- 1College of Tourism and Geographical Science, Leshan Normal University, Leshan, China
- 2College of Geography and Tourism, Hengyang Normal University, Hengyang, China
A series of NE-to E-trending ductile shear zones are widely distributed in the Khondalite Belt, a Paleoproterozoic collisional orogen in the North China Craton. Available geochronological investigations have been mainly focused on metamorphic and magmatic events in the Khondalite Belt, but the deformation age of ductile shear zones remains poorly constrained. In this paper, we conducted field-based structural and U-Pb geochronological studies on the Helanshan ductile shear zones (HDSZ) and Qianlishan ductile shear zones (QDSZ) in the western Khondalite Belt. The results revealed that four pre-kinematic intrusions were reworked by the shear zones and yielded monazite U-Pb ages of 1954 ± 3 Ma, 1942 ± 10 Ma, 1925 ± 5 Ma and 1918 ± 4 Ma, suggesting that the HDSZ and QDSZ probably appeared at some time after ∼1918 Ma. In the HDSZ, a granitic mylonite gave titanite and apatite U-Pb ages of 1897 ± 32 Ma and 1866 ± 47 Ma. Similar apatite U-Pb ages of 1860 ± 78 Ma and 1823 ± 50 Ma were also reported from another two mylonitized rocks. Comparably, three mylonites from the QDSZ displayed apatite ages of 1878 ± 39 Ma, 1805 ± 16 Ma and 1801 ± 10 Ma. Notably, these titanite and apatite U-Pb ages of 1897–1801 Ma are in good agreement with those of 1904–1823 Ma obtained from zircon overgrowth rims in mylonites. We regard that the above-stated U-Pb ages of 1904–1801 Ma together recorded the timing of the shear zone activity in the western Khondalite Belt. Combined with previous structural and geochronological data, we propose that the post-collisional orogen-parallel ductile shear zones in the Khondalite Belt have developed at ca. 1.90–1.80 Ga.
1 Introduction
Assessment of the timing of high-temperature (T > 550°C) ductile shear zones has been one of the major challenges in geochronology, especially the early Precambrian shear zones that underwent long-term complex evolution history (Sibson, 1977; Ramsay, 1980; Law, 2014; Cao and Neubauer, 2016; Fossen and Cavalcante, 2017; Oriolo et al., 2018; Zhang et al., 2022; Ribeiro et al., 2023). Traditionally, K-Ar and Rb-Sr systems in K-bearing minerals (e.g., muscovite, biotite and hornblende) are used for medium-to low-temperature thermochronology, but they are easily perturbed by post-kinematic tectono-thermal events (Reiners et al., 2005; Oriolo et al., 2018; Qiu et al., 2023). In contrast, U-Pb system is more effective to date shear zones under higher temperature conditions, and U-bearing minerals (e.g., zircon, monazite, titanite and apatite) are resistant to physical and chemical weathering (Fitch, 1972; Schoene, 2014; Harrigan et al., 2024). In high-temperature shear zones, syn-kinematically (re) crystallized U-bearing minerals from mylonites have the potential to directly record the timing of shear deformation (Oriolo et al., 2018; Qiu et al., 2023; Harrigan et al., 2024). Additionally, zircon and monazite U-Pb geochronology is commonly applied to determine emplacement ages of pre-, syn- and post-kinematic intrusions in shear zones and adjacent blocks, which indirectly provide temporal constraints on shear zone activity (van der Pluijm et al., 1994; Gong et al., 2014; Simonetti et al., 2021; Li et al., 2023; Sun and Dong, 2023). Therefore, the integration of U-Pb ages from shear zone-related intrusions and mylonites can help to better understand the tectonic evolution of high-temperature ductile shear zones.
The Khondalite Belt has been proposed as an Orosirian collisional orogen in the northwestern North China Craton, one of the oldest continental blocks around the world (Figure 1; e.g.; Zhao et al., 2005; Zhao et al., 2012; Yin et al., 2009; Yin et al., 2011; Guo et al., 2012; Zhao and Zhai, 2013; Kusky et al., 2016; Wei et al., 2023). This belt was inferred to result from the amalgamation of the Yinshan and Ordos Blocks at ∼1.95 Ga, and it experienced a protracted (>100 Myr) orogenic history, characterized by polyphase deformation, high-grade metamorphism and magmatism in the late Paleoproterozoic (Zhao et al., 2005; Zhao et al., 2012; Yin et al., 2020; Yin et al., 2023; Qiao et al., 2022; Qiao et al., 2024a; Shi et al., 2023; Shi et al., 2025; Wu et al., 2024). Of particular interest is the development of a series of orogen-parallel ductile shear zones in the western Khondalite Belt (Lu et al., 1996; Gong et al., 2014; Qiao et al., 2024b). However, available geochronological investigations were mainly focused on metamorphic and magmatic events in this region (e.g., Yin et al., 2009; Yin et al., 2011; Zhou and Geng, 2009; Dan et al., 2012; Qiao et al., 2016; Li et al., 2017; Li et al., 2022; Gou et al., 2019; Wu et al., 2020; Wu et al., 2024), and comparatively the shear zone activity has attracted less attention. Gong et al. (2014) conducted biotite 40Ar/39Ar dating of a mylonite in the Helanshan Complex but failed to obtain a meaningful age. Recently, Qiao et al. (2024a), Qiao et al. (2024b) carried out zircon U-Pb geochronological studies on mylonites in the western Khondalite Belt and interpreted that metamorphic ages of zircon overgrowth rims probably recorded the deformation age of shearing. There is still the problem whether these zircon U-Pb ages can solely represent the timing of shear zones. In this study, we conducted monazite, titanite and apatite U-Pb geochronology on shear zone-related intrusions and high-temperature mylonites in the Helanshan and Qianlishan Complexes. Combined with available data, these new results will shed light on the deformation history of the ductile shear zones in the western Khondalite Belt.
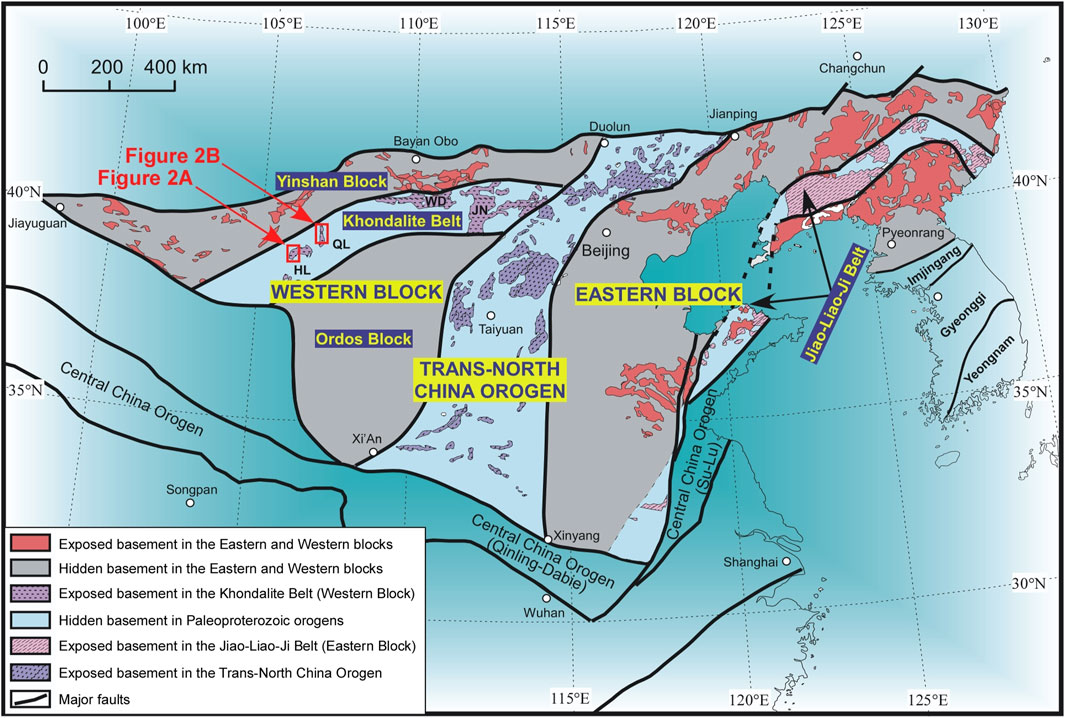
Figure 1. Tectonic subdivision of the Precambrian basement of the North China Craton (modified after Zhao et al., 2005). Abbreviations: HL, Helanshan Complex; QL, Qianlishan Complex; WD, Wulashan-Daqingshan Complex; JN, Jining Complex.
2 Geological setting and samples
The Khondalite Belt is regarded as a typical Paleoproterozoic continent-continent collisional orogen in the northwestern North China Craton, resulting from the final amalgamation of the Ordos Block in the south and the Yinshan Block in the north at ∼1.95 Ga (e.g., Zhao et al., 2005; Zhao et al., 2012; Yin et al., 2009; Yin et al., 2011; Guo et al., 2012; Qiao et al., 2016; Jiang et al., 2022; Wu et al., 2024; Shi et al., 2025). From west to east, this belt exposes the Helanshan, Qianlishan, Daqingshan-Wulashan and Jining Complexes (Figure 1; Zhao et al., 2005). Lithologically, these complexes are dominated by granulite-facies metasedimentary rocks, mainly including graphite-bearing sillimanite-garnet gneisses, felsic paragneisses, garnet-bearing quartzites, diopside-bearing marbles and calc-silicate rocks, collectively termed as “khondalites” (Lu et al., 1996; Zhao et al., 2005; Zhai, 2022). The khondalites spatially occur in association with S-type granites, charnockites, tonalite-trondhjemite-granodiorite (TTG) gneisses and mafic granulites (Lu et al., 1996; Zhao et al., 2005; Zhai, 2022). Available geochronological investigations unraveled that the protolith of the khondalites was approximately deposited at 2.00–1.95 Ga, and subsequently experienced high-grade metamorphism at 1.95–1.85 Ga (e.g., Xia et al., 2006a; Xia et al., 2006b; Wan et al., 2006; Wan et al., 2009; Wan et al., 2013; Dong et al., 2007; Yin et al., 2009; Yin et al., 2011; Zhou and Geng, 2009; Jiao et al., 2013; Jiao et al., 2015; Jiao et al., 2020; Liu et al., 2013; Liu et al., 2014; Liu et al., 2017; Santosh et al., 2013; Cai et al., 2017; He et al., 2017; Wang et al., 2017; Gou et al., 2019; Wu et al., 2020; Wu et al., 2024; Shi et al., 2023; Shi et al., 2025; Zhu et al., 2023). Noticeably, similar clockwise P-T paths with post-peak (near-) isothermal decompression have been obtained from medium- to high-pressure pelitic and mafic granulites, indicating orogenic processes that involved initial crustal thickening and then exhumation (e.g., Zhou et al., 2010; Cai et al., 2014; Cai et al., 2017; Yin et al., 2014; Yin et al., 2015; Liu et al., 2017; Jiao et al., 2017; Xu et al., 2018; Xu et al., 2023; Wu et al., 2020). A series of orogen-parallel ductile shear zones are widespread throughout the Khondalite Belt, for example, the nearly E-W-striking Xiashihao-Jiuguan ductile shear zone in the Wulashan-Daqingshan Complex and the NE(E)-SW(W)-striking Xuwujia ductile shear zone in the Jining Complex (Guo and Zhai, 1992; Gan and Qian, 1996; Lu et al., 1996; Wang et al., 1999; Gong et al., 2014).
The Helanshan and Qianlishan Complexes are situated in the westernmost segment of the Khondalite Belt, and they were unconformably overlain by the Mesoproterozoic sedimentary rocks (the Changcheng-Jixian System) (Figure 2; Lu et al., 1996; Zhao et al., 2005; Qiao, 2019). The Helanshan and Qianlishan Complexes contain typical rock assemblages of the khondalites, named the Helanshan and Qianlishan Group, respectively (Figure 2; Lu et al., 1996; Zhao et al., 2005; Yin et al., 2009; Yin et al., 2011). Available geochronological data reveal that U-Pb ages of detrital zircons from the Helanshan and Qianlishan Group mainly ranged from 2.20 Ga to 2.00 Ga, and yielded a single peak at ca. 2.03 Ga (e.g., Yin et al., 2009; Yin et al., 2011; Dan et al., 2012; Qiao et al., 2016; Qiao et al., 2022; Wu et al., 2020; Wu et al., 2024). Medium-to high-pressure pelitic and felsic granulites have been discovered in the studied area, and they mainly showed a major metamorphic age group at ca. 1.95 Ga (e.g., Yin et al., 2009; Yin et al., 2011; Qiao et al., 2016; Qiao et al., 2022; Qiao et al., 2023; Xu et al., 2018; Xu et al., 2023; Gou et al., 2019; Wu et al., 2020; Wu et al., 2024). Multiple magmatic events in this region have been reported at ca. 2.06–2.00 Ga, 1.95 Ga, 1.93–1.90 Ga and 1.88–1.82 Ga (e.g., Yin et al., 2009; Yin et al., 2011; Yin et al., 2020; Dan et al., 2012; Li et al., 2022; Qiao et al., 2021; Qiao et al., 2022; Qiao et al., 2023; Qiao et al., 2024a). In addition, previous structural analyses show that NE- to E-trending ductile shear zones (i.e., HDSZ and QDSZ) commonly appeared in the Helanshan and Qianlishan Complexes, which are mainly characterized by intensive ductile deformation, high-temperature (T > 650°C) granitic, pelitic and felsic mylonites (Lu et al., 1996; Gong et al., 2014; Yin et al., 2020; Qiao et al., 2021; Qiao et al., 2022; Qiao et al., 2024a; Qiao et al., 2024b).
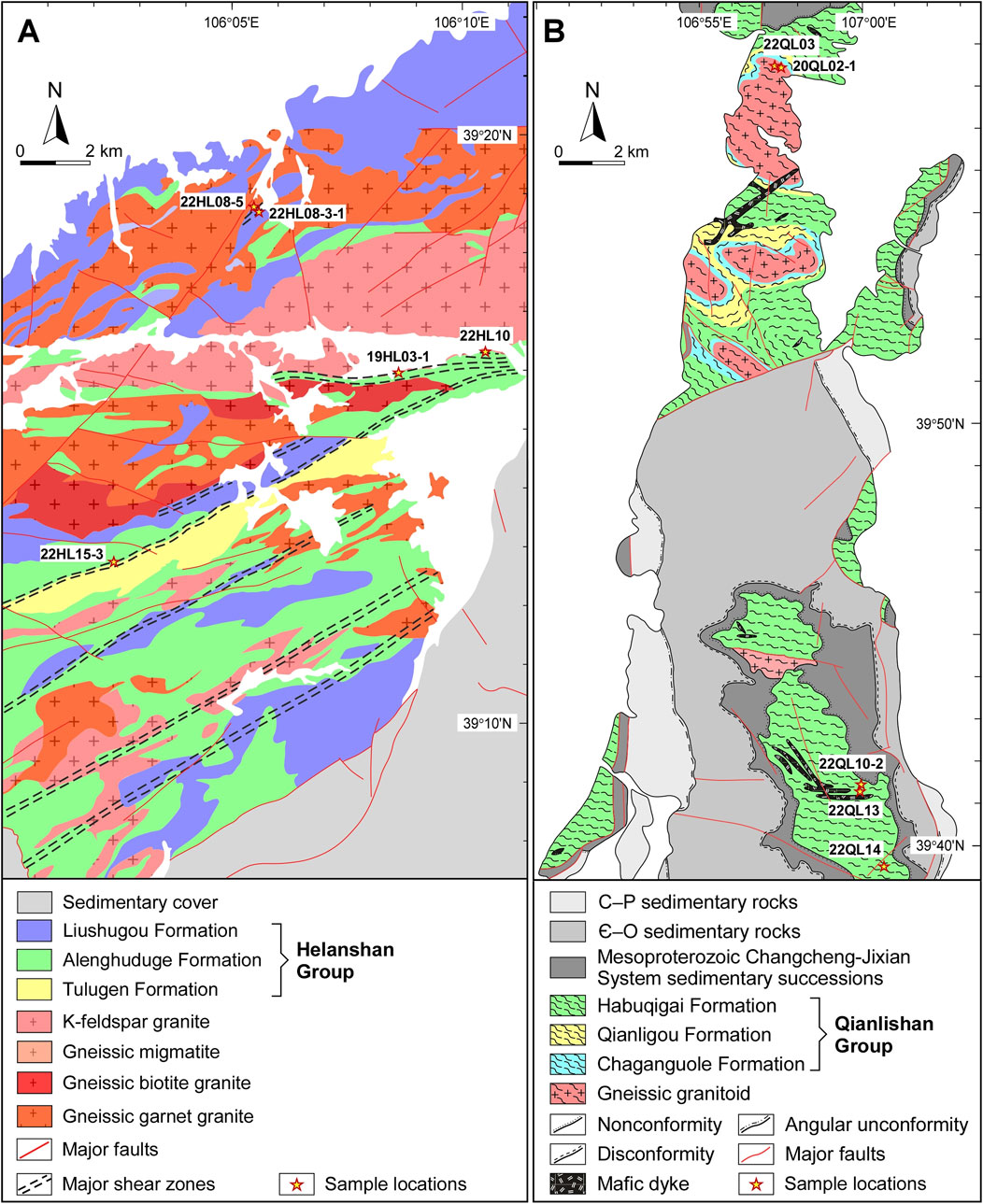
Figure 2. Simplified geological map of high-grade metamorphic complexes in the western Khondalite Belt (modified after Yin et al., 2009; Yin et al., 2011; Qiao et al., 2024a; Qiao et al., 2024b). (A) The Helanshan Complex. (B) The Qianlishan Complex.
Based on structural observations in the field, we have selected ten rock samples in the HDSZ and QDSZ for U-Pb geochronology, and the sample locations were presented in Figure 2. Of these, six samples were collected from high-temperature granitic mylonites (Samples 19HL03-1, 22HL08-3-1, 22HL10, 22QL13, 20QL02-1 and 22QL03-1). Their detailed outcrop descriptions and microtectonic features have been given in Qiao et al. (2024a), Qiao et al. (2024b). Other four samples (Samples 22HL08-5, 22HL15-3, 22QL10-2 and 22QL14) were collected from granitic dykes and plutons that have been variably reworked by the ductile shear zones (Figure 3), suggesting that they were pre-kinematic intrusions.
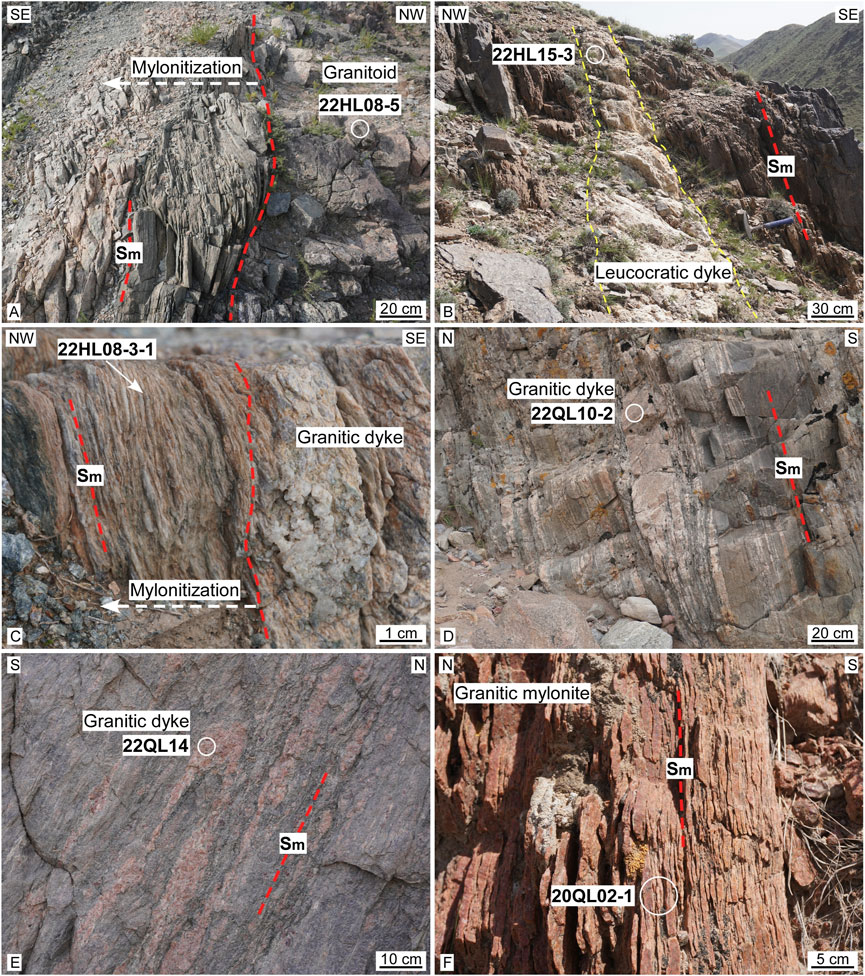
Figure 3. Typical field photos showing shear zone-related structures and dating samples in the western Khondalite Belt. (A) A granitoid pluton (Sample 22HL08-5) has been reworked by the ductile shear zone and developed mylonitic foliations (Sm). (B) A leucocratic dyke (Sample 22HL15-3) in the shear zone was subjected to ductile deformation, showing parallelism with mylonitic foliations in the wall rocks. (C) A granitic dyke was affected by the shear zone activity to become granitic mylonite (Sample 22HL08-3-1). (D,E) A boudinaged granitic dyke (Sample 22QL10-2) and a strongly-folded granitic dyke (Sample 22QL14) in the shear zone. (F) A granitoid pluton suffered mylonitization and generated granitic mylonite (Sample 20QL02-1).
3 Analytical methods
Monazite, titanite and apatite have been extracted from ten rock samples by conventional heavy liquid and magnetic separation techniques. These grains were then handpicked, mounted in epoxy, polished and photographed in reflected and transmitted light. Subsequently, their internal textures were determined through cathodoluminescence (CL) and backscattered electron (BSE) images. U-Pb dating and trace element analyses were conducted by Laser Ablation Inductively Coupled Plasma Mass Spectrometry (LA-ICP-MS) at the Guangzhou Tuoyan Analytical Technology Co., Ltd., Guangzhou, China. Detailed analytical methods followed those described in Ren et al. (2024) and Xing et al. (2024). Test data were processed offline with the software Iolite4 (Paton et al., 2011). Tera-Wasserburg diagrams and age calculations were made using IsoplotR (Vermeesch, 2018). The uncertainties on intercept age and weighted mean age were presented at the 95% confidence level (2σ). The monazite, titanite and apatite U-Pb data in this paper are provided in the Supplementary files (Supplementary Tables S1–S3).
4 U-Pb geochronology
4.1 Monazite from pre-kinematic intrusions
4.1.1 Sample 22HL15-3
Monazite from sample 22HL15-3 are euhedral in morphology and 150–200 μm in grain size. Meanwhile, these monazite grains mainly show gray homogeneous structures in the BSE images (Figure 4A). A total of eighteen analytical spots have been made in this sample, and the U-Pb data were presented in Supplementary Table S1. These spots yielded 207Pb/206Pb apparent ages from 1903 ± 23 Ma to 1931 ± 19 Ma, and they were plotted on the concordia line in the Tera-Wasserburg (T-W) diagram (Figures 4B,C). These data well defined a weighted mean 207Pb/206Pb age of 1918 ± 4 Ma (n = 18, MSWD = 0.71, Figure 4C).
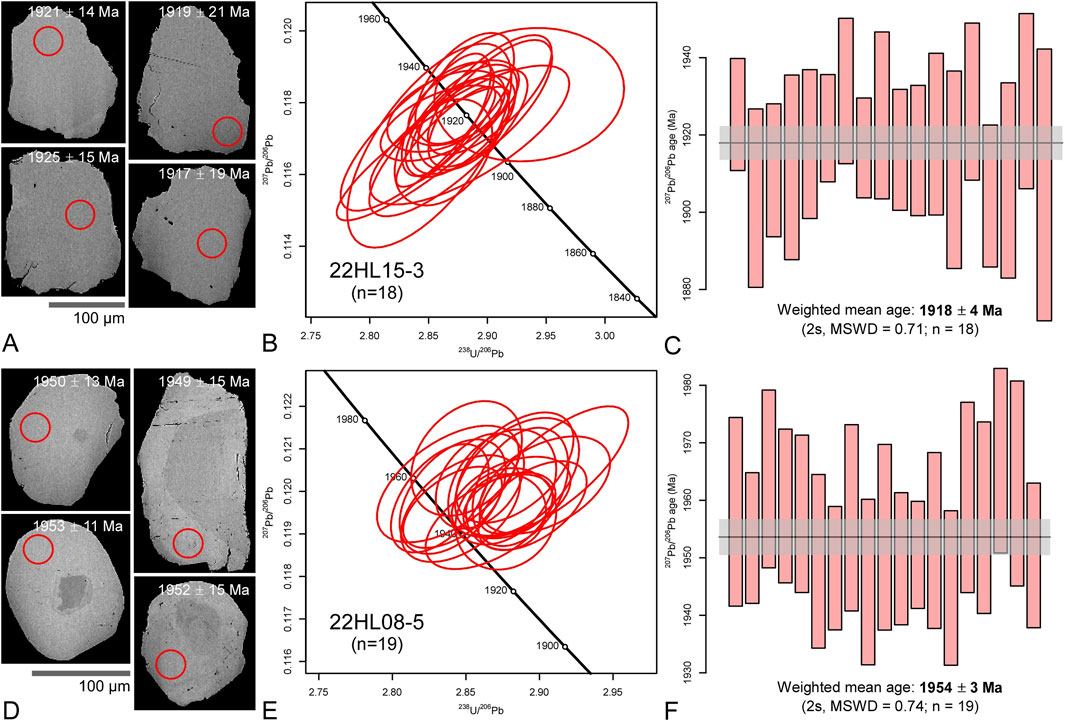
Figure 4. Representative BSE image, Tera-Wasserburg diagram and bar chart showing monazite and their U-Pb data from the Helanshan Complex. (A–C) Sample 22HL15-3. (D–F) Sample 22HL08-5. Red circles show the locations of LA-ICP-MS analytical spots and 207Pb/206Pb ages with 2σ error are labeled.
4.1.2 Sample 22HL08-5
Monazite grains from sample 22HL08-5 are subhedral to anhedral in morphology, with sizes of 100–200 μm. BSE images reveal that most monazite grains are homogeneous, and some of them have low luminescent cores (Figure 4D). In this study, nineteen spots were analyzed on homogeneous zones of monazite grains. As shown in Supplementary Table S1 and Figures 4E,F, these data gave a weighted mean 207Pb/206Pb age of 1954 ± 3 Ma (MSWD = 0.74, n = 19).
4.1.3 Sample 22QL10-2
Monazite grains from sample 22QL10-2 generally show euhedral to anhedral in morphology, ranging in size from 50 to 100 μm. In the BSE images, they have homogeneous internal structures (Figure 5A). Fifteen monazite grains were analyzed in the sample and showed a weighted mean 207Pb/206Pb age of 1942 ± 10 Ma (n = 15, MSWD = 0.39, Figures 5B,C).
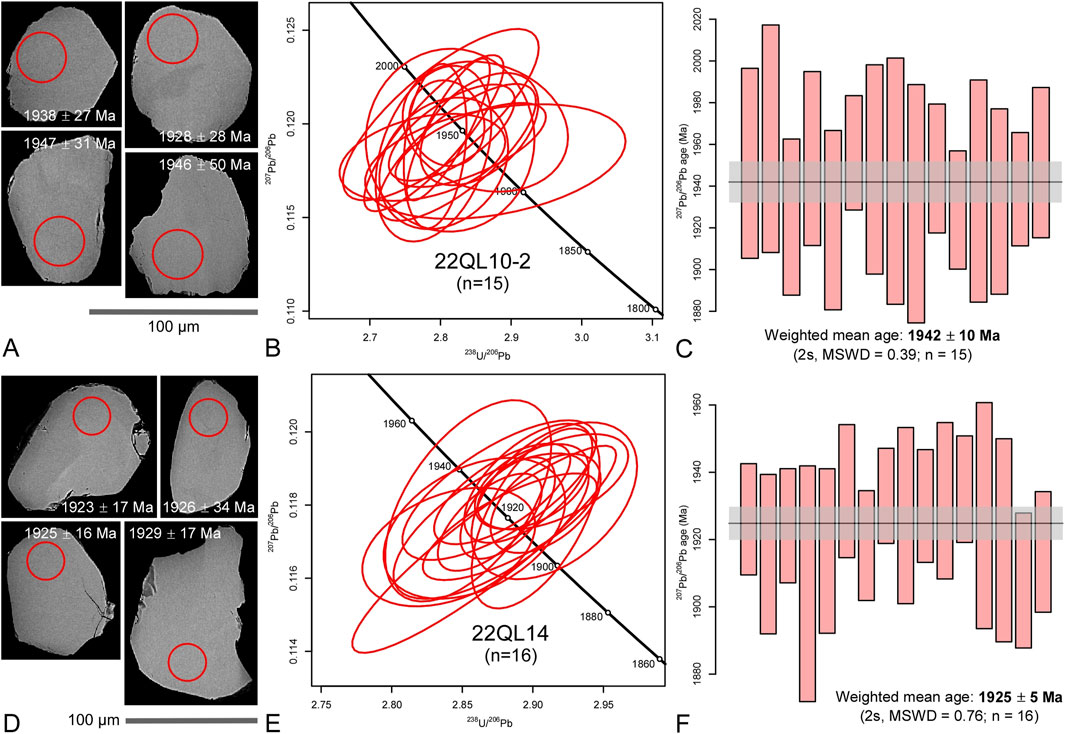
Figure 5. Representative BSE image, Tera-Wasserburg diagram and bar chart showing monazite and their U-Pb data in the Qianlishan Complex. (A–C) Sample 22QL10-2. (D–F) Sample 22QL14. Red circles show the locations of LA-ICP-MS analytical spots and 207Pb/206Pb ages with 2σ error are labeled.
4.1.4 Sample 22QL14
Monazite from sample 22QL14 are euhedral to anhedral and vary from 50 to 150 μm in grain size. BSE images reveal that they displayed homogeneous gray structures (Figure 5D). A total of sixteen grains were conducted in this sample and yielded a weighted mean 207Pb/206Pb age of 1925 ± 5 Ma (n = 16, MSWD = 0.76, Figures 5E,F).
4.2 Titanite from granitic mylonite (Sample 19HL03-1)
Titanite in the sample 19HL03-1 are euhedral and vary from 50 to 150 µm in grain size. In the CL images, large titanite grains mainly contained dark cores with (out) some inclusions, surrounded by the luminescent homogeneous rims (Figure 6A). In contrast, small titanite grains displayed homogeneous internal structures (Figure 6A). A total of twenty-five spots were made on the titanite rims and homogeneous grains, and they defined a lower intercept age of 1897 ± 32 Ma in the T-W diagram (n = 25; MSWD = 0.9; Figure 6B; Supplementary Table S2).
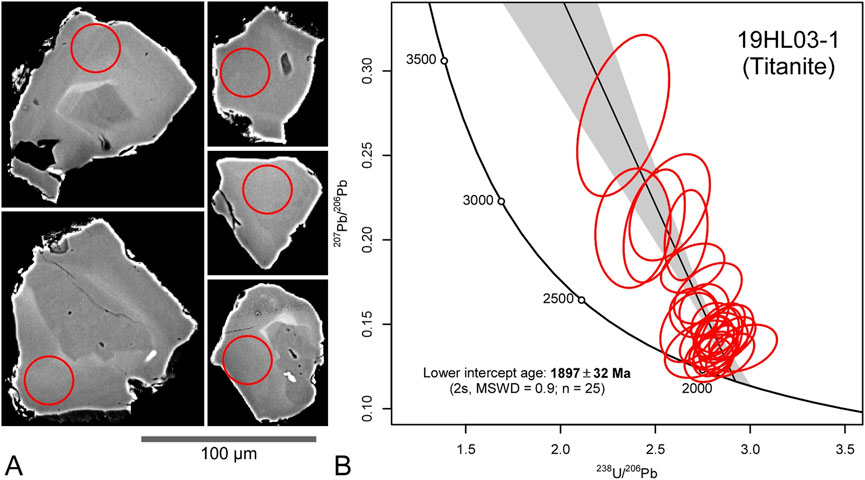
Figure 6. Diagram showing internal texture of titanite and their U-Pb data for Sample 19HL03-1. (A) Representative CL images. Red circles show the locations of LA-ICP-MS analytical spots. (B) Tera-Wasserburg diagram.
4.3 Apatite from granitic mylonites
4.3.1 Sample 19HL03-1
The apatite in this sample are euhedral to subhedral in shape, with the grain size of 100–200 µm. Meanwhile, CL images reveal that apatite grains are low luminescent and inclusion-free (Figure 7A). As listed in Supplementary Table S3, fourteen data-points were performed on apatite from this sample, and they yielded a lower intercept age of 1866 ± 47 Ma in the Tera-Wasserburg diagram (n = 14; MSWD = 1.5; Figure 8A).
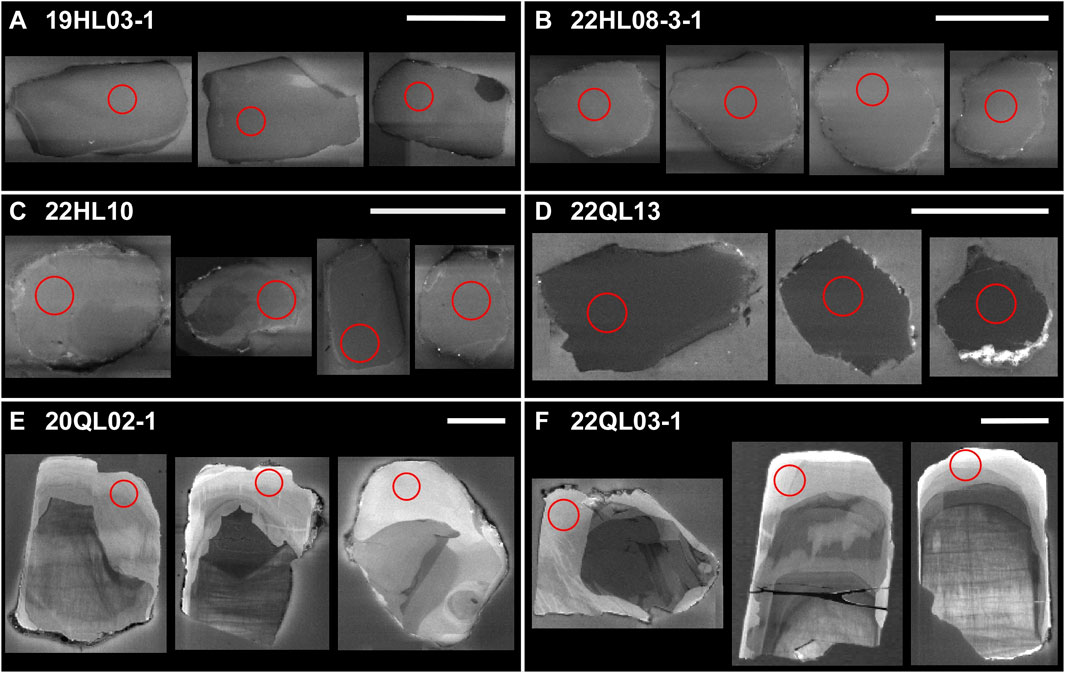
Figure 7. Representative CL images showing internal texture of apatite from mylonites. (A) Sample 19HL03-1. (B) Sample 22HL08-3-1. (C) Sample 22HL10. (D) Sample 22QL13. (E) Sample 20QL02-1. (F) Sample 22QL03-1. Red circles show the locations of LA-ICP-MS analytical spots. All scale bars are 100 μm.
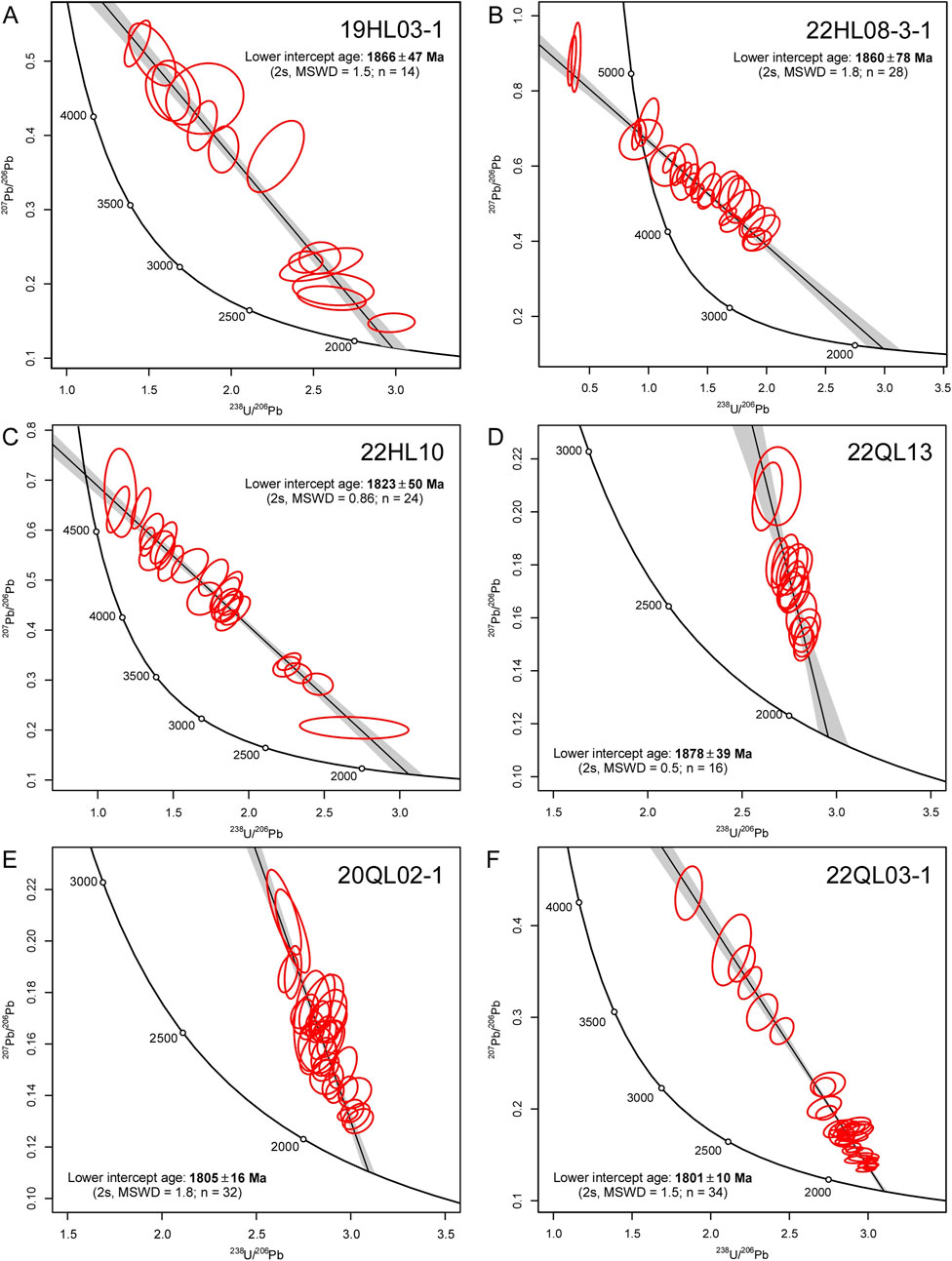
Figure 8. Tera-Wasserburg diagrams showing apatite U-Pb dating results. (A) Sample 19HL03-1. (B) Sample 22HL08-3-1. (C) Sample 22HL10. (D) Sample 22QL13. (E) Sample 20QL02-1. (F) Sample 22QL03-1.
4.3.2 Sample 22HL08-3-1
Apatite grains separated from the sample 22HL08-3-1 (Figure 3C) are subhedral to anhedral and 50–150 µm in size. CL images reveal that almost all apatite grains in this sample are homogeneous (Figure 7B). Twenty-eight spots were analyzed in sample 22HL08-3-1, and these data defined a lower intercept age of 1860 ± 78 Ma in the Tera-Wasserburg diagram (n = 28; MSWD = 1.8; Figure 8B; Supplementary Table S3).
4.3.3 Sample 22HL10
Apatite grains are mainly euhedral to anhedral in morphology, with their grain size of 50–100 µm. Most of the apatite grains in this sample are homogeneous, and some of them have small dark cores (Figure 7C). Twenty-four spots were conducted on luminescent homogeneous zones of apatite grains, and yielded a lower intercept age of 1823 ± 50 Ma in the Tera-Wasserburg diagram (n = 24; MSWD = 0.86; Figure 8C; Supplementary Table S3).
4.3.4 Sample 22QL13
Apatite grains from sample 22QL13 are generally euhedral to anhedral in morphology, with grain sizes ranging from 50 to 100 µm. They commonly showed low-luminescent and homogenous internal structures (Figure 7D). As shown in Supplementary Table S3 and Figure 8D, sixteen data-points were obtained from sample 22QL13. They displayed a lower intercept age of 1878 ± 39 Ma in the T-W diagram (n = 16; MSWD = 0.5; Figure 8D).
4.3.5 Sample 20QL02-1
Most apatite grains in this sample (Figure 3F) are euhedral to subhedral, mainly with 200–250 µm in grain size. In the CL images, these apatite grains have low luminescent cores, surrounded by bright rims (Figure 7E). A total of thirty-two spots were analyzed on the apatite rims. In the Tera-Wasserburg diagram, these data yielded a well-constrained lower intercept age of 1805 ± 16 Ma (n = 32; MSWD = 1.8; Figure 8E; Supplementary Table S3).
4.3.6 Sample 22QL03-1
Apatite separated from the sample are euhedral and large, varying from 200 to 300 µm in grain size. CL images reveal that the apatite grains are characterized by clear core-rim textures, similar to those of Sample 20QL02-1 (Figures 7E,F). Meanwhile, thirty-four spots were totally made on high luminescent apatite rims, and they defined a well-constrained lower intercept age of 1801 ± 10 Ma in the Tera-Wasserburg diagram (n = 34; MSWD = 1.5; Figure 8F; Supplementary Table S3).
5 Discussion
5.1 Timing of orogen-parallel shear zones in the western Khondalite Belt
Based on detailed structural analyses, the crystallization ages of pre-, syn- and post-kinematic intrusions can place important constraints on the timing of development of HDSZ and QDSZ. As presented in Table 1 and Figure 9, we have summarized available shear zone-related geochronological data in the western Khondalite Belt. In the HDSZ, two pre-kinematic granitic dykes (Samples 22HL08-5 and 22HL15-3) were affected by ductile shear deformation and yielded monazite weighted mean 207Pb/206Pb ages of 1954 ± 3 Ma and 1918 ± 4 Ma (Figures 3A,B, 4). They were interpreted as emplacement ages of the dykes and provided a lower age limit of the shear zone activity. Similar ages of 1942 ± 10 Ma and 1925 ± 5 Ma were also obtained from the boudinaged and strongly folded dykes in the QDSZ (Samples 22QL10-2 and 22QL14; Figures 3D,E, 5). These monazite U-Pb ages of 1953–1918 Ma indicate that the HDSZ and QDSZ must have developed at some time after ∼1918 Ma (Figure 9). This inference is supported by magmatic zircon U-Pb ages of 1952–1920 Ma from pre-kinematic intrusions in studied area (Table 1; Qiao et al., 2024a; Qiao et al., 2024b). Two syn-kinematic granitic dykes have been reported in the HDSZ, and they showed zircon U-Pb ages of 1887 ± 21 Ma and 1871 ± 28 Ma (Figure 9; Qiao et al., 2024a). Notably, a post-kinematic undeformed leucocratic dyke clearly intruded the mylonites in the HDSZ, and it was dated at 1816 ± 28 Ma (Qiao et al., 2024a), interpreted to approximately represent the upper bound of deformation age of shear zones. Taken together, the above-stated igneous zircon and monazite U-Pb ages of pre-, syn- and post-kinematic intrusions suggest that the ductile shear zone activity in the western Khondalite Belt took place at some time between 1918 ± 4 Ma and 1816 ± 28 Ma (Figure 9).
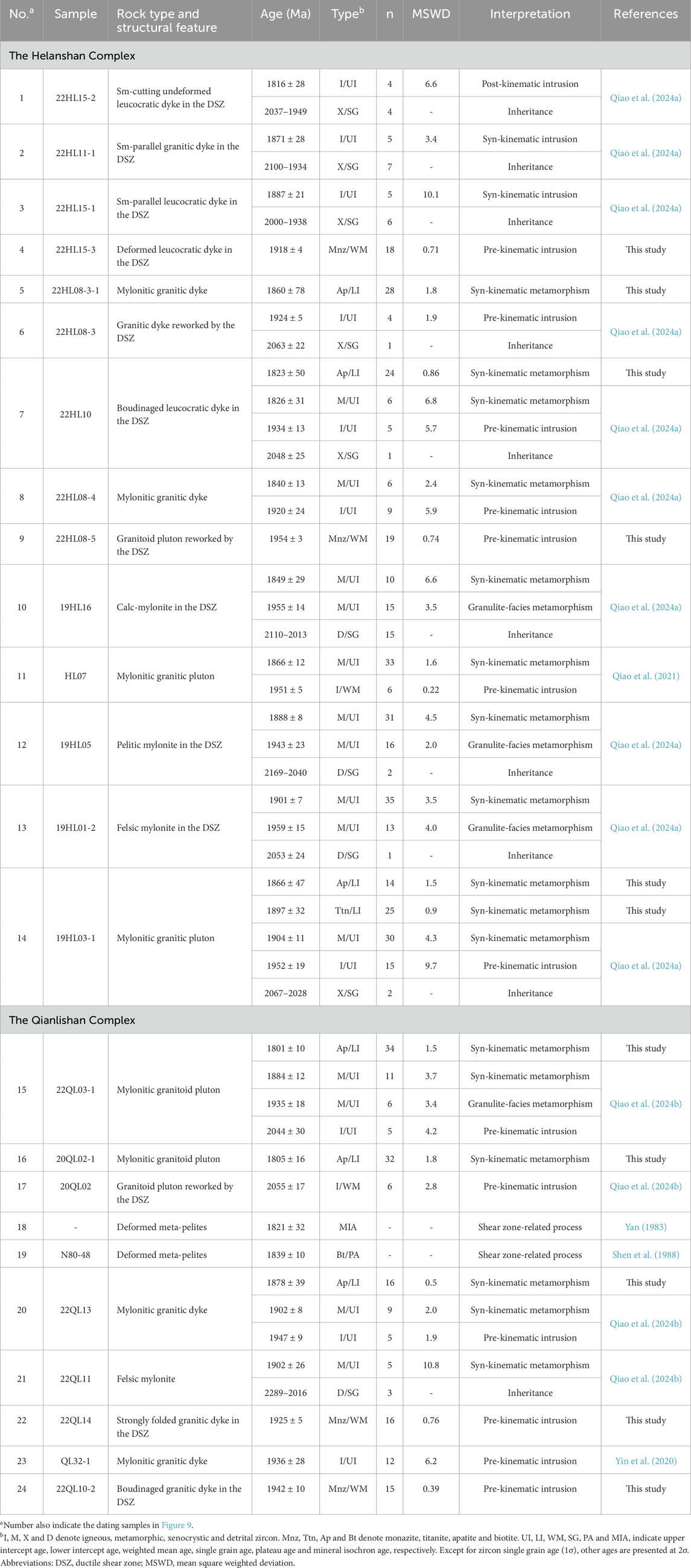
Table 1. Summary of available shear zone-related geochronological data in the western Khondalite Belt.
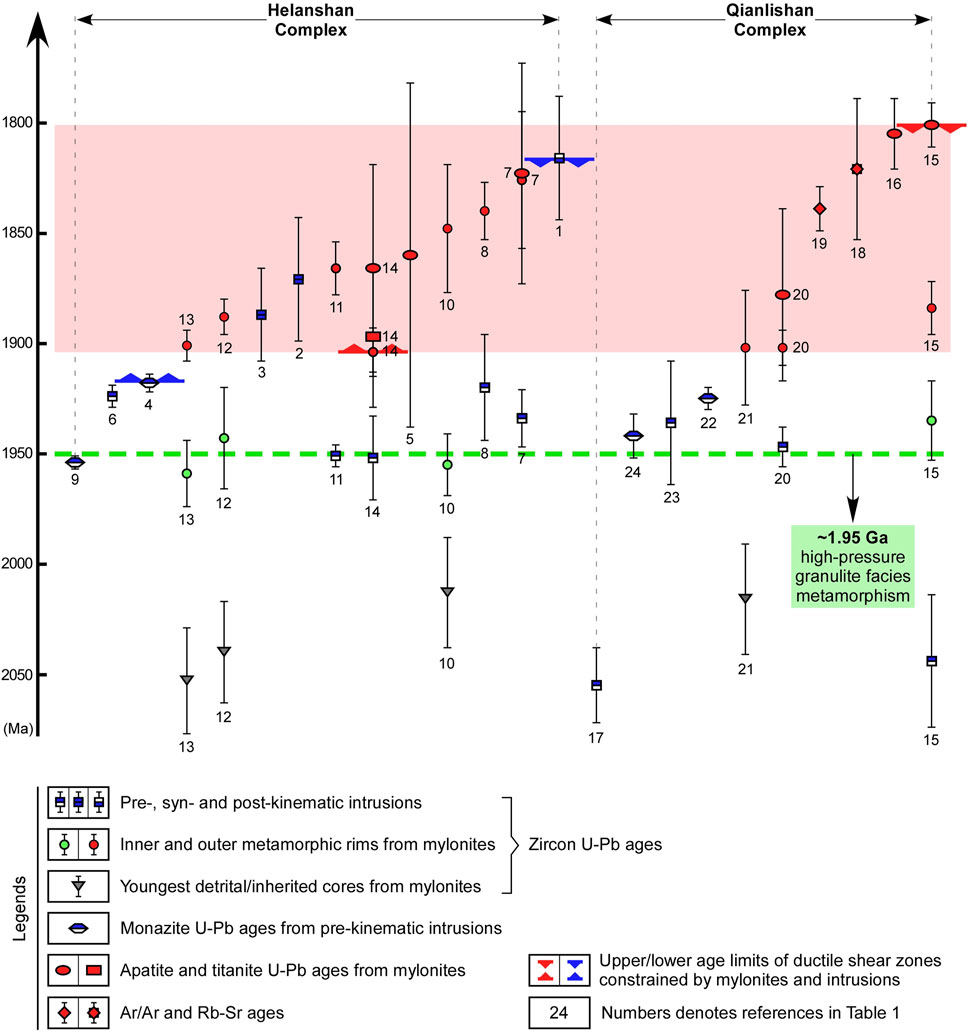
Figure 9. Diagram showing the summary of available shear zone-related geochronological data in the western Khondalite Belt. The structures, age interpretations and references of dating samples are presented in Table 1. See the text for details.
Additionally, the timing of high-temperature ductile shear deformation (i.e., mylonitization) in the HDSZ and QDSZ can be also constrained by dating zircon, titanite and apatite from mylonites. Qiao et al. (2024a) reported that zircon metamorphic overgrowth rims from six mylonitized rocks in the HDSZ gave 207Pb/206Pb ages of 1904–1826 Ma (Table 1). Of particular interest is a 1952 ± 19 Ma granitic pluton underwent shear deformation to become mylonite (Sample 19HL03-1), and it contained zircon overgrowth rims with metamorphic ages of 1904 ± 11 Ma (Qiao et al., 2024a). In the same sample, homogeneous grains and overgrowth rims of titanite gave similar age of 1897 ± 32 Ma (Figure 6). Meanwhile, apatite grains from this sample showed a younger lower intercept age of 1866 ± 47 Ma in the Tera-Wasserburg diagram (Sample 19HL03-1; Figures 7A, 8A). In the HDSZ, another two granitic dykes (1924 ± 5 Ma and 1934 ± 13 Ma; Qiao et al., 2024a) were affected by shear zone activity, and yielded apatite U-Pb ages of 1860 ± 78 Ma and 1823 ± 50 Ma (Samples 22HL08-3-1 and 22HL10; Figures 7B,C, 8B,C), of which the latter is coincident with metamorphic ages of 1826 ± 31 Ma from zircon rims in the same sample. Therefore, we believe that these U-Pb ages from zircon overgrowth rims, titanite and apatite together constrained the timing of the development of HDSZ at ca. 1904–1823 Ma (Figure 9).
In the QDSZ, a 1947 ± 9 Ma granitic dyke was subjected to the mylonitization and yielded U-Pb ages of 1902 ± 8 Ma and 1878 ± 39 Ma from zircon overgrowth rims (Qiao et al., 2024b) and apatite (Sample 22QL13; Figures 7D, 8D), respectively. Comparably, a metamorphic zircon U-Pb age of 1902 ± 26 Ma was also found in felsic mylonite in the Qianlishan Complex (Qiao et al., 2024b). We regard that these ages of 1902–1878 Ma represented the timing of the onset of shear deformation of the QDSZ. In addition, an older 2044 ± 30 Ma granitoid pluton was observed to undergo regional high-grade metamorphism and coevally developed gneissosity at 1935 ± 18 Ma (Qiao et al., 2024b). This gneissic pluton was subsequently reworked by a E-trending shear zone to become granitic mylonite, of which zircon overgrowth rims yielded a younger age group of 1884 ± 12 Ma (Sample 22QL03-1; Qiao et al., 2024b). Notably, apatite grains from this sample are characterized by core-rim textures (Figure 7F), and the rims were dated at 1801 ± 10 Ma (Figure 8F). A similar apatite U-Pb age of 1805 ± 16 Ma was reported from a granitic mylonite in another outcrop of the same shear zone (Sample 20QL02-1; Figures 7E, 8E). These apatite U-Pb ages of 1805–1801 Ma are largely consistent with the Rb-Sr mineral isochron age of 1821 ± 32 Ma (Yan, 1983) and biotite Ar-Ar age of 1839 ± 10 Ma (Shen et al., 1988), obtained from deformed meta-pelites in studied area. Based on these data, we propose that the shear zone activity in the Qianlishan Complex probably continued to ∼1801 Ma (Figure 9). It is worth noting that the above-stated U-Pb ages from the QDSZ are comparable with those from the HDSZ, and they are further inferred to record the deformation age of the orogen-parallel shear zones in the western Khondalite Belt at ca. 1904–1801 Ma (Table 1; Figure 9).
5.2 Tectonic implications
Orogen-parallel ductile shear zones are conspicuous structures throughout the Khondalite Belt, and a robust reconstruction of temporal framework of these structures needs to combine geochronological data that were obtained from different segments of this belt by multiproxy approaches. In the Wulashan-Daqingshan Complex, several pre-kinematic gabbro and granitic dykes were reworked by the nearly E-trending ductile shear zones, four of which displayed magmatic zircon ages ranging from 1987 ± 19 Ma to 1951 ± 9 Ma (Liu et al., 2013; Wan et al., 2013). In the Jining Complex, the Xuwujia norite dyke that underwent ductile shear deformation yielded a crystallization age of 1931 ± 8 Ma (Guo and Zhai, 1992; Peng et al., 2010). The above-stated emplacement ages of 1987–1931 Ma were largely comparable with those igneous zircon and monazite U-Pb ages of 1954–1918 Ma from intrusions that predated the HDSZ and QDSZ in the western Khondalite Belt (Table 1; Figure 9). These U-Pb ages were considered to provide a maximum age limit on the development of orogen-parallel shear zones at ∼1.92 Ga. Meanwhile, syn-kinematic granitic dykes with zircon U-Pb ages of 1887–1858 Ma were reported in the Helanshan and Wulashan-Daqingshan Complexes (Gong et al., 2014; Qiao et al., 2024a). Later, a post-kinematic undeformed granitic dyke (1816 ± 28 Ma; Qiao et al., 2024a) intruded the mylonite in the HDSZ, constraining the minimum deformation age of shearing at ∼1.82 Ga. This inference is supported by the observation that the Xiashihao-Jiuguan shear zone in the Wulashan-Daqingshan Complex was cut by an 1819 ± 3 Ma undeformed granite (Wang et al., 1999). A similar magmatic zircon U-Pb age of 1822 ± 17 Ma from a post-kinematic granite was also obtained in this region (Liu et al., 2013). Consequently, these available geochronological data from shear zone-related igneous rocks unravel that orogen-parallel shear zones have approximately developed in the period between ∼1.92 Ga and ∼1.82 Ga.
In addition to the HDSZ and QDSZ, previous investigations have conducted geochronological studies on the ductile shear zones in the Wulashan-Daqingshan Complex, and metamorphic zircons from five mylonites gave U-Pb ages between 1906 ± 13 Ma and 1853 ± 6 Ma (Liu et al., 2013; Wan et al., 2013). A mylonitic metapelite in the Jining Complex consistently presented a metamorphic zircon U-Pb age of 1866 ± 22 Ma (Li et al., 2011). Comparably, these ages of 1906–1853 Ma showed similarities with those of 1904–1826 Ma and 1902–1884 Ma in the Helanshan and Qianlishan Complexes, respectively (Qiao et al., 2024a; Qiao et al., 2024b). The zircon U-Pb data indicate that the ductile shear zones in the Khondalite Belt probably started to deform at ∼1.90 Ga. This is also evidenced by the titanite U-Pb age of 1897 ± 32 Ma from granitic mylonite in the HDSZ (Table 1; Figure 9). Moreover, Gong et al. (2014) postulated that the shear deformation was likely to begin at ∼1.89 Ga and continued to ∼1.81 Ga, inferred from three biotite Ar-Ar ages (1885 ± 20 Ma, 1819 ± 14 Ma and 1814 ± 13 Ma) from mylonites in the Wulashan-Daqingshan Complex. In this study, we obtained similar apatite U-Pb ages of 1878–1801 Ma from six mylonites in the HDSZ and QDSZ, of which the youngest two were dated at 1805 ± 16 Ma and 1801 ± 10 Ma (Table 1; Figure 9). Coupled with those Ar-Ar ages, we consider that they possibly constrained the end of the shear zone activity at ∼1.80 Ga. In summary, the above-stated geochronological data reveal that orogen-parallel ductile shear zones in the Khondalite Belt most likely developed at 1.90–1.80 Ga.
The Khondalite Belt has been considered as the result of the collision between the northern Yinshan Block and the southern Ordos Block, and the timing of the final amalgamation was constrained at ∼1.95 Ga, mainly evidenced by metamorphic ages of high-pressure pelitic and mafic granulites (e.g., Zhao et al., 2005; Zhao et al., 2012; Yin et al., 2009; Yin et al., 2011; Yin et al., 2023; Guo et al., 2012; Liu et al., 2014; Liu et al., 2017; Cai et al., 2017; Wu et al., 2020; Shi et al., 2025). Collision-induced Paleoproterozoic polyphase deformation have also been recognized in the Khondalite Belt (e.g., Gan and Qian, 1996; Lu et al., 1996; Gong et al., 2014; Yin et al., 2020; Qiao et al., 2022; Qiao et al., 2023). For instance, three major phases of deformation (D1–D3) were proposed in the western Khondalite Belt (Qiao, 2019; Yin et al., 2020; Qiao et al., 2022; Qiao et al., 2023). The D1 deformation primarily produced overturned to recumbent isoclinal folds at small scale, originally (sub-) horizontal penetrative transposition foliations and NNE-SSW mineral lineations, and these D1 structures were inferred to occur at some time from ∼1976 Ma to ∼1932 Ma (Qiao, 2019; Yin et al., 2020; Qiao et al., 2022). The D2 deformation was featured by NW(W)-SE(E)-trending tight to open doubly plunging upright folds, and they probably developed in the period between ∼1932 Ma and ∼1899 Ma (Qiao et al., 2022; Qiao et al., 2023). Subsequently, the D3 deformation gave rise to the development of nearly NE-to E-trending shear zones at ca. 1904–1801 Ma (Qiao et al., 2022; Qiao et al., 2024a; Qiao et al., 2024b; This study). Similar D1–D3 structures were commonly observed in the middle and eastern Khondalite Belt (e.g., Gan and Qian, 1996; Lu et al., 1996; Liu et al., 2022). Notably, the widespread D3-related ductile shear zones have intensively reworked the previous D1–D2 structures and influenced the main tectonic framework of the Khondalite Belt (Gong et al., 2014; Qiao, 2019; Yin et al., 2020; Liu et al., 2022; Qiao et al., 2022; Qiao et al., 2024a; Qiao et al., 2024b). As mentioned above, these orogen-parallel ductile shear zones underwent long-term evolution at 1.90–1.80 Ga (Figure 9), probably related to post-collisional orogenic processes between the Yinshan and Ordos Blocks, and they have played an important role in accommodating post-collisional deformation and tectonic exhumation of the khondalites (e.g., Gong et al., 2014; Liu et al., 2017; Liu et al., 2022; Yin et al., 2020; Qiao et al., 2022; Qiao et al., 2024a).
6 Conclusion
The nearly NE- to E-trending ductile shear zones commonly occurred in the Helanshan and Qianlishan Complexes of the western Khondalite Belt. Pre-kinematic intrusions yielded monazite U-Pb ages of 1953–1918 Ma, indicating that the shear zones probably appeared after ∼1918 Ma. Titanite and apatite U-Pb ages of 1897–1801 Ma were obtained from mylonites, considered to record the timing of shear zone activity. Combined with previous data, we proposed that the Khondalite Belt remarkably developed a series of post-collisional orogen-parallel ductile shear zones at 1.90–1.80 Ga.
Data availability statement
The original contributions presented in the study are included in the article/Supplementary Material, further inquiries can be directed to the corresponding authors.
Author contributions
HQ: Writing – original draft, Writing – review and editing, Conceptualization, Data curation, Funding acquisition, Investigation, Methodology, Supervision. ML: Investigation, Writing – original draft. CD: Investigation, Writing – original draft. SW: Funding acquisition, Investigation, Writing – original draft, Writing – review and editing.
Funding
The author(s) declare that financial support was received for the research and/or publication of this article. This study was supported by the Science & Technology Department of Sichuan Province (Grant No. 2024ZYD0085), National Natural Science Foundation of China (Grant No. 42302222), Leshan Normal University (Grant No. KYCXTD2023-2, KYPY2023-0006 and RC202009), Provincial Natural Science Foundation of Hunan (Grant No. 2024JJ6102) and the Scientific Research Fund of Hunan Provincial Education Department (Grant No. 22A0499).
Acknowledgments
The editors and reviewers are thanked for their helpful and constructive comments on the manuscript.
Conflict of interest
The authors declare that the research was conducted in the absence of any commercial or financial relationships that could be construed as a potential conflict of interest.
Generative AI statement
The authors declare that no Generative AI was used in the creation of this manuscript.
Publisher’s note
All claims expressed in this article are solely those of the authors and do not necessarily represent those of their affiliated organizations, or those of the publisher, the editors and the reviewers. Any product that may be evaluated in this article, or claim that may be made by its manufacturer, is not guaranteed or endorsed by the publisher.
Supplementary material
The Supplementary Material for this article can be found online at: https://www.frontiersin.org/articles/10.3389/feart.2025.1603597/full#supplementary-material
References
Cai, J., Liu, F., and Liu, P. (2017). Paleoproterozoic multistage metamorphic events in Jining metapelitic rocks from the Khondalite Belt in the North China Craton: evidence from petrology, phase equilibria modelling and U–Pb geochronology. J. Asian Earth Sci. 138, 515–534. doi:10.1016/j.jseaes.2017.02.034
Cai, J., Liu, F., Liu, P., Liu, C., Wang, F., and Shi, J. (2014). Metamorphic P–T path and tectonic implications of pelitic granulites from the daqingshan complex of the khondalite belt, north China craton. Precambrian Res. 241, 161–184. doi:10.1016/j.precamres.2013.11.012
Cao, S., and Neubauer, F. (2016). Deep crustal expressions of exhumed strike-slip fault systems: shear zone initiation on rheological boundaries. Earth-Science Rev. 162, 155–176. doi:10.1016/j.earscirev.2016.09.010
Dan, W., Li, X. H., Guo, J. H., Liu, Y., and Wang, X. C. (2012). Integrated in situ zircon U–Pb age and Hf–O isotopes for the Helanshan khondalites in North China Craton: juvenile crustal materials deposited in active or passive continental margin? Precambrian Res. 222, 143–158. doi:10.1016/j.precamres.2011.07.016
Dong, C., Liu, D., Li, J., Wang, Y., Zhou, H., Li, C., et al. (2007). Palaeoproterozoic Khondalite Belt in the western North China Craton: new evidence from SHRIMP dating and Hf isotope composition of zircons from metamorphic rocks in the Bayan Ul-Helan Mountains area. Chin. Sci. Bull. 52, 2984–2994. doi:10.1007/s11434-007-0404-9
Fitch, T. J. (1972). Plate convergence, transcurrent faults, and internal deformation adjacent to southeast Asia and the western Pacific. J. Geophys. Res. 77 (23), 4432–4460. doi:10.1029/JB077i023p04432
Fossen, H., and Cavalcante, G. C. G. (2017). Shear zones–A review. Earth-Science Rev. 171, 434–455. doi:10.1016/j.earscirev.2017.05.002
Gan, S., and Qian, X. (1996). A plate-tectonic model for the evolution of the Daqingshan granulite belt in Inner Mongolia, China. Acta Geol. Sin. 70 (4), 298–308. doi:10.1109/APEC.1997.581475
Gong, W., Hu, J., Wu, S., Chen, H., Qu, H., Li, Z., et al. (2014). Possible southwestward extrusion of the Ordos Block in the Late Paleoproterozoic: constraints from kinematic and geochronologic analysis of peripheral ductile shear zones. Precambrian Res. 255, 716–733. doi:10.1016/j.precamres.2014.05.001
Gou, L., Zi, J., Dong, Y., Liu, X., Li, Z., Xu, X., et al. (2019). Timing of two separate granulite-facies metamorphic events in the Helanshan complex, North China Craton: constraints from monazite and zircon U–Pb dating of pelitic granulites. Lithos 350, 105216. doi:10.1016/j.lithos.2019.105216
Guo, J., Peng, P., Chen, Y., Jiao, S., and Windley, B. F. (2012). UHT sapphirine granulite metamorphism at 1.93–1.92 Ga caused by gabbronorite intrusions: implications for tectonic evolution of the northern margin of the North China Craton. Precambrian Res. 222, 124–142. doi:10.1016/j.precamres.2011.07.020
Guo, J., and Zhai, M. (1992). Mylonite of granulite facies in Xuwujia, nei monggol. Chin. J. Geol. 27 (2), 190–192.
Harrigan, C. O., Trevino, S. F., Schmitz, M. D., and Tikoff, B. (2024). Determining the initiation of shear zone deformation using titanite petrochronology. Earth Planet. Sci. Lett. 631, 118620. doi:10.1016/j.epsl.2024.118620
He, X., Yin, C., Long, X., Qian, J., Wang, L., and Qiao, H. (2017). Archean to Paleoproterozoic continental crust growth in the Western Block of North China: constraints from zircon Hf isotopic and whole-rock Nd isotopic data. Precambrian Res. 303, 105–116. doi:10.1016/j.precamres.2017.02.018
Jiang, X., Yu, S., Liu, Y., Li, S., Lv, P., Peng, Y., et al. (2022). Episodic metamorphism and anatexis within the khondakite belt, north China craton: constraint from late-paleoproterozoic fluid-fluxed melting of the daqingshan complex. Precambrian Res. 369, 106504. doi:10.1016/j.precamres.2021.106504
Jiao, S., Fitzsimons, I. C., and Guo, J. (2017). Paleoproterozoic UHT metamorphism in the Daqingshan Terrane, North China Craton: new constraints from phase equilibria modeling and SIMS U–Pb zircon dating. Precambrian Res. 303, 208–227. doi:10.1016/j.precamres.2017.03.024
Jiao, S., Fitzsimons, I. C., Zi, J., Evans, N. J., Mcdonald, B. J., and Guo, J. (2020). Texturally controlled U–Th–Pb monazite geochronology reveals Paleoproterozoic UHT metamorphic evolution in the Khondalite belt, North China craton. J. Petrol. 61 (1), egaa023. doi:10.1093/petrology/egaa023
Jiao, S., Guo, J., Harley, S. L., and Peng, P. (2013). Geochronology and trace element geochemistry of zircon, monazite and garnet from the garnetite and/or associated other high-grade rocks: implications for Palaeoproterozoic tectonothermal evolution of the Khondalite Belt, North China Craton. Precambrian Res. 237, 78–100. doi:10.1016/j.precamres.2013.09.008
Jiao, S., Guo, J., Wang, L., and Peng, P. (2015). Short-lived high-temperature prograde and retrograde metamorphism in Shaerqin sapphirine-bearing metapelites from the Daqingshan terrane, North China Craton. Precambrian Res. 269, 31–57. doi:10.1016/j.precamres.2015.08.002
Kusky, T. M., Polat, A., Windley, B. F., Burke, K. C., Dewey, J. F., Kidd, W. S. F., et al. (2016). Insights into the tectonic evolution of the North China Craton through comparative tectonic analysis: a record of outward growth of Precambrian continents. Earth-Science Rev. 162, 387–432. doi:10.1016/j.earscirev.2016.09.002
Law, R. D. (2014). Deformation thermometry based on quartz c-axis fabrics and recrystallization microstructures: a review. J. Struct. Geol. 66, 129–161. doi:10.1016/j.jsg.2014.05.023
Li, W., Cao, S., Dong, Y., Zhan, L., and Tao, L. (2023). Crustal anatexis and initiation of the continental-scale Chongshan strike-slip shear zone on the southeastern Tibetan Plateau. Tectonics 42, e2023TC007864. doi:10.1029/2023TC007864
Li, W., Yin, C., Lin, S., Li, W., Gao, P., Zhang, J., et al. (2022). Paleoproterozoic tectonic evolution from subduction to collision of the khondalite belt in north China: evidence from multiple magmatism in the qianlishan complex. Precambrian Res. 368, 106471. doi:10.1016/j.precamres.2021.106471
Li, W., Yin, C., Long, X., Zhang, J., Xia, X., and Wang, L. (2017). Paleoproterozoic S-type granites from the helanshan complex in inner Mongolia: constraints on the provenance and the paleoproterozoic evolution of the khondalite belt, north China craton. Precambrian Res. 299, 195–209. doi:10.1016/j.precamres.2017.07.009
Li, X., Yang, Z., Zhao, G., Grapes, R., and Guo, J. (2011). Geochronology of khondalite-series rocks of the Jining Complex: confirmation of depositional age and tectonometamorphic evolution of the North China craton. Int. Geol. Rev. 53 (10), 1194–1211. doi:10.1080/00206810903548984
Liu, P., Liu, F., Cai, J., Liu, C., Liu, J., Wang, F., et al. (2017). Spatial distribution, P–T–t paths, and tectonic significance of high-pressure mafic granulites from the Daqingshan–Wulashan Complex in the Khondalite Belt, North China Craton. Precambrian Res. 303, 687–708. doi:10.1016/j.precamres.2017.09.004
Liu, P., Liu, F., Liu, C., Liu, J., Wang, F., Xiao, L., et al. (2014). Multiple mafic magmatic and high-grade metamorphic events revealed by zircons from meta-mafic rocks in the Daqingshan–Wulashan Complex of the Khondalite Belt, North China Craton. Precambrian Res. 246, 334–357. doi:10.1016/j.precamres.2014.02.015
Liu, S., Dong, C., Xu, Z., Santosh, M., Ma, M., Xie, H., et al. (2013). Palaeoproterozoic episodic magmatism and high-grade metamorphism in the North China Craton: evidence from SHRIMP zircon dating of magmatic suites in the Daqingshan area. Geol. J. 48 (5), 429–455. doi:10.1002/gj.2453
Liu, T., Li, W., Liu, Y., Jin, W., Zhao, Y., and Iqbal, M. Z. (2022). Deformation characteristics of the high-grade metamorphic and anatectic rocks in the Daqingshan Paleoproterozoic orogenic belt, Inner Mongolia: a case study from the Shijiaqu-Xuehaigou area. Precambrian Res. 374, 106644. doi:10.1016/j.precamres.2022.106644
Lu, L., Xu, X., and Liu, F. (1996). Early precambrian khondalites in north China. Changchun: Changchun Publishing House.
Oriolo, S., Wemmer, K., Oyhantçabal, P., Fossen, H., Schulz, B., and Siegesmund, S. (2018). Geochronology of shear zones–A review. Earth-Science Rev. 185, 665–683. doi:10.1016/j.earscirev.2018.07.007
Paton, C., Hellstrom, J., Paul, B., Woodhead, J., and Hergt, J. (2011). Iolite: freeware for the visualisation and processing of mass spectrometric data. J. Anal. Atomic Spectrom. 26, 2508. doi:10.1039/C1JA10172B
Peng, P., Guo, J. H., Zhai, M. G., and Bleeker, W. (2010). Paleoproterozoic gabbro-noritic and granitic magmatism in the northern margin of the North China craton: evidence of crust–mantle interaction. Precambrian Res. 183, 635–659. doi:10.1016/j.precamres.2010.08.015
Qiao, H. (2019). Structural and geochronological studies of the qianlishan-helanshan complex in north China. Ph. D. Dissertation. Guangzhou, China: Sun Yat-sen University.
Qiao, H., Deng, P., and Li, J. (2023). Geochronological constraints on the origin of the paleoproterozoic qianlishan gneiss domes in the khondalite belt of the North China craton and their tectonic implications. Minerals 13 (11), 1361. doi:10.3390/min13111361
Qiao, H., Liu, M., and Dai, C. (2024b). Timing and tectonic implications of the development of the orosirian qianlishan ductile shear zones in the khondalite belt, north China craton. Minerals 14 (6), 561. doi:10.3390/min14060561
Qiao, H., Yin, C., Li, Q., He, X., Qian, J., and Li, W. (2016). Application of the revised Ti-in-zircon thermometer and SIMS zircon U-Pb dating of high-pressure pelitic granulites from the Qianlishan-Helanshan Complex of the Khondalite Belt, North China Craton. Precambrian Res. 276, 1–13. doi:10.1016/j.precamres.2016.01.020
Qiao, H., Yin, C., Xiao, W., Zhang, J., Qian, J., and Wu, S. (2022). Paleoproterozoic polyphase deformation in the helanshan complex: structural and geochronological constraints on the tectonic evolution of the khondalite belt, north China craton. Precambrian Res. 368, 106468. doi:10.1016/j.precamres.2021.106468
Qiao, H., Yin, C., Zhang, J., Qian, J., and Wu, S. (2021). New discovery of ∼1866 Ma high-temperature mylonite in the helanshan complex: marking a late-stage ductile shearing in the khondalite belt, north China craton. Acta Geol. Sinica-English Ed. 95 (4), 1418–1419. doi:10.1111/1755-6724.14749
Qiao, H., Zhao, G., Yin, C., Qian, J., Wu, S., Deng, P., et al. (2024a). Geochronology of the paleoproterozoic helanshan ductile shear zones: insights into temporal framework of polyphase deformation in the khondalite belt, north China craton. Precambrian Res. 410, 107481. doi:10.1016/j.precamres.2024.107481
Qiu, E., Zhang, Y., Larson, K. P., and Li, B. (2023). Dating strike-slip ductile shear through combined zircon-, titanite-and apatite U–Pb geochronology along the southern Tan-Lu Fault zone, East China. Tectonics 42, e2022TC007734. doi:10.1029/2022TC007734
Ramsay, J. (1980). Shear zone geometry: a review. J. Struct. Geol. 2 (1-2), 83–99. doi:10.1016/0191-8141(80)90038-3
Reiners, P. W., Ehlers, T. A., and Zeitler, P. K. (2005). Past, present, and future of thermochronology. Rev. Mineral. Geochem. 58 (1), 1–18. doi:10.2138/rmg.2005.58.1
Ren, H., Hu, J., Li, S., Zhou, D., Somerville, I., Wang, L., et al. (2024). Early palaeozoic affinity of hainan island to gondwana: new clues from metamorphic monazite and titanite of the baoban complex. Lithos 482, 107708. doi:10.1016/j.lithos.2024.107708
Ribeiro, B. V., Kirkland, C. L., Kelsey, D. E., Reddy, S. M., Hartnady, M. I., Faleiros, F. M., et al. (2023). Time-strain evolution of shear zones from petrographically constrained Rb–Sr muscovite analysis. Earth Planet. Sci. Lett. 602, 117969. doi:10.1016/j.epsl.2022.117969
Santosh, M., Liu, D., Shi, Y., and Liu, S. (2013). Paleoproterozoic accretionary orogenesis in the North China Craton: a SHRIMP zircon study. Precambrian Res. 227, 29–54. doi:10.1016/j.precamres.2011.11.004
Schoene, B. (2014). U–Th–Pb geochronology. Treatise Geochem. 4, 341–378. doi:10.1016/b978-0-08-095975-7.00310-7
Shen, Q., Fu, Y., and Zhang, M. (1988). 40Ar/39Ar dating on biotite from the qianlishan group in bohai bay, nei monggol. Bull. Inst. Geol. Chin. Acad. Geol. Sci. 18, 68–74.
Shi, Q., Shu, R., Chen, X., Zhao, G., Chen, Y., Wang, Z., et al. (2025). Paleoproterozoic granite in the khondalite belt, northern margin of the North China craton: response to the assembly of the columbia supercontinent. Precambrian Res. 417, 107640. doi:10.1016/j.precamres.2024.107640
Shi, Q., Xu, Z., Santosh, M., Ding, D., Zhao, Z., Li, C., et al. (2023). Mantle magmatism, metamorphism and anatexis: evidence from geochemistry and zircon U–Pb-Hf isotopes of paleoproterozoic S-type granites, khondalite belt of the North China craton. Int. Geol. Rev. 65 (6), 943–968. doi:10.1080/00206814.2022.2151048
Sibson, R. H. (1977). Fault rocks and fault mechanisms. J. Geol. Soc. 133 (3), 191–213. doi:10.1144/gsjgs.133.3.0191
Simonetti, M., Carosi, R., Montomoli, C., Law, R. D., and Cottle, J. M. (2021). Unravelling the development of regional-scale shear zones by a multidisciplinary approach: the case study of the Ferriere-Mollières Shear Zone (Argentera Massif, Western Alps). J. Struct. Geol. 149, 104399. doi:10.1016/j.jsg.2021.104399
Sun, S., and Dong, Y. (2023). High temperature ductile deformation, lithological and geochemical differentiation along the Shagou shear zone, Qinling Orogen, China. J. Struct. Geol. 167, 104791. doi:10.1016/j.jsg.2023.104791
van der Pluijm, V. A., Mezger, K., Cosca, M. A., and Essene, E. J. (1994). Determining the significance of high-grade shear zones by using temperature-time paths, with examples from the Grenville orogen. Geology 22, 743–746. doi:10.1130/0091-7613(1994)022<0743:dtsohg>2.3.co;2
Vermeesch, P. (2018). IsoplotR: a free and open toolbox for geochronology. Geosci. Front. 9 (5), 1479–1493. doi:10.1016/j.gsf.2018.04.001
Wan, Y., Liu, D., Dong, C., Xu, Z., Wang, Z., Wilde, S. A., et al. (2009). The precambrian khondalite belt in the daqingshan area, north China craton: evidence for multiple metamorphic events in the palaeoproterozoic era. Geol. Soc. Lond. Spec. Publ. 323 (1), 73–97. doi:10.1144/SP323.4
Wan, Y., Song, B., Liu, D., Wilde, S. A., Wu, J., Shi, Y., et al. (2006). SHRIMP U–Pb zircon geochronology of Palaeoproterozoic metasedimentary rocks in the North China Craton: evidence for a major Late Palaeoproterozoic tectonothermal event. Precambrian Res. 149 (3-4), 249–271. doi:10.1016/j.precamres.2006.06.006
Wan, Y., Xu, Z., Dong, C., Nutman, A., Ma, M., Xie, H., et al. (2013). Episodic Paleoproterozoic (∼2.45, ∼1.95 and ∼1.85Ga) mafic magmatism and associated high temperature metamorphism in the Daqingshan area, North China Craton: SHRIMP zircon U–Pb dating and whole-rock geochemistry. Precambrian Res. 224, 71–93. doi:10.1016/j.precamres.2012.09.014
Wang, H., Yuan, G., Xing, H., and Wang, J. (1999). One the tectonic implication for Xiashihao-Jiuguan ductile shear zone in Guyang-Wuchuan area, Inner Mongolia. Prog. Precambrian Res. 22 (1), 1–20.
Wang, L., Guo, J., Yin, C., and Peng, P. (2017). Petrogenesis of ca. 1.95 Ga meta-leucogranites from the jining complex in the khondalite belt, north China craton: water-fluxed melting of metasedimentary rocks. Precambrian Res. 303, 355–371. doi:10.1016/j.precamres.2017.04.036
Wei, C., Zhai, M., and Wang, B. (2023). Four phases of Orosirian metamorphism in the north North China Craton (NNCC): insights into the regional tectonic framework and evolution. Earth-Science Rev. 241, 104449. doi:10.1016/j.earscirev.2023.104449
Wu, S., Yin, C., Davis, D. W., Zhang, J., Qian, J., Qiao, H., et al. (2020). Metamorphic evolution of high-pressure felsic and pelitic granulites from the Qianlishan Complex and tectonic implications for the Khondalite Belt, North China Craton. Geol. Soc. Am. Bull. 132 (11-12), 2253–2266. doi:10.1130/B35502.1
Wu, S., Yin, C., Qian, J., Qiao, H., Wang, X., Xia, Y., et al. (2024). Paleoproterozoic ultrahigh-temperature metamorphism in the Helanshan Complex, North China Craton: new constraints from chloritized sapphirine-bearing pelitic granulites. Lithos 488, 107814. doi:10.1016/j.lithos.2024.107814
Xia, X., Sun, M., Zhao, G., and Luo, Y. (2006a). LA-ICP-MS U–Pb geochronology of detrital zircons from the Jining Complex, North China Craton and its tectonic significance. Precambrian Res. 144 (3-4), 199–212. doi:10.1016/j.precamres.2005.11.004
Xia, X., Sun, M., Zhao, G., Wu, F., Xu, P., Zhang, J., et al. (2006b). U–Pb and Hf isotopic study of detrital zircons from the Wulashan khondalites: constraints on the evolution of the Ordos Terrane, Western Block of the North China Craton. Earth Planet. Sci. Lett. 241 (3-4), 581–593. doi:10.1016/j.epsl.2005.11.024
Xing, L., Li, W., Zang, M., Liu, H., Chen, W., and Zhang, M. (2024). Genesis of the sediment-hosted qukuleke Au deposit in the east kunlun orogenic belt, NW China: constraints from geology, apatite U–Pb age, and C–O–S–Pb isotopes. Ore Geol. Rev. 170, 106126. doi:10.1016/j.oregeorev.2024.106126
Xu, X., Gou, L., Dong, Y., Zhang, C., Long, X., Zhao, Y., et al. (2023). Metamorphic P–T evolution of the pelitic granulites from the Helanshan in the North China Craton revealed by phase equilibrium modeling and garnet trace elements: implications for Paleoproterozoic collisional orogenesis. Precambrian Res. 394, 107093. doi:10.1016/j.precamres.2023.107093
Xu, X., Gou, L., Long, X., Dong, Y., Liu, X., Zi, J., et al. (2018). Phase equilibrium modelling and SHRIMP zircon U–Pb dating of medium-pressure pelitic granulites in the Helanshan complex of the Khondalite Belt, North China Craton, and their tectonic implications. Precambrian Res. 314, 62–75. doi:10.1016/j.precamres.2018.05.030
Yan, Y. (1983). “A study on P-T condition and mineralogy of the metamorphic complex of the Qianlishan Group, Nei Mongol,” in Institute of geology, Chinese academy of sciences. Petrology research (second collection) (Beijing: Geological Publishing House).
Yin, C., Qiao, H., Lin, S., Li, C., Zhang, J., Qian, J., et al. (2020). Deformation history of the Qianlishan complex, Khondalite belt, North China: structures, ages and tectonic implications. J. Struct. Geol. 141, 104176. doi:10.1016/j.jsg.2020.104176
Yin, C., Zhao, G., Guo, J., Sun, M., Xia, X., Zhou, X., et al. (2011). U–Pb and Hf isotopic study of zircons of the helanshan complex: constrains on the evolution of the khondalite belt in the western block of the North China craton. Lithos 122 (1-2), 25–38. doi:10.1016/j.lithos.2010.11.010
Yin, C., Zhao, G., and Sun, M. (2015). High-pressure pelitic granulites from the helanshan complex in the khondalite belt, north China craton: metamorphic P-T path and tectonic implications. Am. J. Sci. 315 (9), 846–879. doi:10.2475/09.2015.03
Yin, C., Zhao, G., Sun, M., Xia, X., Wei, C., Zhou, X., et al. (2009). LA-ICP-MS U–Pb zircon ages of the qianlishan complex: constrains on the evolution of the khondalite belt in the western block of the North China craton. Precambrian Res. 174 (1-2), 78–94. doi:10.1016/j.precamres.2009.06.008
Yin, C., Zhao, G., Wei, C., Sun, M., Guo, J., and Zhou, X. (2014). Metamorphism and partial melting of high-pressure pelitic granulites from the Qianlishan Complex: constraints on the tectonic evolution of the Khondalite Belt in the North China Craton. Precambrian Res. 242, 172–186. doi:10.1016/j.precamres.2013.12.025
Yin, C., Zhao, G., Xiao, W., Lin, S., Gao, R., Zhang, J., et al. (2023). Paleoproterozoic accretion and assembly of the Western Block of North China: a new model. Earth-Science Rev. 241, 104448. doi:10.1016/j.earscirev.2023.104448
Zhai, M. (2022). Khondalite revisited-record of special geological processes on Earth. Acta Petrol. Sin. 96 (9), 2967–2997. doi:10.3969/j.issn.0001-5717.2022.09.003
Zhang, H., Hou, G., Zhang, B., and Tian, W. (2022). Kinematics, temperature and geochronology of the Qingyi ductile shear zone: tectonic implications for late Neoarchean microblock amalgamation in the Western Shandong Province, North China craton. J. Struct. Geol. 161, 104645. doi:10.1016/j.jsg.2022.104645
Zhao, G., Cawood, P. A., Li, S., Wilde, S. A., Sun, M., Zhang, J., et al. (2012). Amalgamation of the North China craton: key issues and discussion. Precambrian Res. 222, 55–76. doi:10.1016/j.precamres.2012.09.016
Zhao, G., Sun, M., Wilde, S. A., and Li, S. (2005). Late archean to paleoproterozoic evolution of the North China craton: key issues revisited. Precambrian Res. 136 (2), 177–202. doi:10.1016/j.precamres.2004.10.002
Zhao, G., and Zhai, M. (2013). Lithotectonic elements of Precambrian basement in the North China Craton: review and tectonic implications. Gondwana Res. 23 (4), 1207–1240. doi:10.1016/j.gr.2012.08.016
Zhou, X., and Geng, Y. (2009). Metamorphic age of the khondalites in the helanshan region: constraints on the evolution of the western block in the North China craton. Acta Petrol. Sin. 25, 1843–1852.
Zhou, X., Zhao, G., and Geng, Y. (2010). Helanshan high-pressure pelitic granulites: petrological evidence for collision event in the Western Block of the North China Craton. Acta Petrol. Sin. 26, 2113–2121. doi:10.3724/SP.J.1084.2010.00199
Zhu, W., Tian, W., Wang, B., Zhang, Y., and Wei, C. (2023). Paleoproterozoic crust–mantle interaction in the khondalite belt, north China craton: constraints from geochronology, elements, and Hf-O-Sr-Nd isotopes of the layered complex in the jining terrane. Minerals 13 (4), 462. doi:10.3390/min13040462
Keywords: shear zone, mylonite, U-Pb geochronology, Orosirian, Khondalite Belt, North China Craton
Citation: Qiao H, Liu M, Dai C and Wu S (2025) Dating the Orosirian orogen-parallel shear zones in the western Khondalite Belt, North China Craton: new constraints from monazite, titanite and apatite U-Pb ages. Front. Earth Sci. 13:1603597. doi: 10.3389/feart.2025.1603597
Received: 31 March 2025; Accepted: 24 April 2025;
Published: 14 May 2025.
Edited by:
Xiaoguang Liu, Shandong University of Science and Technology, ChinaReviewed by:
Chen Zhao, Shenyang Center of China Geological Survey, ChinaQiang Shi, Liaoning Technical University, China
Copyright © 2025 Qiao, Liu, Dai and Wu. This is an open-access article distributed under the terms of the Creative Commons Attribution License (CC BY). The use, distribution or reproduction in other forums is permitted, provided the original author(s) and the copyright owner(s) are credited and that the original publication in this journal is cited, in accordance with accepted academic practice. No use, distribution or reproduction is permitted which does not comply with these terms.
*Correspondence: Hengzhong Qiao, cWlhb2h6aEBsc251LmVkdS5jbg==; Shangjing Wu, d3VzaGoyNUBoeW51LmVkdS5jbg==