- 1Faculty of Biosphere-Geosphere Science, Okayama University of Science, Okayama, Japan
- 2Division of Materials and Manufacturing Science, Graduate School of Engineering, Osaka University, Osaka, Japan
- 3Department of Natural Environmental Studies, Graduate School of Frontier Sciences, The University of Tokyo, Kashiwa, Japan
- 4Paläontologisches Institut und Museum der Universität Zürich, Zürich, Switzerland
- 5Osaka Museum of Natural History, Osaka, Japan
- 6Faculty of Science, University of the Ryukyus, Okinawa, Japan
- 7Kitakyushu Museum of Natural History & Human History, Kitakyushu, Japan
- 8Department of Anthropology, National Museum of Nature and Science, Ibaraki, Japan
Islands are a classic focus for evolutionary studies. One topic of great interest has been the evolution of “dwarfs,” significantly smaller island mammals relative to their continental counterparts. Although a consensus has been achieved regarding the multivariate ecological causes behind changes in body size, the processes involved remain largely unexplored. Life history variables, including age at first reproduction, growth rate, and longevity, are likely to be key to understanding the process of insular dwarfism. The Japanese archipelago, with its numerous islands, offers a unique natural experiment for the evolution of different sizes within the same group of organisms; namely, deer. Thus, we investigated eight deer populations with a total number of 52 individuals exhibiting body size variation, both extant and fossil, to clarify the effect of insularity on life history traits. We applied several methods to both extant and extinct populations to resolve life history changes among these deer populations. Skeletochronology, using lines of arrested growth formed in long bones (femur and tibia), successfully reconstructed body growth curves and revealed a gradual change in growth trajectories reflecting the degree of insularity. Slower growth rates with prolonged growth periods in more isolated deer populations were revealed. An extensive examination of bone microstructure further corroborated this finding, with much slower growth and later somatic maturity evident in fossil insular deer isolated for more than 1.5 Myr. Finally, mortality patterns assessed by demographic analysis revealed variation among deer populations, with a life history of insular populations shifting toward the “slow life.”
1 Introduction
Among the most iconic examples of visible evolutionary changes are island mammals that have dramatically changed in body size (Foster, 1964; van der Geer et al., 2010; Benitez-Lopez et al., 2021). While elephants, hippos, and deer on islands across the world became smaller, rodents and other small mammals became larger (van der Geer et al., 2010). These changes have led to the suggestion of the “island rule” (Foster, 1964). Classic examples are found in fossil species: the pygmy elephant (Elephas falconeri) of Sicily, which has a body mass that is only 1% of its mainland ancestor (Larramendi and Palombo, 2015), and the gigantic gymnure (Deinogalerix koenigswaldi) of Miocene Gargano, with a body mass about 200 times more than its ancestor (Lomolino et al., 2013). This leads to a hypothesis about the optimal body size of mammals on isolated islands, although the particular optimum of an insular population varies with the characteristics of the islands and the species, and the interactions among species (Lomolino et al., 2012; Lomolino et al., 2013).
Moreover, as body size is closely related to various aspects of organisms’ lives, recent studies have focused on the relationship between body size and life history, including growth rate, age of reproduction, and longevity on islands (Sander et al., 2006; Köhler and Moyà-Solà, 2009; Marín-Moratalla et al., 2011). A reduction in the body size of large mammals has also been associated with a modification in growth trajectory (Palkovacs, 2003; Long et al., 2019), which in turn affects life span, e.g., the timing of sexual maturity or longevity. Life history theory, an extension of r/K selection theory in ecology (Begon et al., 2006), proposes that mammalian populations can be placed along a fast–slow continuum (Oli, 2004). Species that adapted to unstable environments, under which high intrinsic rates of natural increase (r) were adaptive, evolved a set of life history traits, such as rapid body growth, early sexual maturity, many offspring for a clutch, and a short life span, occupying the “fast” end of the continuum (formerly referred to as “r-selected”). In contrast, in stable environments, populations are maintained close to their carrying capacity (K), with the resulting intraspecific competition favoring those with slow body growth, late sexual maturity, low numbers of offspring per clutch, and long life spans, thus occupying the “slow” end of the continuum (“K-selected”). Based on this theoretical background, life history traits have been estimated for fossil insular species. Such studies have been conducted by applying the histological analysis of bones developed to reconstruct the paleoecology of dinosaurs and other Mesozoic vertebrates (Castanet et al., 2000; Sander et al., 2006; Erickson et al., 2007; Erickson, 2014). This method has also been applied by mammalian paleontologists as a useful tool to infer life history traits in mammals (Klevezal, 1996; Köhler and Moyà-Solà, 2009; Köhler et al., 2012; Marín-Moratalla et al., 2013; Kolb et al., 2015a; Amson et al., 2015; Kolb et al., 2015b). Köhler and Moyà-Solà (2009) showed that the long bone histology in a fossil dwarfed bovine, Myotragus balearicus, from the Balearic Islands of the Mediterranean demonstrated lamellar–zonal tissue throughout the cortex, which is characteristic of ectothermic reptiles. The bone microstructures suggest that M. balearicus had a low metabolism and a very slow growth rate. This life history shifts toward a slow life in M. balearicus was further corroborated by analyses of tooth enamel microstructure, tooth eruption, and mortality pattern, which implied delayed somatic maturity, lower mortality, and extended longevity in this species (Jordana and Köhler, 2011; Marín-Moratalla et al., 2011; Jordana et al., 2012). Following these paleohistological analyses were those of fossil mammals from other Mediterranean islands, e.g., a fossil cervid (Candiacervus) from Crete showing a slight shift toward a slower life history (Kolb et al., 2015a; Miszkiewicz and van der Geer, 2022) and a fossil dwarf hippo (Hippopotamus minor) from Cyprus with no evident modification in bone histology (Kolb et al., 2015b). While bone histological observations and life history implications of fossil dwarfs have accumulated over the past decade, these studies have been based on different species from single islands; therefore, it has been challenging to generalize the evolution of body size and life history across islands. Multiple comparisons of closely related species or populations from both the mainland and the islands are crucial for drawing general conclusions.
Further important issues requiring exploration are the process of body size and life history evolution (Lister, 1996), including how environmental factors such as the lack of predators and competitors, resource limitation on islands, founder effects of island immigrants, and the remoteness and duration of isolation affect processes (Raia and Meiri, 2006; van der Geer et al., 2010; Lomolino et al., 2012; Lomolino et al., 2013; van der Geer et al., 2013). Drastic body size changes have been reported for fossil species or populations (van der Geer et al., 2010). Nevertheless, as these studies focused only on fossil evidence, the ecological processes during these changes remain unclear owing to the lack of detailed ecological information. To better understand these changes, studies on the mainland and insular populations of a single extant species are particularly useful, as they provide ecological contrasts between focal populations and allow control for confounding factors other than living on islands. Variation in isolation duration among the populations can, therefore, mimic the process of insular settlement.
The present study focused on extant and extinct populations of deer from the islands and mainland in the Japanese archipelago (Figure 1). Our targets covered an extant sika deer (Cervus nippon) population that was introduced recently (c.a. 400 years ago) on a small island, four extant insular and mainland deer populations [sika deer, Reeves’ muntjac (Muntiacus reevesi)] with variable body sizes, and extinct insular deer populations (Cervus astylodon and Muntiacini gen. et. sp. indet., referred to as “the Ryukyu muntjac”) that were isolated for more than 1.5 Myr on Okinawa Island (Otsuka and Takahashi, 2000), representing different degrees of insular effect. We also included an extinct gigantic mainland deer (Sinomegaceros yabei) from the late Pleistocene deposits on mainland Honshu with a body size comparable to the largest extant deer, the moose (Alces alces) (Taruno et al., 2017). The immense variation of the island’s size and duration of isolation observed in the eight understudied deer populations enabled us to infer the process of body size and life history changes on the islands.
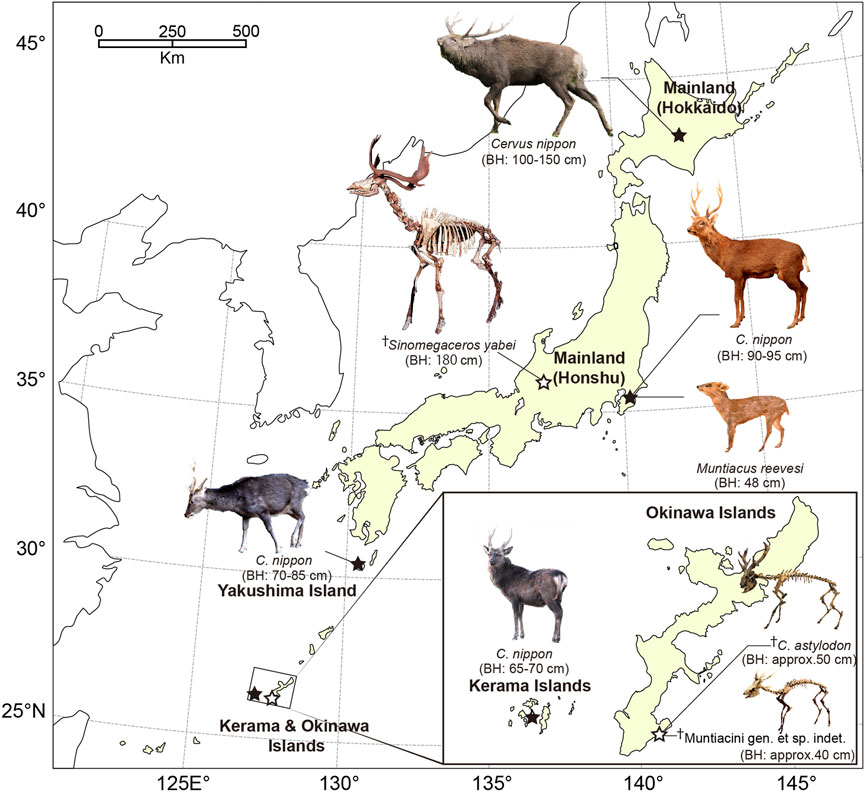
FIGURE 1. Locations of the sampled deer populations in Japan. A dagger (†) indicates fossils excavated from late Pleistocene deposits. The localities of extant and extinct taxa are shown in closed and open stars, respectively. The approximate body height (BH) was also indicated for each species/population. Photography of fossil cervids is courtesy of the Gunma Museum of Natural History (Sinomegaceros yabei) and Okinawa Prefectural Museum & Art Museum (Cervus astylodon and Muntiacini gen. et sp. indet.).
This study tested whether the different degrees of insular effect resulted in changes in the life history traits of Japanese deer populations. Specifically, we hypothesized that a gradual change in life history traits of deer populations would be expected according to the intensity of insular effects, with longer isolation on smaller islands, resulting in a shift toward a more pronounced slow life. To this end, we first applied skeletochronology to long bones, using lines of arrested growth (LAGs) within the primary periosteal bone tissue, to indicate body growth rates. The resulting growth curve parameters were statistically compared among deer populations to test the hypothesis. Subsequently, detailed qualitative descriptions of bone histology were conducted to corroborate the skeletochronological findings on growth patterns and somatic maturity. We focused on periosteal bone tissue and vascularization patterns as indicators of the relative bone growth rates (de Margerie et al., 2002; Köhler and Moyà-Solà, 2009). Finally, we conducted demographic analyses of age data, which were collected either through ecological surveys or tooth wear analysis of fossil deer assemblages to quantitatively compare mortality patterns among deer populations.
2 Materials and methods
2.1 Locality and specimen information for the sampled deer used in the histological analyses
For histological investigation and estimation of growth curves from LAGs, we used the femora and tibiae of extant and fossil deer. All extant deer were wild individuals whose skeletal specimens were stored in museums. Both the tibia and femur were sampled from the same individual for the extant deer. In contrast, all fossil specimens were excavated in disassociated conditions. Therefore, we considered that each of the femora or tibiae originated from a different individual. Table 1 summarizes the sampled populations and sample sizes.
2.1.1 Extant deer populations
Sika deer from mainland Hokkaido: all individuals (N = 7) were hunted in Hokkaido Prefecture (43°N, 143°E), northern Japan (Figure 1). Hokkaido is one of the four main islands constituting the Japanese archipelago and was considered “mainland” in this study. Hokkaido sika deer inhabit subarctic coniferous forests mixed with cool temperate deciduous trees and are the largest subspecies (C. nippon yesoensis, Ohtaishi, 1986; Groves, 2006; Kubo and Takatsuki, 2015). The complete skeletons are housed in the Hokkaido University Museum (HOUMVC), Sapporo, Japan. The age at death was recorded in the museum inventory list, which was assessed based on tooth eruption for fawns and yearlings and the cementum annuli formed at the roots of the incisors for adults.
Sika deer (N = 8) and Reeves’ muntjac (N = 5) from mainland Honshu: all individuals were hunted or collected in Chiba Prefecture (35°N, 140°E, Figure 1), which is in the central region of Honshu. Honshu is one of the four main islands constituting the Japanese archipelago and was considered “mainland” in this study. Both species inhabit evergreen broadleaved forests in the temperate zone. Reeves’ muntjac was introduced to Chiba Prefecture ca. 1960–1980 following an escape from a private park (Chiba Prefecture, 2007). The source population is unknown; the body size of these deer is smaller than that of the continental population but closer to that of the Taiwanese population (Chiba Prefecture, 2007). It is not clear whether the Reeves’ muntjacs studied here or those in Taiwan are dwarfed populations compared with their counterparts on the continent. The complete skeletons of these individuals are stored at the Natural History Museum and Institute, Chiba (CBM), Japan. The age at death was recorded in the museum inventory list, which was assessed based on tooth eruption or cementum annuli for the sika deer and tooth eruption and molar wear condition for Reeves’ muntjac.
Sika deer from Yakushima Island: all individuals (N = 4) were hunted or collected on Yakushima Island (30°18′N, 130°30′E, Figure 1) in Kagoshima Prefecture, Japan, where they inhabit subtropical evergreen broadleaved forests. Yakushima Island deer are considered a subspecies of C. nippon yakushimae (Ohtaishi, 1986) and show considerable phenotypic differences from the mainland populations, including small body size, simplified antlers, and shortened limbs (Ohtaishi, 1986; Terada et al., 2012). Yakushima deer diverged from the population on Kyushu Island (one of the four main islands constituting the Japanese archipelago) ca. 0.1 Mya when the Ohsumi Strait was formed between the two islands (Ohshima, 1990). While Yakushima Island was possibly connected to Kyushu Island by a land bridge during the Last Glacial Maximum (ca. 25,000 ya), the genetic differentiation between the two populations implies a much longer period of isolation for Yakushima deer (Nagata et al., 1999; Terada and Saitoh, 2018). The complete skeletons are stored at the Tochigi Prefectural Museum (TPM), Utsunomiya, Japan. The age at death was recorded in the museum inventory list, which was assessed based on tooth eruption for fawns and yearlings and the cementum annuli of the incisors and molars for adults.
Sika deer from the Kerama Islands: all individuals (N = 7) were collected as carcasses resulting from natural deaths on Kerama Islands (26°12′N, 127°24′E, Figure 1) in Okinawa Prefecture, Japan, by two of the authors (MI and TS) during their fieldwork. Sika deer were introduced to the Kerama Islands from Kyushu ca. 400 years ago, possibly from Kagoshima Prefecture, mainland Kyushu, Japan (Okinawa Prefectural Board of Education, 1996), and inhabit subtropical evergreen broadleaved forests. These deer exhibit the phenotypic characteristics of insular dwarf deer (similar to Yakushima Island deer), including a smaller body size than the possible source population of Kagoshima (Okinawa Prefectural Board of Education, 1996); thus, they are also regarded as a subspecies of sika deer (C. nippon keramae) (Ohtaishi, 1986). Most individuals were complete skeletons, but some bone elements were lost at the time of collection in the field for some individuals. All the specimens are stored at the Faculty of Science, University of the Ryukyus (URB), Nishihara, Japan. The age at death was recorded in the inventory list, which was assessed based on tooth eruption for fawns and yearlings and the cementum annuli for adults. However, no reliable age at death was obtained for individual URB-MAM-34.
2.1.2 Fossil deer populations
Cervus astylodon (femur N = 6, tibia N = 5) and Muntiacini gen. et sp. indet. (the Ryukyu muntjac, femur N = 4, tibia N = 2): these fossil deer species were excavated from the Hananda-Gama Cave site (26°8′7″N, 127°45′32″E, Figure 1) in the southern part of Okinawa Island (Okinawa Prefectural Museum and Art Museum, 2009). These animal remains are mainly isolated materials consisting of limb bones and teeth and are considered to have fallen into the cave through fissures or sinkholes following the decomposition of the carcasses (Okinawa Prefectural Museum and Art Museum, 2009). The detailed geological age of these fossils is not clear due to a lack of suitable specimens for radiocarbon dating. However, based on radiocarbon dating at other sites that have yielded fossils of these species, the estimated geological age of both species is the latest Pleistocene (>20 ka, Okinawa Prefectural Museum and Art Museum, 2009). C. astylodon is a typical insular dwarf due to its very small body size and shortened limbs (van der Geer et al., 2010). The Ryukyu muntjac was formerly referred to as Dicrocerus sp. (van der Geer et al., 2010), which is a genus known mostly from Miocene Europe. However, the finding that the antlers have a clear burr and grow from the posterior parts of the frontals oriented backward clearly indicates that it does not belong to this genus (Gertrud Rößner, personal communication to MOK). Rather, it appears to be closely related to Muntiacus based on the presence of tusk-like canines and a lack of antlers in the females, neither of which are found in Dicrocerus (Azanza et al., 2011). A full revision of this species is required, including a detailed description and comparisons with other fossil and extant taxa. At this moment, we lack reliable information on its ancestry and the degree of dwarfism, though the estimated body mass for this species implied that its body size was comparable to that of the extant continental muntjac species. In this paper, we present it as Muntiacini gen. et sp. indet. and refer to it as “the Ryukyu muntjac.” All examined specimens are stored at the Okinawa Prefectural Museum and Art Museum (OPM), Naha, Japan.
Sinomegaceros yabei (femur N = 2, tibia N = 2): this fossil deer had a gigantic body size comparable to that of an Irish elk (Megaloceros giganteus) (Okumura et al., 2016; Taruno et al., 2017). The skeletal remains were excavated from the Kumaishi-do Cave site (35°45″N, 137°′00″E, Figure 1) in Hachiman-cho, Gujo City, Gifu Prefecture, central Japan. Most of the animal remains obtained from this site are isolated materials, but an incomplete skeleton that includes the skull has also been discovered (Okumura et al., 2016). The geological age of these fossils is the late Pleistocene based on radiocarbon dating of the bones of cervids and proboscideans (Okumura et al., 1982). All sampled specimens are stored at the Osaka Museum of Natural History (OMNH), Osaka, Japan.
Detailed information on the extant and fossil specimens is provided in Supplementary Tables S1–S3.
2.2 Thin sectioning and X-ray computed tomography (CT) scanning
We applied histological analyses of long bones to infer life history traits (Klevezal, 1996; Köhler and Moyà-Solà, 2009; Köhler et al., 2012; Marín-Moratalla et al., 2013; Kolb et al., 2015a; Amson et al., 2015; Kolb et al., 2015b). We used 62 extant and 21 fossil long bones in the histological analyses (Table 1). All specimens were then photographed and standard measurements were taken, following which thin sections of the midshafts of the long bones were prepared as described previously (Chinsamy and Raath, 1992; Sander, 2000). We produced 83 thin sections (62 sections for extant species and 21 sections for extinct ones; see also detail in Supplementary Tables S1–S3). The thin sections were photographed using a digital film scanner (Pixus Mp 800, Canon) and analyzed using an Optiphot2-pol microscope (Nikon). Microscopic photographs were finally taken with a Nikon Df camera.
Neonatal specimens of sika deer (mainland Honshu: CBM-ZZ-757) and Reeves’ muntjac (CBM-ZZ-4974) were scanned using an experimental animal X-ray CT scanner (Latheta LCT-200, Aloka; 24-μm resolution, 80 kV, 0.2 mA) at Okayama University of Science, Okayama, Japan, to determine the initial diameter of the cortical bone and open medullary cavity in these species. Afterward, image segmentation and visualization were conducted using VG-Studio Max (Volume Graphics) version 3.1.
2.3 Age assessment
To compare growth patterns, we estimated the ages as follows: for most of the extant deer samples, age at death was recorded in the museum inventory lists, which were assessed using tooth eruption or the number of dental cementum annuli in the tooth root (Supplementary Tables S3, S4). However, for the fossil deer samples, the long bones were unassociated with skulls and mandibles. Therefore, we estimated the age at death from the number of LAGs in the long bones. Several studies have reported a good correlation between the number of LAGs and the age at death in several vertebrate groups (Castanet et al., 2004), while other studies indicated the limitation of LAGs as reliable ages due to a loss of LAGs by bone remodeling and/or the expansion of the medullary cavity (e.g., Jordana et al., 2016; Miszkiewicz and van der Geer, 2022). A study examining this correlation in extant deer species [red deer (Cervus elaphus)] reported that although the number of LAGs in the tibia corresponded to the actual age, the number in the femur corresponded to the age before deposition of the EFS (Calderon et al., 2019). Therefore, the relationship was examined first using extant deer between the actual age at death and the number of LAGs in bones (see Supplementary Information for details). The results showed that the estimated age of the extant deer based on the number of LAGs, including those that appeared in EFS in both the femur and tibia, was highly correlated with the actual age determined using the tooth cementum annuli (Supplementary Figure S6; Supplementary Table S4). Furthermore, while some old adults >8 years of age (HOUMVC-00037, CBM-ZZ-412, and URB-MAM-193) had fewer LAGs in their long bones than their actual age, the diameters of their expanded medullary cavities were identical or even larger than those in neonates and fawns (<1 year old), suggesting that the first (and also the second in URB-MAM-193) LAG was eliminated in old individuals (Supplementary Table S4). Thus, since the number of LAGs matched the actual age of extant sika deer, we estimated the age of individuals in the fossil deer by counting the number of LAGs in their long bones (Supplementary Table S5). Secondary bone remodeling, which had erased any evidence of the primary bone tissue, including LAGs, was seen only at the inner medullary surface and part of the cortex where ligaments were strongly attached (e.g., the labium laterale in the femur) in adults, indicating good preservation of the primary bone tissues in most parts of the cortex.
2.4 Body mass estimation
Body mass during ontogeny was estimated from the bone diameters at the LAGs (Lehman and Woodward, 2008). Body mass estimation formulas for both the femora and tibiae were obtained and the formula using the mediolateral diameter (MLD) of the femora and tibiae was applied in this study (see Supplementary Information for details). In addition to the diameters at the LAGs, external diaphysial measurements [anteroposterior diameter (APD) and MLD] were collected and used to estimate the body mass at death. The raw measurement data of the diameters at the LAGs, with the estimated body mass used for growth curve modeling, are presented in Supplementary Table S13 (provided separately in Excel format).
2.5 Comparison of growth patterns
Growth curves of body mass were constructed for each specimen that was older than yearlings (i.e., with >1 LAG) that had at least four data points, including start and end points. The exception was the extant Reeves’ muntjac, for which a fawn and a yearling were included in the growth curve fitting because this species reaches maturity early in life (Chiba Prefecture, 2007). Before fitting the growth curves, neonatal body mass data were obtained for both the extant and fossil taxa as the starting point (Supplementary Table S6; Supplementary Figure S7).
To fit the growth curves to the obtained data, the number of LAGs was first transformed to age in years. For each specimen, the body mass (W, in kg) was then formulated with age (x, in years) using the Gompertz curve, as described below:
where a, b, and c represent the asymptote (i.e., the body mass when body growth ceases), growth rate, and inflection point, respectively. The Gompertz curve fitting was rationalized as this model showed the highest goodness of fit, as represented by the lowest AICc among the fitted growth curves (Supplementary Table S7). Furthermore, the growth rate (b) and inflection point (c) were statistically compared among species/populations. First, the normality of the parameter distribution was confirmed by Shapiro–Wilk tests (for each species/population, p > 0.05). We then compared the growth rate and inflection point between the femur and tibia using extant deer samples by paired t-tests. We found that the null hypothesis of no difference in these parameters between the femur and tibia was not rejected (for the growth rate, N = 15, t = 0.10, d.f. = 14, p = 0.92; for the inflection point, N = 15, t = 0.60, d.f. = 14, p = 0.56). Therefore, to increase the sample size, we combined data from the femur and tibia for fossil samples, although further data are needed to clarify the difference in growth curves between the femur and tibia. We conducted a one-way ANOVA and subsequent multiple comparisons using the Tukey–Kramer method to examine differences in growth parameters among the eight species/populations, averaging the growth curve parameters from the femur and tibia for the extant species to avoid repeated sampling from the same individual. Additionally, a two-way ANOVA using bone type (i.e., femur or tibia) and species/populations as factors were conducted. The growth curve parameters were averaged for each population/species to produce a representative growth curve for each group. All statistical analyses were conducted in JMP Pro 16.0 (SAS Institute Inc.).
2.6 Qualitative assessment of bone histology and external fundamental system (EFS) observation
To corroborate the findings derived from the estimated growth curves, we also investigated bone histology in detail. We observed the same histological sections that were used for the growth curve modeling. The nomenclature and definitions of the bone tissues were based on those described by Francillon-Vieillot et al. (1989) and Castanet et al. (1993). We focused on the following bone tissue characteristics: 1) the type of primary periosteal bone tissue and vascularization pattern as an indicator of the relative bone growth rate and 2) the presence of an external fundamental system (EFS). Primary periosteal bone tissue is known as a fibro-lamellar (FBL) bone, which has a high level of laminar to plexiform vascularization (PV), which is an indicator of fast growth, whereas parallel-fibered (PF) bone tissue is formed during periods of slower growth (e.g., de Margerie et al., 2002). The growth rate is correlated with intensity and vascularization patterns (Castanet et al., 2000; Lee et al., 2013; Orlandi-Oliveras et al., 2018), in which lower levels of vascularization associated with the longitudinal orientation of canals indicated a slower bone depositional rate. Additionally, the EFS is characterized by PF bone tissue without vascularization, thereby forming an edge of slow-growing bone tissues as a sign of somatic maturity (e.g., Woodward et al., 2013; Andrade et al., 2015; Jordana et al., 2016). By counting LAGs within the EFS, we estimated the ages at which EFS formation started (see Supplementary Information for details). In addition, somatic maturity was investigated based on the fusion status of both the proximal and distal epiphyses. Finally, we conducted correlation tests between the age at which EFS development began and the inflection point (c), which marks the transition from the initial phase of exponential growth to the subsequent phase of asymptotic growth and is correlated with sexual maturity in laboratory-reared mice (Monteiro and Falconer, 1966). For this purpose, we used the median EFS developmental age for each specimen. For example, OPM-HAN06-588 (Cervus astylodon) had a range of 9–10 years; therefore, we used the value of 9.5 years as the age at which EFS development started. In the extant specimens, we averaged the inflection point obtained from the femur and tibia growth curves. Non-parametric Spearman’s rank correlation coefficient (ρ) was calculated in three datasets (extant only, fossil only, and all individuals combined).
2.7 Comparison of survivorship curves
Because a change in mortality patterns also represents a change in life history, we estimated survivorship curves for both extinct and extant deer populations. We obtained age data for the three extant sika deer populations used in the histological investigations either from published papers or by examining the cementum annuli of the incisor roots: mainland Hokkaido (N = 1,060, Hokkaido Institute of Environmental Sciences, 1997), mainland Honshu (N = 594, Ozaki et al., 2010), and Yakushima Island (N = 74, cementum annuli of museum specimens). Histological analysis of the cementum layers of the lower first incisors was conducted on Yakushima Island deer specimens housed in the University Museum of the University of Tokyo, the Tochigi Prefectural Museum and the Hokkaido University Museum to determine their age at death. This analysis was performed by a specialized laboratory (Matson Laboratory, Montana, USA), which provided age data with high reliability. The age data from three populations were based on randomly culled individuals, so they were considered indicative of the age structure of actual extant populations. Because the hunting of female deer was legally prohibited until 1994 and these deer populations were increasing in number at the time of culling (approximately from the late 1990s to the early 2000s), the deer populations were less affected by hunting pressure (Ozaki et al., 2010). Additionally, we obtained mortality data of sika deer from Kinkazan Island from published literature (N = 264, Kubo et al., 2011). The Kinkazan population is protected for religious reasons and thus free from hunting pressure, which was also the case for C. astylodon and the Ryukyu muntjac inhabiting the Pleistocene Okinawa Island without humans and other predators (Kubo et al., 2011; Kubo et al., 2015). The age data for extant Reeves’ muntjacs from England were obtained from a published reference (N = 84, Chapman et al., 2005).
The age data of two fossil insular deer from Okinawa Island were also obtained from published references (C. astylodon: N = 45, Kubo et al., 2011; Ryukyu muntjac: N = 65, Ozaki, 2009). Kubo et al. (2011) estimated the age at death of C. astylodon based on the height of the lower third molar by applying the molar wear rate of extant sika deer. The variability of the age estimation based on the molar wear rate was tested and discussed previously (Ozaki et al., 2010; Kubo et al., 2011; Kubo and Fujita, 2021). Thus, we adopted the most appropriate age estimates. Ozaki (2009) estimated the age at death of the Ryukyu muntjac by applying the molar wear scoring method developed by Chapman et al. (2005) for extant Reeves’ muntjac. Fossil S. yabei and extant Kerama Islands deer were excluded from these survivorship curve comparisons due to a lack of reliable age data.
To depict the survivorship curves for three culled sika deer populations, we constructed life tables as previously described (Caughley, 1977), assuming stationary age distributions and used probit regression to smooth the age distributions. Age data for the other populations (Kinkazan sika deer, Reeves’ muntjac, and two fossil insular deer) were based on natural deaths; therefore, the age class frequency was divided by the sample size to obtain a multiple of the frequency of mortality (dx). Life tables were then derived from this mortality frequency (Caughley, 1977). The survivorship curves were presented with an initial value of 1,000 individuals.
The observed age class frequencies and derived life tables for each deer population are presented in Supplementary Tables S11, S12.
For more details on the materials and methods used in this study, see the Supplementary Information and Supplementary Figures and the Supplementary Tables therein.
3 Results
3.1 Growth curve models and growth rate comparison using skeletochronology
The LAGs in the long bones of both extant and fossil deer were well-preserved (Supplementary Figures S1–S4), allowing us to measure the bone diameters at the LAGs. A growth curve for each species/population is presented in Figure 2A and Supplementary Figure S10. Among the sika deer populations, deer from the two mainland areas (Honshu and Hokkaido) showed faster growth than those from the two insular populations (Yakushima and Kerama Islands). The growth trajectories of body masses also corresponded with those obtained from investigations of culled individuals (Ochiai and Asada, 1995; Suzuki et al., 2001) and through long-term field observations of wild deer (Agetsuma and Agetsuma-Yanagihara, personal communication), thereby validating our estimation method of body growth from skeletochronology. The extant Reeves’ muntjac showed the fastest growth, which was also reported in an analysis of culled individuals that showed early sexual maturity at ca. 6 months (Chiba Prefecture, 2007).
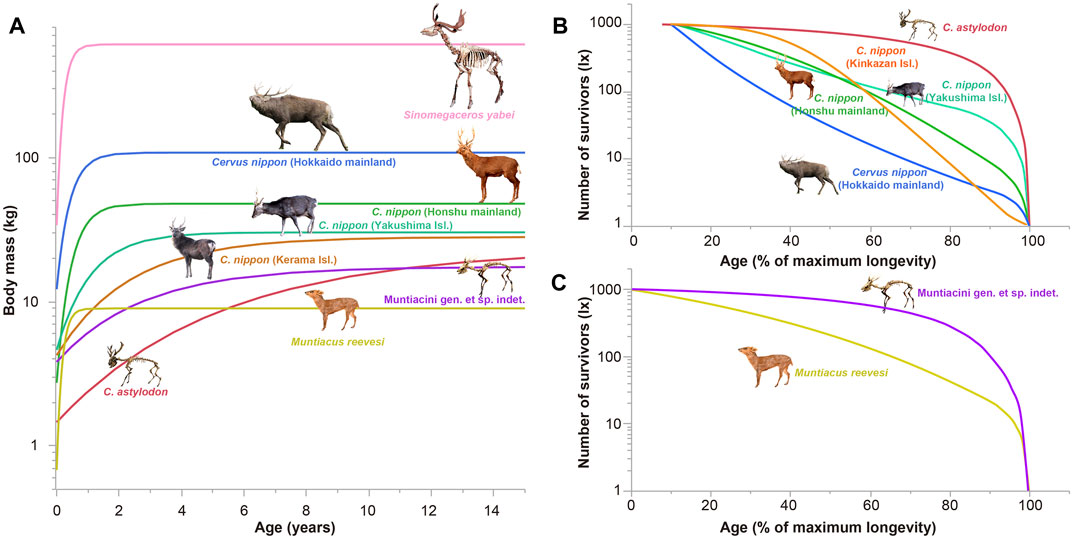
FIGURE 2. Growth (A) and survivorship curves (B, C) for the studied Japanese deer. The insular deer have a significantly slower growth rate and a delayed growth plateau (somatic maturity) compared with the mainland deer (A), and an ecological shift exists in the mortality patterns between the insular and mainland deer (B, C). As the age estimation of C. astylodon was based on the height of the lower third molar, we confined the survivorship curves to juvenile and onward for C. astylodon and sika deer (B).
Compared to the extant references, two fossil insular deer species (C. astylodon and the Ryukyu muntjac) showed remarkably slow and prolonged growth, whereas the Pleistocene giant deer (S. yabei) showed fast growth, which suggested a growth pattern similar to other extant large deer species such as moose (Sand et al., 1995).
Statistical comparisons of growth parameters revealed that both fossil and extant insular deer had significantly slower growth rates than those of Reeves’ muntjac and S. yabei (both p < 0.05, Supplementary Tables S9, S10). However, the growth rates of the four extant sika deer populations and fossil insular deer did not differ significantly from each other (p > 0.05, Supplementary Tables S9, S10) due to the transitional position of extant insular deer, although the limited numbers of samples may also play a role in this observation.
3.2 Variation in bone tissue among deer populations
The histological characteristics of long bones corroborated the statistical comparisons of the growth curves. Figure 3 summarizes the main histological features of the examined deer (also see Supplementary Tables S1–S3).
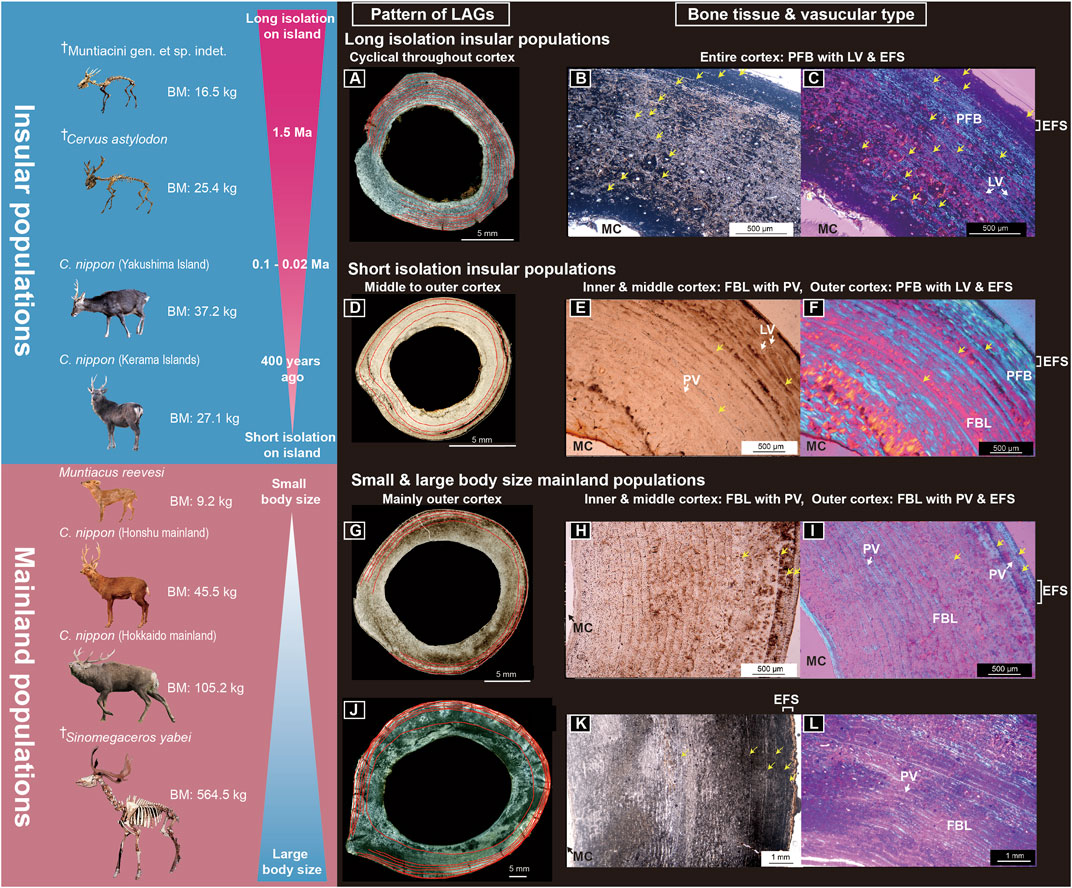
FIGURE 3. Bone histological features of the studied Japanese deer. (A–C) Midshaft cross-section (A), cortex under normal light (B), and cortex under polarized light (C) of a femur of fossil Cervus astylodon (OPM-HAN07-1603). (D–F) Midshaft cross-sections (D), cortex under normal light (E), and cortex under polarized light (F) of a femur of an extant sika deer (Cervus nippon) from Yakushima Island (TPM-M-313). (G–I) Midshaft cross-section (G), cortex under normal light (H), and cortex under polarized light (I) of the femur of an extant sika deer from the Honshu mainland (CBM-ZZ-412). (J–L) Midshaft cross section (J), cortex under normal light (K), and cortex under polarized light (L) of the femur of a fossil Sinomegaceros yabei (OMNH-QV-4062). The red lines in (A, D, G, J), and the yellow arrows in (B, C, E, F, H, I, K) indicate the lines of arrested growth. The outer cortex is at the upper right of (B, C, E, F) and on the right in (H, I, K). BM, body mass; EFS, external fundamental system; FBL, fibro-lamellar bone tissue; LAG, line of arrested growth; LV, longitudinal vascular canal; MC, medullary cavity; PFB, parallel-fibered bone tissue; PV, plexiform to the laminar vascular canal. ✝Fossil cervids. Note: only fossil deer from Okinawa Islands had a distinctly longer-term geographical isolation history and exhibited bone tissue, indicating a slower growth rate (de Margerie et al., 2002).
As observed, all extant deer populations (i.e., the four extant sika deer populations and the extant Reeves’ muntjac), including the gigantic fossil S. yabei, exhibited a similar bone tissue structure to continental cervids (Kolb et al., 2015a; Jordana et al., 2016; Calderon et al., 2019), consisting of a fast-growing FBL bone with PV in most parts of the cortex (Figures 3G–L; Supplementary Figures S2A–I, S3G–I, S4A–O). Additionally, slow-growing PF bone tissue was observed in the outer cortex. This PF bone tissue was more extensively and notably developed in the two insular populations (sika deer from Yakushima and Kerama Islands), with tighter LAGs at the inner to middle cortex compared to those in the mainland deer (Figures 3D–F, Supplementary Figures S2D–F, S3G–I, J–L), suggesting a slower growth rate among insular populations. Furthermore, the cortical bone tissues of two fossil insular deer (C. astylodon and Ryukyu muntjac) differed from those of extant deer and gigantic fossil deer (S. yabei) in that they were primarily PF bone tissue with multiple LAGs, implying a much slower growth rate than that of other deer populations. Their bone tissues were also similar to those of the extinct dwarf bovid (Myotragus balearicus) and typical extant reptiles (e.g., Woodward et al., 2014). Moreover, although the LAGs were spaced evenly throughout the cortex (Figures 3A–C; Supplementary Figures S1A–C, S3A–F), they became closer as they approached the periosteal surface in older individuals, which indicated a decrease in growth rate with age. Only a few areas of the innermost cortex showed a prevalence of FBL bone tissue with primary osteons, which alternated with nonvascular PF bone tissue with LAGs, suggesting that the bone was deposited slowly during most periods of the lifetime. The slow growth rate was further supported by the observed vascularization pattern. Our fossil insular deer samples showed less vascularization, and their vascular canals were oriented longitudinally, which is not observed in other cervids and large mammals (Klevezal, 1996; Kolb et al., 2015a). In summary, the bone histological features of the fossil insular deer supported their slow growth rates.
The results also showed that all extant specimens with EFS had fused epiphyses both in the femora and tibiae (Supplementary Figure S5; Supplementary Table S3). As observed, the proximal epiphyses of the tibiae and femora fused later than the distal epiphyses. Moreover, the EFS developed contemporaneously with proximal fusions. In two mainland sika deer populations, the timing of EFS development and proximal epiphyseal fusion showed a good correspondence with the age when the rate of growth decelerated (Supplementary Figures S8, S9; Supplementary Table S3), including the reported age of somatic maturity in the analyses of culled individuals (Ochiai and Asada, 1995; Suzuki et al., 2001). Notably, the extant sika deer from the Kerama Islands also showed delayed EFS development and epiphyseal fusions compared with other investigated deer populations. Based on the observation of extant specimens, we further investigated the relationship between EFS development and epiphyseal fusion in fossil specimens (Supplementary Figure S5; Supplementary Tables S1, S2). Although most of the fossil specimens did not show preservation of both proximal and distal epiphyses, all specimens with fused proximal epiphyses had EFS and vice versa. Therefore, the fossil species also showed correspondence between epiphyseal fusion and the EFS. The findings revealed that two insular extinct deer showed much delayed EFS development (min. 9 years, max. 16 years for C. astylodon; min. 5 years, max. 11 years for the Ryukyu muntjac, see Supplementary Tables S1, S2) than other investigated deer populations, suggesting delayed somatic maturity.
The histological observation of delayed somatic maturity was further corroborated by the growth curve parameter. We observed a significant positive correlation between the inflection point and the age at which EFS development started (extant only, ρ = 0.87, p = 0.0012; fossil only, ρ = 0.84, p = 0.0003; all data combined, ρ = 0.91, p < 0.0001; Supplementary Figure S11). Although the inflection point was less than the actual age at which the body growth deaccelerated and the age at which EFS development started, the significant positive correlation indicated that the higher inflection point values can be used as an indicator of delayed somatic maturity. Statistical comparison of inflection points among the studied deer showed a significantly higher inflection point in C. astylodon than in other deer populations (p < 0.05, Supplementary Tables S9, S10), implying again the delayed sexual maturity in this species.
3.3 Survivorship curve comparisons among extant and fossil deer
To clarify life history differences in mortality patterns, we compared the survivorship curves of fossil C. astylodon and four other extant sika deer populations from mainland Hokkaido, mainland Honshu, Yakushima Island, and Kinkazan Island (Figure 2B). Because the age estimation of C. astylodon was based on the height of the lower third molar, we restricted the survivorship curves for C. astylodon and sika deer to juveniles and older. The low mortality from the juvenile through to the prime age period characterized the survivorship curve of C. astylodon, followed by increased mortality during senescence, which appeared as an inverted L and was categorized as a type I survivorship curve (Pianka, 1978), including a characteristic of slow-living species. At the other extreme, extant sika deer from mainland Hokkaido showed relatively high mortality in the younger age class, which was followed by a more gradual decrease in the number of survivors from the prime age to senescence, thereby serving as a characteristic of type II survivorship curves. The results also showed that the survivorship curves of sika deer from mainland Honshu and Yakushima Island were located between those of C. astylodon and the Hokkaido sika deer, with a steady decrease in survivorship until the prime age, although the Honshu population showed increased mortality after the prime age. Moreover, although the survivorship curve of the Kinkazan Island deer clustered with that of the Honshu and Yakushima Island populations, it showed a lower mortality rate in the initial phase and a higher rate in the later phase. This slight difference in the pattern may be associated with the lack of hunting pressure on the Kinkazan population. However, the intensity of hunting in sika deer does not strongly affect overall survivorship curves, as described in the Methods section. Furthermore, although Kinkazan deer inhabited the predator-free island, their mortality pattern differed from that of C. astylodon, implying a further advanced insular effect in the latter. A previous study reported a maximum observed age of 25 years for C. astylodon (Kubo et al., 2011), which was greater than that of the extant sika deer (17, 19, 17, and 21 years for the Honshu, Hokkaido, Yakushima, and Kinkazan Island populations, respectively; Supplementary Tables S11, S12).
We also compared survivorship curves between the Ryukyu muntjac and the extant Reeves’ muntjac (Figure 2C). The extant muntjac had a type II survivorship curve, as did the extant sika deer from mainland Honshu. Similarly, the Ryukyu muntjac had a type I survivorship curve, indicating that its mortality rate did not increase until senescence, thereby also implying a strong insular effect on life history traits in Okinawa Island.
4 Discussion
The results of the interdisciplinary analyses of a growth rate comparison, histological examination, and survivorship curve analysis of both extant and extinct deer species supported our expectations that the evolution of a new combination of life history traits amounts to the repeated establishment of a “slow life” in insular environments. Although the sample size of deer for each population was not very large, our findings are among the first to clarify the gradual shift to slow life histories for insular forms, placing the two fossil insular deer populations from Okinawa Island at the slow end of the fast-slow continuum.
The ecological processes of life history change can be inferred from the current dataset of deer populations. As observed in mainland deer populations, any modification of life history traits happened in mainland populations (Hokkaido and mainland Honshu sika deer, Reeves’ muntjac, and S. yabei), similar to the condition of their continental counterparts (Kolb et al., 2015a). The Japanese archipelago is considered an “island” in the literature (e.g., van der Geer et al., 2010). However, from the current dataset, Japanese mainland deer are not experiencing a strong insular effect on life history traits. However, further investigation of sika deer populations on the Asian continent will shed light on the degree of insularity for the Japanese mainland sika deer.
We hypothesize a process of life history evolution on Japanese island deer as follows: the first step can be exemplified by sika deer on the Kerama Islands. As observed, the bone histological features showed signs of slow growth and accompanied the smallest body size and slowest growth rate among understudied extant deer populations. These deer were introduced to the Kerama Islands from the Kyushu mainland by humans ca. 400 years ago (Okinawa Prefectural Board of Education, 1996). Fast changes in body size in <6,000 years have also been documented previously in red deer from Jersey (Lister, 1989). Furthermore, domestic cattle introduced to Amsterdam Island experienced a rapid shrinking of body size in ca. 110 years (Berteaux and Micol, 1992; Rozzi and Lomolino, 2017). Although changes in growth trajectories might exist, they were not shown in these interesting populations. For the Kerama deer, this drastic change in growth trajectory is proposed to have occurred within 400 years, which equates to approximately 80 generations (assuming a generation time of 5 years for the sika deer). The change in the growth trajectory of the Kerama deer is, therefore, proposed to have been brought about by phenotypic plasticity rather than a genetic modification in response to the less abundant understory vegetation in the subtropical evergreen broadleaved forest, accounting for the poor nutritional status of the Kerama deer (Shiroma et al., 1996), which was supported by the observation of a possible malnourished individual (URB-MAM-55; see Supplementary Information for details). Lister (1996) originally hypothesized the importance of phenotypic plasticity in the initial stage of insular dwarfism; underlying this would be epigenetic mechanisms controlling body growth, which respond to habitat environments (Price et al., 2003; Lafuente and Beldade, 2019). These mechanisms are a challenging theme in the study of insular evolution. Another topic to be clarified in the Kerama deer is the life history shift observed in its demographic aspect. Reliable mortality data for the Kerama deer are required for this purpose.
The Yakushima Island deer illustrates the next step in life history evolution. This population also showed a slower growth rate and intermediate histological features, coupled with a moderate shift in survivorship curves toward those of slow-life species. Contrary to the situation of the Kerama deer, the slower growth rate of Yakushima deer may have a genetic basis because: 1) Yakushima deer are not malnourished and have shown a recent population increase; and 2) some of their macromorphological features have a genetic basis (Terada et al., 2012; Terada and Saitoh, 2018). Long-term field observations of the Yakushima deer also supported our finding that life history traits of this population are shifting toward a slow life (Agetsuma and Agetsuma-Yanagihara, personal communication).
The clearest evolutionary transformation was shown in the two fossil deer species isolated from Okinawa Island over 1.5 Ma. Their growth patterns, bone histological characteristics, and mortality patterns differed from those of other deer populations. As with the Kerama deer, phenotypic plasticity may have partially influenced their growth trajectories. However, we currently have no reliable sources of information on the nutritional status of the fossil deer, except for their dietary habits (Kubo et al., 2015; Kubo and Fujita, 2021). Nevertheless, there are reports of healed bone fractures on several leg bones from a museum collection of fossil insular deer species on Okinawa Island have been recorded, which implies that this deer survived long enough to recover from severe leg injuries. These findings refute the possibility that fossil Okinawa deer were malnourished and that their body growth was suppressed due to a lack of resources. The same trend was also observed for the fossil deer and muntjac, further supporting the shift toward slow life as an adaptation to the insular environment. This process is proposed to have started after their initial settlement on Okinawa Island, in part through phenotypic plasticity of the growth trajectory due to a lack of sufficient foods during growing periods. A life history change would then have become genetically fixed through the natural selection of more slow-life individuals, which occurred in the Yakushima Island deer. The outcome would be the development of extremely slow-life animals, despite their small body size. Unfortunately, this life history trait makes these species vulnerable to human exploitation, as animals with slow life histories have overall lower population recruitment compared to fast-life animals, and their population recruitment is most affected by increased adult mortality (Pianka, 1978; Oli, 2004). Consequently, the two Okinawa deer species became extinct at the time of or soon after the Paleolithic human arrival to the Okinawa Islands (Fujita et al., 2014; Fujita et al., 2016), probably due to hunting. This extinction applies to other island dwarfs that became extinct during the Pleistocene era (Alcover et al., 1998; Sondaar, 2000; but see Louys et al., 2021). Recently, Rozzi et al. (2023) analyzed body size changes and the extinction risks of >1,500 island mammals worldwide and clarified that insular dwarfs and giants are susceptible to extinction by the arrival of modern humans. Thus, behind extinction could be a life history shift toward the slow life in these insular extreme forms.
Our findings also agreed with worldwide observations of life history changes in insular mammals (Figure 4). A living cervid (Odocoileus hemionus) from Blakely Island showed slower growth rates than those from the mainland population (Long et al., 2019). As the tooth eruption was delayed in the Blakely Island deer, this life history change may have a genetic basis, like the case of the Yakushima Island deer. With longer isolation but variable island sizes, the life history response varies among mammals from Mediterranean islands. A fossil dwarf hippo (Hippopotamus minor) from Cyprus showed no evident modification in bone histology (Kolb et al., 2015b), although their life history traits remained unclarified. In contrast, a fossil cervid (Candiacervus) from Crete showed slight modifications toward a slower life history (Kolb et al., 2015a; Miszkiewicz and van der Geer, 2022). The Sicilian pygmy elephant (Elephas falconeri) also showed a modification in its life history. This species showed a fast-life history as the age distribution of its fossil assemblage was skewed toward calves and immature individuals (Raia et al., 2003). Nevertheless, a histological study suggested that this pygmy elephant had a much slower growth rate than its mainland relatives, with sexual maturity within the range of extant elephants (Köhler et al., 2021). This contradiction requires further synthetic study of this species. The differences in life history responses among Mediterranean mammals, however, are unsurprising, given that these Mediterranean islands were much bigger than those for which we report life history changes (i.e., Blakely Island, Kerama Islands, Yakushima Island, Okinawa Island, and Mallorca Island). The most explicit change in life history was observed in Myotragus balearicus from Mallorca Island, which underwent an exceptionally long period of isolation (5.2 Ma) in predator-free environments. Myotragus showed a dramatic decrease in bone growth rate and an evolution toward a slow life history (Köhler and Moyà-Solà, 2009; Jordana et al., 2012; Marín-Moratalla et al., 2013), implying the commonality of this evolutionary change in the Okinawa fossil deer. These results propose that both island size and isolation duration -with smaller islands and longer isolation- strongly affected the degree of modification in life history, thereby resulting in life history changes toward the slow life.
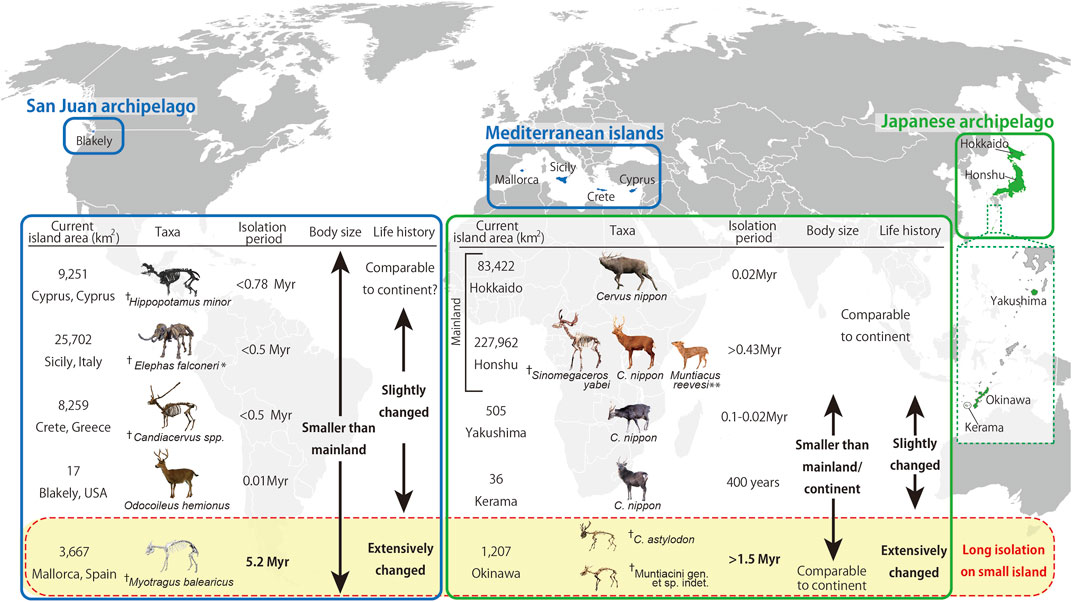
FIGURE 4. Diagram summarizing the evolutionary processes and the relationship between insularity, body size, and life history in extant and fossil large mammals. The present study investigated cervid species/populations of the Japanese Archipelago, while information on animals from other islands were obtained from other sources: Odocoileus hemionus (Long et al., 2019), Hippopotamus minor (van der Geer et al., 2010), Candiacervus spp. (van der Geer et al., 2010), Elephas falconeri (Palombo, 2001; van der Geer et al., 2010), and Myotragus balearicus (Köhler and Moyà-Solà, 2009). Photographs of fossil mammals in Mediterranean islands courtesy of Gunma Museum of Natural History (Elephas falconeri), Alexandra van der Geer (Hippopotamus minor and Candiacervus spp.), and Meike Köhler (Myotragus balearicus). ✝Fossil taxa. * The life history of Elephas falconeri is disputed (Raia et al., 2003; Köhler et al., 2021). ** Muntiacus reevesi was an artificially introduced animal in the 1980s. Therefore, the isolation period of >0.43 Myr is not applicable (Chiba Prefecture, 2007).
5 Conclusion
The extant Japanese sika deer showed modification of their life history traits through a combination of phenotypic plasticity and natural selection processes. This ability underlies the process of insular dwarfism, which has also been recorded in fossil deer species (C. astylodon and the Ryukyu muntjac) from Okinawa Island, which was estimated to have a slow life history. The combination of results from the present and previous studies on the life histories of insular mammals indicates that both island size and isolation duration strongly affect the degree of modification in life history traits, with longer isolation on smaller islands resulting in more prominent life history changes. We also demonstrated that the evolution of a gradual and transitional shift toward a slow life history in large mammals from the mainland to insular populations was the main life history change accompanying insular dwarfism, which makes insular dwarfs vulnerable to human exploitation.
Data availability statement
The datasets presented in this study can be found in online repositories. The names of the repository/repositories and accession number(s) can be found in the article/Supplementary Material.
Ethics statement
Ethical review and approval were not required for the animal study as this study used museum specimens, the investigations of which were officially approved by the curators.
Author contributions
SH, MOK, and MF designed the research. SH, HT, and TN prepared bone histological sections, took measurements, and created visualizations. MOK, MI, and TS collected ecological data. MOK conducted statistical analyses. SH, MOK, MS-V, and MF drafted the original manuscript. All authors also contributed to the manuscript reviews and edits and approved the final manuscript.
Funding
This work was supported by grants from the Sanyo Broadcasting Foundation (to SH and MOK) and JSPS KAKENHI Grant Nos. JP16K18615 (to MOK), JP18H05254 (to TN), and JP19K04060 (to SH and MOK). MS-V is supported by Swiss SNF 31003A_169395. This work was supported in part by JST-CREST Grant No. JPMJCR2194 (to TN).
Acknowledgments
We thank the following curators for access to the museum collections under their care: Shimoinaba, S. (Natural History Museum and Institute, Chiba, Japan), Hayashi, T. (Tochigi Prefectural Museum), Eda, M. (The Hokkaido University Museum), and Usami, K. (Okinawa Prefectural Museum and Art Museum). We also acknowledge Oshiro, I. for his advice on the fossil Okinawa deer and support during this study. Furthermore, we thank Ochiai, K. and Shimoinaba, S. for providing ecological data on sika deer and Reeves’ muntjac in Honshu. We appreciate that Agetsuma, N. and Agetsuma-Yanagihara, Y. shared data from their long-term field survey of Yakushima deer. We are also grateful to Nakamura, K. and Nomura, H. for technical assistance in thin-sectioning and Kodaira, S. and Kodama, R. for CT scanning. We thank Kawada, S. (National Museum of Nature and Science, Tokyo), van der Geer, A. (Naturalis Biodiversity Center), Köhler, M. (ICP), Takakuwa, Y. (Gunma Museum of Natural History), Kikuchi, H. and Umemura, Y. for providing photos of Odocoileus hemionus, Hippopotamus minor, Candiacervus spp., Myotragus balearicus, Sinomegaceros yabei, Elephas falconeri, and sika deer (Yakushima Island and Hokkaido mainland, respectively). We are grateful for the comments provided by Weisbecker, V. and Köhler, M. on the earlier version of this manuscript.
Conflict of Interest
The authors declare that the research was conducted in the absence of any commercial or financial relationships that could be construed as a potential conflict of interest.
Publisher’s note
All claims expressed in this article are solely those of the authors and do not necessarily represent those of their affiliated organizations, or those of the publisher, the editors, and the reviewers. Any product that may be evaluated in this article, or claim that may be made by its manufacturer, is not guaranteed or endorsed by the publisher.
Supplementary material
The Supplementary Material for this article can be found online at: https://www.frontiersin.org/articles/10.3389/feart.2023.1095903/full#supplementary-material
Supplementary Data Sheet S1 | Supplementary information on methodological details, Supplementary Tables S1–S12, and Supplementary Figures S1–S11.
Supplementary Data Sheet S2 | Raw data of LAG and external diaphysial measurements of specimens used in the histological analyses, together with estimated body mass (Supplementary Table S13).
References
Alcover, J. A., Sans, A., and Palmer, M. (1998). The extent of extinctions of mammals on islands. J. Biogeogr. 25, 913–918. doi:10.1046/j.1365-2699.1998.00246.x
Amson, E., Kolb, C., Scheyer, T. M., and Sánchez-Villagra, M. R. (2015). Growth and life history of Middle Miocene deer (Mammalia, Cervidae) based on bone histology. Comptes Rendus Palevol 14, 637–645. doi:10.1016/j.crpv.2015.07.001
Andrade, R. C. L. P., Bantim, R. A., Lima, F. J., dos Santos Campos, L., Eleutério, L. H. S., and Sayão, J. (2015). New data about the presence and absence of the external fundamental system in archosaurs. Cad. Cult. Ciência 14, 200–211. doi:10.14295/cad.cult.cienc.v14i1.932
Azanza, B., DeMiguel, D., and Andrés, M. (2011). The antler-like appendages of the primitive deer Dicrocerus elegans: Morphology, growth cycle, ontogeny, and sexual dimorphism. Estud. Geol. 67, 579–602. doi:10.3989/egeol.40559.207
Begon, M., Harper, J. L., and Townsend, C. R. (2006). Ecology: From individuals to ecosystems. 4th Edition. Oxford: Blackwell Publishing Ltd.
Benitez-Lopez, A., Santini, L., Gallego-Zamorano, J., Mila, B., Walkden, P., Huijbregts, M. A. J., et al. (2021). The island rule explains consistent patterns of body size evolution in terrestrial vertebrates. Nat. Ecol. Evol. 5, 768–786. doi:10.1038/s41559-021-01426-y
Berteaux, D., and Micol, T. (1992). Population studies and reproduction of the feral cattle (Bos taurus) of Amsterdam Island, Indian Ocean. J. Zool. 228, 265–276. doi:10.1111/j.1469-7998.1992.tb04607.x
Calderon, T., DeMiguel, D., Arnold, W., Stalder, G., and Köhler, M. (2019). Calibration of life history traits with epiphyseal closure, dental eruption and bone histology in captive and wild red deer. J. Anat. 235, 205–216. doi:10.1111/joa.13016
Castanet, J., Croci, S., Aujard, F., Perret, M., Cubo, J., and de Margerie, E. (2004). Lines of arrested growth in bone and age estimation in a small primate: Microcebus murinus. J. Zool. 263, 31–39. doi:10.1017/s0952836904004844
Castanet, J., Francillon-Vieillot, H., Meunier, F. J., and de Ricqlès, A. (1993). “Bone and individual aging,” in Bone vol 7: Bone growth. Editor B. K. Hall (Boca Raton: CRC Press), 245–283.
Castanet, J., Rogers, K. C., Cubo, J., and Boisard, J-J. (2000). Periosteal bone growth rates in extant ratites (ostriche and emu). Implications for assessing growth in dinosaurs. CR Acad Sci Paris, Sci. vie/Life Sci. 323, 543–550. doi:10.1016/s0764-4469(00)00181-5
Chapman, N. G., Brown, W. A. B., and Rothery, P. (2005). Assessing the age of Reeves' muntjac (Muntiacus reevesi) by scoring wear of the mandibular molars. J. Zool. 267, 233–247. doi:10.1017/s0952836905007405
Chiba Prefecture (2007). Chiba prefecture, deer research group on boso: Science report on emergent survey of invasive alien species (Reeves’ muntjac) in Boso. Chiba: Chiba Prefecture.
Chinsamy, A., and Raath, M. A. (1992). Preparation of fossil bone for histological examination. Palaeontol. Afr. 29, 39–44.
de Margerie, E., Cubo, J., and Castanet, J. (2002). Bone typology and growth rate: Testing and quantifying ‘amprino’s rule’ in the mallard (Anas platyrhynchos). Comptes Rendus Biol. 325, 221–230. doi:10.1016/s1631-0691(02)01429-4
Erickson, G. M., Curry Rogers, K., Varricchio, D. J., Norell, M. A., and Xu, X. (2007). Growth patterns in brooding dinosaurs reveals the timing of sexual maturity in non-avian dinosaurs and Genesis of the avian condition. Biol. Lett. 3, 558–561. doi:10.1098/rsbl.2007.0254
Erickson, G. M. (2014). On dinosaur growth. Annu. Rev. Earth Planet. Sci. 42, 675–697. doi:10.1146/annurev-earth-060313-054858
Francillon-Vieillot, H., Buffrénil, V. D., Castanet, J., Géraudie, J., Meunier, F. J., Sire, J. Y., et al. (1989). “Microstructure and mineralization of vertebrate skeletal tissues,” in Skeletal biomineralization: Patterns, processes and evolutionary trends. Editor J. G. Carter (New York: Van Nostrand Reinhold), 471–530.
Fujita, M., Yamasaki, S., Katagiri, C., Oshiro, I., Sano, K., Kurozumi, T., et al. (2016). Advanced maritime adaptation in the Western Pacific coastal region extends back to 35,000-30,000 years before present. Proc. Natl. Acad. Sci. U. S. A. 113, 11184–11189. doi:10.1073/pnas.1607857113
Fujita, M., Yamasaki, S., Sugawara, H., and Eda, M. (2014). Body size reduction in wild boar (Sus scrofa) from the late Pleistocene Maehira Fissure Site in Okinawa-jima Island, Japan, with relevance to human arrival. Quat. Int. 339, 289–299. doi:10.1016/j.quaint.2014.04.043
Groves, C. (2006). The genus Cervus in eastern Eurasia. Eur. J. Wildl. Res. 52, 14–22. doi:10.1007/s10344-005-0011-5
Hokkaido Institute of Environmental Sciences (1997). Results of a survey related to sika deer and brown bear sighting on Hokkaido. Sapporo: Hokkaido Institute of Environmental Sciences. (in Japanese).
Jordana, X., and Köhler, M. (2011). Enamel microstructure in the fossil bovid Myotragus balearicus (Majorca, Spain): Implications for life-history evolution of dwarf mammals in insular ecosystems. Palaeogeogr. Palaeoclimatol. Palaeoecol. 300, 59–66. doi:10.1016/j.palaeo.2010.12.008
Jordana, X., Marín-Moratalla, N., DeMiguel, D., Kaiser, T. M., and Köhler, M. (2012). Evidence of correlated evolution of hypsodonty and exceptional longevity in endemic insular mammals. Proc. Biol. Sci. 279, 3339–3346. doi:10.1098/rspb.2012.0689
Jordana, X., Marín-Moratalla, N., Moncunill-Solè, B., Nacarino-Meneses, C., and Köhler, M. (2016). Ontogenetic changes in the histological features of zonal bone tissue of ruminants: A quantitative approach. Comptes Rendus Palevol 15, 255–266. doi:10.1016/j.crpv.2015.03.008
Kawamura, Y. (2009). “Fossil record of sika deer in Japan,” in Sika deer: Biology and management of native and introduced populations. Editors D. R. McCullough, S. Takatsuki, and K. Kaji (Tokyo: Springer), 11–25.
Klevezal, G. A. (1996). Recording Structures of Mammals. Determination of Age and Reconstruction of Life History. Rotterdam: A. A. Balkema.
Köhler, M., Herridge, V., Nacarino-Meneses, C., Fortuny, J., Moncunill-Solè, B., Rosso, A., et al. (2021). Palaeohistology reveals a slow pace of life for the dwarfed Sicilian elephant. Sci. Rep. 11, 22862. doi:10.1038/s41598-021-02192-4
Köhler, M., Marín-Moratalla, N., Jordana, X., and Aanes, R. (2012). Seasonal bone growth and physiology in endotherms shed light on dinosaur physiology. Nature 487, 358–361. doi:10.1038/nature11264
Köhler, M., and Moyà-Solà, S. (2009). Physiological and life history strategies of a fossil large mammal in a resource-limited environment. Proc. Natl. Acad. Sci. U. S. A. 106, 20354–20358. doi:10.1073/pnas.0813385106
Kolb, C., Scheyer, T. M., Lister, A. M., Azorit, C., de Vos, J., Schlingemann, M. A., et al. (2015). Growth in fossil and extant deer and implications for body size and life history evolution. BMC Evol. Biol. 15, 19. doi:10.1186/s12862-015-0295-3
Kolb, C., Scheyer, T. M., Veitschegger, K., Forasiepi, A. M., Amson, E., van der Geer, A. A., et al. (2015). Mammalian bone palaeohistology: A survey and new data with emphasis on island forms. PeerJ 3, e1358. doi:10.7717/peerj.1358
Kubo, M. O., and Fujita, M. (2021). Diets of Pleistocene insular dwarf deer revealed by dental microwear texture analysis. Palaeogeogr. Palaeoclimatol. Palaeoecol. 562, 110098. doi:10.1016/j.palaeo.2020.110098
Kubo, M. O., Fujita, M., Matsu’ura, S., Kondo, M., and Suwa, G. (2011). Mortality profiles of late Pleistocene deer remains of Okinawa island: Evidence from the hananda-gama cave and yamashita-cho cave I sites. Anthropol. Sci. 119, 183–201. doi:10.1537/ase.091215
Kubo, M. O., and Takatsuki, S. (2015). Geographical body size clines in sika deer: Path analysis to discern amongst environmental influences. Evol. Biol. 42, 115–127. doi:10.1007/s11692-015-9303-1
Kubo, M. O., Yamada, E., Fujita, M., and Oshiro, I. (2015). Paleoecological reconstruction of late Pleistocene deer from the Ryukyu islands, Japan: Combined evidence of mesowear and stable isotope analyses. Palaeogeogr. Palaeoclimatol. Palaeoecol. 435, 159–166. doi:10.1016/j.palaeo.2015.06.001
Lafuente, E., and Beldade, P. (2019). Genomics of developmental plasticity in animals. Front. Genet. 10, 720. doi:10.3389/fgene.2019.00720
Larramendi, A., and Palombo, M. R. (2015). Body Size, biology and encephalization quotient of Palaeoloxodon ex gr. P. falconeri from Spinagallo Cave (Hyblean plateau, Sicily). Hystrix-Italian J. Mammal. 26, 102–109. doi:10.4404/hystrix-26.2-11478
Lee, A. H., Huttenlocker, A. K., Padian, K., and Woodward, H. N. (2013). “Analysis of growth rates,” in Bone histology of fossil tetrapods: Advancing methods, analysis, and interpretation. Editors K. Padian, and E. T. Lamm (Berkeley: University of California Press), 217–251.
Lehman, T. M., and Woodward, H. N. (2008). Modeling growth rates for sauropod dinosaurs. Paleobiology 34, 264–281. doi:10.1666/0094-8373(2008)034[0264:mgrfsd]2.0.co;2
Lister, A. M. (1996). Dwarfing in island elephants and deer: Processes in relation to time of isolation. Symp. Zool. Soc. 69, 277–292.
Lister, A. M. (1989). Rapid dwarfing of red deer on Jersey in the Last Interglacial. Nature 342, 539–542. doi:10.1038/342539a0
Lomolino, M. V., Sax, D. F., Palombo, M. R., and van der Geer, A. A. (2012). Of mice and mammoths: Evaluations of causal explanations for body size evolution in insular mammals. J. Biogeogr. 39, 842–854. doi:10.1111/j.1365-2699.2011.02656.x
Lomolino, M. V., van der Geer, A. A., Lyras, G. A., Palombo, M. R., Sax, D. F., and Rozzi, R. (2013). Of mice and mammoths: Generality and antiquity of the island rule. J. Biogeogr. 40, 1427–1439. doi:10.1111/jbi.12096
Long, E. S., Courtney, K. L., Lippert, J. C., and Wall-Scheffler, C. M. (2019). Reduced body size of insular black-tailed deer is caused by slowed development. Oecologia 189, 675–685. doi:10.1007/s00442-019-04367-3
Louys, J., Braje, T. J., Chang, C. H., Cosgrove, R., Fitzpatrick, S. M., Fujita, M., et al. (2021). No evidence for widespread island extinctions after Pleistocene hominin arrival. Proc. Natl. Acad. Sci. U. S. A. 118, e2023005118. doi:10.1073/pnas.2023005118
Marín-Moratalla, N., Jordana, X., García-Martínez, R., and Köhler, M. (2011). Tracing the evolution of fitness components in fossil bovids under different selective regimes. Comptes Rendus Palevol 10, 469–478. doi:10.1016/j.crpv.2011.03.007
Marín-Moratalla, N., Jordana, X., and Köhler, M. (2013). Bone histology as an approach to providing data on certain key life history traits in mammals: Implications for conservation biology. Mamm. Biol. 78, 422–429. doi:10.1016/j.mambio.2013.07.079
Miszkiewicz, J. J., and van der Geer, A. A. E. (2022). Inferring longevity from advanced rib remodelling in insular dwarf deer. Biol. J. Linn. Soc. 136, 41–58. doi:10.1093/biolinnean/blac018
Monteiro, L. S., and Falconer, D. S. (1966). Compensatory growth and sexual maturity in mice. Animal Sci. 8, 179–192. doi:10.1017/s0003356100034565
Nagata, J., Masuda, R., Tamate, H. B., Hamasaki, S., Ochiai, K., Asada, M., et al. (1999). Two genetically distinct lineages of the sika deer, Cervus nippon, in Japanese islands: Comparison of mitochondrial D-loop region sequences. Mol. Phylogenet Evol. 13, 511–519. doi:10.1006/mpev.1999.0668
Ochiai, K., and Asada, M. (1995). Growth in the body size of sika deer (Cervus nippon) on the Boso Peninsula, central Japan. J. Nat. Hist. Mus. Inst. Chiba 3, 223–232. (in Japanese with English abstract).
Ohshima, K. (1990). The history of straits around the Japanese Islands in the Late-Quaternary. Quat. Res. 29, 193–208. (in Japanese with English abstract). doi:10.4116/jaqua.29.193
Ohtaishi, N. (1986). Preliminary memorandum of classification, distribution and geographic variation on Sika deer. Honyurui Kagaku Mamm. Sci. 53, 13–17. (in Japanese with English abstract). doi:10.11238/mammalianscience.26.2_13
Okinawa Prefectural Board of Education (1996). A report of conservation and management of sika deer in Kerama Islands. Okinawa: Okinawa Prefectural Board of Education.
Okinawa Prefectural Museum and Art Museum (Editor) (2009). Excavation of the hananda-gama cave site, Okinawa (Naha: Kokusai-insatsu Co.).
Okumura, K., Ishida, S., Kawamura, Y., Kumada, M., and Tamiya, S. (1982). Latest Pleistocene mammalian assemblage of Kumaishi-do cave, Gifu prefecture and the significance of its 14C age. Earth Sci. (Chikyu Kagaku) 36, 214–218. (in Japanese with English abstract). doi:10.15080/agcjchikyukagaku.36.4_214
Okumura, K., Ishida, S., Taruno, H., and Kawamura, Y. (2016). Yabe's giant deer and elk remains from the Late Pleistocene of Kumaishi-do Cave, Gifu Prefecture (Part 1): Antlers, a skull, mandibles, and teeth. Bull. Osaka Mus. Nat. Hist. 70, 1–82. (in Japanese with English abstract).
Oli, M. K. (2004). The fast–slow continuum and mammalian life-history patterns: An empirical evaluation. Basic Appl. Ecol. 5, 449–463. doi:10.1016/j.baae.2004.06.002
Orlandi-Oliveras, G., Nacarino-Meneses, C., Koufos, G. D., and Köhler, M. (2018). Bone histology provides insights into the life history mechanisms underlying dwarfing in hipparionins. Sci. Rep. 8, 17203. doi:10.1038/s41598-018-35347-x
Otsuka, H., and Takahashi, A. (2000). Pleistocene vertebrate faunas in the Ryukyu islands: Their migration and extinction. Tropics 10, 25–40. doi:10.3759/tropics.10.25
Ozaki, M. (2009). “Estimation of age at death from tooth wear of lower molars of excavated deer and comparison of age structures with modern deer populations,” in Excavation of the hananda-gama cave site, Okinawa (Okinawa Prefectural Museum and Art Museum. Naha: Kokusai-insatsu Co.), 24–34. (in Japanese).
Ozaki, M., Kaji, K., Matsuda, N., Ochiai, K., Asada, M., Ohba, T., et al. (2010). The relationship between food habits, molar wear and life expectancy in wild sika deer populations. J. Zool. 280, 202–212. doi:10.1111/j.1469-7998.2009.00653.x
Palkovacs, E. P. (2003). Explaining adaptive shifts in body size on islands: A life history approach. Oikos 103, 37–44. doi:10.1034/j.1600-0706.2003.12502.x
Palombo, M. R. (2001). “Endemic elephants of the mediterranean islands: Knowledge, problems and perspectives,” in The World of Elephants - International Congress Rome, 486–491.
Price, T. D., Qvarnstrom, A., and Irwin, D. E. (2003). The role of phenotypic plasticity in driving genetic evolution. Proc. Biol. Sci. 270, 1433–1440. doi:10.1098/rspb.2003.2372
Raia, P., Barbera, C., and Conte, M. (2003). The fast life of a dwarfed giant. Evol. Ecol. 17, 293–312. doi:10.1023/a:1025577414005
Raia, P., and Meiri, S. (2006). The island rule in large mammals: Paleontology meets ecology. Evolution 60, 1731–1742. doi:10.1554/05-664.1
Rozzi, R., and Lomolino, M. V. (2017). Rapid dwarfing of an insular mammal - the feral cattle of Amsterdam island. Sci. Rep. 7, 8820. doi:10.1038/s41598-017-08820-2
Rozzi, R., Lomolino, M. V., van der Geer, A., Silvestro, D., Lyons, S. K., Bover, P., et al. (2023). Dwarfism and gigantism drive human-mediated extinctions on islands. Science 379, 1054–1059. doi:10.1126/science.add8606
Sand, H., Cederlund, G., and Danell, K. (1995). Geographical and latitudinal variation in growth patterns and adult body size of Swedish moose (Alces alces). Oecologia 102, 433–442. doi:10.1007/bf00341355
Sander, P. M. (2000). Longbone histology of the tendaguru sauropods: Implications for growth and biology. Paleobiology 26, 466–488. doi:10.1666/0094-8373(2000)026<0466:lhotts>2.0.co;2
Sander, P. M., Mateus, O., Laven, T., and Knotschke, N. (2006). Bone histology indicates insular dwarfism in a new Late Jurassic sauropod dinosaur. Nature 441, 739–741. doi:10.1038/nature04633
Shiroma, T., and Ohta, H. (1996). “Geographical variation in cranial morphology in sika deer with a special reference on Kerama deer in A report of conservation and management of sika deer in Kerama Islands,”. Editor Okinawa Prefectural Board of Education (Okinawa: Okinawa Prefectural Board of Education), 13–55. (in Japanese).
Suzuki, M., Onuma, M., Yokoyama, M., Kaji, K., Yamanaka, M., and Ohtaishi, N. (2001). Body size, sexual dimorphism, and seasonal mass fluctuations in a larger sika deer subspecies, the Hokkaido sika deer (Cervus nippon yesoensis Heude, 1884). Can. J. Zool. 79, 154–159. doi:10.1139/z00-181
Taruno, H., Kawamura, Y., Ishida, S., and Okumura, K. (2017). Yabe’s giant deer and elk remains from the late Pleistocene of kumaishi-do cave, Gifu prefecture, central Japan (Part 2): Postcranial bones. Bull. Osaka Mus. Nat. Hist. 71, 17–142.
Terada, C., and Saitoh, T. (2018). Phenotypic and genetic divergence among island populations of sika deer (Cervus nippon) in southern Japan: A test of the local adaptation hypothesis. Popul. Ecol. 60, 211–221. doi:10.1007/s10144-018-0607-8
Terada, C., Tatsuzawa, S., and Saitoh, T. (2012). Ecological correlates and determinants in the geographical variation of deer morphology. Oecologia 169, 981–994. doi:10.1007/s00442-012-2270-7
van der Geer, A., Lyras, G., de Vos, J., and Dermitzakis, M. (2010). Evolution of Island Mammals: Adaptation and Extinction of Placental Mammals on Island. West Sussex: John Wiley & Sons Ltd.
van der Geer, A. A., Lyras, G. A., Lomolino, M. V., Palombo, M. R., and Sax, D. F. (2013). Body size evolution of palaeo-insular mammals: Temporal variations and interspecific interactions. J. Biogeogr. 40, 1440–1450. doi:10.1111/jbi.12119
Woodward, H. N., Horner, J. R., and Farlow, J. O. (2014). Quantification of intraskeletal histovariability in Alligator mississippiensis and implications for vertebrate osteohistology. PeerJ 2, e422. doi:10.7717/peerj.422
Keywords: bone histology, deer, demography, growth rate, life history, fossils, islands, skeletochronology
Citation: Hayashi S, Kubo MO, Sánchez-Villagra MR, Taruno H, Izawa M, Shiroma T, Nakano T and Fujita M (2023) Variation and process of life history evolution in insular dwarfism as revealed by a natural experiment. Front. Earth Sci. 11:1095903. doi: 10.3389/feart.2023.1095903
Received: 11 November 2022; Accepted: 17 March 2023;
Published: 22 May 2023.
Edited by:
Fabrizio Antonioli, Istituto Nazionale di Geofisica e Vulcanologia (INGV), ItalyReviewed by:
Paul Palmqvist, University of Malaga, SpainJustyna Miszkiewicz, The University of Queensland, Australia
Copyright © 2023 Hayashi, Kubo, Sánchez-Villagra, Taruno, Izawa, Shiroma, Nakano and Fujita. This is an open-access article distributed under the terms of the Creative Commons Attribution License (CC BY). The use, distribution or reproduction in other forums is permitted, provided the original author(s) and the copyright owner(s) are credited and that the original publication in this journal is cited, in accordance with accepted academic practice. No use, distribution or reproduction is permitted which does not comply with these terms.
*Correspondence: Shoji Hayashi, c2hvamktaGF5YXNoaUBvdXMuYWMuanA=; Mugino O. Kubo, bXVnaW5vQGsudS10b2t5by5hYy5qcA==
†These authors have contributed equally to this work and share first authorship