- 1Instituto Argentino de Nivología, Glaciología y Ciencias Ambientales (IANIGLA), CCT-Mendoza, CONICET, Parque General San Martín, Mendoza, Argentina
- 2Natural History Museum of Utah, University of Utah, Salt Lake City, UT, United States
- 3Department of Geology & Geophysics, University of Utah, Salt Lake City, UT, United States
- 4Instituto de Estudios Andinos “Don Pablo Groeber” (IDEAN, UBA-CONICET), Departamento de Ciencias Geológicas, Facultad de Ciencias Exactas y Naturales, Universidad de Buenos Aires, Ciudad Universitaria C1428EHA, Buenos Aires, Argentina
- 5Evolutionary Studies Institute, University of the Witwatersrand, Johannesburg, South Africa
- 6Geology, Facultad de Ciencias Exactas y Naturales (FCEN), Universidad Nacional de Cuyo (UNCuyo), Mendoza, Argentina
Paleoenvironmental factors such as climate change are often hypothesized as critical for ecosystem changes over evolutionary time scales. Theoretically, these changes should be observable in the fossil record, but the robust linkage of biotic shifts to climate events remains difficult. The Late Triassic Period is an ideal interval for testing such hypotheses within terrestrial ecosystems because of a number of large-scale climate events, such as the Carnian Pluvial Episode (CPE), and a rich, well-studied fossil record. Nonetheless, at regional to global scales, few linkages have been identified during most of the Late Triassic. Here, we synthesize a multi-proxy basin-scale dataset of paleoenvironmental data, including new information from clay mineralogy and paleosol major- and trace-element geochemistry, to understand paleoclimate changes in the Ischigualasto-Villa Unión Basin of northwestern Argentina. In combination with diversity and relative abundance information from the well-sampled plant and tetrapod fossil record in the basin, we then use accepted evolutionary models of abiotic forcing of changes in ecosystems to test previously proposed hypotheses of floral and faunal variations at a basin scale. We find that although many patterns are best explained by sampling issues and taphonomy, pseudosuchian archosaur diversity and rhynchosaur relative abundance conform to predictions of paleoenvironmental forcing as the climate changed from warmer, drier conditions to more temperate humid conditions. These data demonstrate how high-resolution multi-proxy data and a well-sampled fossil record at a basin-scale can be used to test hypotheses of abiotic forcing of ecosystems in deep time, and highlights where future efforts should be directed in terms of filling data gaps and testing new hypotheses.
1 Introduction
Biotic systems are constantly interacting and changing, so they are in dynamic equilibrium (e.g., Vrba, 1995; Faith et al., 2021). Both intrinsic (biotic) and extrinsic (abiotic) factors are proposed as drivers of evolutionary events such as speciation, extinction, and migration (e.g., Vrba, 1995; Benton et al., 2021; Dunne et al., 2021). One of the challenges in the deep-time paleobiologic record is to understand the relative contributions of biotic and abiotic forcing on the assembly and evolution of ecosystems. Given that there is abundant observational evidence for climate change affecting extant ecosystems (e.g., Moritz et al., 2008; Tingley et al., 2009; Rowe et al., 2015), and strong inferential evidence for climate-caused mass extinction events in the geologic past (e.g., see summary in Whiteside and Grice, 2016), a key question is to what extent do abiotic factors such as climate change drive evolution through Earth’s history?
The Triassic Period is an ideal study system to help answer this question in deep time, because its record of climate and ecosystem evolution is a particularly dynamic ∼50 million year interval. The Triassic was a critical time for the origin and early diversification of Mesozoic ecosystems. On land, during the Triassic, several modern vertebrate clades originated and diversified, such as neopterygian fishes, lissamphibians, lepidosaurs, turtles, dinosaurs, and mammaliaforms (e.g., Fraser and Sues, 2011; Jones et al., 2013; Friedman, 2015; Schoch and Sues, 2015; Giles et al., 2017; Stocker et al., 2019). In Gondwana, which has a particularly rich non-marine fossil record of this time, the tetrapod assemblage is dominated by synapsid and archosauromorph lineages (e.g., Romer, 1970; Ezcurra, 2010; Abdala et al., 2020; Bordy et al., 2020; Schultz et al., 2020). The Late Triassic is also the time of dinosaur origin and diversification, the origin of turtles, the diversification of non-mammaliaform cynodonts that are closely related to the first mammaliaforms, and the decline of basal archosauromophs and early synapsids (see Supplementary Material) (Irmis, 2011; Irmis et al., 2011; Whiteside et al., 2011, 2015; Abdala et al., 2020; Dunne et al., 2021). Among terrestrial plants, this period is characterized by the dominance of seed- over spore-based lineages, with gymnosperms typically having greater relative abundance in fossil assemblages than pteridophytes (McElwain, 2018). In Gondwana, the most significant floral change is the replacement of the late Paleozoic Glossopteris flora by the Triassic Dicroidium flora (Balme and Helby, 1973; Zamunner et al., 2001; Abu Hamad et al., 2008; Chandra et al., 2008; Mays and Mcloughlin, 2019). Thus, recovery after the end-Permian extinction led to the diversification of the Dicroidium flora during the Early Triassic (Bodnar et al., 2021), and the Late Triassic is the climax of this floral assemblage in Gondwana (McLoughlin, 2001, 2011; Kustatscher et al., 2018).
From a climate perspective, the Triassic experienced enhanced monsoonal circulation because of the large exposed land area of Pangea across the equator (Kutzbach and Gallimore, 1989; Parrish, 1993; Wilson et al., 1994; Péron et al., 2005; Sellwood and Valdes, 2006; Holz, 2015; Harris et al., 2017). However, there is a common perception of a globally hot, dry, and seasonal climate globally (e.g., Retallack, 2009; Holz, 2015), despite evidence of much more varied climate regimes. In Gondwana, fluctuating climate conditions dominated with arid to semiarid conditions during the Early Triassic (Sellwood and Valdes, 2006; Boucot et al., 2013; Harris et al., 2017; Dunne et al., 2021), and more humid seasonality conditions during the Middle Triassic (Sellwood and Valdes, 2006; Mancuso et al., 2021). The Late Triassic was dominated by seasonal sub-humid conditions with one or more semi-arid intervals, particularly in the interior of the landmass (Sellwood and Valdes, 2006; Dunne et al., 2021; Mancuso et al., 2021). In particular, the “Carnian Pluvial Episode” (CPE) (Simms and Ruffell, 1989, 1990), which recorded an extensive rise in humidity and temperature in many depositional environments, has been documented in Gondwana and in the Tethys and Panthalassa oceans (e.g., Dal Corso et al., 2012, 2015, 2018; Ogg, 2015; Ruffell et al., 2016; Sun et al., 2016; Miller et al., 2017; Mancuso et al., 2020a; Tomimatsu et al., 2021). The CPE is particularly interesting due to the fact that it has been linked with major changes in terrestrial ecosystems (e.g., Goddéris et al., 2008; Benton et al., 2018; Bernardi et al., 2018; Dal Corso et al., 2020).
1.1 Climate and Evolution in the Triassic of Gondwana
A long-standing question has been how spatial and temporal differences in paleoclimate have influenced the distribution and diversity of Triassic biota, and data from the Ischigualasto-Villa Unión Basin have been key parts of these discussions for over 50 years (e.g., Romer, 1970; Colbert, 1971; Robinson, 1971, 1973; Dolby and Balme, 1976; Shubin and Sues, 1991; Foster et al., 1994; Zavattieri and Batten, 1996; Cirilli, 2010; Ezcurra, 2010; Césari and Colombi, 2013; Whiteside et al., 2015; Kustatscher et al., 2018; Dunne et al., 2021; Liu et al., 2021; Mancuso et al., 2021). The literature contains a variety of hypotheses that relate non-marine Triassic flora and fauna to various paleoclimate conditions; these typically focus on the richness and/or relative abundance of certain taxa to general climate variables such as warmer/colder or wetter/drier. Many of these hypotheses were developed from global datasets and/or other regions, but are important to evaluate because they apply to plant and animal groups preserved within the Ischigualasto-Villa Unión Basin.
More specifically, some groups of plants have been associated with specific climatic conditions, for example, Equisetopsida and Polypodiopsida have been linked to wet episodes of the seasonal subtropical climate during the Triassic (e.g., Channing et al., 2011; Husby, 2013; Bodnar et al., 2018; Coturel et al., 2018). The clade Gymnospermopsida is the most diverse group of plants during the Triassic. The different taxa referred to in this group have been conserved in different environments and exhibit a great variety of forms, which is why they would have developed successfully in a variety of subtropical zone environments with hot and seasonally humid climates (Fritts, 1976; Retallack, 1977; Anderson and Anderson, 1983, 1985, 1989; Holmes, 1985; He et al., 1997; Artabe et al., 2001, 2003; Kerp et al., 2001; Spalletti et al., 2003; Bomfleur et al., 2014). With respect to the recognized microflorisic sub-provinces in Gondwana, the Onslow microflora is suggested to indicate hot and humid climatic conditions, while the Ipswich microflora is an hypothesized to be indicative of relatively colder temperature zones (Dolby and Blame 1976). Changes in plant richness are also associated with changes in environmental and climatic parameters. These events are interpreted as successive ecological disturbances, rather than as abrupt changes in biodiversity (Sun et al., 2012; Hochuli et al., 2016; Mishra et al., 2017; Fielding et al., 2019; Nowak et al., 2019, 2020; Vajda et al., 2020).
To summarize, the literature contains the following hypotheses that propose a relationship between Triassic plant and non-marine tetrapod assemblage composition and climate:
Hypothesis 1. Plant species richness correlated with humid/arid conditions.
1a. High species richness was associated with higher humidity intervals and lower species richness with seasonally arid periods (Berry and Raison, 1981; Sun et al., 2012; Nowak et al., 2020).
1b. Taxa such as Equisetopsida, Polypodiopsida, and Voltziales preferred humid conditions (Bomfleur et al., 2013; Bodnar et al., 2018; Coturel et al., 2018).
1c. Umkomasiales increased relative abundance in different climatic conditions (Retallack, 1977; Bomfleur and Kerp, 2010; Bomfleur et al., 2014; D’Angelo, 2019).
1d. Others Pteridospermales such as Peltaspermales (Doubinger et al., 1995; Lausberg and Kerp, 2000; Kerp et al., 2001; Di Michele, 2013), Ginkgoales (He et al., 1997; Barboni and Dutra, 2015), Coniferales (Fritts, 1976; Holmes, 1985), and Gnetales (Wang and Zheng, 2010) increased relative abundance during intervals with marked seasonality.
1e. Cycadales and Benettitales were more abundant in hot and dry climatic conditions (Taylor et al., 2009).
Hypothesis 2. Synapsids, particularly traversodontid cynodonts, were more diverse (higher richness) in wetter areas (Whiteside et al., 2011).
2a. Dicynodonts preferred wetter intervals (Liu et al., 2021).
Hypothesis 3. Cynodont richness increased during warm conditions (Liu et al., 2021).
Hypothesis 4. Pseudosuchian richness was highest in warmer, less seasonal conditions (Dunne et al., 2021).
Hypothesis 5. Rhynchosaur abundance increased during more arid intervals (Azevedo et al., 1990).
1.2 Testing for a Climate-Biota Relationship
Despite an abundance of hypotheses linking changes in the Triassic fossil record to climate, there are significant challenges to observing and interpreting a robust correlation between richness or abundance and climate variables. Taphonomic filters and differences in sampling are well-recognized as having the potential to bias observed diversity (Behrensmeyer and Chapman, 1993; Kidwell and Flessa, 1996; Kidwell and Holland, 2002; Falcon-Lang et al., 2009), and equally problematic is the potential mismatch between the spatiotemporal scale/resolution of fossil data, paleoclimatic data, and hypotheses linking the two (e.g., Faith et al., 2021: Fig 3). Although there is a long history of pattern matching approaches, whereby paleontologists try to identify simultaneous changes in the fossil record and paleoenvironmental proxy data, these studies generally have low power and have met with limited success, because they often rely on a posteriori hypotheses from these correlations rather than attempting to test explicitly a priori hypotheses based on our understanding of Earth-life systems (Faith et al., 2021). In contrast, there is an abundance of evolutionary models for biotic response to climate change on geologic timescales (e.g., table 1 in Barnosky, 2001, and references therein). The application of these models to the fossil record provides a stronger way to test for climate-caused biotic change because they make specific predictions about changes in richness and relative abundance, and their timing relative to climate change, that can be tested in the geologic record (Faith et al., 2021). These predictions can also be further refined when ecological preferences and physiological constraints for specific lineages are known from independent evidence (e.g., observations from extant relatives).
Before examining causal models linking climatic and biotic change, it is important to establish the null model. The most basic null hypothesis is that species/lineages/clades do not respond to climate change, but this seems biologically unrealistic given an abundance of modern observational evidence to the contrary (e.g., Huntley, 1991; Thuiller et al., 2005; Moritz et al., 2008; Tingley et al., 2009; Minin and Voskova, 2014; Rowe et al., 2015). A null hypothesis that better matches our understanding of ecological systems is essentially an extension of Hubbell’s neutral theory (e.g., Hubbell, 2001; Rosindell et al., 2011, 2012), predicting that each species responds individually to climate change, and therefore net origination and extinction at any particular time interval is at equilibrium. In both cases, there should be no elevated turnover correlated with climate change events, and the pattern would be indistinguishable from normal background origination and extinction.
Models of evolution driven by changes in the physical environment were termed ‘Court Jester’ hypotheses by Barnosky (2001), to distinguish them from Red Queen hypotheses of evolution driven by biotic interactions. He concluded that Court Jester processes occurred at larger spatiotemporal scales (Barnosky, 2001: Fig 7), making them possibly easier to detect in the fossil record. The most relevant models for this study are the ‘relay’ and ‘turnover pulse’ hypotheses proposed by Vrba (1985, 1992, 1993, 1995). She proposed that because species are adapted to both the physical and biologic aspects of their habitat, the primary driver of species turnover is climate change (Vrba, 1985, 1992, 1993). Therefore, during times of stable climate, this should result in relatively little speciation and extinction, but during climate change intervals, there should be elevated “pulses” of origination and extinction observed (Vrba, 1985, 1993, 1995). This is similar to the mathematically-derived ‘stationary model’ of Stenseth and Maynard Smith (1984), but whereas Vrba’s hypothesis focused on turnover within the clade(s), Stenseth and Maynard Smith explicitly modeled communities, and did not distinguish between actual phyletic speciation/extinction, local extirpation, and immigration/emigration (Vrba, 1993).
Vrba (1995) refined the turnover pulse hypothesis with the relay model, which made detailed predictions about the order in which lineages should speciate and go extinct in relation to a climate event. Given a theoretical climate variable that changed from X to Y, Vrba (1995) predicted the following sequence of events: 1) extinction of species adapted to X; 2) minor speciation of remaining species adapted to X; 3) minor extinctions of species adapted to Y; and 4) speciation of species adapted to Y. So, to use Vrba’s (1995: Fig. 3.3) example of a warming trend, there should be an initial pulse of extinction of lineages adapted to cooler conditions (though some speciation of these lineages may still occur), followed by a pulse of speciation of lineages adapted to warmer conditions (though a few warm-adapted species will still go extinct in this interval). In the same contribution, Vrba (1995) proposed the traffic light model, which examined climate forcing of immigration/emigration. This model is less relevant to the current study because we are examining biotic change within a single basin, and do not have equivalent extrabasinal records with which to compare to.
It is important to emphasize that Vrba’s (1985, 1992, 1993, 1995) predictions for the turnover pulse and relay models were restricted to speciation (origination) and extinction of species (i.e., changes in species richness); she did not discuss changes in abundance. Despite this, some subsequent studies have applied these models to changes in species’ relative abundance through time, without explicitly justifying why this should be the case (e.g., Barnosky, 2001; Bobe et al., 2002). Nonetheless, some logical predictions for abundance can be made as long as the climate event is not so sudden that it appears “instantaneous” in the geologic record. As a climate variable changes over geologic timescales, one might predict an observation of a species declining in abundance prior to its ultimate extinction, or conversely increasing in abundance after its initial origination. In contrast, some studies have observed remarkable stability in species abundance over geologic timescales (dubbed “community inertia”) despite experiencing major climatic changes (e.g., McGill et al., 2005). If this is the case more generally, species abundances may be stable until they reach a climate threshold or ‘tipping point’, after which they suddenly go extinct. In this model, the climate threshold would also instigate speciation and an increase in abundance rapid enough that it is not observed in the fossil record.
Although these evolutionary models provide explicit and testable a priori hypotheses to infer climate-caused biotic change, patterns in the fossil record cannot simply be taken at face value. First, it is important to ask whether the spatial and temporal resolution of available data match that of the hypothesis being tested (Vrba, 1995; Faith et al., 2021: Fig. 3). Time and spatial averaging of fossil assemblages are pervasive (Behrensmeyer et al., 1992; Behrensmeyer and Chapman, 1993; Kidwell and Holland, 2002), so it is critical to recognize whether the effects of these processes has caused any biotic change to be averaged over a larger scale than that of the climate change itself. This consideration also applies to paleoclimate proxy data; do these proxies reflect changes just within a few meters, at the local landscape, regional, or continental to global scales? Similarly, taphonomic and sampling filters can easily distort observed patterns in the fossil record, leading to erroneous inferences about how these data compare to model predictions (e.g., Vrba, 1995: Figs. 3.3–3.4). As one basic example, richness scales with number of fossil specimens and sites, so if we observe a pulsed change in richness in a dataset it may simply reflect change in sample size rather than a paleobiologic signal. Therefore, taphonomic and sampling control of richness and abundance patterns must be evaluated before using these data to test for climate response.
1.3 Hypothesis Predictions
With the above caveats, the turnover pulse and relay models make the following predictions for previously proposed hypotheses that link Triassic floral and tetrapod clades to paleoclimate conditions:
Hypothesis1a: More humid conditions will be associated with a pulse of plant species first appearances (FAs), and more arid conditions will be associated with a pulse of plant species last appearances (LAs).
Hypothesis1b: During more humid conditions there will be a pulse of hydrophytic and hygrophytic plant species FAs and xerophytic plant species LAs; and during more arid conditions, there will be a pulse of mesophytic and xerophytic plant species FAs and hydrophytic/hygrophytic plant species LAs.
Hypothesis 2: The onset of wetter conditions will be associated with a pulse of synapsid FAs, particularly among traversodontids and dicynodonts, and conversely a pulse of LAs with drier conditions.
Hypothesis 3: The onset of warmer conditions will be associated with a pulse of cynodont FAs, and conversely a pulse of LAs with cooler conditions.
Hypothesis 4: Warmer conditions correlate with a pulse of pseudosuchian archosaur FAs, whereas cooler or more seasonal conditions correlate with a pulse of pseudosuchian LAs.
Hypothesis 5: Rhynchosaur relative abundance increases with the onset of more arid conditions, and decreases with the onset of wetter conditions.
2 Geological Setting
Throughout the Late Permian-Triassic, subduction along the southwestern margin of Gondwana produced the Bermejo half-graben systems along northwest-trending pre-existing Paleozoic sutures (Figure 1) (e.g., Uliana and Biddle, 1988; Uliana et al., 1989; Lovecchio et al., 2020). The extensional Ischigualasto-Villa Unión Basin (IVUB), part of this extensional system, developed as an elongated half-graben rift with NW-SE orientation associated with the Valle Fértil Fault (Figure 1), which is interpreted to be a Triassic normal fault. The IVUB had a half-graben geometry with a deeper area located toward the northwest, at least during the synrift phase(s) as show the architectural and stacking patterns, and paleogeographic distribution of deposits and source areas (Currie et al., 2009; Mancuso and Caselli, 2012; Benavente et al., 2022). The IVUB records the most extensive Triassic Gondwana succession with 2,000–6,000 m of alluvial, fluvial, and lacustrine sediments preserving an exceptionally diverse and abundant floral and faunal fossil assemblage (Stipanicic and Marsicano, 2002). The Triassic infill of the IVUB has not been affected by deep burial (Ruiz and Introcaso, 1999), similar to the adjacent Cuyana rift Basin. The deposits of the two basins have only been affected by the Andes uplift and buried by a relatively thin Cenozoic sedimentary column with no exhumation processes involved (Abarzúa, 2016; Benavente et al., 2019).
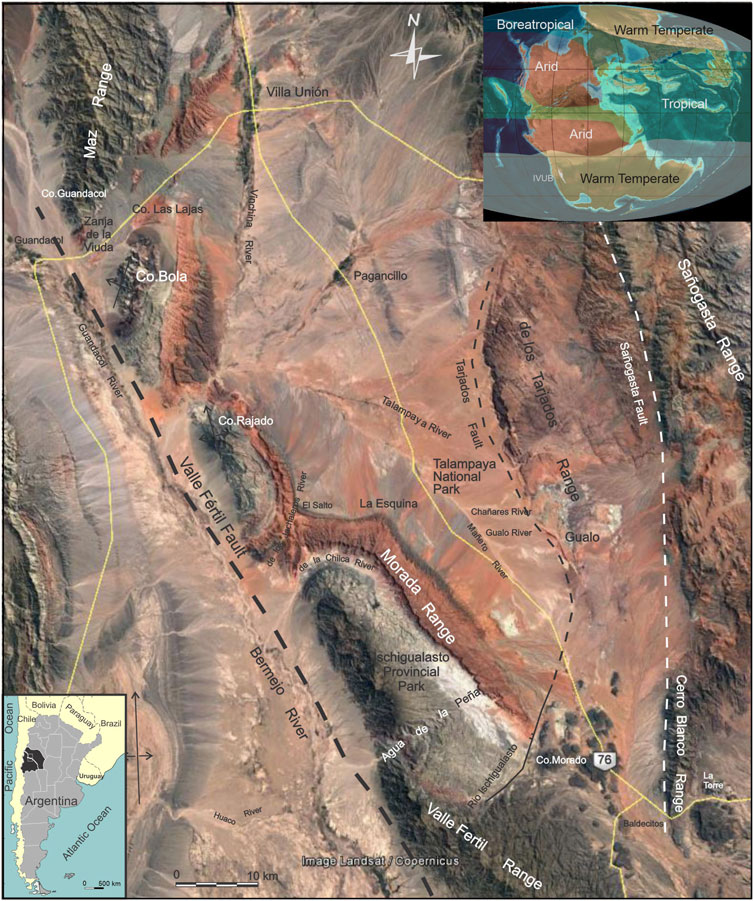
FIGURE 1. Satellite image of the Ischigualasto-Villa Unión Basin with localities relevant to this study labeled. The satellite image is taken from Google Earth. Paleogeographic map for the Late Triassic Period (modified from https://deeptimemaps.com/) with paleoclimate inferred zones from lithological indicators (modified from Boucot et al., 2013).
The Agua de la Peña Group, which includes the Chañares, Los Rastros, Ischigualasto, and Los Colorados formations (Mancuso 2005), unconformably overlies the Tarjados Formation redbeds (López-Gamundí et al., 1989; Caselli, 1998). The four units have conformable gradational boundaries. Between each formation there is a clear change in the depositional system, which makes lithostratigraphic recognition possible, but without marked discontinuity. The lower Carnian Chañares Formation consists of tuffaceous sandstone, siltstone, orthoconglomerate, and paraconglomerate deposited in fluvial and lacustrine-margin settings, and alluvial fans environments, respectively (Rogers et al., 2001; Mancuso et al., 2014; Marsicano et al., 2016), and transitions into the Los Rastros Formation, characterized by a deep lacustrine black shale and deltaic sandstone (Mancuso and Caselli, 2012; Mancuso et al., 2020a; Benavente et al., 2022). Recent radioisotopic ages for the Chañares and Los Rastros formations reveal that they span the latest Ladinian/early Carnian through mid-Carnian (Marsicano et al., 2016; Mancuso et al., 2020a; Irmis et al., 2022). Therefore, both units are considered a lateral variation of the same sedimentary succession (Mancuso and Caselli, 2012; Mancuso et al., 2014, 2020a). The Chañares-Los Rastros succession preserves warmer and more humid conditions than before or after, representing the first detailed paleoclimate data for the CPE in Gondwana based on a multiproxy paleoclimate interpretation (sedimentology, mineralogy, paleontology, and C and O stable isotope data) from the same strata (Mancuso et al., 2020a; Benavente et al., 2022).
The lacustrine-deltaic Los Rastros Formation is conformably overlain by upper Carnian-lower Norian sandstone, mudstone, and tuff deposited in the moderate sinuosity fluvial system of the Ischigualasto Formation (Currie et al., 2009; Martínez et al., 2011; Colombi et al., 2017, 2021). This unit is well-dated by radioisotopic ages (Rogers et al., 1993; Martínez et al., 2011; Desojo et al., 2020; Colombi et al., 2021), and preliminary age modeling indicates it spans from ∼232.5 to ∼224.5 Ma or younger (Irmis et al., 2022). This fluvial system records the wide development of a floodplain, including extensive paleosols (Tabor et al., 2006; Currie et al., 2009). The paleontological content, and their taphonomic features, sedimentological, and pedologic (type of paleosols) evidence suggest seasonal semi-arid conditions, with evidence of semi-humid conditions recorded in the Valle de la Luna Member (Tabor et al., 2004, 2006; Colombi and Parish, 2008; Césari and Colombi, 2013).
Finally, the Ischigualasto Formation is conformably overlain by Norian sandstone and mudstone moderate-sinuosity fluvial red beds of the Los Colorados Formation (Caselli et al., 2001; Santi Malnis et al., 2020). Paleomagnetic dating suggests an age range 227 to 213 Ma, indicating that the Los Colorados Formation was deposited during the early-middle Norian Stage (Kent et al., 2014). The climate condition of the Los Colorados succession is controversial; some authors propose arid conditions mainly based on the red color of the sediments (López-Gamundí et al., 1989; Milana and Alcober, 1994; Cladera et al., 1998), whereas more detailed sedimentological analysis supported the interpretation of moderately sinuous fluvial systems that lack evidence of aridity such as intraclast breccias, desiccation cracks, and eolian or evaporite lenses (Bossi, 1971; Caselli et al., 2001; Arcucci et al., 2004).
3 Materials and Methods
The Flow diagram (Figure 2) summarizes the sedimentological, chronostratigraphic, paleoclimate, and paleontological dataset analyzed in this contribution.
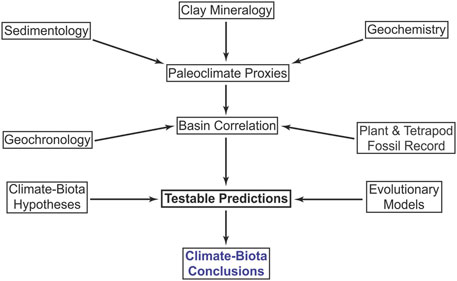
FIGURE 2. Conceptual flow diagram explaining how we synthesized data to test hypotheses for biotic response to climate change in Triassic sediments of the Ischigualasto-Villa Unión Basin, Argentina. Multiple types of geologic data (Sections 3–3.1) were used to infer paleoclimatic proxies (Sections 4.1–4.4), which were combined with data from the fossil record (Sections 3.2, 4.5–4.6) and geochronologic age constraints through basin correlation. These integrated data were then used to test predictions (Sections 1.3, 3.3, 4.7) derived from applying explicit evolutionary models (Section 1.2) to previously-proposed hypotheses (Section 1.1).
We measured and sampled a detailed stratigraphic section of the Ischigualasto Formation at centimeter scale in the NW area of the basin at the Cerro Bola locality (Figure 1). The Rock Color Chart of the Geological Society of America (GSA 1948) was used for Munsell color descriptions. Fresh, unweathered hand samples were collected for clay mineralogical and XRF elemental analysis. The sedimentological analysis consisted of determining facies defined by sedimentary structures and textures and recorded using the acronyms of the lithofacies code proposed by Miall (1996). Facies associations were identified to interpret the depositional environments and sub-environments (Supplementary Table S1).
Paleosol profiles were recognized and described using established criteria (Retallack, 1988; Kraus and Aslan, 1993; Kraus, 1999; Tabor et al., 2006). The top of paleosol was identified where a marked change in grain size, color, and/or sedimentary structures occur, whereas the based was identified where unaltered parent material occurs (Tabor et al., 2006). Thus, the paleosols were classified based on the occurrence of morphological features observable in outcrop and hand sample (e.g., thickness, color, type, and distribution of mottling, soil structure), following previously established by Tabor et al. (2006) for the Ischigualasto Formation.
The mineralogical composition of samples was analyzed with X-ray diffraction (XRD) of the <2 µm sample fraction using a PANalytical X’Pert PRO diffractometer; each sample was prepared with three methods (air-dried, glycolated, and calcined) (see Supplementary Material). For mixed-layer illite/smectite, the contribution of illite was determined by the Reichweite value (R) calculated following Moore and Reynolds (1997). Representative mudstone and silty mudstone samples were analyzed for major and trace element abundances using a Bruker AXS TRACER III-V energy-dispersive hand-held X-ray fluorescence (XRF) unit (see Supplementary Material). Raw data were collected as a spectrogram and converted to weight percent for major elements and ppm for trace elements using a matrix-specific mudstone calibration (Rowe et al., 2012).
3.1 Paleoclimate Proxies
We use multiple proxies including the clay mineral assemblages, kaolinite/illite, smectite/illite, and smectite/kaolinite ratios, molar chemical index of alteration (CIA molar), K/Al, Ba/Sr (Supplementary Tables S3, S4) to reconstruct physical and chemical weathering intensity and seasonality through time (Chamley, 1989; Liu et al., 2005; Clift et al., 2014). The ratios of kaolinite/illite and smectite/illite are proxies for the intensity of chemical weathering, but the latter ratio also includes seasonality as a variable. The smectite/kaolinite ratio reveals a history of chemical vs. physical weathering rates, where the relatively higher ratios suggest a strengthened chemical weathering and weak physical erosion; by contrast, the lower ratios indicate intensified physical erosion and weakened chemical weathering (Chamley, 1989; Weaver, 1989; Ruffell et al., 2002; Fürsich et al., 2005).
The Chemical Index of Alteration (CIA), proposed by Nesbitt and Young (1982), is calculated based on the major element chemistry of mudrocks. We used the convention of converting the raw abundances into moles by dividing the weight percent by molecular weight, which gives the relative abundance on an atomic stoichiometric basis (Retallack, 2001; Sheldon and Tabor, 2009). The CIA (molar) results in a proportion between alumina and alkalis plus calcium:
and was proposed as a more sensitive measure of the degree of chemical weathering (Goldberg and Humayun, 2010; Retallack et al., 2011; Horn et al., 2018b). The CIA, K/Al, and kaolinite/illite proxies are based on the principle that larger cations (Al) remain fixed in the weathering profile. In contrast, smaller cations (Ca, Na, K) are selectively leached during alteration. Further, the chemical weathering intensity can be estimated based on the ratio for a single element (i.e., K) mobilized during weathering of silicates in comparison to that of a non-mobile element (e.g., Al) (Gaillardet et al., 1999; Garzanti et al., 2014).
Variation of the Ba/Sr ratio has also been considered a proxy for leaching intensity (Gallet et al., 1996; Sheldon and Tabor, 2009), because an increase in chemical weathering intensity rapidly leaches out Sr compared to Ba (Nesbitt and Young, 1982). However, it is necessary to consider that different parent materials have variable initial concentrations and can reduce the leaching elements in high water table soils.
Based on the comprehensive discussion in Sheldon and Tabor (2009) and Tabor and Myers (2015), we selected the following paleotemperature and paleoprecipitation equations. Sheldon et al. (2002) proposed the following based on ΣBases/Al for the Bw or Bt horizons for precipitation:
This equation was used on the Ischigualasto horizons interpreted as protosols. On the other hand, the calcium-magnesium weathering index (CALMAG) in relation to MAP developed for vertisols (Nordt and Driese, 2010) was applied to the Ischigualasto levels interpreted as vertisol and calcisols:
In this study, the CIA-K equation (Sheldon et al., 2002) was not used because it is inappropriate for calcisols and gleyed soils (Sheldon and Tabor, 2009; Tabor and Myers, 2015). For our Ischigualasto data, MAP estimates from the CIA-K equation overestimates the paleoprecipitation compared to other weathering and humidity indexes.
The relationship between MAT and “clayeyness” (Al/Si) of the Bw or Bt horizon was proposed by Sheldon (2006) for poorly-developed paleosols:
This regression was used for Ischigualasto levels that show incipient paleosol features such as poor mottling and poorly defined soil horizons (horizonation). The paleosol weathering index (PWI) to estimate MAT proposed by Gallagher and Sheldon (2013) take on Na and K are more easily weathered and leached from soils than are Mg and Ca:
were used for mottled paleosols with well-developed Bt horizons. The salinization estimation equation ((K2O+ Na2O)/Al2O3; Sheldon et al., 2002) was not used for warmer Mesozoic climates, because that equation underestimates MAT in environments with seasonal precipitation (Sheldon and Tabor, 2009; Tabor and Myers, 2015). The use of several equations to estimate MAP and MAT was previously proposed (Sheldon and Tabor, 2009; Tabor and Myers, 2015), and is appropriate for the Ischigualasto succession because of the different kinds of paleosols recorded. These equations provide complimentary data based on the characteristic of each equation reducing the over- and under-estimation of paleoprecipitation and paleotemperature from using a single relationship (Supplementary Table S5). However, the use of more than one equation shows an artificial discontinuity in the dispersion graph (Supplementary Figure S1).
3.2 Paleontological Data
A literature review allowed us to generate a database that includes the plant (micro and macro-fossil) and tetrapod taxa known from each unit of the Agua de la Peña Group, as of November 2021. The tables with the taxa registered in each unit are available in (Supplementary Tables S6–S8). The complete list of the references consulted can be found also in the (Supplementary Table S9).
The plant macrofossil occurrences from the Los Rastros, Ischigualasto, and Los Colorados formations were organized by more inclusive taxonomic groups. This was carried out following the systematic schemes proposed by Stewart and Rothwell (1993) for gymnosperms, Smith et al. (2006), and PPG (2016) for ferns (Supplementary Table S10). For palynomorphs recorded from the same formations, an attempt was made to establish the botanical affinity of the miospores with the plant group interpreted as the most likely producer based on Ruffo Rey (2021) and references therein (Supplementary Table S11). Lastly, the hypothesized temperature preference and humidity requirement of the taxa were assigned according to Zhang et al. (2020) and Ruffo Rey (2021) (Supplementary Table S12).
For tetrapod fossils, we assembled a database of taxa and specimens using Martínez et al. (2011) as a foundation, augmenting it with data from Desojo et al. (2020) and other more recent publications (Supplementary Table S13). The Chañares tetrapod data are derived from Mancuso et al. (2014) and Ordoñes et al. (2020), whereas the Los Rastros tetrapod data are assembled from Marsicano et al. (2004, 2007, 2010) and Mancuso and Marsicano (2008). Given the thermal tolerance of modern groups (Bennett, 2018), Liu et al. (2021) proposed preferred temperatures for Permo-Triassic tetrapod groups. Additionally, Dunne et al. (2021) explored the paleolatitudinal distribution of the major Late Triassic tetrapod groups, defined the paleoclimatic ranges that they occupied, and proposed a range of climate preferences for each major Late Triassic tetrapod group (see Figure 6 in Dunne et al., 2021). Based on the inferences of this previous work, we grouped the major tetrapod clades recorded in the Late Triassic Agua de la Peña Group into relative temperature and precipitation preferences (Supplementary Table S14).
3.3 Hypothesis Testing
To test the previously proposed hypotheses for climate forcing of the fossil biota preserved in the Ischigualasto Formation, we used the stratigraphic bins of Martínez et al. (2011) to examine changes in FAs, LAs, and relative abundance of taxa through the section. Because there is always going to be a ‘background’ rate of FAs and LAs, we focused on outlier datapoints where these rates are elevated; i.e., possible evidence of Vrba’s pulses. This record of FAs/LAs was also compared to specimen sample size for each bin to examine how sampling might be driving the observed appearance and disappearance of taxa. To further test the effect of sampling on observed diversity at a larger scale, we used rarefaction (Raup, 1975; Tipper, 1979), implemented in the software package Analytic Rarefaction v. 1.3 (Holland, 2003), to examine whether different sample sizes might explain the different species richness of the Scaphonyx-Exaeretodon-Herrerasaurus biozone vs. the Exaeretodon biozone. Finally, to examine whether observed differences in relative abundance relate to sample size changes across bins, we used PAST v. 4.03 (Hammer et al., 2001) to calculate adjusted residuals for each bin and conduct a composite Chi-squared test for the taxon of interest vs. the rest of the dataset (Grayson and Delpech, 2003; Faith and Lyman, 2019).
4 Results
The fluvial paleoenvironment recorded in the Ischigualasto Formation is well-described in numerous studies from the past 75+ years (Frenguelli, 1944, 1948; Bossi, 1971; Rogers et al., 1993; Currie et al., 2009; Colombi et al., 2021). The Ischigualasto Formation sequence we measured and described at the Cerro Bola locality (Figures 1, 3) preserves similar sedimentological characteristics to previously studied localities (Supplementary Table S1). The fluvial system is dominated by multi-story channels and single-story channels associated with levee facies in some cases and floodplain facies in others. The crevasse channel and crevasse splay facies are interbedded with floodplain facies. These floodplain facies are dominated by paleosols (protosol, vertisol, gleyed vertisol, argilllic vertisol, argillic calcisol, and calcisol). In agreement with previous work, the system at this locality shows a general flow to the north (NE-NW) (Currie et al., 2009). The correlation though basin for the Ischigualasto Formation was based on a distinctive thick volcaniclastic horizon used as the datum among the Ischigualasto Provincial Park, Cerro Bola, and Las Lajas sections (see Supplementary Figure S3).
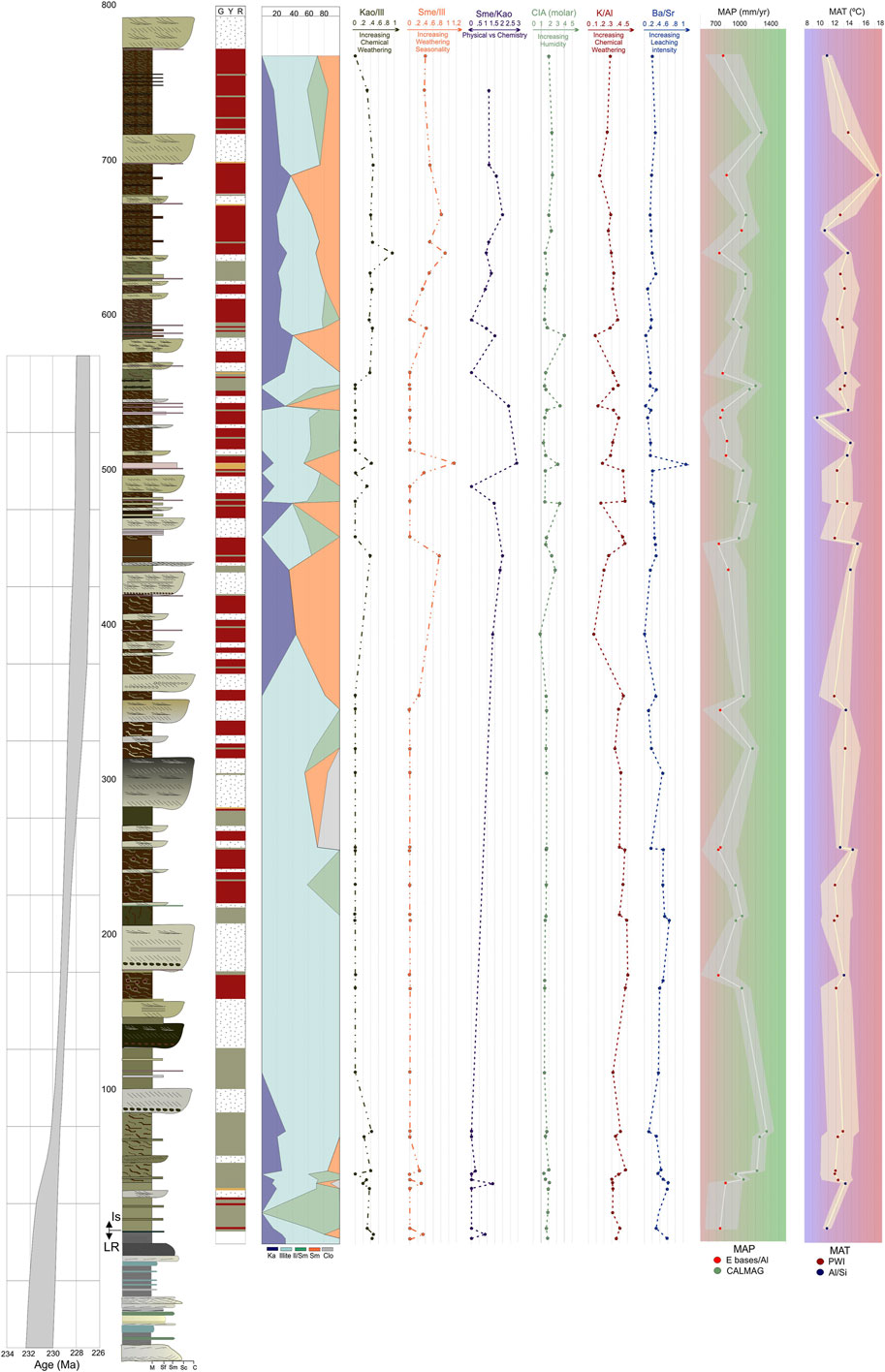
FIGURE 3. Detailed stratigraphic section measured of the Ischigualasto Formation at the Cerro Bola area with the plot of the paleoclimate proxies. Geochronologic age constraints for the Ischigualasto Formation are from the preliminary Bayesian age model with uncertainty envelope (gray shading) for the unit based on radioisotopic ages (Irmis et al., 2022).
4.1 Clay Mineralogy
Ischigualasto Formation clay mineralogy at the Cerro Bola locality is characterized by assemblages that include smectite, illite, mixed-layer illite/smectite, kaolinite, and chlorite (Figure 3, Supplementary Table S2). This compares well with previously published clay mineralogy records from other localities (Agua de la Peña, Río Ischigualasto, and Zanja de la Viuda) in the basin, which also show variable dominance of smectite, illite, kaolinite, chlorite, and mixed-layer illite/smectite (Bossi, 1970; Ovejero and Bossi, 1984).
Abundant illite might suggest four possible processes: the leaching of smectite (Chamley, 1989); leaching of acidic rock such as volcanic (Singer, 1980); burial diagenesis (Fursich et al., 2005); and/or enhanced weathering under an increasing hydrolysis index (Fürsich et al., 2005; Do Campo et al., 2010). The illite abundance is variable up-section and its crystallinity is low, which rules out leaching and diagenesis processes (Fürsich et al., 2005; Barrenechea et al., 2018). Therefore, the most likely explanation for abundant illite is an enhanced hydrolysis index (HI) due to increased erosion at the catchment of the basins (Fürsich et al., 2005; Do Campo et al., 2010; Rostási et al., 2011; Mancuso et al., 2020b). In the cases where these assemblages include smectite, this suggests seasonal conditions that point to enhanced erosion during the wet season (Chamley, 1989). Mixed-layer illite/smectite with a value of R3 is interpreted as an indicator of leaching (Bossi, 1970; Moore and Reynolds, 1997). Chlorite is commonly found in soils as a primary (authigenic) product, but it can also be a detrital source (Moore and Reynolds, 1997). The few levels that recorded low proportions of chlorite have subtle pedogenic features; therefore, we interpret that the presence of chlorite in these samples might be due to transport from a different source for sediment supply, possibly granitic bedrock (Chamley, 1989). Thus, the second assemblage group (illite, mixed-layer illite/smectite, smectite, and trace chlorite) can be interpreted as formed in high erosion rates under seasonal climate conditions. In the assemblages of the third and fourth groups, the presence of kaolinite might point to reworked felsic volcanic deposits; and/or to more warm and humid conditions (Singer, 1984; Kalm et al., 1996). The assemblages of illite and kaolinite, including detrital illite, most likely indicate enhanced leaching from a high water regime under warm and humid conditions. Therefore, these assemblages can be interpreted as produced by an enhanced HI under warm, humid conditions (Chamley, 1989; Milroy et al., 2019). Finally, in different proportions, the fourth category of assemblages containing illite, mixed-layer illite/smectite, smectite, and kaolinite can also be interpreted as formed by enhanced HI in relatively more humid and seasonal conditions (Chamley, 1989; Do Campo et al., 2010).
Overall, the general clay mineralogy trend suggests a fluctuation between humid and warm conditions (Los Rastros Fm) (Mancuso et al., 2020a; Benavente et al., 2022) and more seasonal and less humid conditions represented by the abundance of smectite and relatively less kaolinite up-section (Ischigualasto Fm). The overall more seasonal and less humid conditions in the Ischigualasto Formation begin with warm and humid seasonal conditions in the first ∼100 m, with a variable combination of kaolinite, smectite, illite, and mix-layer illite/smectite, followed by less humid seasonal conditions with enhanced HI, characterized by the dominance of illite. Finally, above the ∼380 m level there is a return to more humid seasonal conditions, with a variable combination of kaolinite, smectite, illite, and mix-layer illite/smectite. The high variability found in the mineralogical assemblages of the studied unit reflects variable sources of minerals for the fluvial system (Bossi, 1970).
4.2 Mineralogical Proxies
Clay mineralogical proxies (kaolinite/illite, smectite/illite, and smectite/kaolinite ratios) display temporal trends in both physical erosion and chemical weathering (see Supplementary Material). The kaolinite/illite ratio trend suggests moderate to high chemical weathering in the first ∼100 m and upper ∼380 m of the section, and low chemical weathering between ∼100 and 380 m. The smectite/illite ratio trend suggests moderate weathering seasonality in the lowermost ∼100 m, low weathering seasonality between ∼100 and 380 m, and high weathering seasonality above ∼380 m. The smectite/kaolinite ratio suggests predominantly physical weathering in the first ∼100 m, and fluctuation between physical and chemical weathering above the ∼380 m level in the section.
4.3 Major and Trace Element Proxies
The trend in CIA molar estimates supports a sub-humid interpretation for the first ∼380 m of section and above ∼600 m in section, and more variable humid conditions between ∼380 and 600 m. The K/Al ratio reflects high chemical weathering below ∼380 m, and variable chemical weathering above this horizon. Finally, the Ba/Sr ratio suggests extensive leaching conditions below ∼380 m, and less leaching above (see Supplementary Material).
4.4 Paleoprecipitation and Paleotemperature Estimates
Our MAP estimates vary between 721 and 1,343.5 mm/yr, and our MAT estimates vary between 9.41 and 17.52°C (Figure 2; Supplementary Table S5). Despite this variability, the general trends through the section appear to vary around a mean MAP of 950 mm/yr, and display a subtle directional change in MAT from 12.5 to 13.5°C. Particularly notable is that lower dispersion of MAT values is observed in between the base of the section and ∼380 m level, in contrast with the higher dispersion recorded above this interval. However, of the seven data points with the lowest MAP and highest MAT values, three occur in the lower part of the section (Figure 3; Supplementary Table S5).
Therefore, despite some small discrepancies, these multiple proxies are in general agreement with the stratigraphic trend in paleoenvironmental conditions. Evidence of warm and humid conditions with moderate seasonality and chemical weathering dominate the lower part section (basal 100 m). Towards the middle part of the section (from 100 to 380 m) there is evidence for predominantly sub-humid to semi-arid conditions with variable chemical weathering and moderate to low seasonality. Finally, above the 380 m level, these data suggest more humid seasonal conditions with variation in seasonality and chemical weathering.
4.5 Plant Fossil Record
The Upper Triassic, particularly the Stage Carnian, is the interval where the Dicroidium flora is hypothesized to have diversified and spread across Gondwana (McLoughlin, 2001, 2011; Kustatscher et al., 2018; Bodnar et al., 2021). The formations of the Agua de la Peña Group preserve the typical Dicroidium floral assemblage; the main differences are in the specific richness of each unit (see Supplementary Tables S6, S7).
The Los Rastros Formation preserves the highest species richness with 72 taxa, followed by the Ischigualasto Formation with 24 taxa, and finally, the Los Colorados Formation with only three taxa (Figure 4A; Supplementary Table S6). Members of Lycopodiopsida and Czekanowskiales are only recorded in the Los Rastros Formation (Figure 4A; Supplementary Table S6). Moreover, the Los Rastros Formation preserves a higher number of taxa belonging to the groups Equisetopsida, Polypodiopsida, Umkomasiales, Peltaspermales, Ginkgoales, Voltziales, and gymnosperms with uncertain affinity, in comparison with the Ischigualasto and Los Colorados formations (Figure 4A; Supplementary Table S6). In contrast, Cycadales and Bennettitales are better represented in the Ischigualasto Formation, while the number of Gnetales is equal in the Los Rastros and Ischigualasto formations (Figure 4A; Supplementary Table S6).
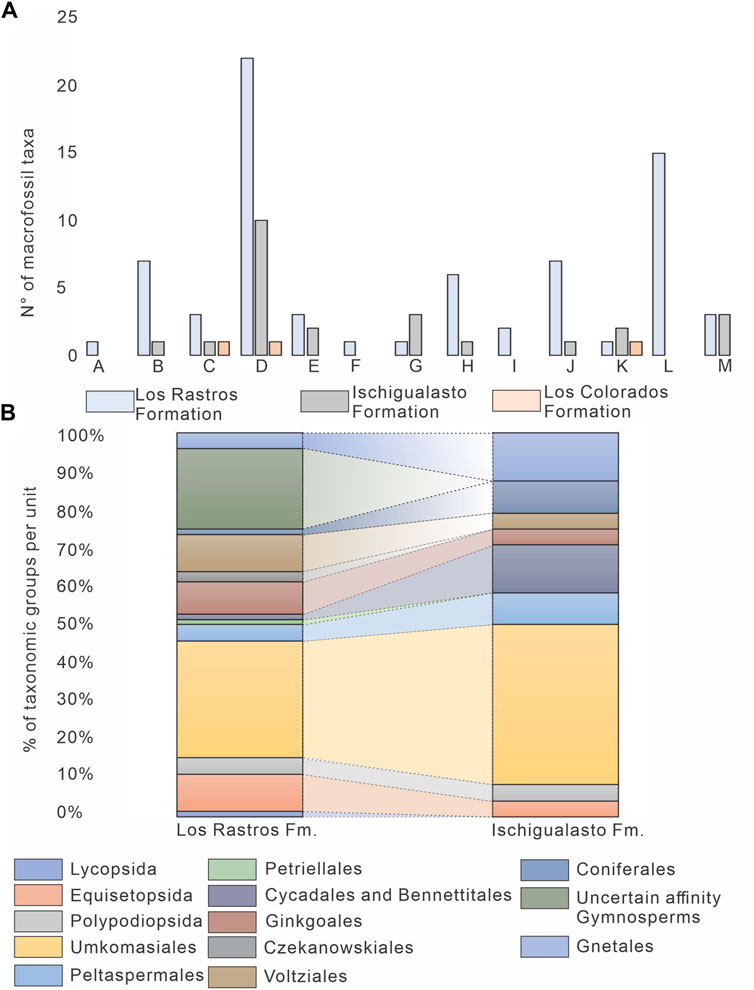
FIGURE 4. Paleobotanical record of the Los Rastros, Ischigualasto, and Los Colorados formations. (A). Number of macrofossil taxa per taxonomic group in each unit. A. Lycopsida, B. Equisetopsida, C. Polypodiopsida, D. Umkomasiales, E. Peltaspermales, F. Petriellales, G. Cycadales and Bennettitales, H. Ginkgoales, I. Czekanowskiales, J. Voltziales, K. Coniferales, L. Uncertain affinity Gymnosperms, M, Gnetales. (B). Percentage of taxa of each taxonomic group registered in the Los Rastros and Ischigualasto formations.
This trend in species richness, particularly the number of taxa per group, shows the predominance of Umkomasiales followed by Ginkgoales, Voltziales, and gymnosperms with uncertain affinity in the Los Rastros Formation, whereas in the Ischigualasto Formation the Umkomasiales continue to dominate but Cycadales, Bennettitales, and Coniferales represent a higher percentage of diversity, and Equisetopsida, Ginkgoales, Voltziales, and gymnosperms with uncertain affinity are more species-poor (Figure 4B; Supplementary Table S6). Groups such as the Petriellales and Czekanowskiales are only recorded in the Los Rastros Formation, and taxa such as Polypodiopsida (ferns) represent a similar percentage of the vegetation in the Los Rastros and the Ischigualasto formations (Figure 4B; Supplementary Table S6). The scarcity of plant macrofossils in the Chañares and Los Colorados formations means their assemblages cannot be used to assess changes in richness (see Supplementary Material).
The palynomorph record is more diverse, with 42 taxa recorded for the Chañares Formation, 212 taxa from the Los Rastros Formation, and 90 taxa for the Ischigualasto Formation (Supplementary Table S7). Miospores in the Chañares Formation indicate the presence of Lycopodiopsida, Polypodiopsida, Peltaspermales, Umkomasiales (Perez Lonaize et al., 2018). In the Los Rastros Formation, palynological data indicate the presence of taxa not represented by macrofossil remains, such as Caytoniales, and different groups of Coniferales such as Podocarpaceae and Araucariaceae (Supplementary Table S7). The same trend is observed with spores indicating the presence of Lycopodiopsida in the Ischigualasto Formation (Supplementary Table S7).
4.5.1 Plant Paleoecology
The plant macrofossil record in the Los Rastros Formation is dominated by taxa that previous authors have inferred to be hygrophytic (60%), followed by mesophytic taxa (38%), and hydrophytic taxa (2%). In the Ischigualasto Formation, inferred hydrophytic taxa dominate (58%) followed by mesophytic (42%) taxa. Finally, the overlying Los Colorados Formation is also dominated by taxa inferred to be hygrophytic (67%) and mesophytic (33%), but this comprises only three taxa (Figure 5A; Supplementary Table S10). Although the number of taxa interpreted as megathermic taxa in the Los Rastros Formation was represented by 64% and 36% were mesothermic taxa (Supplementary Table S10). In the Ischigualasto Formation 75% were megathermic and 25% mesothermic taxa. Finally, the three taxa mentioned in the Los Colorados Formation are interpreted as megathermic taxa (Figure 5B; Supplementary Table S10).
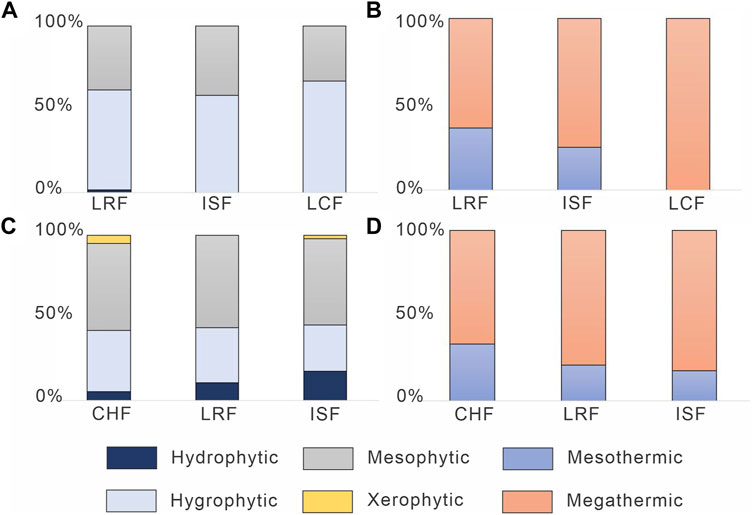
FIGURE 5. Inferred plant temperature and humidity preference based on previous authors. (A). Percentages of hydrophytic, hygrophytic, and mesophytic macrofossil taxa. (B). Percentage of mesothermic and megathermic macrofossil taxa. (C). Percentages of hydrophytic, hygrophytic, mesophytic, and xerophytic microfossil taxa. (D). Percentage of mesothermic and megathermic microfossil taxa. CHF, Chañares Formation; ISF, Ischigualasto Foramtion; LCF, Los Colorados Formation; LRF, Los Rastros Formation.
Interestingly, there appear to be no substantial changes in the floral composition, at least with respect to moisture requirement, among the three units (Figure 5A). However, inferred temperature tolerance shows a progressive increase from the Los Rastros Formation to the Los Colorados Formation based on the proportion of megathermic taxa compared to mesothermic elements (Figure 5B). But, this trend should be interpreted with caution given that the Los Colorados assemblage contains only three taxa.
In the Chañares palynomorph assemblage, inferred mesophytic taxa dominate (53%), with 37% inferred hygrophytic taxa, and ∼5% hydrophytic and xerophytic taxa (Figure 5C; Supplementary Table S11). In the Los Rastros Formation, 56% of the palynomorph taxa are interpreted as mesophytic, 33% as hygrophytic, and 11% hydrophytic (Figure 5C; Supplementary Table S11). In the Ischigualasto Formation, 52% are inferred to be mesophytic taxa, 28% hygrophytic, 18% hydrophytic, and 2% xerophytic (Figure 5C; Supplementary Table S11). In terms of temperature tolerance, 67% of Chañares Formation taxa are interpreted as megathermic taxa and 33% mesothermic (Figure 5D; Supplementary Table S11), whereas 79% of Los Rastros Formation taxa are interpreted as megathermic and 21% as mesothermic (Figure 5D; Supplementary Table S11). Finally, in the Ischigualasto Formation 83% of taxa are interpreted as megathermic and 17% are interpreted as mesothermic (Figure 5D; Supplementary Table S11).
The general trend suggests that the Chañares and Ischigualasto formations are similar in containing a small number of inferred xerophytic taxa, but the latter unit has a higher proportion of hydrophytic taxa (Figure 5C). In general, the proportion of hydrophytic elements increases up-section from the Chañares through Ischigualasto formations (Figure 5C). The lack of inferred xerophytic taxa in the Los Rastros Formation could be evidence that the pulse of warm and humid conditions during the CPE displaced species with drier habitat preferences. Finally, through the section there is a decrease in inferred mesothermal taxa with a relative increase in megathermal taxa (Figure 5D).
4.6 Tetrapod Fossil Record
In terms of species richness, the Chañares Formation is dominated by synapsids (dicynodonts and cynodonts) followed by archosauriforms (proterochampsids, pseudosuchians, and dinosauromorphs) (Figure 6; Supplementary Table S8). The body fossil and ichnological record of the Los Rastros suggest at least the presence of these same groups plus temnospondyls, but ichnotaxonomic richness is not comparable to the body fossil record (Figure 6; Supplementary Table S8). Therapsids (dicynodont and cynodont) continue to dominate in the Ischigualasto assemblage, but are proportionally less species-rich due to the rise in species richness of pseudosuchians, rhynchosaurs, and dinosaurs (Figure 6; Supplementary Table S8). Finally, in the Los Colorados Formation, the tetrapod assemblage is dominated by dinosaurs and pseudosuchians, with a lower proportion of therapsids (dicynodont and cynodont) and testudinatans (Figure 6; Supplementary Table S8). Thus, through the Late Triassic Agua de la Peña Group, dinosaurs and pseudosuchians taxa become more species-rich, coincided with a decrease in early archosauromorph and synapsid richness (Figure 6; Supplementary Table S8), as discussed by previous authors (Irmis, 2011; Marsicano et al., 2016). However, it is important to note that the quality of the body fossil record, as well as the ichnological record, is driven by preservational conditions in the basin (see Supplementary Material).
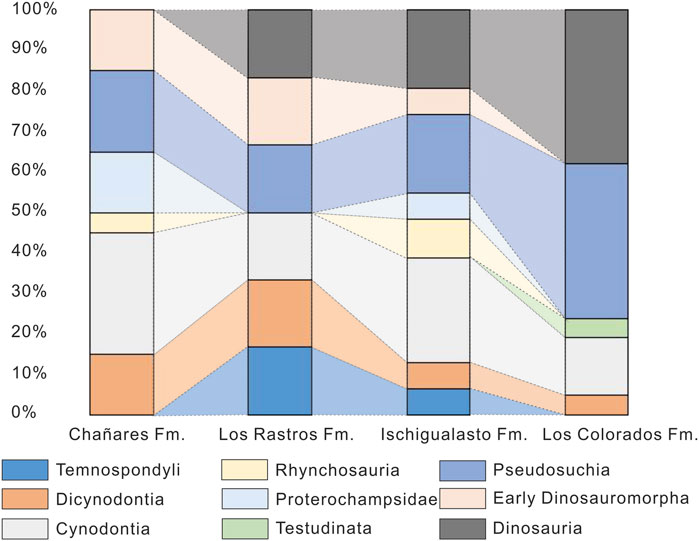
FIGURE 6. Paleovertebrate record in the units of the Agua de la Peña Group. Percentage of taxa of each taxonomic group registered in each formation.
4.6.1 Tetrapod Paleoecology
The habitat preferences inferred for fossil tetrapods by previous authors are relatively consistent through the Agua de la Peña Group (Figure 7A; Supplementary Table S15). All the Agua de la Peña units are dominated by taxa (60%) that are thought to prefer temperate conditions (between 20 and 30 C) followed by taxa (40%) that are thought to prefer high temperature (>30°C). A similar split is observed for inferred seasonality preferences, with 60% of taxa tolerant of high seasonal variation, and 40% of taxa tolerant low seasonal variation (Figure 7B; Supplementary Table S15). In contrast, there appears to be a trend of increasing species richness among taxa that are inferred to prefer wetter conditions (>2 mm/day), from 15% in the Chañares to 40% in the Los Colorados Formation, whereas taxa that might prefer mid-range precipitation values (between 1.5 and 2 mm/day) decrease (Figure 7C; Supplementary Table S15). The tolerance to seasonal variation in precipitation displays a similar pattern to temperature (Figure 7D; Supplementary Table S15).
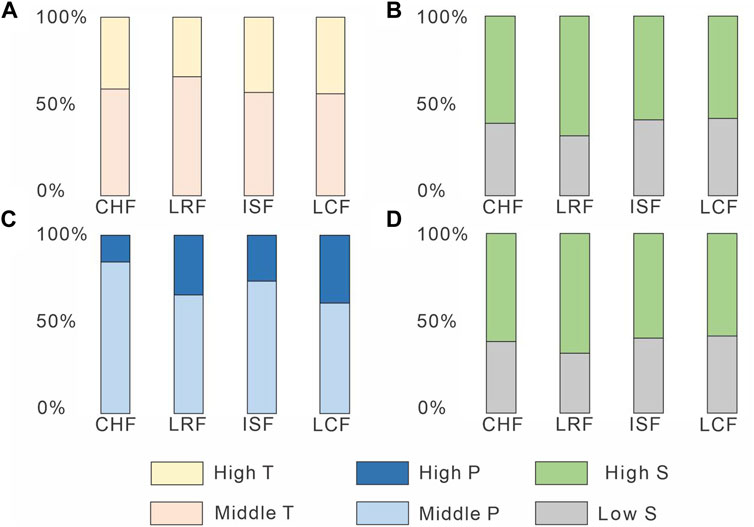
FIGURE 7. Inferred tetrapod habit preference (based on previous authors) for the Upper Triassic Agua de la Peña Group. (A). Percentage of prefered range temperature tetrapod taxa. (B). Percentage of tolerance to seasonal variation in temperature. (C). Percentage of prefered range precipitation tetrapod taxa. (D). Percentage of tolerance to seasonal variation in precipitation. CHF, Chañares Formation; ISF, Ischigualasto Foramtion; LCF, Los Colorados Formation; LRF, Los Rastros Formation.
4.7 Hypothesis Testing
The bulk of fossil assemblages from the Chañares, Los Rastros, and Los Colorados formations are restricted to narrow intervals that are stratigraphically disjunct from each other and from the more continuous record of the Ischigualasto Formation. Therefore, only the Ischigualasto Formation is densely sampled enough to test the hypothesis predictions we outline at the beginning of this contribution. However, because the Ischigualasto Formation is unevenly sampled for plant fossils, with diagnostic specimens largely restricted to a few levels in the middle part of the formation (Figure 8), there are not enough data points to evaluate changes in diversity, relative abundance, or FA/LA pulses. As such, we cannot directly test the aforementioned floral predictions (hypotheses 1a-1e in Section 1.3) in the context of changes within the Ischigualasto Formation. Therefore, the rest of this section focuses on the tetrapod body fossil record, which is well-sampled and spans nearly the entire formation.
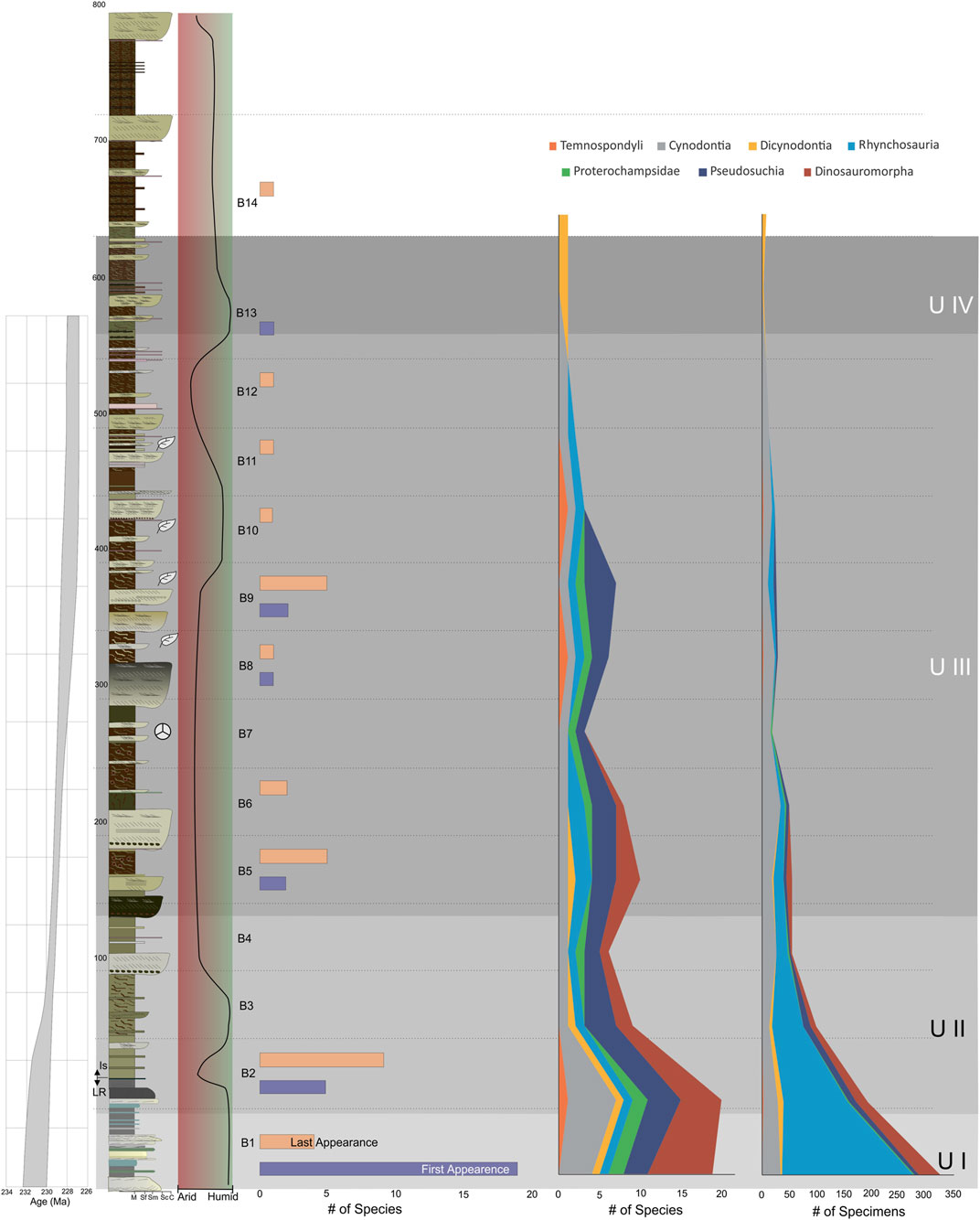
FIGURE 8. Ischigualasto Formation stratigraphic section including paleobotany levels distribution, first and last appearance of tetrapod record, and tetrapod diversity (number of species) and abundance (number of specimens) in the different Ischigualasto time bins as defined by Martínez et al. (2011). Data modified from Colombi and Parrish (2008), Colombi et al. (2011), Cesari and Colombi (2013), Martínez et al. (2011), Desojo et al. (2020), and Drovandi et al. (2021). Age model same as Figure 2.
4.7.1 Species Richness
As noted by previous authors (e.g., Martínez et al., 2011; Colombi et al., 2021), one of the most striking patterns in the Ischigualasto Formation tetrapod fossil record is the long-term decline in species richness up-section. The lowest 50 m (bins 1 & 2) of the formation are most diverse, both in total richness and for most individual clades (e.g., Cynodontia, Proterochampsidae, Dinosauromorpha). In fact, the absence of some taxa higher in the section has been used to define biozones in the formation, with the lowest 300 m of the formation grouped into the Scaphonyx-Exaeretodon-Herrerasaurus biozone, and the overlying ∼350 m separated into the Exaeretodon biozone (Martínez et al., 2011). The boundary between the two biozones is defined by the LA of the rhynchosaur Scaphonyx and dinosaur Herrerasaurus, rather than the FA of any taxon.
Given that species richness scales directly with sampling (i.e., the more specimens you find, the more species you are likely to find) (e.g., Raup, 1972, 1975), a natural question is whether the declining species richness of the Ischigualasto Formation reflects original assemblage composition or is at least in part an artifact of sample size, given that the number of specimens for each stratigraphic bin also declines up-section (Figure 8). In fact, a simple bivariate regression reveals a strong linear relationship (R2 = 0.8179) between the number of specimens and species richness of each bin (Supplementary Figure S4). Similarly, when compared at the same number of specimens, the 95% confidence bounds greatly overlap for rarefaction curves of the Scaphonyx-Exaeretodon-Herrerasaurus and Exaeretodon biozones (Supplementary Figure S4). Both of these analyses suggest that sampling (i.e., number of collected specimens) is a significant control on observed tetrapod species richness in the Ischigualasto Formation. Therefore, absolute species richness of the Ischigualasto Formation cannot be taken at face value for paleoecological interpretation, and must take sampling into account.
4.7.2 First and last Appearances
Given the patterns of tetrapod species richness observed in the Ischigualasto Formation, it is unsurprising that FAs and LAs follow a similar trend, with the bulk of these events concentrated in the lower part of the formation, though there appear to be two LA pulses in the middle part of the formation (Figure 8). It is important to note that the FAs/LAs of the first and last stratigraphic bin are almost certainly artifacts. With the exception of the dicynodont Jachaleria, all other taxa are restricted to the Ischigualasto Formation (i.e., they do not occur in the underlying Los Rastros Formation or overlying Los Colorados Formation). As such, there is necessarily a pulse of FAs in the lowest bin of the Ischigualasto Formation, and any taxa present in the highest bin also have their LA in that bin. Because the Los Rastros and lower Los Colorados Formation have a very poor body fossil record, with present data it is impossible to evaluate whether any Ischigualasto Formation taxa might have had longer stratigraphic ranges that extended into these formations. As such, the FAs of the oldest stratigraphic bin and LAs of the youngest stratigraphic bin are excluded from further analysis and interpretation.
Before using these data to test hypotheses of climate forcing (Section 1.1), it is important to evaluate the effect of sampling on observed FAs and LAs, given that it appears to be a primary control of tetrapod richness in the Ischigualasto fossil record. The number of first appearances in a bin is correlated with the number of specimens in each bin (R2 = 0.5296) suggesting some sampling control, but the number of last appearances per bin is less so, with only a weak correlation (R2 = 0.2790) (Supplementary Figure S5). This suggests that observed FA pulses are difficult to interpret (with no bins where number of FAs is much greater than expected given sample size), LA pulses might be more informative. Three LA pulses are observed in the Formation: bin 2, bin 4, and bin 9 (Figure 8), all of which have more LAs than expected given their specimen sample size (Supplementary Figure S5). The pulse in bin 2 (LAs of 1 temnospondyl, 5 synapsid, 1 proterochampsid, and 2 dinosauromorph species) is difficult to interpret; although it is the second best sampled bin, this interval still contains ∼40% fewer specimens than the underlying bin 1, so one might expect a large number of rare taxa to disappear from the record in bin 2 as sampling decreases. That said, this interval does preserve a short-term change towards more arid conditions (Figures 3, 8). Bin 5 (LAs of 1 synapsid, 1 pseudosuchian, and 3 dinosauromorph species) does not appear to correlate with any clear changes in available paleoclimate proxies. Bin 9 is the most intriguing LA pulse, because it is associated with a significant change to more humid seasonal conditions inferred from our paleoclimate proxies at the ∼380 m level (Figures 3, 8). All of the LAs in bin 9 are archosauriform reptile species (1 proterochampsid, 4 pseudosuchians).
How do these results compare with our predictions for paleoclimate forcing of species FAs and LAs? None can be fully tested, mostly because Ischigualasto tetrapod FAs appear to be controlled by sampling. Therefore, we cannot confidently use the record of FA pulses to interrogate our hypotheses. Nonetheless, to the extent possible, below we evaluate the extent to which our results conform to or contradict predictions, with a focus on the LA record.
Hypothesis 2: The only significant pulse for synapsids is in bin 2, with three FAs and four LAs. This bin is associated with a short-term pulse in arid conditions, which is consistent with the prediction for a pulse in synapsid LAs. Alternatively, most of these LAs are relatively rare taxa, and their disappearance could just as well be explained by declining sample size up-section.
Hypothesis 3: The only observed cynodont FA and LA pulse is the aforementioned event in bin 2, but there is no clear paleotemperature proxy change in this interval. Indeed, paleotemperature estimates are relatively stable for the lower half of the Formation (bins 1–10) (Figure 3). Therefore, this hypothesis is not supported by the available data.
Hypothesis 4: A significant pulse (4 species) in pseudosuchian archosaur LAs is observed in bin 9, which is associated with a shift to more humid seasonal conditions (∼380 m). However, this hypothesis specifically links a pseudosuchian LA pulse to cooler, more seasonal conditions, rather than changes in aridity/humidity. Available paleoclimate proxies do not show clear evidence for cooler conditions at this time, but all proxies display increasing variability and clay mineralogy (smectite/illite ratio) suggests evidence for increasing seasonality (Figure 3). Thus, our data could be consistent with this hypothesis, but the lack of a strong association with paleotemperature changes makes it equivocal.
A final important observation is that the number of FAs in a bin is strongly correlated with the number of LAs in the same bin (R2 = 0.8571) (Supplementary Figure S5). This contrasts with the predictions of Vrba’s relay model, where FA and LA pulses should be separated in time (e.g., Vrba, 1995: Fig. 3.3). There could be several explanations for this. One possibility is that empirical reality (at least in the case of the Ischigualasto Formation) does not match the theoretical model, suggesting other processes are affecting the first and last appearances of taxa, or that the model needs revision. An alternative, and perhaps more likely explanation, is that this result is an artifact of the resolution of our data. As Vrba (1995) discussed, taphonomic and sampling biases can appear to compress the record so that chronologically separate events appear to occur at the same time in the fossil record. Specifically in our case, bin size and duration seems a likely culprit. According to the age model (Figures 3, 8), each of these bins represent anywhere from ∼20,000 to ∼780,000 years (mean duration 290,000 years; median duration 300,000 years). Thus, in a single data point (i.e. bin) we are time-averaging first and last appearances over 103–106 years, and it is exactly at this timescale that Vrba (1992, 1995) hypothesized the relay model should play out. So it seems reasonable that our bins are simply too large in duration to distinguish in time between associated but temporally separate FA and LA pulses.
4.7.3 Relative Abundance
One of the most striking patterns in tetrapod relative abundance in the Ischigualasto Formation is that rhynchosaurs (specifically Scaphonyx) are exceedingly common near the base of the formation, but quickly decline and disappear by the middle third of the Formation (bin 8) (Figure 8), so much so that the lowest biozone in the Formation is named in part for Scaphonyx (Martínez et al., 2011). But this trend could be driven by sampling, given that the decline in Scaphonyx relative abundance mirrors the up-section decline in overall specimen sample size. The adjusted residuals (ARs) (Supplementary Table S16) indicate this is not the case; the positive ARs for bins 1 and 2 indicate Scaphonyx is over-represented relative to what is expected given sample size, and the negative ARs for bins 5–12 indicate it is under-represented relative to what is expected. Furthermore, the Chi-squared test indicates this difference is significant (p < 1.77E-38). These data suggest that the abundance of Scaphonyx in bins 1 and 2, and its subsequent decline, are at least in part an actual paleoecological signal. As such, how do these data compare to predictions for rhynchosaur relative abundance changes in relation to paleoclimate?
Hypothesis 5: The rapid decline in Scaphonyx abundance is associated with the onset of more arid, slightly warmer, and less seasonal conditions (∼100 m level). This directly contradicts the predictions for this hypothesis, which suggests that rhynchosaur relative abundance should increase with more arid conditions. In fact, rhynchosaurs (Teyumbaita n. sp.) become slightly more abundant higher in the section (bins 9 and 10) with a pulse of more humid conditions. Therefore, our data appear to falsify this hypothesis, at least as it applies to the Ischigualasto Formation.
It could be that this hypothesis is simply wrong, and rhynchosaur relative abundance is unrelated to aridity. Alternatively, the original formulators of the hypothesis may have been caught-out by the subtleties of the paleoclimate record. This hypothesis was based on relative abundance data from the Middle-Upper Triassic fossil assemblage of the Paraná Basin, southern Brazil (Azevedo et al., 1990). Here, rhynchosaurs are most abundant in the Santa Maria and Caturrita formations (Azevedo et al., 1990), a sequence with an overall semi-arid inferred climate regime (see review in Mancuso et al., 2021). But, more recent paleoclimate studies indicate that the upper Santa Maria and lower Caturrita formations (lower Candelaria sequence) were relatively wetter than underlying and overlying strata (Horn et al., 2018a,b; Mancuso et al., 2021), and these are precisely the intervals with the most abundant rhynchosaurs (Azevedo et al., 1990: Figs. 5, 6). This is also consistent with the rarity/absence of rhynchosaurs in the more arid Upper Triassic deposits of western North America (Irmis, 2011; Whiteside et al., 2011, 2015). Thus, it may be that rhynchosaur abundance is instead correlated with more humid conditions.
5 Discussion
5.1 Geographic and Chronologic Scale of Climate Proxies
During the early Late Triassic (Carnian to early Norian), the IVUB was deposited between 44.6°S and 49.6°S paleolatitude, including 95% confidence intervals (230 Ma paleopole of van Hinsbergen et al., 2015; see Mancuso et al., 2021: Fig. 3). The most recent Carnian Global Circulation Model (GCM) predicts a MAT of 0.85°C and MAP of 1,161 mm/year for the IVUB, with warmest and coldest monthly means of 37.6°C to −35.9°C, and wettest and driest monthly means of 1,238 mm/year to 1,083 mm/year (Dunne et al., 2021).
Our new proxy data estimates of MAP from the Ischigualasto Formation match well with the GCM predictions (Dunne et al., 2021), with a range of values from 721–1,343 mm/year (mean of 959 mm, median of 969 mm, standard deviation of ±176 mm) that overlaps with the GCM estimate of 1,161 mm/year (Dunne et al., 2021). In contrast, the GCM MAT estimate of 0.85°C (Dunne et al., 2021) is considerably lower than our proxy estimates of 9.4–17.5°C (mean of 12.7°C, median of 12.6°C, standard deviation of ±3.6°C). This discrepancy in MAT estimates is stark but may have a relatively simple explanation. The Ischigualasto Formation climate values were extracted from the GCM results using an inferred paleolatitude of 52°S, as reconstructed using the plate kinematic model in the Paleobiology Database. However, if a paleolatitude of 44.6°S-49.6°S is used (van Hinsbergen et al., 2015), this results in MAT values between 6–14°C, much closer to our proxy estimates. Because the latitudinal gradient in this area of Gondwana is less severe for MAP, these lower paleolatitudes would result in values between 700–1,400 mm/year, still consistent with our proxy estimates. From a clay mineralogy standpoint, the high smectite/illite ratios in the upper part of the Ischigualasto Formation are consistent with the aforementioned high temperature seasonality predicted by GCM results (Dunne et al., 2021).
The Chañares-Los Rastros-Ischigualasto succession preserves a nearly continuous record of the Late Triassic from the early Carnian through early Norian (∼236.5–224.5 Ma). Recently published multi-proxy data (Mancuso et al., 2020a; Benavente et al., 2022) support a general trend from seasonal semi-arid to sub-humid conditions during the early Carnian (∼236.5–235 Ma, Chañares Formation) to humid and warm conditions during middle Carnian (∼235–231.3 Ma, Los Rastros Formation) (Figure 9). The warmer and wetter phase was proposed as possible evidence of the Carnian Pluvial Episode in Gondwana (Mancuso et al., 2020a; Benavente et al., 2022). The new multi-proxy data presented here for the Ischigualasto Formation supply evidence of a fluctuating climate trend for the late Carnian to early Norian (∼231.3–224.5 Ma) at the Cerro Bola locality (Figure 9). The first ∼100 m during the late Carnian (∼231–229.5 Ma) record seasonal warm, humid conditions with a short episode of sub-humid to semi-arid conditions (Figure 9). Subsequently, during the latest Carnian (∼229.5–227.8 Ma) between ∼100–380 m, the Ischigualasto Formation was deposited under seasonal sub-humid to semi-arid conditions, followed by more variable, humid, more seasonal conditions at the very end of the Carnian and during the earliest Norian (∼227.8–224.5 Ma) (Figure 9). The estimates of MAT and MAP for the Ischigualasto paleosols show a similar trend, with a mean around 950 mm/yr for MAP but with higher values in the lowermost and upper parts of the section, and a subtle change in MAT from 12.5 to 13.5°C, but lower than estimated for the Los Rastros (Mancuso et al., 2020a; Benavente et al., 2022). Our new multi-proxy data agrees well with paleosol data from the Ischigualasto Provincial Park (Tabor et al., 2004, 2006). These data from further southeast in the basin also suggested a complex pattern (see Supplementary Material Correlation through basin and Supplementary Figure S3 for more detailed information about the correlation between Ischigualasto Provincial Park and Cerro Bola area), beginning with a humid episode (the lower quarter of the unit), followed by relatively dry conditions (the middle half of the unit), finishing with more humid conditions again (the upper quarter) with cool temperatures between ∼0–10°C (estimating using δ18Ocarb values from pedogenic carbonate nodules). In contrast, paleobotanical and sedimentological data from this same area suggest generally seasonal arid to semi-arid conditions with a semi-humid episode in the middle of the unit (Colombi and Parrish, 2008; Césari and Colombi, 2013; Colombi et al., 2013).
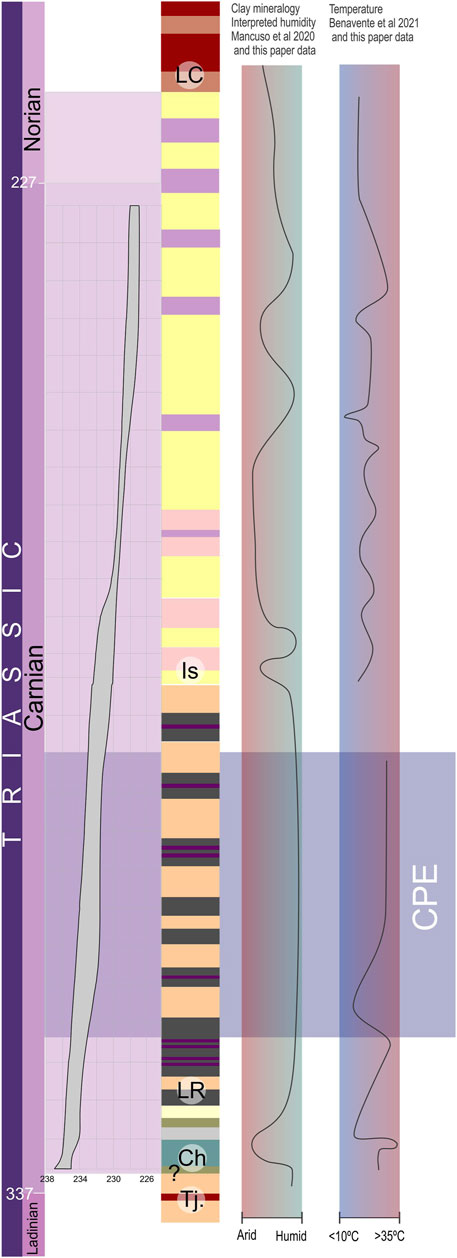
FIGURE 9. Summary of Agua de la Peña Group paleoclimate interpretations. Geochronologic age constraints for the Chañares, Los Rastros, and Ischigualasto formations modified from the preliminary age model with uncertainty envelope (gray shading) for the unit based on radioisotopic ages (Irmis et al., 2022). Ch, Chañares Formation; Is, Ischigualasto Formation; LC, Los Colorados Formation; LR, Los Rastros Formation; Tj, Tarjados Formation.
These differences likely reflect the spatial and temporal scale at which the proxies record paleoenvironment. The proxies for MAP and MAT are dependent upon local geochemical conditions and thus could easily vary across the basin. At the same time, they are somewhat time-averaged given how long it takes paleosols to develop. Sedimentological indicators can also be quite localized with significant lateral variation, and paleosol morphology (e.g., pedotype) is driven in part by changes in the local water table. Therefore, it is not surprising that studies using different proxies from different parts of the basin may observe variation in inferred paleoclimate conditions. From a palaeontological perspective, using fossils to interpret climate conditions is circular if one is then comparing that climate record with the fossil record. Regardless, the differences between the climate reconstruction from geochemical, clay mineralogy, and paleosol morphology data compared to the paleontological interpretations (macro and microflora, vertebrate) (Colombi and Parrish, 2008; Césari and Colombi, 2013; Colombi et al., 2013) can be explained due to the strong dependence of fossil preservation with local sedimentological conditions (see Supplementary Material). The floral record is essentially preserved under high water-table conditions (Agua de la Peña locality and around the site), independent of larger-scale changes in climate (Colombi and Parrish, 2008; Césari and Colombi, 2013). In addition, vertebrate fossil assemblages are mainly preserved on well-drained low water-table deposits (Colombi et al., 2013). Therefore, the paleontological record largely reflects local taphonomic and sedimentological conditions instead of basin-wide or regional climate.
Clay mineralogy data for the Chañares, Los Rastros, and Ischigualasto formations has also been documented for other parts of the IVUB (Bossi, 1970; Ovejero and Bossi, 1984; Tabor et al., 2006; Mancuso et al., 2020a). The mineralogical assemblages found at the Cerro Bola locality agree with previous Ischigualasto Formation mineralogical data from Agua de la Peña and Zanja de la Viuda localities (Bossi, 1970; Ovejero and Bossi, 1984; Tabor et al., 2006). These clay assemblages record varying proportions of smectite, illite, kaolinite, chlorite, and mixed-layer illite/smectite. Smectite is the dominant clay mineral, followed by kaolinite and illite, at Ischigualasto Provincial Park (Bossi, 1970; Tabor et al., 2006); in contrast, at the Cerro Bola and Zanja de la Viuda localities (Figure 1), illite is the dominant component followed by a similar contribution of kaolinite and smectite (Figure 2; see Figure 5 in Ovejero and Bossi, 1984). Nevertheless, within the Ischigualasto Formation, there are consistent assemblages across the basin that highlight the general pattern of an enhanced hydrolysis index due to increased leaching. Those intervals are characterized by a higher contribution of kaolinite and smectite and might record geologically brief episodes of discrete enhanced humidity (380–420, 465, 550, 580, and 680 m levels at Cerro Bola). Similarly, the mineralogical assemblages found at the Gualo locality and Cerro Bola for the Chañares and Los Rastros formations (Mancuso et al., 2020a) agree with the mineralogy previously documented at the Río Ischigualasto, Zanja de la Viuda localities (Bossi, 1970; Ovejero and Bossi, 1984). In general, illitization processes have been more pervasive in the Ischigualasto deposits than in the Chañares and Los Rastros assemblages (Mancuso et al., 2020a).
Interestingly, Bossi (1970) suggested that clay mineral assemblages are relatively consistent within their depositional subenvironments. For example, illite dominates lateral accretion fluvial elements and kaolinite and to a less extent smectite are predominant in fluvial lateral suspension and vertical accretion deposits. These are the natural authigenic subenvironments for those clay minerals and support the primary composition of the mineralogical assemblages that are most likely reflecting a paleoclimate signal. In our analysis, we have found that the lake center deposits (Los Rastros Formation - Bossi´s vertical accretion deposits) are dominated by kaolinite and less common smectite; and the fluvial floodplains with occasional ponds (Ischigualasto Formation - Bossi´s fluvial lateral suspension) are dominated by variable proportions of kaolinite-illite- smectite-illite/smectite.
Analyzing the mineralogical proxies (kaolinite/illite, smectite/illite, and smectite/kaolinite ratios), the general trend of highly dispersed values in lower and upper levels of the Ischigualasto is also recorded in the sections from Agua de la Peña and Zanja de la Viuda (Bossi, 1970; Ovejero and Bossi, 1984). Therefore, the clay mineralogy proxy evidence suggests basin-wide seasonal semi-arid to sub-humid paleoclimate trend during deposition of the early Carnian Chañares Formation, humid and warm conditions for the middle Carnian Los Rastros Formation, followed by variable seasonal less humid conditions for the late Carnian-early Norian Ischigualasto Formation. This consistency across the basin thus provides some confidence in using our results to compare with the basin-wide fossil record. Unfortunately, other proxies included in this contribution can not be compared directly with other localities of the IVUB; however, as mentioned previously, the general climate trend we observed compares well with paleosol data from the Ischigualasto Provincial Park (Tabor et al., 2004, 2006).
5.2 Paleobotanical Record and Links With Climatic Proxies
Although limitations in sampling mean that we cannot compare the paleobotanical and climate record using our hypothesis testing framework, the plant fossil record suggests that changes in vegetation accompanied broad changes in climatic conditions across the formations. In the Los Rastro Formation, vegetation was stable both in time and space (Pedernera et al., 2020). This stability could be related to the more humid and stable climatic conditions proposed for the unit (Mancuso et al., 2020a). On the other hand, the lower number of plant macrofossil taxa recorded in the Ischigualasto Formation (Figure 4), and lower number of taxa per taxonomic group (see Supplementary Material) could be related to the high variability of the MAP and MAT, though we caution that a more direct comparison would require sample standardization techniques (e.g., rarefaction or quorum sub-sampling). These plant fossils come from specific fossiliferous levels restricted temporally and spatially (Colombi and Parrish, 2008; Drovandi et al., 2021). Indeed, the taphofacies described by Colombi and Parrish (2008) are both temporally and spatially restricted; these authors indicate that the observed patterns are due to the fluvial architecture and paleosols related to the evolution of the climate during the deposition of the Ischigualasto Formation. An extensive systematic sampling of the unit to reduce sampling gaps could help clarify changes in the plant fossil record allowing one to more directly test the hypothesis predictions outlined in Section 1.3.
The palynomorph assemblages of the Chañares and Ischigualasto formations have been considered part of a ‘transitional zone’ between the Ipswich and the Onslow subprovinces (Césari and Colombi, 2013; Perez Lonaize et al., 2018). In contrast, the Los Rastros microfloral assemblage is typical of the Ipswich sub-province (Ottone et al., 2005; Ottone and Mancuso, 2006; Césari and Colombi, 2016). Traditionally, the Onslow microflora has been used as an indicator of hot and humid climatic conditions, whereas the Ipswich microflora has been considered an indicator of relatively cooler temperature zones (Dolby and Blame, 1976). However, the microfloristic assemblages described in the formations of the Agua de la Peña Group do not agree with MAT and MAP estimates proposed for the same units. The Chañares Formation has been interpreted as seasonal semi-arid to sub-humid (Mancuso et al., 2020a), the Los Rastros Formation humid and warm (Mancuso et al., 2020a), and the Ischigualasto Formation data indicate variable semi-arid to humid variable conditions for the unit (Figure 3). As such, one might predict that the Chañares and Ischigualasto microfloral assemblages would be most similar to the Ipswich microflora, whereas the microflora of the Los Rastros Formation would have a greater presence of Onslow characteristic elements; this is the opposite of what is preserved. Therefore, the differences in the palynological assemblages among the Chañares, Los Rastros, and Ischigualasto formations may not be associated with climatic variations and these differences could be linked with the intrabasin spatial distribution of habitats for plant microspores producers. It is very possible that the Los Rastros Formation was also located in this microfloral province transition zone (cf. Césari and Colombi, 2013), and the more humid conditions during the CPE restricted typical Onslow miospore plant producers to distal upland areas that are not preserved in this unit. These complexities reinforce the need for independent paleoclimate proxies (such as those in this contribution) for properly interpreting possible climate influence on floras in deep time.
Ultimately, systematic sampling for palynomorphs and plant macrofossils throughout this sequence, particularly the Ischigualasto Formation, is necessary to develop a well-sampled record at many different stratigraphic levels. Only with this improved high-resolution record will there be enough data points to properly test proposed hypotheses linking plant diversity and relative abundance with climate (Pardoe et al., 2021).
5.3 Tetrapod Record and Links With Climatic Proxies
Assessing changes in tetrapod composition in relation to paleoclimate across the different formations of the Agua de la Peña Group is very difficult given that there are large stratigraphic gaps between samples (e.g., upper Los Rastros and lower Los Colorados formations), and different styles of preservation that are not directly comparable (i.e., predominantly ichnofossils from the Los Rastros compared to body fossils from all other formations). Indeed, whether one examines changes in clade species richness (Figure 6) or inferred habitat preference (Figure 7), there are no changes that seem clearly related to climate differences between the formations. This highlights the challenges of the traditional pattern-matching approach; namely, that other factors (e.g., preservation, sampling) can mask any original direct correlation with climate variables. In any case, it is not necessarily clear that one should expect such simple correlations, given that it does not reflect known evolutionary models (e.g., Faith et al., 2021).
The synapsid fossil record from the IVUB exemplifies these difficulties. The decrease in dicynodont diversity from the Chañares to Ischigualasto Formation, and their absence in the upper levels of the Norian Los Colorados Formation, follows global trends, so it seems imprudent to try to ascribe it to regional/basin-scale climate change. In contrast, cynodonts, in particular probainognathians, proliferated on many continents during the Carnian and into the Norian and Rhaetian, even in relatively nearby regions (see Abdala et al., 2020 for a review). Therefore, their absence above bin 2 in the Ischigualasto Formation (with the exception of Exaeretodon) does seem to be a more local pattern. One might hypothesize this change reflects the more arid seasonal conditions above the 100 m level, but it also could simply be a result of declining sample size not picking up rare taxa that are only known from a handful of specimens. As another example, higher in section, in the uppermost portion of the Ischigualasto Formation, fossils in Unit IV are scarce (Figure 8, Supplementary Figures S6, S7). They are only represented by 1 specimen (a single bone) of the dicynodont Jachaleria and a few isolated indeterminate archosaur elements (Martínez et al., 2011). In the lowermost Los Colorados Formation, vertebrate representation is also poor, with only 8 specimens of Jachaleria reported (Martínez et al., 2011), although recently a bonebed including a minimum of 200 bones (mostly Jachaleria) has been reported (Colombi et al., 2018). The top of the Ischigualasto Formation and the lower levels of the Los Colorados Formation appear to represent an interval in which fossils are scarce. Martínez et al. (2011) proposed that this extremely low abundance of vertebrates cannot be explained by poor sampling or poorly exposed outcrops, and suggested that this situation could be correlated with more arid conditions and better explained by a true absence of fauna or by a taphonomic bias (Martínez et al., 2011). In contrast, our results regarding the interpretation of aridity point to the opposite (Figure 8), with a change to more humid (bin 13) conditions near the top of the Ischigualasto Formation. So does the low diversity and abundance of tetrapods at this boundary interval reflect actual rarity on the paleolandscape, preservational/sampling bias, or a combination of the two? Examination of the more detailed tetrapod record of the Ischigualasto Formation is equally ambiguous to interpret from a pattern-matching perspective, with few obvious changes in species richness, relative abundance, or stratigraphic ranges (Figure 8; Supplementary Figure S13) coinciding with the main paleoclimate changes inferred from the proxy datasets in this study.
For this reason, building on recent work from other authors (Faith et al., 2021), we instead proposed an approach for evaluating explicit predictions from existing hypotheses using known evolutionary models for vertebrate response to climate change in deep time. The advantage of this approach is that it makes specific a priori predictions based on what we know about evolution that can then be tested using the fossil record, rather than a posteriori trying to match changes in paleoclimate proxies with those from fossil assemblages. Nonetheless, our study demonstrates how difficult this can be even with a rich climate proxy record and abundant fossil dataset. This Ischigualasto case study highlighted how sampling and taphonomy can strongly control patterns in the fossil record even when many specimens are known from many different stratigraphic levels. As a result we were unable to fully test the predictions for each hypothesis, particularly when it came to evaluating pulses of first occurrences. Though we observed a few subtle changes that do not appear to be tied to sampling, one might query why there is not a stronger correlation with paleoclimatic changes. One or more processes may be at play. First, it might be that the magnitude and/or pace of climate change observed simply was not great enough to force major changes in ecosystem structure. Alternatively, despite our abundant fossil record, it might be that other changes are subtle enough that they require even greater stratigraphic resolution (e.g., meter scale as opposed to 50 m bins) with even denser specimen sampling.
Even so, there are definitely reasons for optimism. First, we were able to find partial support for one hypothesis, with a pulse of pseudosuchian archosaur LAs associated with a shift to more humid conditions, which was consistent with the predictions for Hypothesis 4. Second, we were able to provide fairly strong evidence to help falsify Hypothesis 5, where we observed the opposite trend in rhynchosaur relative abundance (compared to the climate trend) than predicted. In this case, we can formulate a new hypothesis; namely, that rhynchosaur abundance increased during more humid intervals. Notably, this tetrapod clade is perhaps the best sampled in the formation in terms of number of specimens, exemplifying the predictive power of large sample sizes. And even where we were unable to find evidence for/against hypotheses, lacked the sampling to make evaluations, or found a strong sampling control on diversity and abundance patterns, it highlights where more work should be concentrated in the basin to build datasets that are sufficient for hypothesis testing. Finally, and perhaps most importantly, regardless of specific results for the Ischigualasto Formation or IVUB, our study is an example of how one can use this predictive framework in future studies to evaluate biotic response to climate change in deep time.
6 Conclusion
Our new multiproxy dataset for the Ischigualasto Formation provides insights into key changes in paleoenvironment and paleoclimate during the late Carnian and early Norian. Combined with previous work in other parts of the basin and other formations, we are able to identify and elucidate basin-scale trends in paleoclimate, and compare them to the extensive floral and vertebrate fossil records. Although sampling is a primary control of patterns observed in these fossil assemblages, we observe a small number of changes in species richness and relative abundance that are consistent with predictions from evolutionary models for climate forcing of biotic change. Moreover, our approach to testing for associations highlights data gaps that must be filled by additional sampling, and makes new testable predictions that can be tested by future work.
Data Availability Statement
The original contributions presented in the study are included in the article/Supplementary Material, further inquiries can be directed to the corresponding author.
Author Contributions
ACM and RBI designed the project. ACM and RBI conducted geological sections and palaeoenvironmental interpretation. BTB conducted XRF analyses. ACM and CAB conducted climate studies. ACM, RBI, TEP, and LCG conducted paleontological studies. ACM, RBI, and TEP designed and prepared the figures. RBI and TEP conducted the statistical analysis. ACM, RBI, TEP, and LCG wrote the Supplementary Material. ACM and RBI wrote the manuscript with input from all authors.
Funding
Field and laboratory research were supported by the PIP CONICET 11420090100209/10 and PICT 2013-0805 (ACM). Additional financial support was provided by the Consejo Nacional de Investigaciones Científicas y Técnicas (CONICET), and University of Utah.
Conflict of Interest
The authors declare that the research was conducted in the absence of any commercial or financial relationships that could be construed as a potential conflict of interest.
Publisher’s Note
All claims expressed in this article are solely those of the authors and do not necessarily represent those of their affiliated organizations, or those of the publisher, the editors and the reviewers. Any product that may be evaluated in this article, or claim that may be made by its manufacturer, is not guaranteed or endorsed by the publisher.
Acknowledgments
We thank E. Previtera, M. Bourguet, and C. Sancho (IANIGLA-CONICET) for their support during fieldwork. For access permission, we thank Carlos Ariel Ormeño and Ana Mercado Luna (Dirección de Patrimonio Arqueológico y Paleontológico, Secretaría de Cultura de La Rioja) and Administración de Parques Nacionales. We are deeply indebted to the staff of Parque Nacional Talampaya for their constant assistance in the field. Andrea Brunelle, Brenda Bowen, and Isaac Hart (University of Utah) kindly provided XRF access. We thank Tyler Faith for discussions on testing the relationship between paleoclimate and the fossil record, and for assistance with calculating adjusted residuals. Emma Dunne generously shared the individual GCM paleoclimate values for the Ischigualasto Formation from her 2021 study. This is LCG´s R-419 contribution to the IDEAN. We thank the editor and two referees for comments that improved the manuscript.
Supplementary Material
The Supplementary Material for this article can be found online at: https://www.frontiersin.org/articles/10.3389/feart.2022.883788/full#supplementary-material
References
Abarzúa, F. (2016). Estratigrafía, análisis de cuenca y aspectos exploratorios en el extremo norte de Cuenca Cuyana, in Precordillera Occidental y Valle de Calingasta. PhD dissertation. Facultad de Ciencias Exactas, Físicas y Naturales (Universidad Nacional de San Juan), 188.
Abdala, F., Gaetano, L. C., Martinelli, A. G., Soares, M. B., Hancox, P. J., and Rubidge, B. S. (2020). Non-mammaliaform Cynodonts from Western Gondwana and the Significance of Argentinean Forms in Enhancing Understanding of the Group. J. S. Am. Earth Sci. 104, 1028841–1028932. doi:10.1016/j.jsames.2020.102884
Abu Hamad, A., Kerp, H., Vörding, B., and Bandel, K. (2008). A Late Permian Flora with Dicroidium from the Dead Sea Region, Jordan. Rev. Palaeobot. Palynology 149, 85–130. doi:10.1016/j.revpalbo.2007.10.006
Anderson, J. M., and Anderson, H. M. (1983). Palaeoflora of Southern Africa: Molteno Formation (Triassic) 1, Introduction. Rotterdam: Dicroidium. A.A. Balkema.
Anderson, J. M., and Anderson, H. M. (1985). Palaeoflora of Southern Africa: Prodromus of South African Megafloras. Rotterdam: Devonian to Lower Cretaceous. Balkema.
Anderson, J. M., and Anderson, H. M. (1989). Palaeoflora of Southern Africa: Molteno Formation (Triassic). 2. Gymnosperms (Excluding Dicroidium). Rotterdam: A.A. Balkema.
Arcucci, A. B., Marsicano, C. A., and Caselli, A. T. (2004). Tetrapod Association and Paleoenvironment of Los Colorados Formation (Argentina): A Significant Sample from Western Gondwana at the End of the Triassic. Geobios 37, 555–568. doi:10.1016/j.geobios.2003.04.008
Artabe, A. E., Morel, E. M., and Spalletti, L. A. (2001). “Paleoecología de las floras Triásicas Argentinas,” in El Sistema Triásico en la Argentina. Editors A. E. Artabe, E. M. Morel, and A. B. Zamuner (La Plata: Fundación Museo de La Plata “Francisco Pascasio Moreno), 199–225.
Artabe, E. A., Morel, E. M., and Spalletti, L. A. (2003). Caracterización de las provincias fitogeográficas Triásicas del Gondwana extratropical. Ameghiniana 40 (3), 1–19.
Azevedo, S. A., Schultz, C. L., and Barberena, M. C. (1990). Novas evidéncias bioestratigráficas e paleoecológicas na análise da evolução explosiva dos rincossauros do Triássico. Paula-Coutiana 4, 23–33.
Balme, B. E., and Helby, R. J. (1973). “Floral Modifications at the Permian-Triassic Boundary in Australia,” in Permian Triassic Systems and Their Mutual Boundary. Editor V. I. Ustritsky (CSPG Special Publications, Memoir), 2, 433–444.
Barboni, R., and Dutra, T. L. (2015). First Record of Ginkgo-Related Fertile Organs (Hamshawvia, Stachyopitys) and Leaves (Baiera, Sphenobaiera) in the Triassic of Brazil, Santa Maria Formation. J. S. Am. Earth Sci. 63, 417–435. doi:10.1016/j.jsames.2015.08.001
Barnosky, A. D. (2001). Distinguishing the Effects of the Red Queen and Court Jester on Miocene Mammal Evolution in the Northern Rocky Mountains. J. Vertebrate Paleontology 21, 172–185. doi:10.1671/0272-4634(2001)021[0172:dteotr]2.0.co;2
Barrenechea, J. F., López-Gómez, J., and De La Horra, R. (2018). Sedimentology, Clay Mineralogy and Palaeosols of the Mid-carnian Pluvial Episode in Eastern Spain: Insights into Humidity and Sea-Level Variations. J. Geol. Soc. 175, 993–1003. doi:10.1144/jgs2018-024
Behrensmeyer, A. K., and Chapman, R. E. (1993). Models and Simulations of Time-Averaging in Terrestrial Vertebrate Accumulations. Short. Courses Paleontol. 6, 125–149. doi:10.1017/s2475263000001082
Behrensmeyer, A. K., Hook, R. W., Badgley, C. E., Boy, J. A., Chapman, R. E., Dodson, P., et al. (1992). “Paleoenvironmental Contexts and Taphonomic Modes,” in Terrestrial Ecosystems through Time: Evolutionary Paleoecology of Terrestrial Plants and Animals. Editors A. K. Behrensmeyer, J. D. Damuth, W. A. Dimichele, R. Potts, H.-D. Sues, and S. L. Wing (Chicago: University of Chicago Press), 15–136.
Benavente, C. A., Mancuso, A. C., and Bohacs, K. M. (2019). Paleohydrogeologic Reconstruction of Triassic Carbonate Paleolakes from Stable Isotopes: Encompassing Two Lacustrine Models. J. S. Am. Earth Sci. 95, 102292. doi:10.1016/j.jsames.2019.102292
Benavente, C. A., Mancuso, A. C., Irmis, R. B., Bohacs, K. M., and Matheos, S. (2022). Tectonically Conditioned Record of Continental Interior Paleoclimate during the Carnian Pluvial Episode: The Upper Triassic Los Rastros Formation, Argentina. Geol. Soc. Am. Bull. 134 (1-2), 60–80. doi:10.1130/B35847.1
Bennett, J. M. (2018). GlobTherm, a Global Database on Thermal Tolerances for Aquatic and Terrestrial Organisms. Sci. Data 5 (1), 180022. doi:10.1038/sdata.2018.22
Benton, M. J., Bernardi, M., and Kinsella, C. (2018). The Carnian Pluvial Episode and the Origin of Dinosaurs. J. Geol. Soc. 175, 1019–1026. doi:10.1144/jgs2018-049
Benton, R. B. J., Butler, R., Close, R. A., Saupe, E., and Rabosky, D. L. (2021). Biodiversity across Space and Time in the Fossil Record. Curr. Biol. 31, R1225–R1236. doi:10.1016/j.cub.2021.07.071
Bernardi, M., Gianolla, P., Petti, F. M., Mietto, P., and Benton, M. J. (2018). Dinosaur Diversification Linked with the Carnian Pluvial Episode. Nat. Commun. 9, 1–10. doi:10.1038/s41467-018-03996-1
Berry, J. A., and Raison, J. K. (1981). “Responses of Macrophytes to Temperature,” in Physiological Plant Ecology I. Editors O. L. Lange, P. S. Nobel, C. B. Osmond, and H. Ziegler (Berlin, Heidelberg: Springer), 277–338. doi:10.1007/978-3-642-68090-8_11
Bobe, R., Behrensmeyer, A. K., and Chapman, R. E. (2002). Faunal Change, Environmental Variability and Late Pliocene Hominin Evolution. J. Hum. Evol. 42, 475–497. doi:10.1006/jhev.2001.0535
Bodnar, J., Coturel, E. P., Falco, J. I., and Beltrán, M. (2021). An Updated Scenario for the End-Permian Crisis and the Recovery of Triassic Land Flora in Argentina. Hist. Biol. 33 (12), 3654–3672. doi:10.1080/08912963.2021.1884245
Bodnar, J., Drovandi, J. M., Morel, E. M., and Ganuza, D. G. (2018). Middle Triassic Dipterid Ferns from West-Central Argentina and Their Relationship to Palaeoclimatic Changes. Acta Palaeontol. Pol. 63 (2), 397–416. doi:10.4202/app.00459.2018
Bomfleur, B., and Kerp, H. (2010). Dicroidium Diversity in the Upper Triassic of North Victoria Land, East Antarctica. Rev. Palaeobot. Palynology 160 (3-4), 67–101. doi:10.1016/j.revpalbo.2010.02.006
Bomfleur, B., Decombeix, A. L., Escapa, I. H., Schwendemann, A. B., and Axsmith, B. (2013). Whole–plant Concept and Environment Reconstruction of a Telemachus Conifer (Voltziales) from the Triassic of Antarctica. Int. J. Plant Sci. 174 (3), 425–444. doi:10.1086/668686
Bomfleur, B., Decombeix, A. L., Schwendemann, A. B., Escapa, I. H., Taylor, E. L., Taylor, T. N., et al. (2014). Habit and Ecology of the Petriellales, an Unusual Group of Seed Plants from the Triassic of Gondwana. Int. J. Plant Sci. 175 (9), 1062–1075. doi:10.1086/678087
Bordy, E. M., Abrahams, M., Sharman, G. R., Viglietti, P. A., Benson, R. B. J., McPhee, B. W., et al. (2020). A Chronostratigraphic Framework for the Upper Stormberg Group: Implications for the Triassic-Jurassic Boundary in Southern Africa. Earth-Science Rev. 203, 1031201–1031224. doi:10.1016/j.earscirev.2020.103120
Bossi, G. E. (1970). Asociaciones mineralógicas de las arcillas en la cuenca de Ischigualasto – Ischichuca. Parte II: Perfiles de la Hoyada de Ischigualasto. Acta Geol. Lilloana 11 (4), 73–100.
Bossi, G. E. (1971). Análisis de la Cuenca de Ischigualasto-Ischichuca. I Congreso Hispano-Luso-Americano de Geologia Economica. Madrid: Ibérica, 611–626.
Boucot, A. J., Xu, C., Scotese, C. R., and Morley, R. J. (2013). Phanerozoic Climate: an Atlas of Lithologic Indicators of Climate. SEPM Concepts Sedimentol. Paleontol. 11.
Caselli, A. T. (1998). Estratigrafía y sedimentología de las formaciones Patquía (Pérmico) y Talampaya (Triásico inferior) en las Sierras Pampeanas Noroccidentales y Precordillera Central (provincias de La Rioja y San Juan). Buenos Aires, Argentina: Universidad de Buenos Aires, 437.
Caselli, A. T., Marsicano, C. A., and Arcucci, A. B. (2001). Sedimentología y paleontología de la Formación Los Colorados, Triásico Superior (provincias de La Rioja y San Juan, Argentina). Rev. Asoc. Geol. Argent. 56, 173–188.
Césari, S. N., and Colombi, C. E. (2013). A New Late Triassic Phytogeographical Scenario in Westernmost Gondwana. Nat. Commun. 4, 18891–18896.
Césari, S. N., and Colombi, C. E. (2016). Palynology of the Late Triassic Ischigualasto Formation, Argentina: Paleoecological and Paleogeographic Implications. Palaeogeogr. Palaeoclimatol. Palaeoecol. 449, 365–384.
Chandra, S., Singh, K. J., and Jha, N. (2008). First Report of the Fertile Plant Genus Umkomasia from Late Permian Beds in India and its Biostratigraphic Significance. Palaeontology 51, 817–826. doi:10.1111/j.1475-4983.2008.00767.x
Channing, A., Zamuner, A., Edwards, D., and Guido, D. (2011). Equisetum thermale Sp. Nov. (Equisetales) from the Jurassic San Agustín Hot Spring Deposit, Patagonia: Anatomy, Paleoecology, and Inferred Paleoecophysiology. Am. J. Bot. 98 (4), 680–697. doi:10.3732/ajb.1000211
Cirilli, S. (2010). Upper Triassic-Lowermost Jurassic Palynology and Palynostratigraphy: A Review. Geological Society of London Special Publication 334, 285–314. doi:10.1144/sp334.12
Cladera, G., Rougier, G., de la Fuente, M., and Arcucci, A. B. (1998). Niveles fosilíferos del sector superior de la Formación Los Colorados (Triásico Tardio) en las proximidades de «La Esquina», Provincia de La Rioja, Argentina. Acta Geol. Lilloana 18, 159.
Clift, P. D., Wan, S., and Blusztajn, J. (2014). Reconstructing Chemical Weathering, Physical Erosion and Monsoon Intensity since 25 Ma in the Northern South China Sea: A Review of Competing Proxies. Earth-Science Rev. 10, 86–102. doi:10.1016/j.earscirev.2014.01.002
Colombi, C. E., and Parrish, J. T. (2008). Late Triassic Environmental Evolution in Southwestern Pangea: Plant Taphonomy of the Ischigualasto Formation. Palaios 23, 778–795. doi:10.2110/palo.2007.p07-101r
Colombi, C. E., Montañez, I. P., and Parrish, J. T. (2011). Registro de la relación isotópica de carbono en la paleoflora de la Formación Ischigualasto (Triásico Superior), noroeste argentino: implicaciones paleoatmosféricas. Rev. Bras. Paleontol. 14, 39–50. doi:10.4072/rbp.2011.1.04
Colombi, C. E., Rogers, R. R., and Alcober, O. A. (2013). Vertebrate Taphonomy of the Ischigualasto Formation. Society of Vertebrate Paleontology Memoir 12, 31–50. doi:10.1080/02724634.2013.809285
Colombi, C. E., Limarino, C. O., and Alcober, O. A. (2017). Allogenic Controls on the Fluvial Architecture and Fossil Preservation of the Upper Triassic Ischigualasto Formation, NW Argentina. Sediment. Geol. 362, 1–16. doi:10.1016/j.sedgeo.2017.10.003
Colombi, C. E., Martínez, R. N., Césari, S. N., Alcober, O. A., Limarino, C. O., and Montañez, I. P. (2021). A High-Precision U–Pb Zircon Age Constraints the Timing of the Faunistic and Palynofloristic Events of the Carnian Ischigualasto Formation, San Juan, Argentina. J. S. Am. Earth Sci. 111, 103433. doi:10.1016/j.jsames.2021.103433
Colombi, C., Martínez, R., Santi Malnis, P., Apaldetti, C., Yañez, I., Abelín, D., et al. (2018). Bonebed en las facies basales de la Formación Los Colorados (Noriano), Cuenca de Ischigualasto-Villa Unión, San Juan, Argentina. Actas Reunión de Comunicaciones de la Asociación Paleontológica Argentina, 54.
Coturel, E. P., Bodnar, J., Morel, E. M., Ganuza, D., Sagasti, A. J., and Beltrán, M. (2018). New Species of Osmundaceous Fertile Leaves from the Upper Triassic of Argentina. Acta Palaeobot. 58 (2), 107–119.
Currie, B. S., Colombi, C. E., Tabor, N. J., Shipman, T. C., and Montañez, I. P. (2009). Stratigraphy and Architecture of the Upper Triassic Ischigualasto Formation, Ischigualasto Provincial Park, San Juan, Argentina. J. S. Am. Earth Sci. 27, 74–87. doi:10.1016/j.jsames.2008.10.004
D'Angelo, J. A. (2019). Molecular Structure of the Cuticles of Dicroidium and Johnstonia (Corystospermaceae, Triassic, Argentina). Ecophysiological Adaptations of Two Chemically Indistinguishable, Morphology-Based Taxa. Rev. Palaeobot. Palynology 268, 109–124. doi:10.1016/j.revpalbo.2019.05.007
Dal Corso, J., Ruffell, A., and Preto, N. (2018). The Carnian Pluvial Episode (Late Triassic): New Insights into This Important Time of Global Environmental and Biological Change. J. Geol. Soc. 175, 986–988. doi:10.1144/jgs2018-185
Dal Corso, J., Bernardi, M., Sun, Y., Song, H., Seyfullah, L. J., Preto, N., et al. (2020). Extinction and Dawn of the Modern World in the Carnian (Late Triassic). Sci. Adv. 6, 1–12, eaba0099. doi:10.1126/sciadv.aba0099
Dal Corso, J., Gianolla, P., Newton, R. J., Franceschi, M., Roghi, G., Caggiati, M., et al. (2015). Carbon Isotope Records Reveal Synchronicity between Carbon Cycle Perturbation and the “Carnian Pluvial Event” in the Tethys Realm (Late Triassic). Glob. Planet. Change 127, 79–90. doi:10.1016/j.gloplacha.2015.01.013
Dal Corso, J., Mietto, P., Newton, R. J., Pancost, R. D., Preto, N., Roghi, G., et al. (2012). Discovery of a Major Negative δ13C Spike in the Carnian (Late Triassic) Linked to the Eruption of Wrangellia Flood Basalts. Geology 40, 79–82. doi:10.1130/g32473.1
Di Michele, W. A. (2013). Wetland-dryland Vegetational Dynamics in the Pennsylvanian Ice Age Tropics. Int. J. Plant Sci. 175 (2), 123–164.
Do Campo, M., del Papa, C., Nieto, F., Hongn, F., and Petrinovic, I. (2010). Integrated Analysis for Constraining Palaeoclimatic and Volcanic Influences on Clay–Mineral Assemblages in Orogenic Basins (Palaeogene Andean Foreland, Northwestern Argentina). Sediment. Geol. 228, 98–112. doi:10.1016/j.sedgeo.2010.04.002
Dolby, J. H., and Balme, B. E. (1976). Triassic Palynology of the Carnarvon Basin, Western Australia. Rev. Palaeobot. Palynology 22, 105–168. doi:10.1016/0034-6667(76)90053-1
Doubinger, J., Vetter, P., Langiaux, J., Galtier, J., and Broutin, J. (1995). La flore fossile du bassin houiller de Saint-Étienne. Muséum National d'Histoire Naturelle, Paris 164.
Drovandi, J. M., Correa, G. A., Colombi, C. E., and Césari, S. N. (2021). Dicroidium (Zuberia) zuberi (Szajnocha) Archangelsky from Exceptional Carnian Leaf Litters of the Ischigualasto Formation, Westernmost Gondwana. Hist. Biol., 1–14. doi:10.1080/08912963.2021.1974017
Dunne, E. M., Farnsworth, A., Greene, S. E., Lunt, D. J., and Butler, R. J. (2021). Climatic Drivers of Latitudinal Variation in Late Triassic Tetrapod Diversity. Palaeontology 64, 101–117. doi:10.1111/pala.12514
Ezcurra, M. D. (2010). Biogeography of Triassic Tetrapods: Evidence for Provincialism and Driven Sympatric Cladogenesis in the Early Evolution of Modern Tetrapod Lineages. Proc. R. Soc. Lond. Biol. Sci. 277, 2547–2552. doi:10.1098/rspb.2010.0508
Faith, J. T., and Lyman, R. L. (2019). Paleozoology and Paleoenvironments: Fundamentals, Assumptions, Techniques. Cambridge: Cambridge University Press.
Faith, J. T., Du, A., Behrensmeyer, A. K., Davies, B., Patterson, D. B., Rowan, J., et al. (2021). Rethinking the Ecological Drivers of Hominin Evolution. Trends Ecol. Evol. 36, 797–807. doi:10.1016/j.tree.2021.04.011
Falcon-Lang, H. J., Nelson, W. J., Elrick, S., Looy, C. V., Ames, P. R., and Dimichele, W. A. (2009). Incised Channel Fills Containing Conifers Indicate that Seasonally Dry Vegetation Dominated Pennsylvanian Tropical Lowlands. Geology 37, 923–926. doi:10.1130/g30117a.1
Fielding, C. R., Frank, T. D., McLoughlin, S., Vajda, V., Mays, C., Tevyaw, A. P., et al. (2019). Age and Pattern of the Southern High-Latitude Continental End- Permian Extinction Constrained by Multiproxy Analysis. Nat. Commun. 10, 1–12. doi:10.1038/s41467-018-07934-z
Foster, C. B., Balme, B. E., and Helby, R. (1994). First Record of Tethyan Palynomorphs from the Late Triassic of East Antarctica. J. Aust. Geol. Geophys. 15, 239–246.
Fraser, N. C., and Sues, H.-D. (2011). The Beginning of the ‘Age of Dinosaurs': A Brief Overview of Terrestrial Biotic Changes during the Triassic. Earth Environ. Sci. Trans. R. Soc. Edinb. 101, 189–200. doi:10.1017/s1755691011020019
Freguelli, J. (1944). La serie del llamado “Rético” en el oeste Argentino (Nota preliminar). Notas del Museo de La Plata. Geología 30, 261–279.
Freguelli, J. (1948). Estratigrafía y edad del llamado “Rético” en la Argentina. An. Soc. Argent. Estud. Geográficos, GAEA 8, 159–309.
Fürsich, F. T., Singh, I. B., Joachimski, M., Krumm, S., Schlirf, M., and Schlirf, S. (2005). Palaeoclimate Reconstructions of the Middle Jurassic of Kachchh (Western India): an Integrated Approach Based on Palaeoecological, Oxygen Isotopic, and Clay Mineralogical Data. Palaeogeogr. Palaeoclimatol. Palaeoecol. 217, 289–309.
Gaillardet, J., Dupre, B., and Allegre, C. J. (1999). Geochemistry of Large River Suspended Sediments: Silicate Weathering or Recycling Tracer? Geochim. Cosmochim. Acta 63, 4037–4051. doi:10.1016/s0016-7037(99)00307-5
Gallagher, T. M., and Sheldon, N. D. (2013). A New Paleothermometer for Forest Paleosols and its Implications for Cenozoic Climate. Geology 41, 647–650. doi:10.1130/g34074.1
Gallet, S., Jahn, B., and Torii, M. (1996). Geochemical Characterization of the Luochuan Loess-Paleosol Sequence, China, and Paleoclimatic Implications. Chem. Geol. 133, 67–88. doi:10.1016/s0009-2541(96)00070-8
Garzanti, E., Padoan, M., Setti, M., Lopez-Galindo, A., and Villa, I. M. (2014). Provenance versus Weathering Control on the Composition of Tropical River Mud (Southern Africa). Chem. Geol. 366, 61–74. doi:10.1016/j.chemgeo.2013.12.016
Giles, S., Xu, G.-H., Near, T. J., and Friedman, M. (2017). Early Members of 'living Fossil' Lineage Imply Later Origin of Modern Ray-Finned Fishes. Nature 549, 265–268. doi:10.1038/nature23654
Goddéris, Y., Donnadieu, Y., de Vargas, C., Pierrehumbert, R. T., Dromart, G., and van de Schootbrugge, B. (2008). Causal or Casual Link between the Rise of Nannoplankton Calcification and a Tectonically-Driven Massive Decrease in Late Triassic Atmospheric CO2? Earth Planet. Sci. Lett. 267, 247–255.
Goldberg, K., and Humayun, M. (2010). The Applicability of the Chemical Index of Alteration as Paleoclimatic Indicator: An Example from the Permian of the Parana Basin, Brazil. Palaeogeogr. Palaeoclimatol. Palaeoecol. 293, 175–183. doi:10.1016/j.palaeo.2010.05.015
Grayson, D. K., and Delpech, F. (2003). Ungulates and the Middle-To-Upper Paleolithic Transition at Grotte XVI (Dordogne, France). J. Archaeol. Sci. 30, 1633–1648. doi:10.1016/s0305-4403(03)00064-5
Hammer, Ø., Harper, D. a. T., and Ryan, P. D. (2001). PAST: Paleontological Statistics Software Package for Education and Data Analysis. Palaeontol. Electron. 4 (1), 1–9.
Harris, R., McCall, R., Randall, O., Tawang, M. H. B., Williams, R., Fairman, J. G., et al. (2017). Climate Change during the Triassic and Jurassic. Geol. Today 33, 210–215. doi:10.1111/gto.12210
He, S.-A., Yin, G., and Pang, Z.-J. (1997). “Resources and Prospects of Ginkgo biloba in China,” in Ginkgo Biloba a Global Treasure. Editor T. Hori (Tokyo): Springer), 373–383.
Hochuli, P. A., Sanson-Barrera, A., Schneebeli-Hermann, E., and Bucher, H. (2016). Severest Crisis Overlooked-Worst Disruption of Terrestrial Environments Postdates the Permian-Triassic Mass Extinction. Sci. Rep. 6 (1), 1–7. doi:10.1038/srep28372
Holland, S. M. (2003). Analytic Rarefaction. v1.3 ed.: Available at: https://strata.uga.edu/software/.
Holmes, R. L. (1985). A Users Manual for Program COFECHA [Computer-Assisted Quality Control in Tree-Ring Dating and Measurement]. Laboratory of Tree-Ring Research, 2–23.
Holz, M. (2015). Mesozoic Paleogeography and Paleoclimates – A Discussion of the Diverse Greenhouse and Hothouse Conditions of an Alien World. J. S. Am. Earth Sci. 61, 91–107. doi:10.1016/j.jsames.2015.01.001
Horn, B. L. D., Goldberg, K., and Schultz, C. L. (2018b). A Loess Deposit in the Late Triassic of Southern Gondwana, and its Significance to Global Paleoclimate. J. S. Am. Earth Sci. 81, 189–203. doi:10.1016/j.jsames.2017.11.017
Horn, B. L. D., Goldberg, K., and Schultz, C. L. (2018a). Interpretation of Massive Sandstones in Ephemeral Fluvial Settings: A Case Study from the Upper Candelária Sequence (Upper Triassic, Paraná Basin, Brazil). J. S. Am. Earth Sci. 81, 108–121. doi:10.1016/j.jsames.2017.10.009
Hubbell, S. P. (2001). The Unified Theory of Biodiversity and Biogeography. Princeton: Princeton University Press.
Huntley, B. (1991). How Plants Respond to Climate Change: Migration Rates, Individualism and the Consequences for Plant Communities. Ann. Bot. 67, 15–22. doi:10.1093/oxfordjournals.aob.a088205
Husby, C. (2013). Biology and Functional Ecology of Equisetum with Emphasis on the Giant Horsetails. Botanical Rev. 79 (2), 147–177. doi:10.1007/s12229-012-9113-4
Irmis, R. B. (2011). Evaluating Hypotheses for the Early Diversification of Dinosaurs. Earth Environ. Sci. Trans. R. Soc. Edinb. 101, 397–426. doi:10.1017/s1755691011020068
Irmis, R. B., Mundil, R., Martz, J. W., and Parker, W. G. (2011). High-Resolution U-Pb Ages from the Upper Triassic Chinle Formation (New Mexico, USA) Support a Diachronous Rise of Dinosaurs. Earth Planet. Sci. Lett. 309, 258–267. doi:10.1016/j.epsl.2011.07.015
Irmis, R. B., Mundil, R., Mancuso, A. C., Carrillo-Briceño, J. D., Ottone, E. G., and Marsicano, C. A. (2022). South American Triassic Geochronology: Constraints and Uncertainties for the Tempo of Gondwanan Non-marine Vertebrate Evolution. J. S. Am. Earth Sci. 103770. doi:10.1016/j.jsames.2022.103770
Jones, M. E. H., Anderson, C. L., Hipsley, C. A., Müller, J., Evans, S. E., and Schoch, R. R. (2013). Integration of Molecules and New Fossils Supports a Triassic Origin for Lepidosauria (Lizards, Snakes, and Tuatara). BMC Evol. Biol. 13 (208), 201–221. doi:10.1186/1471-2148-13-208
Kalm, V. E., Rutter, N. W., and Rokosh, C. D. (1996). Clay Minerals and Their Paleoenvironmental Interpretation in the Baoji Loess Section, Southern Loess Plateau, China. Catena 27, 49–61. doi:10.1016/0341-8162(96)00008-2
Kent, D. V., Santi Malnis, P., Colombi, C. E., Alcober, O. A., and Martínez, R. N. (2014). Age Constraints on the Dispersal of Dinosaurs in the Late Triassic from Magnetochronology of the Los Colorados Formation (Argentina). Proc. Natl. Acad. Sci. 111, 7958–7963. doi:10.1073/pnas.1402369111
Kerp, H., Hass, H., and Mosbrugger, V. (2001). “New data on Nothia aphylla Lyon 1964 ex El-Saadawy et Lacey 1979, a poorly known plant from the Lower Devonian Rhynie Chert,” in Plants Invade the Land: Evolutionary and Environmental Perspectives. Editors P. G. Gensel, and D. Edwards (New York: Columbia University Press), 52–82. doi:10.7312/gens11160-005
Kidwell, S. M., and Flessa, K. W. (1996). The Quality of the Fossil Record: Populations, Species, and Communities. Annu. Rev. Earth Planet. Sci. 24, 433–464. doi:10.1146/annurev.earth.24.1.433
Kidwell, S. M., and Holland, S. M. (2002). The Quality of the Fossil Record: Implications for Evolutionary Analyses. Annu. Rev. Ecol. Syst. 33, 561–588. doi:10.1146/annurev.ecolsys.33.030602.152151
Kraus, M. J. (1999). Paleosols in Clastic Sedimentary Rocks: Their Geologic Applications. Earth Sci. Rev. 47, 41–70. doi:10.1016/s0012-8252(99)00026-4
Kraus, M. J., and Aslan, A. (1993). Eocene Hydromorphic Paleosols: Significance for Interpreting Ancient Floodplain Processes. J. Sediment. Pet.. 63, 453–463. doi:10.1306/d4267b22-2b26-11d7-8648000102c1865d
Kustatscher, E., Ash, S. R., Karasev, E., Pott, C., Vajda, V., Yu, J., et al. (2018). “Flora of the Late Triassic,” in The Late Triassic World: Earth in a Time of Transition. Editor L. H. Tanner (Cham, Switzerland: Springer International Publishing), 545–622. doi:10.1007/978-3-319-68009-5_13
Kutzbach, J. E., and Gallimore, R. G. (1989). Pangaean Climates: Megamonsoons of the Megacontinent. J. Geophys. Res. D 94, 3341–3357. doi:10.1029/jd094id03p03341
Lausberg, S., and Kerp, H. (2000). Conifer-dominated Flora from the Lower Rotliegend Near Alsenz, Saar-Nahe-Basin (Germany). Feddes Repert. 111 (7/8), 399–426. doi:10.1002/fedr.20001110706
Liu, J., Angielczyk, K. D., and Abdala, F. (2021). Permo-Triassic Tetrapods and Their Climate Implications. Glob. Planet. Change 205, 1036181–1036189. doi:10.1016/j.gloplacha.2021.103618
Liu, Z., Colin, C., Trentesaux, A., Siani, G., FrankBlamart, N. D., and Farid, S. (2005). Late Quaternary Climatic Control on Erosion and Weathering in the Eastern Tibetan Plateau and the Mekong Basin. Quat. Res. 63, 316–328. doi:10.1016/j.yqres.2005.02.005
López-Gamundí, O. R., Alvarez, L., Andreis, R., Bossi, G., Espejo, I., Fernandez-Seveso, F., et al. (1989). “Cuencas Intermontanas,” in Cuencas Sedimentarias Argentinas. Serie Correlación Geológica, No 6. Editors G. Chebli, and L. Spalletti (Instituto Superior de Correlación Geológica, Universidad Nacional de Tucumán), 123–167.
Lovecchio, J. P., Rohais, S., Joseph, P., Bolatti, N. D., and Ramos, V. A. (2020). Mesozoic Rifting Evolution of SW Gondwana: a Poly-Phased, Subduction-Related, Extensional History Responsible for Basin Formation along the Argentinean Atlantic Margin. Earth-Science Rev. 203, 1031381–1031427. doi:10.1016/j.earscirev.2020.103138
Mancuso, A. C. (2005). Revisión y aportes a la estratigrafía de la sección inferior del Grupo Agua de la Peña (Triásico Medio, Argentina). XVI Congr. Geol. Argent. La Plata, Argent. Actas 3, 415–422.
Mancuso, A. C., and Caselli, A. (2012). Paleolimnology Evolution in Rift Basins: the Ischigualasto-Villa Unión Basin (Central-western Argentina) during the Triassic. Sediment. Geol. 275-276, 38–54. doi:10.1016/j.sedgeo.2012.07.012
Mancuso, A. C., and Marsicano, C. A. (2008). Paleoenvironments and Taphonomy of a Triassic Lacustrine System (Los Rastros Formation, Central-Western Argentina). Palaios 23, 535–547. doi:10.2110/palo.2007.p07-064r
Mancuso, A. C., Benavente, C. A., Irmis, R. B., and Mundil, R. (2020a). Evidence for the Carnian Pluvial Episode in Gondwana: New Multiproxy Climate Records and Their Bearing on Early Dinosaur Diversification. Gondwana Res. 86, 104–125. doi:10.1016/j.gr.2020.05.009
Mancuso, A. C., Krapovickas, V., Benavente, C. A., and Marsicano, C. A. (2020b). An Integrative Physical, Mineralogical, and Ichnological Approach to Characterize Underfilled Lake-Basin. Sedimentology 67, 3088–3118.
Mancuso, A. C., Gaetano, L. C., Leardi, J. M., Abdala, F., and Arcucci, A. B. (2014). The Chañares Formation: a Window to the Palaeobiology of a Middle Triassic Vertebrate Fauna. Lethaia 47, 244–265. doi:10.1111/let.12055
Mancuso, A. C., Horn, B. L. D., Benavente, C. A., Schultz, C. L., and Irmis, R. B. (2021). The Paleoclimatic Context for South American Triassic Vertebrate Evolution. J. S. Am. Earth Sci. 110 (103321), 1–26. doi:10.1016/j.jsames.2021.103321
Marsicano, C., Domnanovich, N., and Mancuso, A. C. (2007). Dinosaur Origins: Evidence from the Footprint Record. Hist. Biol. 19, 83–91. doi:10.1080/08912960600866920
Marsicano, C. A., Arcucci, A. B., Mancuso, A., and Caselli, A. T. (2004). Middle Triassic Tetrapod Footprints of Southern South America. Ameghiniana 41, 171–184.
Marsicano, C. A., Mancuso, A. C., Palma, R. M., and Krapovickas, V. (2010). Tetrapod Tracks in a Marginal Lacustrine Setting (Middle Triassic, Argentina): Taphonomy and Significance. Palaeoecol. Palaeogeogr. Palaeoclimatol. 291, 388–399. doi:10.1016/j.palaeo.2010.03.009
Marsicano, C. A., Irmis, R. B., Mancuso, A. C., Mundil, R., and Chemale, F. (2016). The Precise Temporal Calibration of Dinosaur Origins. Proc. Natl. Acad. Sci. U. S. A. 113, 509–513. doi:10.1073/pnas.1512541112
Martínez, R. N., Sereno, P. C., Alcober, O. A., Colombi, C. E., Renne, P. R., Montañez, I. P., et al. (2011). A Basal Dinosaur from the Dawn of the Dinosaur Era in Southwestern Pangaea. Science 331, 206–210. doi:10.1126/science.1198467
Mays, C., and McLoughlin, S. (2019). Caught between Mass Extinctions—The Rise and Fall of Dicroidium. Deposits Mag. 59, 43–47.
McElwain, J. C. (2018). Paleobotany and Global Change: Important Lessons for Species to Biomes from Vegetation Responses to Past Global Change. Annu. Rev. Plant Biol. 69, 761–787. doi:10.1146/annurev-arplant-042817-040405
McGill, B. J., Hadly, E. A., and Maurer, B. A. (2005). Community Inertia of Quaternary Small Mammal Assemblages in North America. Proc. Natl. Acad. Sci. 102, 16701–16706. doi:10.1073/pnas.0504225102
McLoughlin, S. (2001). The Breakup History of Gondwana and its Impact on Pre-cenozoic Floristic Provincialism. Aust. J. Bot. 49 (3), 271–300. doi:10.1071/bt00023
McLoughlin, S. (2011). Glossopteris: Insights into the Architecture and Relationships of an Iconic Permian Gondwanan Plant. J. Botanical Soc. Bengal 65, 93–106.
Miall, A. D. (1996). The Geology of Fluvial Deposits: Sedimentary Facies, Basin Analysis and Petroleum Geology. Berlin: Springer-Verlag, 582.
Milana, J. P., and Alcober, O. (1994). Modelo tectosedimentario de la Cuenca Triásica de Ischigualasto (San Juan, Argentina). Rev. Asoc. Geol. Argent. 49, 217–235.
Miller, C. S., Peterse, F., da Silva, A. C., Baranyi, V., Reichart, G. J., and Kürschner, W. M. (2017). Astronomical Age Constraints and Extinction Mechanisms of the Late Triassic Carnian Crisis. Nat. Sci. Rep. 7, 1–7. doi:10.1038/s41598-017-02817-7
Milroy, P., Wright, V. P., and Simms, M. J. (2019). Dryland Continental Mudstones: Deciphering Environmental Changes in Problematic Mudstones from the Upper Triassic (Carnian to Norian) Mercia Mudstone Group, South‐west Britain. Sedimentology 66, 2557–2589. doi:10.1111/sed.12626
Minin, A. A., and Voskova, A. V. (2014). Homeostatic Responses of Plants to Modern Climate Change: Spatial and Phenological Aspects. Russ. J. Dev. Biol. 45 (3), 127–133. doi:10.1134/s1062360414030023
Mishra, S., Aggarwal, N., and Jha, N. (2017). Palaeoenvironmental Change across the Permian-Triassic Boundary Inferred from Palynomorph Assemblages (Godavari Graben, South India). Palaeobiodiversity Palaeoenvironments 98, 177–204. doi:10.1007/s12549-017-0302-3
Moore, D. M., and Reynolds, R. C. (1997). X-Ray Diffraction and the Identification and Analysis of Clay Minerals. Oxford: Oxford University Press, 337.
Moritz, C., Patton, J. L., Conroy, C. J., Parra, J. L., White, G. C., and Beissinger, S. R. (2008). Impact of a Century of Climate Change on Small-Mammal Communities in Yosemite National Park, USA. Science 5899, 261–264. doi:10.1126/science.1163428
Nesbitt, H. W., and Young, G. M. (1982). Early Proterozoic Climates and Plate Motions Inferred from Major Element Chemistry of Lutites. Nature 299, 715–717. doi:10.1038/299715a0
Nordt, L. C., and Driese, S. D. (2010). New Weathering Index Improves Paleorainfall Estimates from Vertisols. Geology 38, 407–410. doi:10.1130/g30689.1
Nowak, H., Schneebeli-Hermann, E., and Kustatscher, E. (2019). No Mass Extinction for Land Plants at the Permian-Triassic Transition. Nat. Commun. 10, 1–8. doi:10.1038/s41467-018-07945-w
Nowak, H., Vérard, C., and Kustatscher, E. (2020). Palaeophytogeographical Patterns across the Permian-Triassic Boundary. Front. Earth Sci. 8, 609. doi:10.3389/feart.2020.613350
Ogg, J. G. (2015). The Mysterious Mid-Carnian “Wet Intermezzo” Global Event. J. Earth Sci. 26, 181–191. doi:10.1007/s12583-015-0527-x
Ordoñez, A., Marsicano, C. A., and Mancuso, A. C. (2020). New Material of Dinodontosaurus (Therapsida, Anomodontia) from West-Central Argentina and a Reassessment of the Triassic South American Dinodontosaurus Assemblage Zone. J. S. Am. Earth Sci., 102597.
Ottone, E. G., and Mancuso, A. C. (2006). Algas Chlorococcales Como Indicadores Paleoambientales: Nuevos Datos De La Formación Los Rastros, Triásico Medio A Superior Del Centro–Oeste De Argentina. Revista del Museo Argentino de Ciencias Naturales, Nueva Serie 8, 209–220. doi:10.22179/revmacn.8.321
Ottone, E. G., Mancuso, A. C., and Resano, M. (2005). Miospores and Chlorococcalean Algae from the Los Rastros Formation, Middle to Upper Triassic of Central-Western Argentina. Ameghiniana 42, 347–362.
Ovejero, R., and Bossi, G. E. (1984). Asociaciones mineralogicas de lasarcillas de la Cuenca de Ischigualasto-Ischichuca. Parte III: Perfil Zanja de la Viuda. Noveno Congreso Geologico Argentio Actas V, 197–208.
Pardoe, H. S., Cleal, C. J., Berry, C. M., Cascales-Miñana, B., Davis, B. A., Diez, J. B., et al. (2021). Palaeobotanical Experiences of Plant Diversity in Deep Time. 2: How to Measure and Analyse Past Plant Biodiversity. Palaeogeogr. Palaeoclimatol. Palaeoecol. 580, 110618. doi:10.1016/j.palaeo.2021.110618
Parrish, J. T. (1993). Climate of the Supercontinent Pangaea. J. Geol. 101, 215–233. doi:10.1086/648217
Pedernera, T. E., Mancuso, A. C., Ottone, E. G., and Benavente, C. A. (2020). Paleobotany of the Upper Triassic Los Rastros Formation, Ischigualasto-Villa Unión Basin, La Rioja, Argentina. J. S. Am. Earth Sci. 102, 102660. doi:10.1016/j.jsames.2020.102660
Perez Loinaze, V. S., Vera, E. I., Fiorelli, L. E., and Desojo, J. B. (2018). Palaeobotany and Palynology of Coprolites from the Late Triassic Chañares Formation of Argentina: Implications for Vegetation Provinces and the Diet of Dicynodonts. Palaeogeogr. Palaeoclimatol. Palaeoecol. 502, 31–51. doi:10.1016/j.palaeo.2018.04.003
Péron, S., Bourquin, S., Fluteau, F., and Guillocheau, F. (2005). Paleoenvironment Reconstructions and Climate Simulations of the Early Triassic: Impact of the Water and Sediment Supply on the Preservation of Fluvial Systems. Geodin. Acta 18, 431–446.
Pteridophyte Phylogeny Group (2016). A Community‐derived Classification for Extant Lycophytes and Ferns. J. Syst. Evol. 54 (6), 563–603.
Raup, D. M. (1972). Taxonomic Diversity during the Phanerozoic. Science 177, 1065–1071. doi:10.1126/science.177.4054.1065
Raup, D. M. (1975). Taxonomic Diversity Estimation Using Rarefaction. Paleobiology 1, 333–342. doi:10.1017/s0094837300002633
Retallack, G. J. (1977). Reconstructing Triassic Vegetation of Eastern Australasia: a New Approach for the Biostratigraphy of Gondwanaland. Alcheringa 1, 253–283. doi:10.1080/03115517708527763
Retallack, G. J. (1988). “Field Recognition of Paleosols,” in Paleosols and Weathering through Geologic Time: Principles and Applications, Special Paper 216. Editors J. Reinhardt, and W. R. Sigleo (Geological Society of America), 1–20. doi:10.1130/spe216-p1
Retallack, G. J. (2001). Soils of the Past: An Introduction to Paleopedology. Oxford, UK: Blackwell Sci.
Retallack, G. J. (2009). Greenhouse Crises of the Past 300 Million Years. Geol. Soc. Am. Bull. 121, 1441–1455. doi:10.1130/b26341.1
Retallack, G. J., Sheldon, N. D., Carr, P. F., Fanning, M., Thompson, C. A., Williams, M. L., et al. (2011). Multiple Early Triassic Greenhouse Crises Impeded Recovery from Late Permian Mass Extinction. Palaeogeogr. Palaeoclimatol. Palaeoecol. 308, 233–251. doi:10.1016/j.palaeo.2010.09.022
Robinson, P. L. (1971). A Problem of Faunal Replacement on Permo-Triassic Continents. Palaeontology 14, 131–153.
Robinson, P. L. (1973). “Palaeoclimatology and Continental Drift,” Implications of Continental Drift to the Earth Sciences. Editors D. H. Tarling, and S. K. Runcorn (London: Academic Press), Vol. 1, 451–476.
Rogers, R. R., Arcucci, A. B., Abdala, F., Sereno, P. C., Forster, C. A., and May, C. L. (2001). Paleoenvironment and Taphonomy of the Chañares Formation Tetrapod Assemblage (Middle Triassic), Northwestern Argentina: Spectacular Preservation in Volcanogenic Concretions. Palaios 16, 461–481. doi:10.1669/0883-1351(2001)016<0461:patotc>2.0.co;2
Rogers, R., Swisher, C., Sereno, P., Monetta, A., Forster, C., and Martínez, R. (1993). The Ischigualasto Tetrapod Assemblage (Late Triassic, Argentina) and 40Ar/39Ar Dating of Dinosaur Origins. Science 260, 794–797. doi:10.1126/science.260.5109.794
Romer, A. S. (1970). “The Triassic Faunal Succession and the Gondwanaland Problem,” in Gondwana Stratigraphy, IUGS Symposium (Paris: UNESCO), 375–400.
Rosindell, J., Hubbell, S. P., and Etienne, R. S. (2011). The Unified Neutral Theory of Biodiversity and Biogeography at Age Ten. Trends Ecol. Evol. 26, 340–348. doi:10.1016/j.tree.2011.03.024
Rosindell, J., Hubbell, S. P., He, F., Harmon, L. J., and Etienne, R. S. (2012). The Case for Ecological Neutral Theory. Trends Ecol. Evol. 27, 203–208. doi:10.1016/j.tree.2012.01.004
Rostási, Á., Raucsik, B., and Varga, A. (2011). Palaeoenvironmental Controls on the Clay Mineralogy of Carnian Sections from the Transdanubian Range (Hungary). Palaeogeogr. Palaeoclimatol. Palaeoecol. 300, 101–112.
Rowe, H., Hughes, N., and Robinson, K. (2012). The Quantification and Application of Handheld Energy-Dispersive X-Ray Fluorescence (ED-XRF) in Mudrock Chemostratigraphy and Geochemistry. Chem. Geol. 324-325, 122–131. doi:10.1016/j.chemgeo.2011.12.023
Rowe, K. C., Rowe, K. M. C., Tingley, M. W., Koo, M. S., Patton, J. L., Conroy, C. J., et al. (2015). Spatially Heterogeneous Impact of Climate Change on Small Mammals of Montane California. Proc. R. Soc. Lond. Biol. Sci. 282, 1–10. doi:10.1098/rspb.2014.1857
Ruffell, A., McKinley, J. M., and Worden, R. H. (2002). Comparison of Clay Mineral Stratigraphy to Other Proxy Palaeoclimate Indicators in the Mesozoic of NW Europe. Philosophical Trans. R. Soc. Lond. A 360, 675–693. doi:10.1098/rsta.2001.0961
Ruffell, A., Simms, M. J., and Wignall, P. B. (2016). The Carnian Humid Episode of the Late Triassic: a Review. Geol. Mag. 153, 271–284. doi:10.1017/s0016756815000424
Ruffo Rey, L. J. (2021). Vegetation dynamics of Cerro de Las Cabras Formation (Middle Triassic) from palynological assemblages and the Eco-Guild model: New paleoenvironmental and paleoclimatic interpretations. J. S. Am. Earth Sci. 112, 103629. doi:10.1016/j.jsames.2021.103629
Ruiz, F., and Introcaso, A. (1999). Un modelo gravimétrico 3D de la profunda cuenca sedimentaria de Ischigualasto-Villa Unión (San juan y La Rioja) Argentina. Rev. Bras. Geof 17, 3–11. doi:10.1590/s0102-261x1999000100001
Santi Malnis, P., Colombi, C. E., Rothis, L. M., and Alcober, O. (2020). Fluvial Architecture and Paleoenvironmental Evolution of the Los Colorados Formation (Norian): Postrift Stage of the Ischigualasto–Villa Union Basin, NW Argentina. J. Sediment. Res. 90, 1436–1462. doi:10.2110/jsr.2020.65
Schoch, R. R., and Sues, H.-D. (2015). A Middle Triassic Stem-Turtle and the Evolution of the Turtle Body Plan. Nature 523, 584–587. doi:10.1038/nature14472
Schultz, C. L., Martinelli, A. G., Soares, M. B., Pinheiro, F. L., Kerber, L., Horn, B. L. D., et al. (2020). Triassic Faunal Successions of the Paraná Basin, Southern Brazil. J. S. Am. Earth Sci. 104, 102846. doi:10.1016/j.jsames.2020.102846
Sellwood, B. W., and Valdes, P. J. (2006). Mesozoic Climates: General Circulation Models and the Rock Record. Sediment. Geol. 190, 269–287. doi:10.1016/j.sedgeo.2006.05.013
Sheldon, N. D. (2006). Quaternary Glacial-Interglacial Climate Cycles in Hawaii. J. Geol. 114, 367–376. doi:10.1086/500993
Sheldon, N. D., and Tabor, N. J. (2009). Quantitative Paleoenvironmental and Paleoclimatic Reconstruction Using Paleosols. Earth Sci. Rev. 95, 1–52. doi:10.1016/j.earscirev.2009.03.004
Sheldon, N. D., Retallack, G. J., and Tanaka, S. (2002). Geochemical Climofunctions from North America Soils and Application to Paleosols across the Eocene–Oligocene Boundary in Oregon. J. Geol. 110, 687–696. doi:10.1086/342865
Shubin, N. H., and Sues, H.-D. (1991). Biogeography of Early Mesozoic Continental Tetrapods: Patterns and Implications. Paleobiology 17, 214–230. doi:10.1017/s0094837300010575
Simms, M. J., and Ruffell, A. H. (1989). Synchroneity of Climatic Change in the Late Triassic. Geology 17, 265–268. doi:10.1130/0091-7613(1989)017<0265:soccae>2.3.co;2
Simms, M. J., and Ruffell, A. H. (1990). Climatic and Biotic Change in the Late Triassic. J. Geol. Soc., London 147, 321–327. doi:10.1144/gsjgs.147.2.0321
Singer, A. (1980). The Paleoclimatic Interpretation of Clay Minerals in Soils and Weathering Profiles. Earth-Sciences Rev. 15, 303–326. doi:10.1016/0012-8252(80)90113-0
Singer, A. (1984). The Paleoclimatic Interpretation of Clay Minerals in Sediments—A Review. Earth-Sciences Rev. 21, 251–293. doi:10.1016/0012-8252(84)90055-2
Smith, A. R., Pryer, K. M., Schuettpelz, E., Korall, P., Schneider, H., and Wolf, P. G. (2006). A Classification for Extant Ferns. Taxon 55, 705–731. doi:10.2307/25065646
Spalletti, L. A., Artabe, A. E., and Morel, E. M. (2003). Geological Factors and Evolution of Southwestern Gondwana Triassic Plants. Gondwana Res. 6, 119–134. doi:10.1016/s1342-937x(05)70648-1
Stenseth, N. C., and Maynard Smith, J. (1984). Coevolution in Ecosystems: Red Queen Evolution or Stasis? Evolution 38, 870–880. doi:10.1111/j.1558-5646.1984.tb00358.x
Stewart, W. N., and Rothwell, G. W. (1993). Paleobotany and the Evolution of Plants. Cambridge: Cambridge University Press.
Stipanicic, P. N., and Marsicano, C. (2002). Léxico Estratigráfico de la Argentina: TRIÁSICO. Asociación Geológica Argentina.
Stocker, M. R., Nesbitt, S. J., Kligman, B. T., Paluh, D. J., Marsh, A. D., Blackburn, D. C., et al. (2019). The Earliest Equatorial Record of Frogs from the Late Triassic of Arizona. Biol. Lett. 15, 1–5. doi:10.1098/rsbl.2018.0922
Sun, Y., Joachimski, M. M., Wignall, P. B., Yan, C., Chen, Y., Jiang, H., et al. (2012). Lethally Hot Temperatures during the Early Triassic Greenhouse. Science 338, 366–370. doi:10.1126/science.1224126
Sun, Y. D., Wignall, P. B., Joachimski, M. M., Bond, D. P. G., Grasby, S. E., Laib, X. L., et al. (2016). Climate Warming, Euxinia and Carbon Isotope Perturbations during the Carnian (Triassic) Crisis in South China. Earth Planet. Sci. Lett. 444, 88–100. doi:10.1016/j.epsl.2016.03.037
Tabor, N. J., and Myers, T. S. (2015). Paleosols as Indicators of Paleoenvironment and Paleoclimate. Annu. Rev. Earth Planet. Sci. 43, 333–361. doi:10.1146/annurev-earth-060614-105355
Tabor, N. J., Montañez, I. P., Zierenberg, R., and Currie, B. S. (2004). Mineralogical and Geochemical Evolution of a Basalt-Hosted Fossil Soil (Late Triassic, Ischigualasto Formation, Northwest Argentina); Potential for Paleoenvironmental Reconstruction. Geol. Soc. Am. Bull. 116, 1280–1293. doi:10.1130/b25222.1
Tabor, N. J., Montañez, I. P., Kelso, K. A., Currie, B. S., Shipman, T. A., and Colombi, C. E. (2006). Late Triassic Soil Catena: Landscape and Climate Controls on Paleosol Morphology and Chemistry across the Carnian-Age Ischigualasto–Villa Union Basin, Northwestern Argentina. Geological Society of America Special Paper 416, 17–42.
Taylor, T. N., Taylor, E. L., and Krings, M. (2009). “Cycadophytes,” in Paleobotany, the Biology and Evolution of Fossil Plants. Editors T. N. Taylor, E. L. Taylor, and M. Krings (Amsterdam): Academic Press), 703–741. doi:10.1016/b978-0-12-373972-8.00017-6
Thuiller, W., Lavorel, S., Araújo, M. B., Sykes, M. T., and Prentice, I. C. (2005). Climate Change Threats to Plant Diversity in Europe. Proc. Natl. Acad. Sci. 102 (23), 8245–8250. doi:10.1073/pnas.0409902102
Tingley, M. W., Monahan, W. B., Beissinger, S. R., and Moritz, C. (2009). Birds Track Their Grinnellian Niche through a Century of Climate Change. Proc. Natl. Acad. Sci. 106 (Suppl. 2), 19637–19643. doi:10.1073/pnas.0901562106
Tipper, J. C. (1979). Rarefaction and Rarefiction-The Use and Abuse of a Method in Paleoecology. Paleobiology 5, 423–434. doi:10.1017/s0094837300016924
Tomimatsu, Y., Nozaki, T., Sato, H., Takaya, Y., Kimura, J. I., Chang, Q., et al. (2021). Marine Osmium Isotope Record during the Carnian “Pluvial Episode” (Late Triassic) in the Pelagic Panthalassa Ocean. Glob. Planet. Change 197, 103387. doi:10.1016/j.gloplacha.2020.103387
Uliana, M. A., and Biddle, K. (1988). Mesozoic-Cenozoic Paleogeographic and Geodynamic Evolution of Southern South America. Rev. Bras. Geociências 18, 172–190. doi:10.25249/0375-7536.1988182172190
Uliana, M. A., Biddle, K. T., and Cerdan, J. (1989). Mesozoic Extension and the Formation of Argentine Sedimentary Basins. Tulsa: American Association of Petroleum Geologists, Memoir 46, 599–614.
Vajda, V., McLoughlin, S., Mays, C., Frank, T. D., Fielding, C. R., and Tevyaw, A. (2020). End-Permian (252 Mya) Deforestation, Wildfires and Flooding -An Ancient Biotic Crisis with Lessons for the Present. Earth PlanetScience Lett. 529, 115875. doi:10.1016/j.epsl.2019.115875
Van Hinsbergen, D. J. J., De Groot, L. V., Van Schaik, S. J., Spakman, W., Bijl, P. K., and Sluijs, A. (2015). A Paleolatitude Calculator for Paleoclimate Studies. PLoS ONE 10, 1–21. doi:10.1371/journal.pone.0126946
Vitt, L. J., and Caldwell, J. P. (2014). Herpetology: An Introductory Biology of Amphibians and Reptiles. Amsterdam: Academic Press, 757.
Vrba, E. S. (1985). Environment and Evolution: Alternative Causes of the Temporal Distribution of Evolutionary Events. South Afr. J. Sci. 81, 229–236.
Vrba, E. S. (1992). Mammals as a Key to Evolutionary Theory. J. Mammal. 73, 1–28. doi:10.2307/1381862
Vrba, E. S. (1993). Turnover-pulses, the Red Queen, and Related Topics. Am. J. Sci. 293-A, 418–452. doi:10.2475/ajs.293.a.418
Vrba, E. S. (1995). “On the Connections between Paleoclimate and Evolution,” in Paleoclimate and Evolution, with Emphasis on Human Origins. Editors E. S. Vrba, G. H. Denton, T. C. Partridge, and L. H. Burckle (New Haven: Yale University Press), 24–45.
Wang, X., and Zheng, S. (2010). Whole Fossil Plants of Ephedra and Their Implications on the Morphology, Ecology and Evolution of Ephedraceae (Gnetales). Chin. Sci. Bull. 55 (15), 1511–1519. doi:10.1007/s11434-010-3069-8
Whiteside, J. H., and Grice, K. (2016). Biomarker Records Associated with Mass Extinction Events. Annu. Rev. Earth Planet. Sci. 44, 581–612. doi:10.1146/annurev-earth-060115-012501
Whiteside, J. H., Grogan, D. S., Olsen, P. E., and Kent, D. V. (2011). Climatically Driven Biogeographic Provinces of Late Triassic Tropical Pangea. Proc. Natl. Acad. Sci. 108, 8972–8977. doi:10.1073/pnas.1102473108
Whiteside, J. H., Lindström, S., Irmis, R. B., Glasspool, I. J., Schaller, M. F., Dunlavey, M., et al. (2015). Extreme Ecosystem Instability Suppressed Tropical Dinosaur Dominance for 30 Million Years. Proc. Natl. Acad. Sci. 112, 7909–7913. doi:10.1073/pnas.1505252112
Wilson, K. M., Pollard, D., Hay, W. W., Thompson, S. l., and Wold, C. N. (1994). General Circulation Model Simulations of Triassic Climates: Preliminary Results. Geological Society of America Special Paper 288, 91–116. doi:10.1130/spe288-p91
Zamuner, A. B., Zavattieri, A. M., Artabe, A. E., and Morel, E. M. (2001). “Paleobotánica,” in El Sistema Triásico en la Argentina. Editors A. E. Artabe, E. M. Morel, and A. B. Zamuner (La Plata): Fundación Museo de La Plata “Francisco Pascasio Moreno”), 143–184.
Zavattieri, A. M., and Batten, D. J. (1996). “Miospores from Argentinian Triassic Deposits and Their Potential for Intercontinental Correlation,” In Palynology: Principles and Applications. Editors J. Jansonius, and D. C. Mcgregor (Dallas: Association of Stratigraphic Palynologists Foundation), 2, 767–778.
Zhang, J., Lenz, O. K., Hornung, J., Wang, P., Ebert, M., and Hinderer, M. (2020). Palynology and the Eco-Plant Model of Peat-Forming Wetlands of the Upper Triassic Haojiagou Formation in the Junggar Basin, Xinjiang, NW China. Palaeogeogr. Palaeoclimatol. Palaeoecol. 556, 109888. doi:10.1016/j.palaeo.2020.109888
Keywords: Carnian Pluvial Episode, Gondwana, Ischigualasto Formation, Ischigualasto-Villa Unión Basin, multiproxy climate dataset
Citation: Mancuso AC, Irmis RB, Pedernera TE, Gaetano LC, Benavente CA and Breeden III BT (2022) Paleoenvironmental and Biotic Changes in the Late Triassic of Argentina: Testing Hypotheses of Abiotic Forcing at the Basin Scale. Front. Earth Sci. 10:883788. doi: 10.3389/feart.2022.883788
Received: 25 February 2022; Accepted: 03 May 2022;
Published: 13 June 2022.
Edited by:
Jacopo Dal Corso, China University of Geosciences Wuhan, ChinaReviewed by:
Piero Gianolla, Department of Physics and Earth Science, ItalyAlastair Ruffell, Queen’s University Belfast, United Kingdom
Copyright © 2022 Mancuso, Irmis, Pedernera, Gaetano, Benavente and Breeden III. This is an open-access article distributed under the terms of the Creative Commons Attribution License (CC BY). The use, distribution or reproduction in other forums is permitted, provided the original author(s) and the copyright owner(s) are credited and that the original publication in this journal is cited, in accordance with accepted academic practice. No use, distribution or reproduction is permitted which does not comply with these terms.
*Correspondence: Adriana C. Mancuso, YW1hbmN1QG1lbmRvemEtY29uaWNldC5nb2IuYXI=