- 1Department of Earth and Environmental Sciences, University of Kentucky, Lexington, KY, United States
- 2Embrapa Pantanal, Corumbá, Brazil
- 3Embrapa Agricultura Digital, Campinas, Brazil
- 4Universidade Federal de Mato Grosso do Sul, Câmpus do Pantanal, Corumbá, Brazil
Shallow lakes in tropical floodplains provide significant ecosystem services that can be altered by natural and anthropogenic forces. Despite their importance, little is known about the infill patterns and timescales and the magnitude of these changes in tropical floodplain lakes. Here, we present a global meta-analysis of sediment core-derived accumulation rate data for shallow floodplain lakes in tropical lowlands to quantify the timescales of basin infill. Environmental variables (e.g., sediment accumulation rates, bathymetry, surface area) were compiled from the literature or derived from remote sensing imagery, resulting in a database (n = 76 lakes) that includes various lake morphologies. Our results show an exponential increase in sediment accumulation rates in many of these lakes over the past 50 years, which we interpret as a response to growing human populations and deforestation, particularly in topographically steep watersheds with pronounced seasonal rainfall. Over centennial periods, tropical floodplain lakes accumulate sediment faster than many other extratropical lakes. The dataset suggests that complete infill of some tropical floodplain lakes will occur in as little as a few centuries. Our findings also reveal the critical environmental and human factors that influence sediment accumulation patterns and affect ecosystem services in shallow tropical floodplain lakes. These findings have important implications for water and sediment management in low latitude watersheds, many of which are located in densely populated and/or developing nations.
1 Introduction
Tropical floodplain lakes provide important ecosystem services that are often overlooked (Street and Semenov, 1990). Less than 10% of all lakes are situated in the tropics, but their importance is disproportionately large compared with their number or aggregate surface area (Lewis, 1996). Tropical floodplain lakes provide supporting, provisioning, regulating, and cultural services that are critical to regional livelihoods and add to the natural heritage of low latitude regions (Apitz, 2011; Settele et al., 2014). For example, floodplain lakes in the Pantanal Basin in central South America, the world’s largest tropical wetland, showcase a wide variety of valuable ecosystem services (McGlue et al., 2011; Assine et al., 2015; McGlue et al., 2015). Supporting services consist of primary and secondary productivity, which contribute to biodiversity and form the base of food webs. Aquatic plant biomass is high in many tropical floodplain lakes due to the extended growing season and high mean annual air temperature (Talling, 2001); such is the case in several of the Pantanal’s large floodplain lakes (Lo et al., 2017; Sílio-Calzada et al., 2017). Provisioning services include natural resources, such as fisheries, or irrigation water for agriculture that directly support economic assets and development. For example, saline-alkaline lakes in the Pantanal of Nhecolândia precipitate mineral-rich sediments that support traditional cattle ranching and sustainable organic beef production (Guerreiro et al., 2019). Regulating services include the storage, transformation, and transportation of nutrients and other biochemical compounds that sustain the healthy functioning of the broader ecosystem (Settele et al., 2014). Recently, Rasbold et al. (2021) argued that an important regulating service of the Pantanal’s lakes is long-term atmospheric carbon sequestration. Cultural services include the aesthetic and educational value that lakes bring to a region and its people, including opportunities for ecotourism (e.g., Street and Semenov, 1990; Schulz et al., 2019). The “Large Lakes of the Pantanal” have served as trade corridors for the fishers, hunters, and gatherers among pre-colonial indigenous populations, and continue to provide ecotourism and sport fishing opportunities today (Peixoto, 2009; Oliveira and Milheira, 2020).
Ecosystem services tied to lakes are dependent on the maintenance of pelagic (open water) environments and the presence of a water column. When aquatic environments shift to alternative states due to changes in climate or human engineering, so too may ecosystem services transform, or in some instances, be lost entirely (e.g., Colloff and Baldwin, 2010; Nobre et al., 2020). For example, excessive summer rainfall can impact the rate of river avulsions in the plains of the Pantanal (Bergier et al., 2018), whereas extreme drought can lead to reduced water availability, resulting in fish stock depletion and food insecurity for ribeirinhos, local people living closer to floodplains (Marengo et al., 2020). Floodplain lakes and wetlands associated with meandering rivers can permanently transform into dry landforms over decades or centuries with avulsions (Assine et al., 2015), fundamentally altering the spatial distribution of open water environments and ecosystem services (Louzada et al., 2020; Louzada et al., 2021).
Sedimentation influences the persistence of open water environments and the lifespan of tropical floodplain lakes. Natural or human-driven changes that increase sediment loads to lakes, through distinct inlet channels, overbank sedimentation, or from overland flow, may reduce open water persistence. In the Pantanal, Lo et al. (2019) observed accelerating sediment accumulation over the past 100 years in Lake Uberaba that has resulted in a shoaling and contraction of the Pantanal’s largest floodplain lake. Shallow floodplain lakes in the Pantanal are relatively well-studied compared with other tropical lowland lakes. However, it remains unknown if observations from the Pantanal are representative of tropical floodplain lakes more broadly. Out of 422 lakes in a recent global study of lake sedimentation, < 5% were in tropical climates (Baud et al., 2021).
Here, a meta-analysis of floodplain lake data from the tropics attempts to address this knowledge deficit. Three questions motivate this work: 1) At what rates do tropical floodplain lakes accumulate sediment? 2) How rapidly will extant tropical floodplain lakes completely fill with sediment, given current trajectories in sedimentation and environmental conditions? 3) What environmental factors control sediment accumulation rates in these settings? To address these questions, data from tropical floodplain lakes (e.g., sediment core-based accumulation rates, morphometrics, bathymetry) were compiled using the peer-reviewed literature, supplemented where necessary with new bathymetric modeling and remote sensing data.
2 Materials and Methods
The criteria used to establish an internally consistent database that allows for the analysis of tropical floodplain lake attributes are described as follows. The database was compiled using published papers on lake studies that were electronically searched using keywords including “tropics lake sediment [place],” “lake sediment tropics 14C,” “lake sediment core tropics 210Pb,” “lake paleoclimate [country],” and “geochronology floodplain lake [location].” The tropical lowland floodplain lakes considered here were classified as one of five types, all of which are present in the Pantanal wetlands, however, the classes of lakes were not used as keywords. The Pantanal region (western Brazil) was used as the main reference due to the availability of raw data and the presence of different types of lakes (Figure 1). Wetzel (2001) defined three classes of floodplain lakes based on morphology, including (channel, dish, and oxbow lake types). Here, two additional floodplain lake types were included: distal lakes and blocked valley lakes. Channel lakes experience high hydraulic throughflow during river flooding and are often characterized by seasonal or perennial tie channels to a major river. A subset of the channel lakes is oxbow lakes, which occur where a meander is cut off from an active river channel. Distal lakes are a subclass of channel lakes defined by their location on the terminal fringe of a distributary fluvial system (e.g., Hartley et al., 2010). Blocked valley lakes extend away from the floodplain, and their outlets have been restricted by levee buildup or resistant channel-margin lithologies that help to pond water. Dish lakes are typically isolated from river channels and are sustained by a combination of flood waters, precipitation, and groundwater input. Dish lakes often experience fluctuations in water level and hydrochemistry stemming from their closed hydrology and evaporation. Many dish lakes are found in the Nhecôlandia region of the central Pantanal, an abandoned lobe of the Taquari River megafan (Figure 1) (Furian et al., 2013; McGlue et al., 2017).
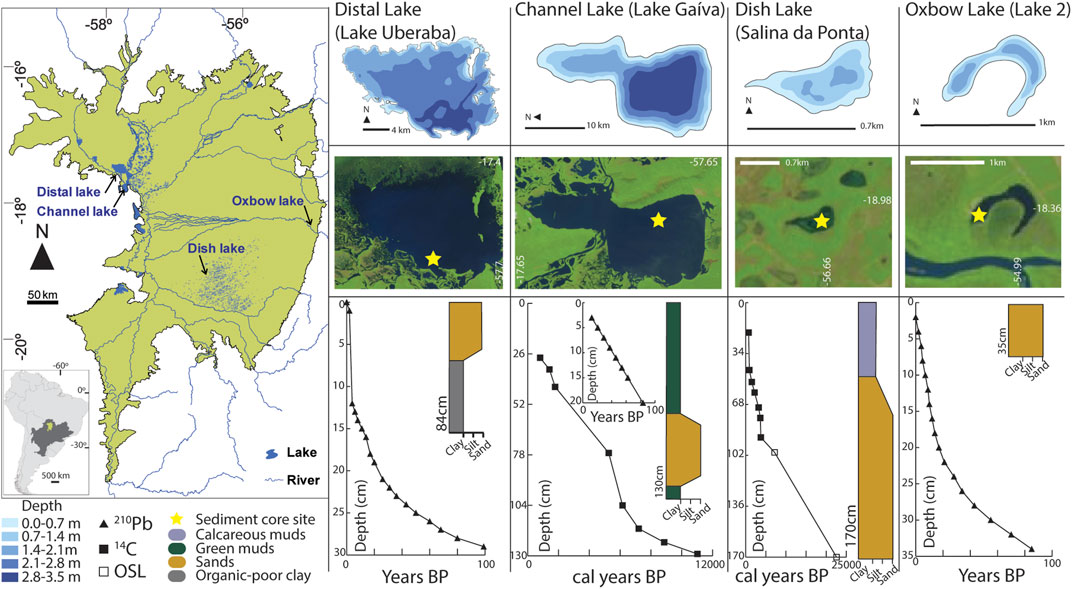
FIGURE 1. Map of the Pantanal lowlands (green shaded area, left) and endmember floodplain lake types present in the basin. Age-depth models, stratigraphy, and bathymetry were collated from published articles and matched with satellite images (Sentinel 2, 10 m resolution, January 22–24, 2019).
To be included in the database, lakes needed to meet the criteria defined here. Lakes needed to be located in tropical rainforest (Af), tropical monsoon (Am), or tropical savanna (Aw) Köppen climate regions (Beck et al., 2018). Shallow depths are typical for floodplain lakes; a ∼13 m threshold was selected for this study, though other authors have set lower maximum values (Hamilton and Mitchell, 1996; Hayashi and van der Kamp, 2000; Padisák and Reynolds, 2003). Lowland environments consist of areas affected by annual river flooding (Erenstein, 2006) and with elevation ≤500 m above mean sea level (a.m.s.l.). In order to be included in the database, lakes that met the environmental criteria described above needed at least one sediment core that was dated using radiocarbon (14C), optically stimulated luminescence (OSL), or lead-210 (210Pb). In most cases, lakes in the database lack published bathymetric maps (a common limitation in other studies; see also Ang et al., 2021; Liu and Song, 2022). However, average lake depth values were commonly available, and these data were employed in the calculation of lake volume. Lakes without any depth information were excluded. Artificial lakes, reservoirs, and impoundments were also not considered in this study (Figure 2).
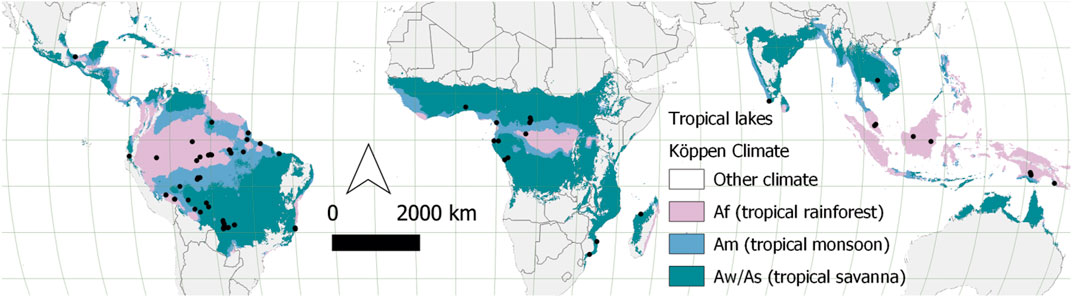
FIGURE 2. Global distribution of the 76 lakes included in this study across the Köppen tropical climate types.
2.1 Linear and Mass-Based Sediment Accumulation Rates
Linear sediment accumulation rates (LAR, mm/yr) were computed by dividing the length of a sediment core section by the time interval established by 14C or OSL to provide a long-term context to the more recent changes in accumulation rates. For lakes with sediment cores dated with 210Pb, age models were computed using unsupported or “excess” 210Pb (210Pbxs) and the constant rate of supply (CRS) model, which provides information on sediment accumulation over the last ∼100–150 years depending on 210Pbxs activity concentrations and allows for variability in sedimentation rates over time (Appleby and Oldfield, 1978; Ritchie and McHenry, 1990; Appleby and Oldfield, 1992). In contrast, lake studies that utilized the constant initial concentration (CIC) or constant flux:constant sedimentation (CF:CS) models only reported one mean sedimentation rate for entire cores. To facilitate a comparison of rates among studies/cores whose 210Pb data were modeled with CRS, CIC, and CF:CS, we calculated a mean rate for each core modeled by CRS using linear regression (Binford et al., 1993). This mean rate encompassed all data points available in the published literature.
Where older sediment was recovered in relatively long cores, 14C and OSL age dates were used to calculate long-term sediment accumulation rates over hundreds or thousands of years from the core basal age. Several studies published in the 1980s and 1990s that were included did not report calibrated 14C age dates; in those cases, 14C dates were converted to calendar ages using the online software Calib 8.20 and Calibomb (Reimer and Reimer, 2004; Stuiver et al., 2021). The SHCal20 curve was selected for lakes in the Southern Hemisphere, whereas the IntCal20 curve was used for lakes in the Northern Hemisphere (Hogg et al., 2013; Reimer et al., 2020). The median of the calibrated age range was used as the calendar age at a given core depth. Although Blaauw and Christen (2005) reported tradeoffs associated with using the median 14C age, most cores have fewer than five dated horizons each, thereby minimizing the potential error of this approach. Age inversions were excluded from rate calculations. We adopted the framework of Sadler (1981) for comparisons among cores with 14C or OSL age-depth models. This technique afforded the opportunity to test the influence of measurement duration on sediment accumulation rate among sites.
Mass accumulation rates (MAR, g/cm2/yr) were determined by multiplying the LAR by sediment dry bulk density (g/cm3), which was estimated based on grain size when such information was unavailable in the original reference (Avnimelech et al., 2001; Verstraeten and Poesen, 2001; Shi et al., 2003). Sediment accumulation rates must be reported as a volumetric rate (g/cm2/yr) to obtain the most accurate, quantitative estimates of infill (Clemens et al., 1987). When the dry bulk density was not reported, we approximated sediment lithologic composition and clay content based on the core details presented in the study and applied the conversion of Shi et al. (2003) for non-marine sediments. Such an assumption is a potential source of uncertainty in the calculations but is unavoidable for reasonable estimates of lake infill time.
2.2 Lake Infill
Lake infill is defined here as the amount of time needed to entirely fill a lake and create a new aquatic environment (e.g., a marsh or river floodplain) and assuming that the sediment accumulation rate will continue unchanged into the future. In order to estimate lake infill time, it is important to have accurate data on lake basin volume. Lake volume was determined by multiplying the lake surface area by the mean depth, or depth of the coring station when mean depth was unavailable. For lakes that experience a large seasonal change in surface area, we selected the annual mean lake extent from cloud-free satellite imagery. In contrast, we imported a point cloud of individual depth measurements or digitized isobaths to QGIS 3.4 if detailed bathymetric data were available. A Delaunay triangular interpolated network (TIN) was then calculated and converted into raster format to derive surface volume in ArcMap 10.7.1. The infill time estimates were determined by dividing the lake volume by the MAR.
2.3 Statistical Analysis
The database of sedimentation rate versus duration of measurement was plotted on a log scale and the linear regression was associated with a power law. These regression analyses provided the most straightforward interpretation in log-log plots to accommodate the large range of values. In addition, regression analysis is widely applied when the response variable and the explanatory variable are continuous (Crawley, 2012). However, exponential functions were more suitable to describe accelerating rates of change for 210Pb-derived sediment accumulation rates.
Lake surface areas, elevations, numbers of inlet channels, and distances to the dominant river channel were compiled for all lakes in the database, either from the literature or measured directly from Google Earth™ images. The lake watershed area, including potential riverine input during the wet season, was delineated in QGIS 3.4 using shuttle radar topography mission data (SRTM; 30 m resolution). The watershed variables were calculated by: 1) converting the digital elevation model (DEM) to a projected and equal-area coordinate system, 2) filling in sinks (Wang and Liu, 2006), 3) extracting a channel network and drainage basins (Conrad et al., 2015), 4) calculation of upslope area (O’Callaghan and Mark, 1984), 5) conversion to polygon, and 6) calculation of zonal statistics. The average and maximum slopes were derived from the same DEM using the slope function in the Raster Terrain Analysis toolbox of QGIS 3.4. Average annual rainfall and temperature were derived from the WorldClim data set (Fick and Hijmans, 2017).
In order to aid in the interpretation of controls on sediment accumulation in these lakes, ten environmental and human factors (lake depth, surface area, elevation, distance to the dominant river channel, average slope of watershed, annual rainfall, short-term accumulation rate, infill time, watershed human population density, and tree loss) were compiled using Paleontological Statistics (PAST) software (Hammer et al., 2001). In the 210Pb dataset (see Supplementary Material), we applied multivariate statistics through the principal components analysis (PCA) technique using the “correlation” option, which normalizes all variables using division by their standard deviations; this is appropriate given that the matrix contains variables with many different units and has been applied successfully in other tropical lake environments (e.g., Parinet et al., 2004). The PCA finds a small number of linear combinations in the variables to capture most of the variation in the data frame (Crawley, 2012). Tree loss and population density were utilized in this study to approximate the anthropogenic influence on shallow lake sediment accumulation. Tree loss was calculated from publicly available University of Maryland data over 2000 to 2012 (Hansen et al., 2013, version 1.0). This value (0–1, where 1 is complete loss of forest cover) does not specify land uses but rather expresses whether a given area lost tree cover (≥5 m canopy) between 2000 and 2012. The population density information was taken from a publicly available global shapefile and averaged across each watershed (Center for International Earth Science Information Network, 2018). Multivariate statistics was not possible for long-term MAR dataset, because variables such as land use are not available over the thousands of years that the 14C age models span.
3 Results
3.1 Lake Morphometry
Seventy-six 76) lakes met the selection criteria and were included in the database. Most lakes (n = 61) are situated in the southern hemisphere, but the full range falls within 18°N and 25°S (Figure 2). Most lakes in the database are in tropical savanna regions, fewer in the tropical rainforest and tropical monsoon climate regions. The altitude of all lakes ranged between <1 and 391 m a.m.s.l., and the average annual precipitation at the lakes ranged between 780- and 6,323-mm. Watershed size varied from 17 to 5 × 106 km2, with a median of 21,750 km2, and the average watershed slope ranged from 0.5° to 13.4°, with a median of 3.2°. Maximum lake depths ranged from 1.0 to 13.0 m, with a median of 2.6 m. The deepest lakes were channel and blocked valley types. Lake surface areas ranged from 0.02 to 2,500 km2, with a median of 1.70 km2. Lake volumes ranged from 4 × 10–5–19 km3, with a median of 0.006 km3. Channel lakes (n = 38) comprised 50% of the data set, whereas blocked valley (n = 15) and dish lakes (n = 12) were less common. Oxbow lakes (n = 9) were found near the hinterlands of the Pantanal and the Amazon River basins, and therefore these lakes are situated on relatively high elevation floodplains. Only two examples of distal lakes were available: Lake Uberaba (Pantanal) and Lake Pemerak (Borneo). Most lakes in the database (n = 46) are hydrologically open, whereas the remaining lakes are closed at the surface and lack active channel connections to a proximal river. Many lakes in the database had only one inlet channel (n = 24) or lacked inflowing streams altogether (n = 25). In most cases (n = 49), the distance from the sediment coring station to the nearest inlet channel was ≤2 km. The straight-line distance from the coring station to the nearest major river channel, excluding tie channels, was ≤5 km for most lakes in the database (n = 43).
3.2 Lake Sediment Accumulation Rates
3.2.1 Long-Term Sediment Accumulation Rates (14C and OSL Dating)
The range of 14C-derived sediment accumulation rates, referred to herein as long-term rates, was 0.02–8.20 mm/yr. (Table 1). Similar to Sadler (1981), long-term rates for each lake core were plotted against the duration of measurement (Figure 3). The fitted power functions for this study (n = 74) and for global lakes (n = 860) from Sadler (1981) were significantly different with a 99% confidence interval. When plotting the LARs of discrete intervals between two adjacent dated core samples, the dish and blocked valley lake data sets were each characterized by power function slopes > −0.9 (Figure 4). The channel and oxbow lake data sets were approximately −0.7, so over durations of measurement of 102–104 years, their average LARs increase rapidly in the last century but less so compared with the dish and blocked valley lakes. Distal lakes (here only Lake Melintang, Indonesia) experienced the least change in sediment accumulation but consistently accumulated sediment on the upper end of all long-term LARs in this study.
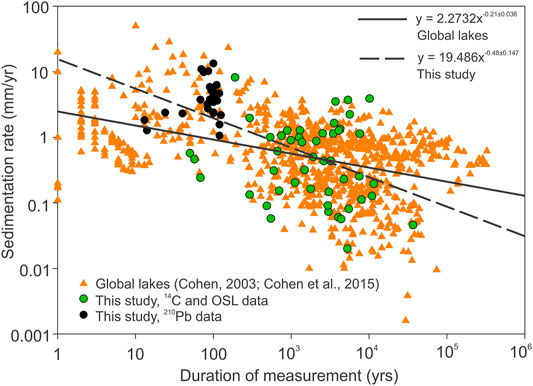
FIGURE 3. Global lacustrine sediment accumulation rates (orange triangles; Cohen, 2003; Cohen et al., 2015) and plotted against the accumulation rates found in this study (adapted from Sadler, 1981). Each lake with 210Pb chronology is represented by one black circle, including CRS, CIC, and CF:CS models. Lakes with 14C and OSL chronology are shown as green circles. The inverse power law function for this study shows greater sediment accumulation rates for periods of <1,000 years than for the global lakes data set.
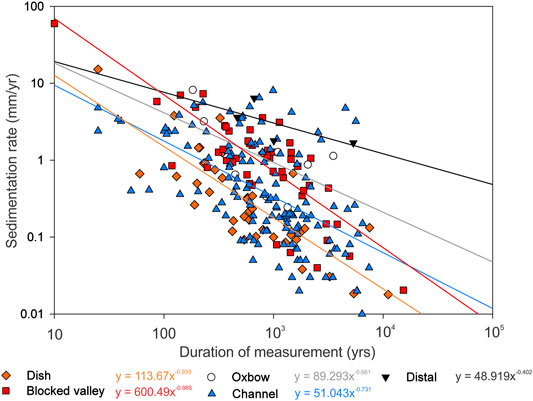
FIGURE 4. 14C ages for every available core in the database. Dish and blocked valley lake data indicate that their rates of change are faster than those of oxbow and channel lakes. The distal lake trend suggests a higher but approximately constant sediment accumulation rate over time, independent of duration of measurement.
3.2.2 Short-Term 210Pb-Derived Sediment Accumulation Rates
The range of 210Pb-derived sediment accumulation rates, referred to herein as short-term rates, was 1.06–5.18 mm/yr. (Table 1; Figure 3). On tropical floodplains, lake LARs have increased exponentially by five-to six-fold in the past century. Most of these increases occurred from the 1960s onward across all lake types (Figure 5). Prior to 1960, the dish and blocked valley lakes recorded LARs that were higher than the LARs of channel, oxbow, and distal lakes. After the 1960s, channel and oxbow lakes notably recorded more sediment influx and deposition. Though consistent with the pattern of increasing sedimentation rates through time, the distal lakes class records the most modest increase of all lakes in the database (Figure 5). Among three cores (Lakes Negra, Castelo, Caranã) where both 210Pb and 14C were available, the average 14C -based linear accumulation rate was one order of magnitude lower than the averaged 210Pb accumulation rate.
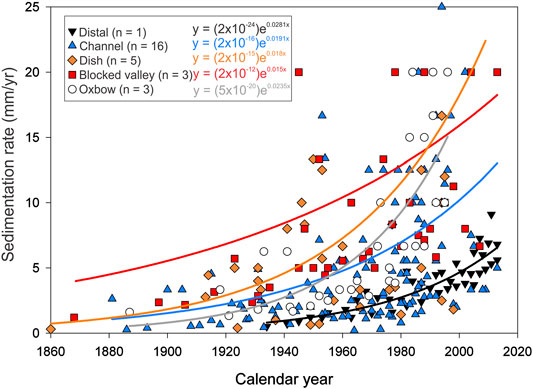
FIGURE 5. The 210Pb data from every available core interval including CRS, CIC, and CF:CS models illustrate an exponential acceleration in sediment accumulation rates over the last ∼50 years. Lake sediment accumulation rates dated with CIC and CF:CS models are represented by one point. The dish and blocked valley lake types have experienced more rapid increases in sediment accumulation compared with the other lake types.
3.3 Lake Infill Chronology
Lake infill times estimated from long-term MARs and lake volumes are between 1,000 and 100,000 years; MARs are on the order of 0.01–0.10 g/cm2/yr. using 14C or OSL datasets (Figure 6A). Alternatively, the infill time based on short-term (210Pbxs-based) rates ranged from 100 to 10,000 years, with MARs on the order of 0.1–1.0 g/cm2/yr. (Figure 6B).
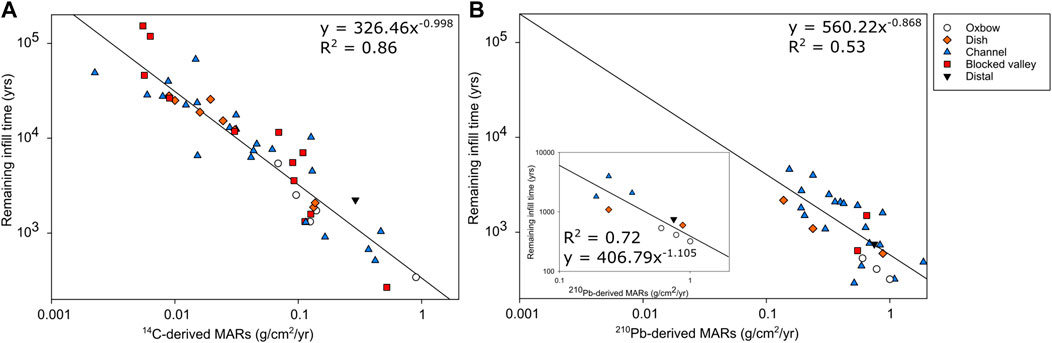
FIGURE 6. Whole-core average sediment accumulation rates are plotted to illustrate how 210Pb-derived rates in panel (A) and 14C-derived mass accumulation rates in panel (B) affect infill time estimates.
Volume estimates for lakes where both average depth and detailed bathymetry were available were used to estimate the potential error arising from the use of generalized depth information (Figure 7). In the ten lakes where bathymetric data were available (Table 2), the lake volume estimates were consistently lower than those determined using only a single mean lake depth, indicating that the application of average depth values artificially increases lake volume. Our comparisons using TINs of bathymetry in ArcGIS resulted in calculations that were consistently lower than those of simple volumes, in some cases >80% lower (Figure 7).
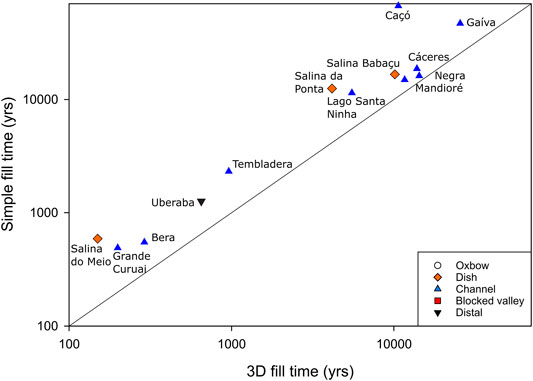
FIGURE 7. 3D infill time versus simple estimates of infill time based on an average lake depth value. The diagonal 1:1 line denotes identical fill time for simple and 3D volumes; values above the line occur when the simple fill time exceeds the 3D fill time.
3.4 Statistical Analysis
The linear regression shows a negative correlation between 210Pb-derived sedimentation rates and time (Figure 3). When compared with a global lakes study published by Sadler (1981) (y = 2.2732x−0.21±0.036), our results show that tropical lakes present a lower negative correlation (y = 19.486x−0.48±0.147), meaning that lake infill has accelerated through time. With respect to the long-term 14C-derived rates, the distal lake type presented the highest negative correlation (y = 48.919x−0.402) and the blocked valley lake type presented the lowest negative correlation (y = 600.49x−0.985) (Figure 4). The most extreme trajectory towards fast sedimentation rates occurs in the dish lake setting, most specifically after 1960 CE (Figure 5).
The PCA of lake depth, surface area, elevation, distance to the dominant river channel, average watershed slope, annual rainfall, short-term LAR, infill time, population density, and tree loss) revealed that PC1 and PC2 account for ∼46% of the total variance (Figure 8). Principal component 1 accounted for 27% of the variance and loaded positively on lake depth, annual rainfall, tree loss, short-term LAR, average slope, and population density (Figure 8). The distance to the dominant river channel, elevation, and infill time loaded negatively in the PC1. Thus, PC1 reflects key controls on lake sedimentation and infill time. We interpret that the lakes in low elevation, deforested watersheds marked by high annual rainfall infill with sediment comparatively faster than those at higher elevations with native vegetation or intact managed forests. The lack of orthogonality of infill time, however, suggests that PC2 is an equally important control. Watershed average slope and population density loaded positively on PC2 and negatively for lake depth and infill time. Such a pattern emerging from PC2 may reflect erosion within the watershed, accelerated by the landscape gradient, human settlement, and land-use changes. This result suggests that lakes found in steeper watersheds with higher populations are susceptible to more rapid infill than those located in low gradient, sparsely populated watersheds. These results have important implications for the effective management of lake resources at the watershed scale.
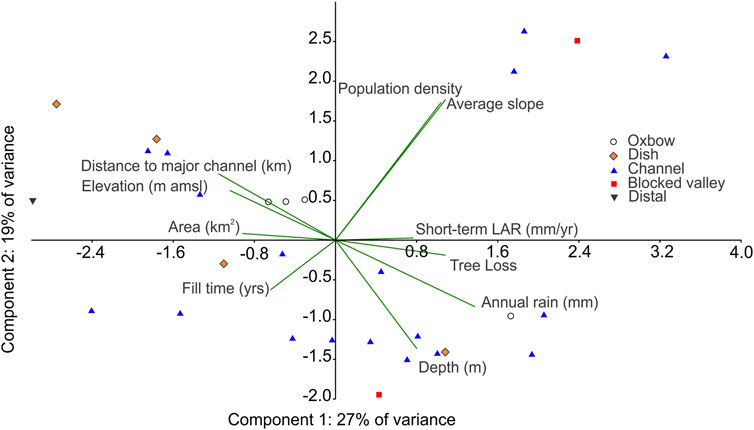
FIGURE 8. Principal component analysis (PCA) plot. PC1 and PC2 account for 46% of the total variance based on the 29 lakes with available 210Pb chronology in our dataset.
4 Discussion
4.1 Recent Sediment Accumulation Rates Increasing in Tropical Lowland Lakes
The multivariate and linear regression analyses of tropical floodplain lake datasets conducted in this study suggest that deforestation and human population densities may explain the onset of increasing rates of sedimentation in the second haft of the 20th century independent of soil type. There is a large body of literature that implicates land-use changes by humans, particularly deforestation, as causal agents of erosion not only in the tropics but in other climatic regions (e.g., Owens et al., 2005; Syvitski and Kettner, 2011; Restrepo et al., 2015). For example, Ivory et al. (2021) reported that deforestation and reduction of tree cover have led to increased sediment input into Lake Tanganyika in the last few decades. Similarly, Baud et al. (2021) found that global lake sedimentation rates have increased by nearly four times since the “Great Acceleration” (post-1960s). Schiefer et al. (2013) reported a steadily increasing lake sedimentation rate in the late 20th century in western Canada linked to the oil, gas, and timber extraction industries. Deforestation in much of the rest of the world increased rapidly during the late 20th century, which has frequently been recorded in lacustrine records (e.g., Roulet et al., 2000; Dearing and Jones, 2003; Long et al., 2021). Now, the Global South, consisting of many developing nations in the tropics, has overtaken developed nations in population growth and resource consumption (Chant and McIlwaine, 2009; Adams et al., 2019; State of the Tropics, 2020). Population density in the tropics is projected to increase rapidly (e.g., Laurance et al., 2014), hence >50% of the world’s population is expected to reside in the tropics by 2050. Land conversion for livestock and agriculture has intensified in the tropics since the “Green Revolution” in the second half of the 20th century (e.g., Evenson and Gollin, 2003; Le Huong and Thanh Son, 2020; Sinha et al., 2020; Alavez-Vargas et al., 2021; Jankowski et al., 2021), leading to increased sediment supply to some lowland lakes. Owens (2020) describes that greater sediment accumulation rates have become a threat to water security. Increased sedimentation is likely to continue strengthening with the tree loss that often accompanies population growth (Wolman and Schick, 1967; Walling, 1999; Papangelakis et al., 2019).
Another mechanism by which tropical floodplain lakes may lose areas of open water is through colonizing vegetation (Barlow et al., 2018). Abundant rainfall and warm temperatures year-round contribute to the rapid proliferation of aquatic macrophytes (Chambers et al., 2008). Lakes with sediment accumulation rates >4 mm/yr. (e.g., Lake Uberaba, Brazil; Laguna La Tembladera, Peru; and Waigani Lake, Papua New Guinea) commonly develop marshy littoral zones leading to terrestrialization at the expense of open water environments (Osborne et al., 1993; López-Blanco et al., 2017; Lo et al., 2019). This phenomenon has been documented for the Paraguay River, as large floating islands of Cyperaceae (baceiros) were found to constrict channel margin environments (Costa and Telmer, 2006; Pott and Silva, 2015; Coutinho et al., 2018). Floating Cyperaceae mats grow and die rapidly, forming an aggrading, organic-rich soil that woody vegetation can colonize (Lemes do Prado et al., 1994; Pivari et al., 2008). Recent studies have suggested that this process influences floodplain lakes as well, resulting in considerable basinward expansion of the shoreline and shallow littoral zones at the expense of pelagic environments (Lo et al., 2017; Rasbold et al., 2021). Similarly, Ryken et al. (2015) found that sedge wetlands have a first-order control in retaining sediments and excess nutrient runoff from land-use changes.
Seasonally intense rainfall is another key characteristic of tropical climates that enhances erosion, sediment transport, and export to lake basins. For example, sediment accumulation patterns in the lake-wetland continuum are highly dependent on flood frequency and intensity (e.g., Sílio-Calzada et al., 2017). Rainfall erosivity combined with high intensity of land use changes or total removal of vegetation cover produce increased sediment delivery downstream (Krishnaswamy et al., 2001; Carlson et al., 2014). Recent data suggest that pulses of sediment export may become stronger with wetter rainy seasons and drier dry seasons in sub-Saharan Africa (Salerno et al., 2019). Similar severity of increased droughts and unprecedented flooding linked to Atlantic Ocean warming has been demonstrated for Amazonia (Barichivich et al., 2018). This suggests that floodplain lakes without perennial tie channel connections to a dominant river (e.g., oxbow lakes, dish lakes) may experience higher sediment influx.
The lacustrine sedimentary record in the Pantanal Basin illustrates the continuous impacts of population growth and deforestation. For example, the upper Taquari River oxbow lakes experienced increased sedimentation rates related to the expansion of planted pastureland and croplands (e.g., Leite et al., 2012). These unnamed oxbow lakes have sandy sediments that were likely derived from the upper watershed, which is part of the cerrado biome (composed of savannas and grasslands amid humid and dry forests), where the agricultural activity has been most severe, in evidence by large gullies, soil loss, and increased avulsions (e.g., Gomes et al., 2019; Louzada et al., 2020).
Our study results suggest that increased sediment transport, perhaps linked to deforestation and other land-use changes for agriculture that started in the 1950s–1970s, maybe driving rapid sediment accumulation in tropical floodplain lakes. The environmental variables that seem to most affect tropical floodplain lake infill time are annual rainfall and landscape features (including tree loss, elevation, and distance to the dominant fluvial channel). This outcome is consistent with Ndehedehe et al.’s (2021) results, who reported that anthropogenic activities and climate change have resulted in the accelerating decline of permanent open water features in tropical fluvial environments. Indeed, cropland density in temperate and high latitude lake watersheds has been implicated as the most direct predictor of accelerated sediment accumulation rates (Baud et al., 2021). This correlates closely with tree loss because the density of cropland frequently comes at the expense of tree cover (e.g., Gibbs et al., 2010). Globally, lowland areas have been more exploited for agricultural, and pastoral uses than relatively elevated hinterland regions, but highland cropland expansions in Southeast Asia are also increasing sediment inputs to lowland areas (Zeng et al., 2018). The distance to the dominant river channel controls the rate of overbank sedimentation, as hydrological connectivity is the main control of floodplain sediment accumulation rates (Walling and He, 1998; Rodriguez et al., 2019). The net cumulative effect of these factors is that the short-term sediment accumulation rates are much higher than those integrated over longer periods. Yet this two-dimensional infill requires consideration of three-dimensional space vis-à-vis lake volume.
4.2 Completion of Lake Infill
When comparisons were possible, our data (n = 13) showed that lake volumes calculated from single depth measurements produced inaccurate (overestimated) lake volumes. This has been reported previously for lakes in other climatic settings. Cross and Moore (2014) compared the volume estimates from variable bathymetric transect spacing and found that increased spacing produced lower volume estimates relative to a modeled volume for four lakes and one reservoir. None of Cross and Moore’s (2014) lakes are in floodplain environments, and so they likely function differently. This may help to explain the higher lake volume estimates relative to modeled volumes based on bathymetric data, which were on average 49% lower. Lakes in floodplains are complex open-water systems (Crisman et al., 2005). For example, paleotopography and the shape of the basin in shallow lakes can influence the infill pattern, which is clearly not captured by an average depth approximation (e.g., Debret et al., 2014; Bennett and Buck, 2016). The complex spatiotemporal distribution of sediments can vary with depth and distance to the nearest major river channel, such that completion of infill is best reported as a range (e.g., Kossoni and Giresse, 2010).
The infill times given in this study are maximum estimates because the true lake volumes are likely to be lower than current approximations based on a single reported depth at the coring site. Published studies of shallow tropical lakes, where completion of infill is expected on the order of hundreds or a few thousand years, further lend support to the accelerating infill process (e.g., Rasanen et al., 1991; Horbe et al., 2011; Aleman et al., 2013; Bremond et al., 2017). In the Pantanal Basin, five of the nine lakes with data are predicted to infill in <1,000 years, and four lakes will infill in <10,000 years according to this study. A similar pattern is predicted for shallow tropical lakes outside of the Pantanal, where lakes infill within 102–103 years (e.g., Kossoni and Giresse, 2010). The actual sediment accumulation rates in all lakes considered in this study may be higher considering the land use/land cover changes recorded in the tropical lowlands (e.g., Biswas and Dhara, 2019). We can be confident that the rate of accumulation of sediment volume is increasing throughout the lake basins (Bennett and Buck, 2016).
We show that some tropical floodplain lakes may infill in ≤150–200 years and demonstrate that small changes in lake depths have major implications for calculations of lake volume. Although recent middle-lower Amazon lake models have improved bathymetric estimates (e.g., Park et al., 2020; Ang et al., 2021), ground-truthing or field validation using hydrographic surveying remains extremely valuable as a snapshot of actual conditions at the time of the survey. An average depth or the depth at the lake depocenter continues to be a crude estimate of the true lake volume, which our meta-analysis reveals to be standard for most lake core studies and consequently, a significant source of error when attempting to quantify infill timescales. The Global Surface Water Explorer has been an important assessment tool for lake surface areas (Busker et al., 2019), so predictive modeling has improved for parts of the world with extensively published studies and high-resolution LiDAR data. Where limnogeology is a nascent field, as is the reality in the world’s tropical floodplain lakes with limited remote sensing, bathymetric surveys are sorely needed to validate lake volume estimates based on laser and radar altimetry (e.g., Carabajal and Boy, 2021). The lack of published depth data for small, shallow lakes remains a key limitation (e.g., Webster et al., 2022), but several remote sensing products have attempted to refine predictive bathymetric modeling. HydroLAKES include volume estimates for lakes >10 ha, and the GLOBathy repository provides a refined model for estimating bathymetry in lakes (Messager et al., 2016; Khazaei et al., 2022). This study did not include GLOBathy data because the 1,503 lakes with observational data that were used to validate the model were outside of the tropics. The HydroLAKES database assumes that the average depth can be reasonably predicted from surrounding topography, but the low gradient of floodplain environments betrays subtle but important variations of actual lake depth.
4.3 Aquatic Ecosystem Services
Lake volume reduction has an outsized impact on shallow small lakes, which are common in tropical floodplain environments (Nõges, 2009). Hydrological changes have large impacts because small changes will dramatically alter the submerged lake margins where the ratio of lake area to depth is high. The effects of lake surface area reduction have been reported in tropical mountain lake districts (Mosquera et al., 2017) and in semi-arid Brazilian lakes and reservoirs (Rocha et al., 2018; Nobre et al., 2020), but the impacts on fisheries, irrigation, and other ecosystem services remain unclear for tropical floodplain lakes (e.g., Tan et al., 2020). Quantitative estimates of the loss of ecosystem services in global shallow lakes are in the early stages of development (Xu et al., 2018; Janse et al., 2019), and existing datasets pertain to localized sites such as Lake Malombe, Malawi (Makwinja et al., 2021).
In the Pantanal, the intensive land use changes owing to cattle ranching and settlements that expanded in the 1970s have been implicated in higher rates of gully formation and land surface degradation of the upper Taquari River Basin (Louzada et al., 2020; Louzada et al., 2021). The Pantanal’s large floodplain lakes Gaíva, Mandioré, and Baía Vermelha, are directly connected to the Paraguay River via tie channels, and while these lakes are far from urban areas, they are immediately downstream of areas that have been deforested (McGlue et al., 2011; Schulz et al., 2019). The terrestrialization process can lead to conditions in which the extant flora and fauna struggle to thrive, which can influence local biodiversity (Lo et al., 2019; Abel et al., 2021). These changes in tropical lowlands are particularly critical given their traditional role as a sustainable support system for local populations both presently and historically (e.g., Shepard et al., 2020).
Varying resolution of global datasets of land use and soil type precluded their consideration in this study. Global anthropogenic land-use shows extensive development of pasture and cropland in areas outside of the tropics, except on the Indian subcontinent (Klein Goldewijk et al., 2011). Large swaths of South America were not systematically modified until the 20th century, so changes in land use that occurred in the 20th century are captured in the 210Pb core chronologies. One variable not specifically considered in our analysis was soil type. Soil types are cataloged in the United Nations Food and Agriculture Organization Soil Map of the World, but the scale of that map (1:5 million) is not adequate for small-scale watershed analyses (e.g., Reynolds et al., 2000; Selcer, 2015). Future refinement of the database could include soil information if it can be obtained at the watershed scale.
5 Conclusion
Since the 1960s, the rate of sediment accumulation in tropical floodplain lakes has increased by as much as an order of magnitude relative to previous periods. This outcome aligns with other studies that have noted shallow lakes are rapidly losing resilience to climate change, as documented by annual lake area decreases (e.g., Havens et al., 2016; Sílio-Calzada et al., 2017; Bartczak et al., 2019; Lo et al., 2019). The infill times for tropical floodplain lakes are between 1,000–10,000 years when using long-term sediment accumulation rate information, but the average infill times for these lakes decrease to 100–1,000 years when using the short-term sediment accumulation rates calculated using 210Pb data, and therefore warrant consideration in water and sediment management planning. We show that 14C, 210Pb, and OSL data help form expanded age-depth models to compare temporal changes to sediment accumulation, bypassing the temporal limitations of using only 210Pb models. Simple geometric lake volume estimates based on an average depth overestimated the true volume calculated from detailed bathymetry, which was available for <20% of the lakes in this study. Hence, the infill times are conservative maximum estimates in most cases and are likely to be much faster given that most lake volumes were overestimated when an average depth was assumed. Where possible, locally measured lake and watershed characteristics will provide an improved context for evaluating sediment infill time and the utility of remotely sensed global data sets. Importantly, the rapid loss of these tropical lowland floodplain lakes due to sediment infilling and terrestrialization could alter or eliminate ecosystem services supporting diverse species, fisheries, nutrient cycling, and water resources.
Data Availability Statement
The original contributions presented in the study are included in the article/Supplementary Material, further inquiries can be directed to the corresponding author.
Author Contributions
KY and MM conceived/designed the research. EL performed research. EL, KY, and MM analyzed the data sets. LD-L, IB, and AS provided important feedback and context for data analysis. EL, MM, and KY wrote the paper with feedback from all co-authors.
Funding
The National Science Foundation Graduate Research Fellowship Program supported EL. This study received support from the National Council for Scientific and Technological Development—CNPq (Process: 447402/2014-5) and Bolsa PQ to AS (Process: 314986/2020-0) and the Foundation for support to the Development of Education, Science and Technology of the state of Mato Grosso do Sul FUNDECT (TO 063/2017). A generous grant from the University of Kentucky Student Sustainability Council facilitated the publication of this study in an open-access journal.
Conflict of Interest
The author IB was employed by Embrapa Pantanal and Embrapa Agricultura Digital.
The remaining authors declare that the research was conducted in the absence of any commercial or financial relationships that could be construed as a potential conflict of interest.
Publisher’s Note
All claims expressed in this article are solely those of the authors and do not necessarily represent those of their affiliated organizations, or those of the publisher, the editors and the reviewers. Any product that may be evaluated in this article, or claim that may be made by its manufacturer, is not guaranteed or endorsed by the publisher.
Acknowledgments
We thank A. Cohen for sharing the global lake data plotted in Figure 3. The authors acknowledge the comments and suggestions from the reviewers that helped to improve the manuscript. We also thank the University of Kentucky’s Department of Earth and Environmental Sciences for the financial support of this project.
Supplementary Material
The Supplementary Material for this article can be found online at: https://www.frontiersin.org/articles/10.3389/feart.2022.875919/full#supplementary-material
References
Abel, C., Horion, S., Tagesson, T., De Keersmaecker, W., Seddon, A. W. R., Abdi, A. M., et al. (2021). The Human-Environment Nexus and Vegetation-Rainfall Sensitivity in Tropical Drylands. Nat. Sustain 4, 25–32. doi:10.1038/s41893-020-00597-z
Adams, E. A., Stoler, J., and Adams, Y. (2019). Water Insecurity and Urban Poverty in the Global South: Implications for Health and Human Biology. Am. J. Hum. Biol. 32, e23368. doi:10.1002/ajhb.23368
Alavez-Vargas, M., Birkel, C., Corona, A., and Breña-Naranjo, J. A. (2021). Land Cover Change Induced Sediment Transport Behaviour in a Large Tropical Mexican Catchment. Hydrological Sci. J. 66 (6), 1069–1082. doi:10.1080/02626667.2021.1903472
Aleman, J. C., Blarquez, O., Bentaleb, I., Bonté, P., Brossier, B., Carcaillet, C., et al. (2013). Tracking Land-Cover Changes with Sedimentary Charcoal in the Afrotropics. Holocene 23 (12), 1853–1862. doi:10.1177/0959683613508159
Ang, W. J., Park, E., and Alcantara, E. (2021). Mapping Floodplain Bathymetry in the Middle-Lower Amazon River Using Inundation Frequency and Field Control. Geomorphology 392, 107937. doi:10.1016/j.geomorph.2021.107937
Apitz, S. E. (2011). Sustainable Sediment Management? Integr. Environ. Assess. Manag. 7 (4), 691–693. doi:10.1002/ieam.264
Appleby, P. G., and Oldfield, F. (1992). “Application of Lead-210 to Sedimentation Studies,” in Uranium-series Disequilibrium: Applications to Earth, Marine, and Environmental Sciences. Editors M. Ivanovich, and R. S. Harmon (United Kingdom: Clarendon Press).
Appleby, P. G., and Oldfield, F. (1978). The Calculation of Lead-210 Dates Assuming a Constant Rate of Supply of Unsupported 210Pb to the Sediment. Catena 5 (1), 1–8. doi:10.1016/s0341-8162(78)80002-2
Assine, M. L., Macedo, H. A., Stevaux, J. C., Bergier, I., Padovani, C. R., and Silva, A. (2015). “Avulsive Rivers in the Hydrology of the Pantanal Wetland,” in Dynamics of the Pantanal Wetland in South America. New York: Springer. Editors I. Bergier, and M. Assine, 37, 83–110. The Handbook of Environmental Chemistry. doi:10.1007/698_2015_351
Avnimelech, Y., Ritvo, G., Meijer, L. E., and Kochba, M. (2001). Water Content, Organic Carbon and Dry Bulk Density in Flooded Sediments. Aquac. Eng. 25 (1), 25–33. doi:10.1016/s0144-8609(01)00068-1
Barichivich, J., Gloor, E., Peylin, P., Brienen, R. J. W., Schöngart, J., Espinoza, J. C., et al. (2018). Recent Intensification of Amazon Flooding Extremes Driven by Strengthened Walker Circulation. Sci. Adv. 4, eaat8785. doi:10.1126/sciadv.aat8785
Barlow, J., França, F., Gardner, T. A., Hicks, C. C., Lennox, G. D., Berenguer, E., et al. (2018). The Future of Hyperdiverse Tropical Ecosystems. Nature 559, 517–526. doi:10.1038/s41586-018-0301-1
Bartczak, A., Słowińska, S., Tyszkowski, S., Kramkowski, M., Kaczmarek, H., Kordowski, J., et al. (2019). Ecohydrological Changes and Resilience of a Shallow Lake Ecosystem under Intense Human Pressure and Recent Climate Change. Water 11, 32. doi:10.3390/w11010032
Baud, A., Jenny, J-P., Francus, P., and Gregory-Eaves, I. (2021). Global Acceleration of Lake Sediment Accumulation Rates Associated with Recent Human Population Growth and Land-Use Changes. J. Paleolimnol. 66, 453–467. doi:10.1007/s10933-021-00217-6
Beck, H. E., Zimmermann, N. E., McVicar, T. R., Vergopolan, N., Berg, A., and Wood, E. F. (2018). Present and Future Köppen-Geiger Climate Classification Maps at 1-km Resolution. Sci. Data 5, 180214. doi:10.1038/sdata.2018.214
Bennett, K., and Buck, C. E. (2016). Interpretation of Lake Sediment Accumulation Rates. Holocene 26 (7), 1092–1102. doi:10.1177/0959683616632880
Bergier, I., Assine, M. L., McGlue, M. M., Alho, C. J. R., Silva, A., Guerreiro, R. L., et al. (2018). Amazon Rainforest Modulation of Water Security in the Pantanal Wetland. Sci. Total Environ. 619-620, 1116–1125. doi:10.1016/j.scitotenv.2017.11.163
Binford, M. W., Kahl, J. S., and Norton, S. A. (1993). Interpretation of210Pb Profiles and Verification of the CRS Dating Model in PIRLA Project Lake Sediment Cores. J. Paleolimnol. 9, 275–296. doi:10.1007/bf00677218
Biswas, M., and Dhara, P. (2019). Evolutionary Characteristics of Meander Cut-Off- A Hydro-Morphological Study of the Jalangi River, West Bengal, India. Arab. J. Geosci. 12, 667. doi:10.1007/s12517-019-4711-7
Blaauw, M., and Christen, J. A. (2005). Radiocarbon Peat Chronologies and Environmental Change. J. R. Stat. Soc. C 54 (4), 805–816. doi:10.1111/j.1467-9876.2005.00516.x
Bremond, L., Favier, C., Ficetola, G. F., Tossou, M. G., Akouégninou, A., Gielly, L., et al. (2017). Five Thousand Years of Tropical Lake Sediment DNA Records from Benin. Quat. Sci. Rev. 170, 203–211. doi:10.1016/j.quascirev.2017.06.025
Busker, T., de Roo, A., Gelati, E., Schwatke, C., Adamovic, M., Bisselink, B., et al. (2019). A Global Lake and Reservoir Volume Analysis Using a Surface Water Dataset and Satellite Altimetry. Hydrol. Earth Syst. Sci. 23, 669–690. doi:10.5194/hess-23-669-2019
Carabajal, C. C., and Boy, J.-P. (2021). Lake and Reservoir Volume Variations in South America from Radar Altimetry, ICESat Laser Altimetry, and GRACE Time-Variable Gravity. Adv. Space Res. 68, 652–671. doi:10.1016/j.asr.2020.04.022
Carlson, K. M., Curran, L. M., Ponette-González, A. G., Ratnasari, D., Lisnawati, N., et al. Ruspita (2014). Influence of Watershed-Climate Interactions on Stream Temperature, Sediment Yield, and Metabolism along a Land Use Intensity Gradient in Indonesian Borneo. J. Geophys. Res. Biogeosci. 119, 1110–1128. doi:10.1002/2013jg002516
Center for International Earth Science Information Network – CIESIN – Columbia University (2018). Gridded Population of the World, Version 4 (GPWv4): Population Density, Revision 11. Palisades, NY: NASA Socioeconomic Data and Applications Center. doi:10.7927/H49C6VHW
Chambers, P. A., Lacoul, P., Murphy, K. J., and Thomaz, S. M. (2008). “Global Diversity of Aquatic Macrophytes in Freshwater,” in Freshwater Animal Diversity Assessment. New York: Springer. Editors E. V. Balian, C. Lévêque, H. Segers, and K. Martens.
Chant, S. H., and McIlwaine, C. (2009). Geographies of Development in the 21st Century. Cheltenham, UK: Edward Elgar Publishing.
Clemens, S. C., Prell, W. L., and Howard, W. R. (1987). Retrospective Dry Bulk Density Estimates from Southeast Indian Ocean Sediments - Comparison of Water Loss and Chloride-Ion Methods. Mar. Geol. 76, 57–69. doi:10.1016/0025-3227(87)90017-x
Cohen, A., McGlue, M. M., Ellis, G. S., Zani, H., Swarzenski, P. W., Assine, M. L., et al. (2015). “Lake Formation, Characteristics, and Evolution in Retroarc Deposystems: a Synthesis of the Modern Andean Orogen and its Associated Basins,” in The Geological Society of America Memoir 212: Geodynamics of a Cordilleran Orogenic System—The Central Andes of Argentina and Northern Chile. Boulder, CO: Geological Society of America. Editors P. G. DeCelles, M. N. Ducea, B. Carrapa, and P. A. Kapp.
Cohen, A. S. (2003). Paleolimnology: The History and Evolution of Lake Systems. United Kingdom: Oxford University Press.
Colloff, M. J., and Baldwin, D. S. (2010). Resilience of Floodplain Ecosystems in a Semi-arid Environment. Rangel. J. 32, 305–314. doi:10.1071/rj10015
Conrad, O., Bechtel, B., Bock, M., Dietrich, H., Fischer, E., Gerlitz, L., et al. (2015). System for Automated Geoscientific Analyses (SAGA) V. 2.1.4. Geosci. Model Dev. 8, 1991–2007. doi:10.5194/gmd-8-1991-2015
Costa, M. P. F., and Telmer, K. H. (2006). Utilizing SAR Imagery and Aquatic Vegetation to Map Fresh and Brackish Lakes in the Brazilian Pantanal Wetland. Remote Sens. Environ. 105 (3), 204–213. doi:10.1016/j.rse.2006.06.014
Coutinho, B. A., Pott, V. J., Arrua, B. A., Aoki, C., and Pott, A. (2018). Ecological Succession of Aquatic Macrophytes on Floating Meadows in the Pantanal Wetland. Braz. J. Bot. 41, 65–75. doi:10.1007/s40415-017-0425-9
Crisman, T. L., Mitraki, C., and Zalidis, G. (2005). Integrating Vertical and Horizontal Approaches for Management of Shallow Lakes and Wetlands. Ecol. Eng. 24 (4), 379–389. doi:10.1016/j.ecoleng.2005.01.006
Cross, B. K., and Moore, B. C. (2014). Lake and Reservoir Volume: Hydroacoustic Survey Resolution and Accuracy. Lake Reserv. Manag. 30 (4), 405–411. doi:10.1080/10402381.2014.960115
Dearing, J. A., and Jones, R. T. (2003). Coupling Temporal and Spatial Dimensions of Global Sediment Flux through Lake and Marine Sediment Records. Glob. Planet. Change 39, 147–168. doi:10.1016/s0921-8181(03)00022-5
Debret, M., Bentaleb, I., Sebag, D., Favier, C., Nguetsop, V., Fontugne, M., et al. (2014). Influence of Inherited Paleotopography and Water Level Rise on the Sedimentary Infill of Lake Ossa (S Cameroon) Inferred by Continuous Color and Bulk Organic Matter Analyses. Palaeogeogr. Palaeoclimatol. Palaeoecol. 411, 110–121. doi:10.1016/j.palaeo.2014.06.021
Erenstein, O. (2006). Intensification or Extensification? Factors Affecting Technology Use in Peri-Urban Lowlands along an Agro-Ecological Gradient in West Africa. Agric. Syst. 90 (1-3), 132–158. doi:10.1016/j.agsy.2005.12.005
Evenson, R. E., and Gollin, D. (2003). Assessing the Impact of the Green Revolution, 1960 to 2000. Science 300 (5620), 758–762. doi:10.1126/science.1078710
Fick, S. E., and Hijmans, R. J. (2017). WorldClim 2: New 1‐km Spatial Resolution Climate Surfaces for Global Land Areas. Int. J. Climatol. 37 (12), 4302–4315. doi:10.1002/joc.5086
Furian, S., Martins, E. R. C., Parizotto, T. M., Rezende-Filho, A. T., Victoria, R. L., and Barbiero, L. (2013). Chemical Diversity and Spatial Variability in Myriad Lakes in Nhecolândia in the Pantanal Wetlands of Brazil. Limnol. Oceanogr. 58 (6), 2249–2261. doi:10.4319/lo.2013.58.6.2249
Gibbs, H. K., Ruesch, A. S., Achard, F., Clayton, M. K., Holmgren, P., Ramankutty, N., et al. (2010). Tropical Forests Were the Primary Sources of New Agricultural Land in the 1980s and 1990s. Proc. Natl. Acad. Sci. U.S.A. 107 (38), 16732–16737. doi:10.1073/pnas.0910275107
Gomes, L., Simões, S., Dalla Nora, E., de Sousa-Neto, E., Forti, M., and Ometto, J. (2019). Agricultural Expansion in the Brazilian Cerrado: Increased Soil and Nutrient Losses and Decreased Agricultural Productivity. Land 8, 12. doi:10.3390/land8010012
Guerreiro, R. L., Bergier, I., McGlue, M. M., Warren, L. V., Abreu, U. G. P. d., Abrahão, J., et al. (2019). The Soda Lakes of Nhecolândia: A Conservation Opportunity for the Pantanal Wetlands. Perspect. Ecol. Conservation 17 (1), 9–18. doi:10.1016/j.pecon.2018.11.002
Hamilton, D. P., and Mitchell, S. F. (1996). An Empirical Model for Sediment Resuspension in Shallow Lakes. Hydrobiologia 317, 209–220. doi:10.1007/bf00036471
Hammer, Ø., Harper, D. A. T., and Ryan, P. D. (2001). PAST: Paleontological Statistics Software Package for Education and Data Analysis. Palaeontol. Electron. 4, 9.
Hansen, M. C., Potapov, P. V., Moore, R., Hancher, M., Turubanova, S. A., Tyukavina, A., et al. (2013). High-Resolution Global Maps of 21st-Century Forest Cover Change. Science 342, 850–853. doi:10.1126/science.1244693
Hartley, A. J., Weissmann, G. S., Nichols, G. J., and Warwick, G. L. (2010). Large Distributive Fluvial Systems: Characteristics, Distribution, and Controls on Development. J. Sediment. Res. 80 (2), 167–183. doi:10.2110/jsr.2010.016
Havens, K., Paerl, H., Phlips, E., Zhu, M., Beaver, J., and Srifa, A. (2016). Extreme Weather Events and Climate Variability Provide a Lens to How Shallow Lakes May Respond to Climate Change. Water 8, 229. doi:10.3390/w8060229
Hayashi, M., and van der Kamp, G. (2000). Simple Equations to Represent the Volume-Area-Depth Relations of Shallow Wetlands in Small Topographic Depressions. J. Hydrology 237 (1-2), 74–85. doi:10.1016/s0022-1694(00)00300-0
Hogg, A. G., Hua, Q., Blackwell, P. G., Niu, M., Buck, C. E., Guilderson, T. P., et al. (2013). SHCal13 Southern Hemisphere Calibration, 0-50,000 Years Cal BP. Radiocarbon 55 (4), 1889–1903. doi:10.2458/azu_js_rc.55.16783
Horbe, A. M. C., Behling, H., Nogueira, A. C. R., and Mapes, R. (2011). Environmental Changes in the Western Amazônia: Morphological Framework, Geochemistry, Palynology and Radiocarbon Dating Data. An. Acad. Bras. Ciênc. 83 (3), 863–874. doi:10.1590/s0001-37652011005000030
Ivory, S. J., McGlue, M. M., Peterman, C., Baldwin, P., Lucas, J., Cohen, A., et al. (2021). Climate, Vegetation, and Weathering across Space and Time in Lake Tanganyika (Tropical Eastern Africa). Quat. Sci. Adv. 3, 100023. doi:10.1016/j.qsa.2021.100023
Jankowski, K. J., Deegan, L. A., Neill, C., Sullivan, H. L., Ilha, P., Maracahipes-Santos, L., et al. (2021). Land Use Change Influences Ecosystem Function in Headwater Streams of the Lowland Amazon Basin. Water 13 (12), 1667. doi:10.3390/w13121667
Janse, J. H., van Dam, A. A., Hes, E. M. A., de Klein, J. J. M., Finlayson, C. M., Janssen, A. B. G., et al. (2019). Towards a Global Model for Wetlands Ecosystem Services. Curr. Opin. Environ. Sustain. 36, 11–19. doi:10.1016/j.cosust.2018.09.002
Khazaei, B., Read, L. K., Casali, M., Sampson, K. M., and Yates, D. N. (2022). GLOBathy, the Global Lakes Bathymetry Dataset. Sci. Data 9, 36. doi:10.1038/s41597-022-01132-9
Klein Goldewijk, K., Beusen, A., Van Drecht, G., and De Vos, M. (2011). The HYDE 3.1 Spatially Explicit Database of Human-Induced Global Land-Use Change over the Past 12,000 Years. Glob. Ecol. Biogeogr. 20, 73–86. doi:10.1111/j.1466-8238.2010.00587.x
Kossoni, A., and Giresse, P. (2010). Interaction of Holocene Infilling Processes between a Tropical Shallow Lake System (Lake Ossa) and a Nearby River System (Sanaga River) (South Cameroon). J. Afr. Earth Sci. 56, 1–14. doi:10.1016/j.jafrearsci.2009.05.012
Krishnaswamy, J., Halpin, P. N., and Richter, D. D. (2001). Dynamics of Sediment Discharge in Relation to Land-Use and Hydro-Climatology in a Humid Tropical Watershed in Costa Rica. J. Hydrology 253, 91–109. doi:10.1016/s0022-1694(01)00474-7
Laurance, W. F., Sayer, J., and Cassman, K. G. (2014). Agricultural Expansion and its Impacts on Tropical Nature. Trends Ecol. Evol. 29 (2), 107–116. doi:10.1016/j.tree.2013.12.001
Le Huong, H., and Thanh Son, N. (2020). Response of Streamflow and Soil Erosion to Climate Change and Human Activities in Nam Rom River Basin, Northwest of Vietnam. Environ. Nat. Resour. J. 18 (4), 411–423. doi:10.32526/ennrj.18.4.2020.39
Lemes do Prado, A., Heckman, C. W., and Martins, F. R. (1994). The Seasonal Succession of Biotic Communities in Wetlands of the Tropical Wet-And-Dry Climatic Zone: II. The Aquatic Macrophyte Vegetation in the Pantanal of Mato Grosso, Brazil. Int. Rev. Ges. Hydrobiol. Hydrogr. 79 (4), 569–589. doi:10.1002/iroh.19940790407
Leite, C. C., Costa, M. H., Soares-Filho, B. S., and Hissa, L. B. V. (2012). Historical Land Use Change and Associated Carbon Emissions in Brazil from 1940 to 1995. Glob. Biogeochem. Cycles 26 (2), GB2011. doi:10.1029/2011gb004133
Lewis, W. M. (1996). “Tropical Lakes: How Latitude Makes a Difference,” in Perspective Sin Tropical Limnology. Editors F. Schiemer, and K. T. Boland (Amsterdam, Netherlands: SPB Academic Publishing), 43–64.
Liu, K., and Song, C. (2022). Modeling Lake Bathymetry and Water Storage from DEM Data Constrained by Limited Underwater Surveys. J. Hydrology 604, 127260. doi:10.1016/j.jhydrol.2021.127260
Lo, E. L., Silva, A., Bergier, I., mcGlue, M. M., Silva, B. L. P., Silva, A. P. S., et al. (2017). Spatiotemporal Evolution of the Margins of Lake Uberaba, Pantanal Floodplain (Brazil). Geografia 42 (3), 159–173. doi:10.5016/geografia.v42i3.13096
Lo, E. L., McGlue, M. M., Silva, A., Bergier, I., Yeager, K. M., de Azevedo Macedo, H., et al. (2019). Fluvio-lacustrine Sedimentary Processes and Landforms on the Distal Paraguay Fluvial Megafan (Brazil). Geomorphology 342, 163–175. doi:10.1016/j.geomorph.2019.06.001
Long, K. E., Schneider, L., Connor, S. E., Shulmeister, N., Finn, J., Roberts, G. L., et al. (2021). Human Impacts and Anthropocene Environmental Change at Lake Kutubu, a Ramsar Wetland in Papua New Guinea. Proc. Natl. Acad. Sci. 118, e2022216118. doi:10.1073/pnas.2022216118
López-Blanco, C., Kenney, W. F., and Varas, A. (2017). Recent Flood Management Efforts Obscure the Climate Signal in a Sediment Record from a Tropical Lake. J. Paleolimnol. 58, 467–478. doi:10.1007/s10933-017-0004-x
Louzada, R. O., Bergier, I., and Assine, M. L. (2020). Landscape Changes in Avulsive River Systems: Case Study of Taquari River on Brazilian Pantanal Wetlands. Sci. Total Environ. 723, 138067. doi:10.1016/j.scitotenv.2020.138067
Louzada, R. O., Bergier, I., Roque, F. O., McGlue, M. M., Silva, A., and Assine, M. L. (2021). Avulsions Drive Ecosystem Services and Economic Changes in the Brazilian Pantanal Wetlands. Curr. Res. Environ. Sustain. 3, 100057. doi:10.1016/j.crsust.2021.100057
Makwinja, R., Mengistou, S., Kaunda, E., and Alamirew, T. (2021). Economic Value of Tropical Inland Freshwater Shallow Lakes: Lesson from Lake Malombe, Malawi. Afr. J. Ecol. doi:10.1111/aje.12935
Marengo, J. A., Cunha, A. P. M. A., Nobre, C. A., Ribeiro Neto, G. G., Magalhaes, A. R., Torres, R. R., et al. (2020). Assessing Drought in the Drylands of Northeast Brazil under Regional Warming Exceeding 4 °C. Nat. Hazards 103, 2589–2611. doi:10.1007/s11069-020-04097-3
McGlue, M. M., Guerreiro, R. L., Bergier, I., Silva, A., Pupim, F. N., Oberc, V., et al. (2017). Holocene Stratigraphic Evolution of Saline Lakes in Nhecolândia, Southern Pantanal Wetlands (Brazil). Quat. Res. 88 (3), 472–490. doi:10.1017/qua.2017.57
McGlue, M. M., Silva, A., Assine, M. L., Stevaux, J. C., and Pupim, F. d. N. (2015). “Paleolimnology in the Pantanal: Using Lake Sediments to Track Quaternary Environmental Change in the World's Largest Tropical Wetland,” in Dynamics of the Pantanal Wetland in South America. Editors I. Bergier, and M. L. Assine (Cham: Springer), 37, 51–81. The Handbook of Environmental Chemistry. doi:10.1007/698_2015_350
McGlue, M. M., Silva, A., Corradini, F. A., Zani, H., Trees, M. A., Ellis, G. S., et al. (2011). Limnogeology in Brazil's "forgotten Wilderness": a Synthesis from the Large Floodplain Lakes of the Pantanal. J. Paleolimnol. 46, 273–289. doi:10.1007/s10933-011-9538-5
Messager, M. L., Lehner, B., Grill, G., Nedeva, I., and Schmitt, O. (2016). Estimating the Volume and Age of Water Stored in Global Lakes Using a Geo-Statistical Approach. Nat. Commun. 7, 13603. doi:10.1038/ncomms13603
Mosquera, P. V., Hampel, H., Vázquez, R. F., Alonso, M., and Catalan, J. (2017). Abundance and Morphometry Changes across the High-Mountain Lake-Size Gradient in the Tropical Andes of Southern Ecuador. Water Resour. Res. 53, 7269–7280. doi:10.1002/2017wr020902
Ndehedehe, C. E., Onojeghuo, A. O., Stewart-Koster, B., Bunn, S. E., and Ferreira, V. G. (2021). Upstream Flows Drive the Productivity of Floodplain Ecosystems in Tropical Queensland. Ecol. Indic. 125, 107546. doi:10.1016/j.ecolind.2021.107546
Nobre, R. L. G., Caliman, A., Cabral, C. R., Araújo, F. d. C., Guérin, J., Dantas, F. d. C. C., et al. (2020). Precipitation, Landscape Properties and Land Use Interactively Affect Water Quality of Tropical Freshwaters. Sci. Total Environ. 716, 137044. doi:10.1016/j.scitotenv.2020.137044
Nõges, T. (2009). Relationships between Morphometry, Geographic Location and Water Quality Parameters of European Lakes. Hydrobiologia 633, 33–43. doi:10.1007/s10750-009-9874-x
O’Callaghan, J. F., and Mark, D. M. (1984). The Extraction of Drainage Networks from Digital Elevation Data. Comput. Vis. Graph. Image Process. 28, 323–344.
Oliveira, J. E., and Milheira, R. G. (2020). Ethnoarchaeology of Two Guató Mounds in the Pantanal: Building Dynamics and History in Persistent Places. Mana 26 (3), 1–39. doi:10.1590/1678-49442020v26n3a208
Osborne, P. L., Humphreys, G. S., and Polunin, N. V. C. (1993). Sediment Deposition and Late Holocene Environmental Change in a Tropical Lowland Basin: Waigani Lake, Papua New Guinea. J. Biogeogr. 20, 599–613. doi:10.2307/2845517
Owens, P. N. (2020). Soil Erosion and Sediment Dynamics in the Anthropocene: a Review of Human Impacts during a Period of Rapid Global Environmental Change. Adv. Sediment Sci. Manag. 20 (4), 4115–4143. doi:10.1007/s11368-020-02815-9
Owens, P. N., Batalla, R. J., Collins, A. J., Gomez, B., Hicks, D. M., Horowitz, A. J., et al. (2005). Fine-grained Sediment in River Systems: Environmental Significance and Management Issues. River Res. applic. 21, 693–717. doi:10.1002/rra.878
Padisák, J., and Reynolds, C. S. (2003). Shallow Lakes: the Absolute, the Relative, the Functional and the Pragmatic. Hydrobiologia 506, 1–11. doi:10.1023/B:HYDR.0000008630.49527.29
Papangelakis, E., MacVicar, B., and Ashmore, P. (2019). Bedload Sediment Transport Regimes of Semi‐alluvial Rivers Conditioned by Urbanization and Stormwater Management. Water Resour. Res. 55, 10565–10587. doi:10.1029/2019wr025126
Parinet, B., Lhote, A., and Legube, B. (2004). Principal Component Analysis: an Appropriate Tool for Water Quality Evaluation and Management-Application to a Tropical Lake System. Ecol. Model. 178, 295–311. doi:10.1016/j.ecolmodel.2004.03.007
Park, E., Emadzadeh, A., Alcântara, E., Yang, X., and Ho, H. L. (2020). Inferring Floodplain Bathymetry Using Inundation Frequency. J. Environ. Manag. 273, 111138. doi:10.1016/j.jenvman.2020.111138
Peixoto, J. L. S. (2009). Arqueologia na região das Grandes Lagoas Do Pantanal. Albuquerque Rev. história 1 (2), 193–206. doi:10.46401/ajh.2009.v1.3930
Pivari, M. O., Pott, V. J., and Pott, A. (2008). Macrófitas aquáticas de ilhas flutuantes (baceiros) nas sub-regiões Do Abobral e Miranda, Pantanal, MS, Brasil. Acta Bot. Bras. 22 (2), 563–571. doi:10.1590/s0102-33062008000200023
Pott, A., and da Silva, J. S. V. (2015). “Terrestrial and Aquatic Vegetation Diversity of the Pantanal Wetland,” in Dynamics of the Pantanal Wetland in South America. Editors I. Bergier, and M. L. Assine (Switzerland: Springer), 37, 111–131. The Handbook of Environmental Chemistry. doi:10.1007/698_2015_352
Räsänen, M. E., Salo, J. S., and Jungner, H. (1991). Holocene Floodplain Lake Sediments in the Amazon: 14C Dating and Palaeoecological Use. Quat. Sci. Rev. 10, 363–372. doi:10.1016/0277-3791(91)90037-u
Rasbold, G. G., McGlue, M. M., Stevaux, J. C., Parolin, M., Silva, A., and Bergier, I. (2021). Enhanced Middle Holocene Organic Carbon Burial in Tropical Floodplain Lakes of the Pantanal (South America). J. Paleolimnol. 65, 181–199. doi:10.1007/s10933-020-00159-5
Reimer, P. J., Austin, W. E. N., Bard, E., Bayliss, A., Blackwell, P. G., Bronk Ramsey, C., et al. (2020). The IntCal20 Northern Hemisphere Radiocarbon Age Calibration Curve (0-55 Cal kBP). Radiocarbon 62 (4), 725–757. doi:10.1017/rdc.2020.41
Reimer, R., and Reimer, P. (2004). CALIBomb – Calibration of Post-bomb C-14 Data. Software: Unknown Publisher.
Restrepo, J. D., Kettner, A. J., and Syvitski, J. P. M. (2015). Recent Deforestation Causes Rapid Increase in River Sediment Load in the Colombian Andes. Anthropocene 10, 13–28. doi:10.1016/j.ancene.2015.09.001
Reynolds, C. A., Jackson, T. J., and Rawls, W. J. (2000). Estimating Soil Water-Holding Capacities by Linking the Food and Agriculture Organization Soil Map of the World with Global Pedon Databases and Continuous Pedotransfer Functions. Water Resour. Res. 36, 3653–3662. doi:10.1029/2000wr900130
Ritchie, J. C., and McHenry, J. R. (1990). Application of Radioactive Fallout Cesium-137 for Measuring Soil Erosion and Sediment Accumulation Rates and Patterns: a Review. J. Environ. Qual. 19 (2), 215–233. doi:10.2134/jeq1990.00472425001900020006x
Rocha, C. H. N., Costa, M. R. A., Menezes, R. F., Attayde, J. L., and Becker, V. (2018). Water Volume Reduction Increases Eutrophication Risk in Tropical Semi-arid Reservoirs. Acta Limnol. Bras. 30, e106. doi:10.1590/s2179-975x2117
Rodriguez, M., Steiger, J., Rosales, J., Laraque, A., López, J. L., Castellanos, B., et al. (2019). Multi-annual Contemporary Flood Event Overbank Sedimentation within the Vegetated Lower Orinoco Floodplain, Venezuela. River Res. Appl. 35 (8), 1241–1256. doi:10.1002/rra.3510
Roulet, M., Lucotte, M., Canuel, R., Farella, N., Courcelles, M., Guimarães, J.-R. D., et al. (2000). Increase in Mercury Contamination Recorded in Lacustrine Sediments Following Deforestation in the Central Amazon1The Present Investigation Is Part of an Ongoing Study, the CARUSO Project (CRDI-UFPa-UQAM), Initiated to Determine the Sources, Fate and Health Effects of the Presence of MeHg in the Area of the Lower Tapajós.1. Chem. Geol. 165, 243–266. doi:10.1016/s0009-2541(99)00172-2
Ryken, N., Vanmaercke, M., Wanyama, J., Isabirye, M., Vanonckelen, S., Deckers, J., et al. (2015). Impact of Papyrus Wetland Encroachment on Spatial and Temporal Variabilities of Stream Flow and Sediment Export from Wet Tropical Catchments. Sci. Total Environ. 511, 756–766. doi:10.1016/j.scitotenv.2014.12.048
Sadler, P. M. (1981). Sediment Accumulation Rates and the Completeness of Stratigraphic Sections. J. Geol. 89, 569–584. doi:10.1086/628623
Salerno, J., Diem, J. E., Konecky, B. L., and Hartter, J. (2019). Recent Intensification of the Seasonal Rainfall Cycle in Equatorial Africa Revealed by Farmer Perceptions, Satellite-Based Estimates, and Ground-Based Station Measurements. Clim. Change 153, 123–139. doi:10.1007/s10584-019-02370-4
Schiefer, E., Petticrew, E. L., Immell, R., Hassan, M. A., and Sonderegger, D. L. (2013). Land Use and Climate Change Impacts on Lake Sedimentation Rates in Western Canada. Anthropocene 3, 61–71. doi:10.1016/j.ancene.2014.02.006
Schulz, C., Whitney, B. S., Rossetto, O. C., Neves, D. M., Crabb, L., de Oliveira, E. C., et al. (2019). Physical, Ecological and Human Dimensions of Environmental Change in Brazil's Pantanal Wetland: Synthesis and Research Agenda. Sci. Total Environ. 687, 1011–1027. doi:10.1016/j.scitotenv.2019.06.023
Selcer, P. (2015). Fabricating United: the FAO-UNESCO Soil Map of the World. Hist. Res. Soc. 40, 174–201. doi:10.12759/hsr.40.2015.2.174-201
Settele, J., Scholes, R., Betts, R., Bunn, S., Leadley, P., Nepstad, D., et al. (2014). “Terrestrial and Inland Water Systems,” in Climate Change 2014: Impacts, Adaptation, and Vulnerability. Part A: Global and Sectoral Aspects. Contribution of Working Group II to the Fifth Assessment Report of the Intergovernmental Panel on Climate Change. Editors C. B. Field, V. R. Barros, D. J. Dokken, K. J. Mach, M. D. Mastrandrea, T. E. Biliret al. (Cambridge, UK: Cambridge University Press), 271–359.
Shepard, G. H., Clement, C. R., Lima, H. P., dos Santos, G. M., Moraes, C. P., and Neves, E. G. (2020). “Ancient and Traditional Agriculture in South America: Tropical Lowlands,” in Oxford Research Encyclopedias, Environmental Science (Oxford: Oxford University Press). doi:10.1093/acrefore/9780199389414.013.597
Shi, C., Zhang, D. D., and You, L. (2003). Sediment Budget of the Yellow River Delta, China: the Importance of Dry Bulk Density and Implications to Understanding of Sediment Dispersal. Mar. Geol. 199 (1-2), 13–25. doi:10.1016/s0025-3227(03)00159-2
Sílio-Calzada, A., Barquín, J., Huszar, V. L. M., Mazzeo, N., Méndez, F., and Álvarez-Martínez, M. (2017). Long-term Dynamics of a Floodplain Shallow Lake in the Pantanal Wetland: Is it All about Climate? Sci. Total Environ. 605-606, 527–540. doi:10.1016/j.scitotenv.2017.06.183
Sinha, R. K., Eldho, T. I., and Subimal, G. (2020). Assessing the Impacts of Historical and Future Land Use and Climate Change on the Streamflow and Sediment Yield of a Tropical Mountainous River Basin in South India. Environ. Monit. Assess. 192, 679. doi:10.1007/s10661-020-08623-5
State of the Tropics (2020). State of the Tropics 2020 Report. Townsville, Australia: James Cook University.
Street, R. B., and Semenov, S. M. (1990). “Chapter 3: Natural Terrestrial Ecosystems,” in Climate Change: The IPCC Impacts Assessment. Editors W. J. M. Tegart, G. W. Sheldon, and D. C. Griffiths (Canberra: Australian Government Publishing Service), 3–44.
Stuiver, M., Reimer, P. J., and Reimer, R. W. (2021). CALIB 8.2 [WWW Program]. Available at: http://calib.org (Accessed 8 19, 2021).
Syvitski, J. P. M., and Kettner, A. (2011). Sediment Flux and the Anthropocene. Phil. Trans. R. Soc. A 369, 957–975. doi:10.1098/rsta.2010.0329
Talling, J. F. (2001). Environmental Controls on the Functioning of Shallow Tropical Lakes. Hydrobiologia 458, 1–8. doi:10.1023/a:1013121522321
Tan, Z., Melack, J., Li, Y., Liu, X., Chen, B., and Zhang, Q. (2020). Estimation of Water Volume in Ungauged, Dynamic Floodplain Lakes. Environ. Res. Lett. 15, 054021. doi:10.1088/1748-9326/ab82cb
Verstraeten, G., and Poesen, J. (2001). Variability of Dry Sediment Bulk Density between and within Retention Ponds and its Impact on the Calculation of Sediment Yields. Earth Surf. Process. Landforms 26, 375–394. doi:10.1002/esp.186
Walling, D. E., and He, Q. (1998). The Spatial Variability of Overbank Sedimentation on River Floodplains. Geomorphology 24 (2-3), 209–223. doi:10.1016/s0169-555x(98)00017-8
Walling, D. E. (1999). “Linking Land Use, Erosion and Sediment Yields in River Basins,” in Man and River Systems. Boston: Kluwer Academic Publishers. Editors J. Garnier, and J. Mouchel.
Wang, L., and Liu, H. (2006). An Efficient Method for Identifying and Filling Surface Depressions in Digital Elevation Models for Hydrologic Analysis and Modelling. Int. J. Geogr. Inf. Sci. 20 (2), 193–213. doi:10.1080/13658810500433453
Webster, K. E., McCullough, I. M., and Soranno, P. A. (2022). Deeper by the Dozen: Diving into a Database of 17,675 Depths for U.S. Lakes and Reservoirs. Limnol. Oceanogr. Bull. 31, 1–5. doi:10.1002/lob.10482
Wolman, M. G., and Schick, A. P. (1967). Effects of Construction on Fluvial Sediment, Urban and Suburban Areas of Maryland. Water Resour. Res. 3, 451–464. doi:10.1029/wr003i002p00451
Xu, X., Jiang, B., Tan, Y., Costanza, R., and Yang, G. (2018). Lake-wetland Ecosystem Services Modeling and Valuation: Progress, Gaps and Future Directions. Ecosyst. Serv. 33, 19–28. doi:10.1016/j.ecoser.2018.08.001
Keywords: limnogeology, sedimentation rates, 210Pb, tropical lowland, deforestation, radiocarbon
Citation: Lo EL, Yeager KM, Bergier I, Domingos-Luz L, Silva A and McGlue MM (2022) Sediment Infill of Tropical Floodplain Lakes: Rates, Controls, and Implications for Ecosystem Services. Front. Earth Sci. 10:875919. doi: 10.3389/feart.2022.875919
Received: 14 February 2022; Accepted: 27 April 2022;
Published: 19 May 2022.
Edited by:
Fabiano N. Pupim, Federal University of São Paulo, BrazilReviewed by:
Xavier Benito, National Socio-Environmental Synthesis Center (SESYNC), United StatesMaria Isabel Velez, University of Regina, Canada
Copyright © 2022 Lo, Yeager, Bergier, Domingos-Luz, Silva and McGlue. This is an open-access article distributed under the terms of the Creative Commons Attribution License (CC BY). The use, distribution or reproduction in other forums is permitted, provided the original author(s) and the copyright owner(s) are credited and that the original publication in this journal is cited, in accordance with accepted academic practice. No use, distribution or reproduction is permitted which does not comply with these terms.
*Correspondence: Edward L. Lo, ZWR3YXJkLmxvQHVreS5lZHU=