Transport Systems: the Identification, Classification and Frequencies of Events. Nat. Hazards 72 (1), 169–188. doi:10.1007/s11069-013-0895-4
Wang, J., Zhang, J., Xie, F., Zhang, Y., and Gao, G. (2018). A Study of Snow Accumulating on the Bogie and the Effects of Deflectors on the De-icing Performance in the Bogie Region of a High-Speed Train. Cold Regions Sci. Techn. 148, 121–130. doi:10.1016/j.coldregions.2018.01.010
Wang, J., Zhang, J., Zhang, Y., Liang, X., Krajnovic, S., and Gao, G. (2019). Impact of Rotation of Wheels and Bogie Cavity Shapes on Snow Accumulating on the Bogies of High-Speed Trains. Cold Regions Sci. Techn. 159, 58–70. doi:10.1016/j.coldregions.2018.12.003
Wang, T.-t., Jiang, C.-w., Gao, Z.-x., and Lee, C.-h. (2017). Numerical Simulation of Sand Load Applied on High-Speed Train in Sand Environment. J. Cent. South. Univ. 24 (2), 442–447. doi:10.1007/s11771-017-3446-4
Wang, Z., Pan, X., and Liu, X. (1988). Simulation Research of Snow Drift on the Cut and Tunnel in Wind-Tunnel. Acta Geographica Ainica (03), 265–273.
Wu, Y., Liu, Y., Chen, H., Chen, Y., and Xie, D. (2019). An Investigation into the Failure Mechanism of Severe Abrasion of High-Speed Train Brake Discs on Snowy Days. Eng. Fail. Anal. 101, 121–134. doi:10.1016/j.engfailanal.2019.03.014
Xie, F., Zhang, J., Gao, G., He, K., Zhang, Y., Wang, J., et al. (2017). Study of Snow Accumulation on a High-Speed Train's Bogies Based on the Discrete Phase Model. Jafm 10 (6), 1729–1745. doi:10.29252/jafm.73.245.27410
Zhang, J., Shao, Y., and Huang, N. (2014). Measurements of Dust Deposition Velocity in a Wind-Tunnel experiment. Atmos. Chem. Phys. 14 (17), 8869–8882. doi:10.5194/acp-14-8869-2014
Zhang, J., Teng, Z., Huang, N., Guo, L., and Shao, Y. (2016). Surface Renewal as a Significant Mechanism for Dust Emission. Atmos. Chem. Phys. 16 (24), 15517–15528. doi:10.5194/acp-16-15517-2016
Zhang, J., Wang, J., Wang, Q., Xiong, X., and Gao, G. (2018). A Study of the Influence of Bogie Cut Outs' Angles on the Aerodynamic Performance of a High-Speed Train. J. Wind Eng. Ind. Aerodynamics 175, 153–168. doi:10.1016/j.jweia.2018.01.041
Zhou, X., Kang, L., Gu, M., Qiu, L., and Hu, J. (2016). Numerical Simulation and Wind Tunnel Test for Redistribution of Snow on a Flat Roof. J. Wind Eng. Ind. Aerodynamics 153, 92–105. doi:10.1016/j.jweia.2016.03.008
Zhou, X., Zhang, T., Ma, W., Quan, Y., Gu, M., Kang, L., et al. (2021). CFD Simulation of Snow Redistribution on a Bridge Deck: Effect of Barriers with Different Porosities. Cold Regions Sci. Techn. 181, 103174. doi:10.1016/j.coldregions.2020.103174
Keywords: prevention, particle, accumulation, high-speed train, bogie, spoilers
Citation: Liu Y, Li G and Zhang J (2022) Wind Tunnel Studies on the Prevention of Particle Accumulation Onto the Bogie of High-Speed Train. Front. Earth Sci. 10:829309. doi: 10.3389/feart.2022.829309
Received: 05 December 2021; Accepted: 05 January 2022;
Published: 25 January 2022.
Edited by:
Ning Huang, Lanzhou University, ChinaReviewed by:
Tao Che, Northwest Institute of Eco-Environment and Resources (CAS), ChinaQingwen Zhang, Harbin Institute of Technology, China
Yaning Chen, Xinjiang Institute of Ecology and Geography (CAS), China
Copyright © 2022 Liu, Li and Zhang. This is an open-access article distributed under the terms of the Creative Commons Attribution License (CC BY). The use, distribution or reproduction in other forums is permitted, provided the original author(s) and the copyright owner(s) are credited and that the original publication in this journal is cited, in accordance with accepted academic practice. No use, distribution or reproduction is permitted which does not comply with these terms.
*Correspondence: Jie Zhang, zhang-j@lzu.edu.cn