- 1Williamson Research Centre for Molecular Environmental Science, School of Earth and Environmental Sciences, University of Manchester, Manchester, United Kingdom
- 2National Nuclear Laboratory, Warrington, United Kingdom
The safe disposal of nuclear waste necessitates a thorough understanding of the role microorganisms play on the fate of the waste materials over time. Despite significant volumetric contributions to nuclear waste inventories, little is known about the fate of plastics under the high pH and irradiating conditions of a geological repository. We conducted microbial enrichment experiments to assess the potential for additives in plasticised polyvinyl chloride (PVC) sheet, commonly used in the nuclear industry, to fuel nitrate reduction by a microbial community adapted to grow at pH 10. These additives include plasticisers and flame retardants, amongst others. PVC powder (no additives) served as an additive-free control, and samples of both materials were gamma irradiated (1 MGy) under hyperalkaline conditions, representative of conditioned nuclear waste, to assess the effect of irradiation on bioavailability. Plasticised PVC supported near-complete nitrate reduction, whether irradiated or not, although irradiated PVC sheet supported less nitrate reduction. No nitrate reduction was observed with non-irradiated PVC powder, although irradiated powder supported minor nitrate reduction. These results highlight the bioavailability of volumetrically significant plasticised PVC under conditions relevant to its geological disposal in cementitious intermediate level nuclear waste and highlight the critical need to constrain downstream effects on biogeochemical processes ultimately impacting on the safety case for disposal.
Introduction
The safe disposal of nuclear waste in a geological disposal facility (GDF) demands a thorough understanding of biogeochemical interactions between waste materials and surrounding geosphere. Key to this is understanding the role microorganisms may play in the degradation of organic materials within nuclear waste, and the implications of microbial processes on the chemical speciation, and hence mobility, of key radionuclides in groundwaters permeating the GDF. Research to date has focused on cellulosic materials (e.g., Small et al., 2008), showing that alkaline hydrolysis products can be biodegraded to support microbial life under hyperalkaline (pH > 12) conditions (Bassil et al., 2015; Rout et al., 2015), limiting their radionuclide complexation properties. Although anthropogenic organic waste materials (e.g., plastics and resins) are considered less reactive than cellulose, they represent the majority of the organic inventory in intermediate and low level nuclear waste. There remains a critical need to address their microbial biodegradability under GDF conditions, and their resulting influences on the transport of radionuclides to the biosphere.
Plastics represent a significant volumetric contribution of organic material in intermediate and low level waste inventories worldwide (Abrahamsen et al., 2015). In the UK, halogenated plastics constitute the largest component of the organic-containing waste inventory (NDA, 2014). This waste arises from the use of plastics in maintenance and decommissioning operations at nuclear power plants, and from reprocessing plants and laboratories. PVC is widely used for glove box posting bags, protective suits, and tenting operations. The bulk of the PVC in the UK National Inventory is expected to be flexible films and sheets of PVC derived from these activities (Smith et al., 2013).
Owing to strong intermolecular forces between polymer chains, pure PVC is a rigid. PVC must therefore be rendered flexible in order to be useful for a wide array of applications, which necessitates a variety of additives, including plasticisers, heat stabilizers, fillers, pigments, flame retardants, UV absorbers, colorants, and anti-oxidants (Coaker, 2003). Of these, plasticisers are typically present in the largest quantities, accounting for 30–50% by volume. Historically, the most widely used plasticisers have been phthalate esters, which represented 93% of plasticisers declared in 1993 (European Commission, 2000). Phthalate esters are known to be bioavailable to common soil microorganisms, with more rapid degradation of lower molecular weight phthalates noted (Engelhardt and Wallnöfer, 1975, 1978).
Another significant group of additives present in flexible PVC is flame retardants. The high chlorine content (~57%) of pure PVC renders the polymer inherently flame retardant, however addition of phthalate esters and other organic additives contribute to increased flammability (Coaker, 2003). A common approach to reduce flammability is to supplement the volume of phthalate plasticisers with flame retardant compounds, most typically phosphate esters (Moy, 1998). Triaryl phosphate esters, such as triphenyl phosphate (TPP), are desirable since they serve as plasticisers as well as flame retardants (Coaker, 2003). They have been shown to serve as the sole carbon source for some microbial communities, and some are known to readily biodegrade aerobically and anaerobically in soil (Pickard et al., 1975; Anderson et al., 1997). It is anticipated that TPP and other phosphate ester flame retardants will be abundant in the waste inventory (Baston and Dawson, 2012).
Additives such as plasticisers and flame retardants are not covalently bonded to the PVC polymer, rather they sit between and serve to lubricate otherwise rigid polymer layers. There is concern, therefore, that these additives (e.g., plasticisers and flame retardants) may diffuse out of the PVC material under the high pH and irradiating conditions of a cementitious GDF, with implications for microbial activity and radionuclide mobility (Dawson, 2013). Indeed, phthalate esters are known to form complexes with uranium and other radionuclides (Vazquez et al., 2008, 2009). It is therefore essential that any assessment of organic waste bioavailability in a GDF scenario consider the additives present in PVC, as well as the polymer itself. Here we report on experiments designed to test the hypothesis that additives in plasticised PVC represent a source of carbon and energy for nitrate reducing microorganisms in a high pH environment, relevant to a cementitious GFD. Nitrate is widely anticipated to be present in radioactive waste and to serve as an alternative electron acceptor to oxygen post-closure, prior to conditions favoring reduction of iron and sulfate (McCabe, 1990; Rizoulis et al., 2012; Abrahamsen et al., 2015; Bassil et al., 2015). Nitrate reduction was also found to support the microbial degradation of isosaccharinic acid under alkaline conditions using microorganisms from the same high pH environment (Bassil et al., 2015). As such, this metabolism was deemed highly relevant with which to test the bioavailability of additives in plasticised PVC.
Materials and Methods
PVC Materials and Irradiation
To assess bioavailability of additives used in plasticised PVC, two forms of PVC were used; pure (additive-free) PVC powder (average Mw ~ 43,000, average Mn ~ 22,000, CAS 9002-86-2, Sigma-Aldrich, UK), and PVC sheeting, previously used in a tenting operation at the UK National Nuclear Laboratory. Aliquots of 0.5 g PVC powder or 24 squares of PVC sheet (0.75 cm2 each; collective weight ≈ 0.5 g) were added to 2 ml Ca(OH)2 (1.5 g/L) in pre-scored 5 ml glass ampules (Wheaton, Millville NJ, USA), and flame sealed with an air headspace. Sixteen PVC powder and 20 PVC sheet ampules were irradiated to average cumulative doses of 1.01 ± 0.03 MGy and 1.00 ± 0.01 MGy, respectively, from a 60Co source, roughly equivalent to that received by intermediate level waste after several 1000 years (Abrahamsen et al., 2015; Brown et al., 2017). Irradiations began within 1 week of sealing ampules. Samples were stored at room temperature in the dark prior to use in enrichment experiments.
Microbial Enrichment Tests
To assess bioavailability, enrichments were established with pure and plasticised PVC (irradiated and not irradiated) supplied as sole carbon and electron donor sources for nitrate reduction mediated by a high pH-adapted microbial community. The sediment used as the inoculum was collected from a legacy lime works site in Harpur Hill, Buxton, UK. This environment is broadly analogous to a cementitious radioactive waste repository and is known to harbor high pH-adapted microorganisms, including nitrate-reducing bacteria (Rizoulis et al., 2012; Bassil et al., 2015).
The contents of each of the four batches of PVC ampules (powder and sheet, irradiated and non-irradiated) were homogenized so that PVC added to enrichments was equivalent in terms of volume and (where applicable) radiation dose received. For experiments with PVC powder, 1.5 ml of powder-leachate slurry was added to 35 ml sterile serum vials inside an anaerobic chamber, and 15 ml anoxic sterile medium was added. For experiments with PVC sheet, 12 PVC squares (0.75 cm2) and 1 ml of leachate were transferred to serum vials containing 15 ml medium. The basal medium used across the experiments has been described previously (Nixon et al., 2017). Nitrate reduction tests were amended with 20 mM NaNO3 as the electron acceptor, and 5 mM each of lactate and acetate was added to positive controls as electron donors. Experiments were initiated in triplicate and inoculated with 1% v/v water-sediment slurry. Enrichments were pH-corrected to pH 10 using 10 N sodium hydroxide prior to incubation. Enrichments were incubated at 20°C in the dark for a total of 97 days.
Based on results from pyrolysis GC-MS analysis (see below), additional enrichment experiments were conducted to test the potential for the PVC additives triphenyl phosphate (TPP; Sigma-Aldrich, UK) and phthalate (in the form of phthalic acid; Sigma-Aldrich, UK) to fuel nitrate reduction at pH 10. In the PVC sheet used in this study, these additives serve as a flame retardant and plasticiser, respectively. Phthalate was used instead of a specific phthalate ester since these esters are known to break down into their constituent aliphatic alcohols and phthalate at high pH (Baston and Dawson, 2012). The same conditions were used in these experiments as outlined above, with these compounds supplied as the sole source of carbon and electron donors instead of PVC powder/sheet. TPP and phthalate were tested separately by adding 2 mM to the basal medium. Positive, negative, and sterile controls were established as before, and all enrichments were inoculated with the same batch of sediment inoculum (1% v/v) used in the PVC enrichments.
Analytical Methods
Pyrolysis GC-MS was used to assess bulk organic chemistry of PVC powder and sheet before and after addition of Ca(OH)2 and flame sealing in glass ampules (with or without irradiation). Small aliquots were placed into clean fire-polished quartz tubes and pyrolised in a two-step procedure at 300 and 750°C. This approach was chosen in an attempt to analyse the additives (not covalently bonded to the PVC; desorbed at the lower temperature) independently to the PVC polymer itself (pyrolysed at the higher temperature). At each temperature, samples were heated in a CDS-5200 pyroprobe for 5 s in a flow of helium. The liberated moieties were transferred to an Agilent 7980A gas chromatograph (GC) via a heated transfer line. The GC was fitted with a Zebron ZB-5MS column (Zebron ZB-5MS, 30 m × 250 μm × 0.25 μm, Phenomenex, CA, USA) and coupled to an Agilent 5975 MSD singe quadrapole mass spectrometer (MS) operated in scan mode (scanning a range of m/z 50 to 650 at 2.7 scans s−1; ionization energy 70 eV). The pyrolysis transfer line and rotor oven were set at 325°C, the heated GC interface to 300°C, the electron ionization source at 230°C and the MS quadrapole at 150°C. Helium was used as the carrier gas and the sample itself was introduced in split mode (ratio 75:1, constant flow of 1 ml min−1, gas saver mode active). The oven was programmed from 40 to 300°C increasing at 4°C min−1 and held at 300°C for 10 min. Major compounds in each sample were identified using the NIST Standard Reference Database.
Concentrations of nitrate and nitrite in all enrichment experiments were quantified using ion exchange high performance liquid chromatography (IE-HPLC). Samples (1 ml) were extracted from replicates using a syringe and needle flushed with nitrogen gas. Samples were centrifuged (13,000 g for 7 min at room temperature) to remove bacteria and any solid material, and the supernatant diluted 100-fold with deionised water. Diluted samples were injected onto a Dionex ICS5000 Dual Channel Chromatograph via a Dionex AS-AP autosampler (Thermo Fisher Scientific, Waltham, MA, USA). Molecule separation was achieved by passing samples through a 250 × 0.4 mm Dionex AS11-HC capillary column with a 4 μm pore size, operating with a flow rate of 0.015 ml min−1 and a pressure of 3,400 psi. The mobile phase used was a KOH solution, electronically injected to produce a gradient from 1 to 40 mM.
Total dissolved organic carbon (TOC) concentrations in the liquid phase of enrichment experiments were measured with the high-temperature catalytic oxidation method (680°C) using a TOC analyser (Shimadzu TOC-VCPN, Japan). Samples of supernatant (600 μl) that had been centrifuged at room temperature (14,000 g for 7 min) were diluted 25-fold and introduced to the TOC analyser using an autosampler (ASI-V, Shimadzu, Milton Keynes, UK). Concentrations of total organic carbon and inorganic carbon were obtained from five-point potassium hydrogen phthalate and sodium carbonate standard calibration curves, respectively.
Environmental Scanning Electron Microscopy (ESEM) was used to obtain images of the non-irradiated and irradiated PVC sheet. Single PVC squares were taken from the incubation end-points of enrichments inside an anaerobic chamber (Coy, Grass Lake, MI, USA). PVC squares were submerged in deionised water and gently agitated for a few seconds to remove salts, and air-dried overnight prior to gold-coating for observation in high vacuum mode with an ESEM-Field Emission Gun (ESEM-FEG; FEI XL30).
Microbial Community Analysis
Microbial community composition was examined through the analysis of 16S rRNA gene sequences obtained from DNA collected at days 0 and 97 of the PVC experiment. DNA was extracted at day 0 of the experiment by combining 1 ml samples from all enrichments and at the end of the experiment from 1.2 ml of pooled samples from each triplicate enrichment. In all instances, DNA was extracted using the MoBio PowerLyzer™ PowerSoil DNA Isolation Kit (MoBio Laboratories, Inc., Carlsbad, CA, USA) according to the manufacturer's instructions. Polymerase chain reaction (PCR) was used to amplify the V4 hyper variable regions of the 16S rRNA gene (forward primer, 515F, 5′-GTGYCAGCMGCCGCGGTAA-3′; reverse primer, 806R, 5′-GGACTACHVGGGTWTCTAAT-3′) for 2 × 250-bp pair-end sequencing using the Illumina MiSeq platform [Illumina, San Diego, CA, USA; (Caporaso et al., 2011, 2012)]. PCR amplification was performed using Roche FastStart High Fidelity PCR System (Roche Diagnostics Ltd, Burgess Hill, UK) in 50 μl reactions under the following conditions; initial denaturation at 95°C for 2 min, followed by 36 cycles of 95°C for 30 s, 55°C for 30 s, 72°C for 1 min, and a final extension step of 72°C for 5 min. PCR products were purified and normalized to ~20 ng each using the SequalPrep Normalization Kit (Fisher Scientific, Loughborough, UK). A negative PCR control was conducted in parallel to PVC experiment samples, and found to be devoid of DNA. To rule out contamination from DNA extraction kits that has come to light since these samples were processed (e.g., Sheik et al., 2018), we conducted duplicate DNA extraction controls using the same batch of kits under the same conditions, with only the extraction kit reagents as a potential source of DNA. The resulting extracts were found to be devoid of DNA, as were PCR products following 16S rRNA amplification. The sequencing run was performed using a 4 pM sample library spiked with 4 pM PhiX to a final concentration of 10% following Kozich et al. (2013). Raw sequences were divided into samples by barcodes (up to one mismatch permitted) using a sequencing pipeline. Quality control and trimming was performed using Cutadapt (Martin, 2011), FastQC (http://www.bioinformatics.babraham.ac.uk/projects/fastqc/) and Sickle (Joshi and Fass, 2011). MiSeq error correction was performed using SPADes (Nurk et al., 2013). Forward and reverse reads were incorporated into full-length sequences with Pandaseq (Masella et al., 2012). Chimeras were removed using ChimeraSlayer (Haas et al., 2011) and Operational Taxonomic Units (OTUs) were generated with UPARSE (Edgar, 2013). OTUs were classified by Usearch (Edgar, 2010) at the 97% similarity level, and singletons removed. Rarefraction analysis was conducted using the original detected OTUs in Qiime (Caporaso et al., 2010). Taxonomic assignment was performed by the Ribosomal Database Project (RDP) classifier using an 80% confidence limit (Wang et al., 2007).
Accession Numbers
Raw sequencing data obtained in this study are available at the NCBI Sequence Read Archive (http://www.ncbi.nlm.nih.gov/sra/) under the accession numbers SRR5936785–SRR5936794.
Results
Effects of Radiation
Treatment of pure PVC powder and plasticised PVC sheet with 1 MGy γ-radiation led to changes in the appearance of these materials. PVC powder turned from white to black, whilst PVC sheet turned from translucent with a feint blue hue to a brown opaque color, and appeared to be less flexible. The pH of the homogenized leachate from irradiated PVC powder and sheet vials was measured at 0.3 and 6.6, respectively. In contrast, the pH of the leachate from non-irradiated PVC powder was 10.2 and that of PVC sheet was 8.4. The effects of irradiation on the PVC sheet were also evident at the microscopic scale, characterized by an increase in surface roughness and appearance of large cracks (see Figure 1).
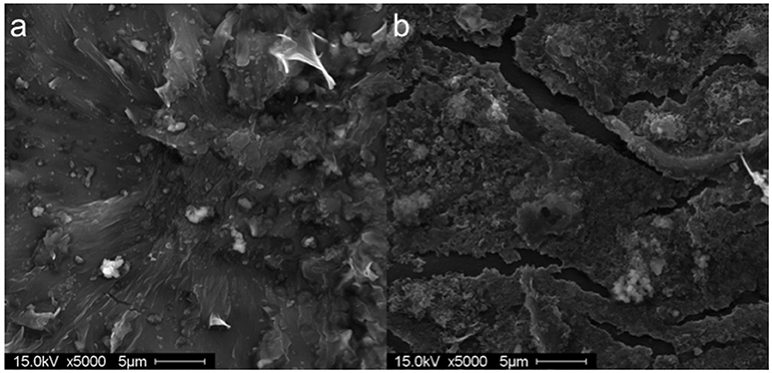
Figure 1. The effects of radiation on PVC sheet at the microscopic scale. Pictured are representative SEM micrographs of non-irradiated (A) and irradiated (B) PVC sheet, exhibiting relatively smooth surface features in the former compared with the coarse surface and appearance of cracks in the latter.
Pyrolysis moieties of the PVC polymer (high temperature step) were consistent with those reported elsewhere, the most prominent of these being benzene, propene, toluene, and xylene moieties (see Supplementary Figures S1, S2; O'Mara, 1970; Ma et al., 2004). Peaks corresponding to these compounds in the pyrolysis GC-MS chromatograms were evident after treatment at high pH and with irradiation, although their relative intensities appeared depleted in the latter (see Supplementary Figure S1C). Pyrolysis GC-MS of the PVC sheet at 300°C indicated the presence of aryl phosphate ester (mostly TPP) and phthalate ester (mostly dinonyl phthalate) moieties in all sheet materials analyzed (Figure 2).
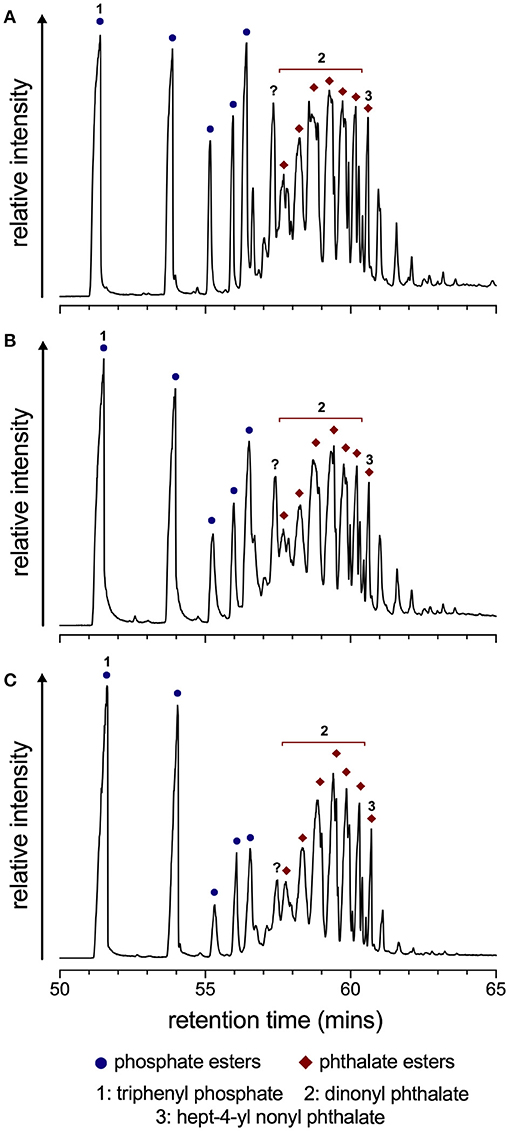
Figure 2. PVC additive moieties detected in PVC sheet starting material (A), after prolonged contact with pH 12.4 Ca(OH)2 solution (B), and after irradiation in addition to contact with pH 12.4 Ca(OH)2 solution (C). Data were obtained using using pyrolysis GC-MS at 300°C. Peaks of interest are labeled according to NIST database-resolved identities, where blue circles denote phosphate esters and red diamonds phthalate esters. The peak labeled with a question mark could not confidently be identified, but its relative abundance decreases from graphs (A–C).
These represent the additives that were desorbed at this lower temperature, and no significant differences could be observed between the starting material (Figure 2A) and samples exposed to high pH (Figure 2B) and high pH as well as ionizing radiation (Figure 2C). Although the relative intensities of some peaks relative to others were lower in those exposed to high pH and radiation compared with the starting material, phthalate, and phosphate moieties appeared to be equally affected, indicating that no preferential loss of one over the other of these groups of additives occurred. Of note, however, is the successive decrease in abundance of the peak labeled with a question mark in Figure 2. A search against the NIST Standard Reference Database returned the compound 4,8-ditert-butyl-N,N-diethyl-2,10-dimethyl-12H-dibenzo[d,g][1,3,2]dioxaphosphocin-6-amine, which to the best of our knowledge is not recognized as a known PVC additive and hence its identity is considered ambiguous.
Enrichment Results
Results from PVC enrichment experiments are summarized in Figure 3. No nitrate reduction was observed with non-irradiated PVC powder, whereas minor nitrate reduction was observed with irradiated PVC powder (3.5 ± 2.6 mM reduced, 2.2 ± 0.7 mM produced; see Figure 3A). In contrast, 90% nitrate reduction was observed in non-irradiated PVC sheet enrichments (19.4 ± 1.7 mM reduced, 13.6 ± 2.4 mM produced), and 63% nitrate reduction was observed with irradiated PVC sheet (13.1 ± 1.9 mM reduced, 12.2 ± 1.4 mM produced; see Figure 3B). By day 35 of the experiment, complete nitrate reduction was observed in the positive control (containing 24 mM nitrate, 5 mM each of lactate and acetate as electron donors), leading to the production of 15.4 ± 2.9 mM nitrite. No nitrate reduction or nitrite production was observed in the negative (no electron donors supplied) or sterile controls.
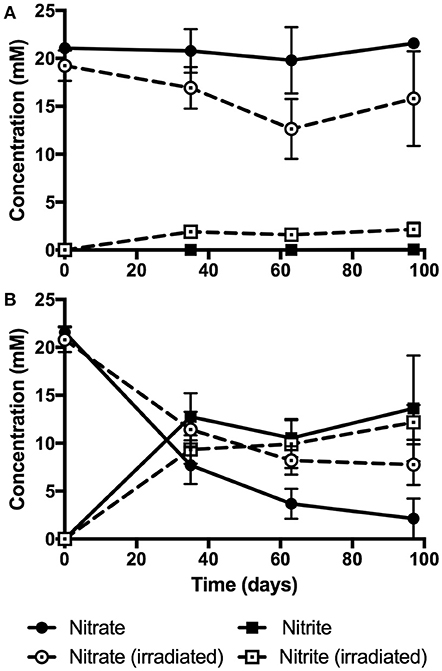
Figure 3. Results from pH 10 nitrate reduction enrichment experiments with non-irradiated and irradiated PVC powder (A) and sheet (B). Results are expressed as average mM concentrations of nitrate (circles) and nitrite (squares) over the 97 days of incubation, as measured by ion chromatography. Dashed lines and open shapes denote irradiated enrichments. Error bars represent standard deviation of triplicate measurements.
Results from enrichment experiments with TPP or phthalate show minor amounts of nitrate reduction were observed with the former (Figure 4A), as evidenced by the minor but significant increase in nitrite over the duration of the experiment (p-value: 0.05). In contrast, phthalate was not found to support nitrate reduction (no significant difference in nitrate concentrations between the phthalate test and no donor control; Figure 4B).
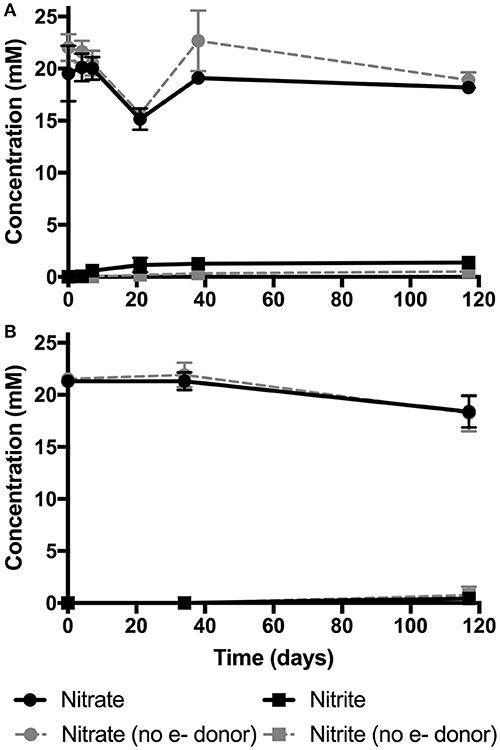
Figure 4. Results from pH 10 nitrate reduction enrichment experiments with TPP (A) and phthalate (B). Results are expressed as average mM concentrations of nitrate (circles) and nitrite (squares) over the 117 days of incubation, as measured by ion chromatography. Controls in which no electron donors were supplied are shown with dashed gray lines for nitrate (circles) and nitrite (squares) in both experiments.
Dissolved organic carbon (DOC) concentrations were observed to increase over the duration of all PVC enrichment experiments, whether supplied with a live or heat-sterilized inoculum, or containing irradiated or non-irradiated PVC materials (Figure 5). Overall concentrations of DOC were higher in PVC sheet enrichments compared with PVC powder enrichments, attributable to the presence of additional organic compounds (Figure 2A).
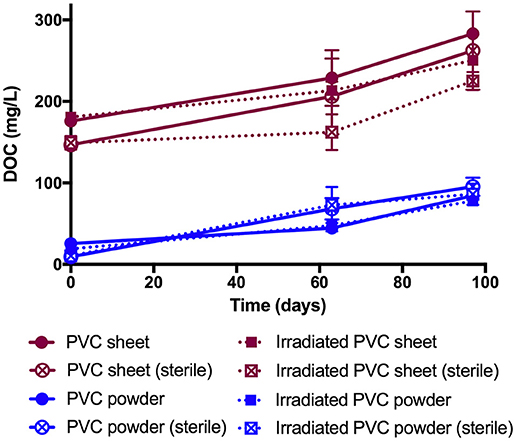
Figure 5. Concentration of DOC measured in PVC enrichment experiments, expressed as mg per liter with time. The aqueous phase of PVC powder enrichments (blue) contain less organic carbon than that of the PVC sheet enrichments owing to the lack of additives. There is no significant difference between both irradiated vs. non-irradiated and sterile vs. live tests (2 tailed student's t-test, p-values > 0.05).
Microbial Community Composition
Changes in the microbial community composition of the enrichments were analyzed using 16S rRNA gene sequencing, and family-resolved community composition at the start and end-points of PVC enrichments are summarized in Figure 6. A total of 811 operational OTUs were detected in the inoculum, compared with 624 and 427 OTUs in the end-points of the irradiated and non-irradiated PVC powder enrichments, respectively. The irradiated and non-irradiated PVC sheet enrichments contained 244 and 325 OTUs, respectively. The 16S rRNA genes detected were dominated by those corresponding to Bacteria (93.5%), with only 6.5% affiliated with Archaea. By the end-points of all PVC enrichments Archaea represented less than 1% of the community (0.2 and 0.4% in non-irradiated and irradiated PVC powder, respectively, and 0.02 and 0.08% in non-irradiated and irradiated PVC sheet, respectively). Family-level diversity is summarized in Figure 6, alongside overall diversity indices (Shannon's H values) based on OTU numbers. Overall diversity was highest in the inoculum, and decreased by the end-point of all enrichment experiments. The highest end-point diversity was observed in the irradiated PVC powder enrichments and the lowest diversity was noted in the PVC sheet enrichments. For both PVC powder and sheet enrichment end points, the higher diversity corresponds to the highest rates of nitrate reduction observed (irradiated PVC powder, non-irradiated PVC film; Figure 6).
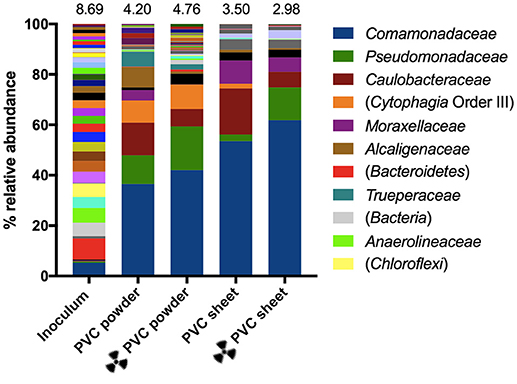
Figure 6. Family-level microbial community composition of the inoculum and end-points of the PVC nitrate reduction enrichments. Families that constituted less than 1% combined abundance were omitted. Where family level could not be resolved, the last matched taxonomic level of identification is given in parentheses. Only taxa that represent 5% or more of the community in any one sample have been included in the legend. Above each bar is the Shannon's H index of diversity based on raw OTU data for each sample.
Family-resolved taxonomy indicates an overall increase in all PVC enrichments of organisms affiliated with the Comamonadaceae, from 4.0% in the inoculum to up to 60.7% in the irradiated PVC sheet end-point. Other families observed to be enriched across all conditions include the Pseudomonadaceae (0.4% in the inoculum, 11.1% in PVC powder, 16.8% in irradiated PVC powder, 2.6% in PVC sheet, 12.78% in irradiated PVC sheet), and Caulobacteraceae (0.1% in the inoculum, 12.6% in PVC powder, 6.7% in PVC irradiated powder, 18.1% in PVC sheet, 6.1% in irradiated PVC sheet). Present at only 0.2% in the inoculum, Moraxellaceae were found to increase in relative abundance in all but the irradiated PVC powder enrichments (4.0% in PVC powder, 0.1% in irradiated PVC powder, 9.0% in PVC sheet, and 5.7% in irradiated PVC sheet). Of those OTUs resolved to genus level, organisms most closely affiliated with Pseudomonas species were prominent in the end-points of all enrichments, representing 2.6 and 12.8% of non-irradiated and irradiated PVC sheet enrichments, respectively, compared with 11.1 and 16.8% in non-irradiated and irradiated PVC powder enrichments, respectively (0.4% in inoculum). Sequences most closely related to species of Brevundimonas and Acinetobacter were both found to be abundant in PVC sheet enrichments despite representing less than 1% of the inoculum. Brevundimonas species constituted 18.1 and 6.1% in non-irradiated and irradiated PVC sheet enrichments respectively. Organisms closely related to Acinetobacter species represented 9.0% of the non-irradiated PVC sheet end-point, compared with 5.7% of the irradiated equivalent. In PVC powder enrichments, sequences closely associated to the genus Hydrogenophaga were abundant, constituting only 0.1% of the inoculum but 16.7 and 29.4% of non-irradiated and irradiated PVC powder enrichment end-points, respectively. In contrast this genus was only present at 0.004% of both irradiated and non-irradiated PVC sheet enrichment end-points.
Discussion
Plasticised PVC is a widely used material in the nuclear industry and therefore represents a significant volume of low and intermediate level nuclear waste. Whether this material can fuel microbial processes under geodisposal conditions is a critical gap in our understanding of the long-term biogeochemical evolution of a GDF. This study shows that plasticised PVC sheet, a volumetrically significant component of low and intermediate level nuclear waste, can fuel nitrate reduction at pH 10. Although gamma irradiation at the doses used here enhanced the bioavailability of the pure polymer (Figure 3A), irradiated PVC sheet was rendered less bioavailable, allowing for almost 30% less nitrate reduction than non-irradiated PVC sheet (Figure 3B). It is plausible that irradiation reduced the degree to which additives were able to leach out of the PVC sheet, essentially locking in the additives that were otherwise bioavailable in the non-irradiation setup (Figure 3). A lack of nitrate reduction in enrichments amended with either triphenyl phosphate (TPP) or phthalate (Figure 4) suggests that PVC sheet additives other than these (such as an unidentified moiety highlighted in Figure 2), or possibly the alcohol groups of the phthalates identified in Figure 2, are fuelling this process. Alkaline hydrolysis led to increased concentrations of dissolved organic carbon in all PVC enrichments, indicating that such hydrolysis is an important step in PVC degradation.
Based on observed OTUs, a number of family-resolved taxa were enriched in all enrichment end-points. Most notable among these are representatives of the Comamonadaceae, the dominant family in all end-points but more abundant in PVC sheet (>50% abundance) compared with powder enrichments (<40% abundance; Figure 6). Given that high levels of nitrate reduction were observed in PVC sheet (but not powder) enrichments, it is possible that members of the Comamonadaceae contributed to the observed levels of nitrate reduction. Members of this family are common in water and soil environments, and its genera and species are highly metabolically diverse. More than half of the known genera within this family include nitrate-reducing strains, and many more are known to draw upon a wide array of organic substrates for their metabolism (Willems, 2014).
Previous studies have assessed microbial degradability of plasticised PVC, yet none have done so under high pH conditions relevant to nuclear waste disposal. In spite of this, findings from previous studies are consistent with those reported here. For instance, whilst plasticised PVC is widely known to be susceptible to biodeterioration (Mersiowsky et al., 2001; Kaczmarek and Bajer, 2007), the PVC polymer matrix itself is not (Booth and Robb, 1968; Mersiowsky et al., 2001). Booth and Robb (1968) inoculated pH neutral soils in which plasticised PVC was buried with species of Pseudomonas and Brevibacterium, and found adipate, azelate, and sebacate plasticisers were susceptible to bacterial degradation, yet phthalate, and phosphate esters present in the PVC were resistant, consistent with results of this study. In contrast, a large number of bacteria are known to draw upon phthalate esters as their sole carbon source, including strains belonging to Pseudomonadaceae and Moraxellaceae found to be abundant in the PVC sheet enrichments reported here [(Vamsee-Krishna and Phale, 2008) and references therein; Figure 6]. The soil bacterium Pseudomonas sp. strain P136 can couple the degradation of phthalate and its derivatives to nitrate reduction (Nozawa and Maruyama, 1988), in contrast to the findings of this study in which phthalate was not found to support nitrate reduction (Figure 4). Results from our experiments suggest that phthalate and phosphate plasticisers are less bioavailable for bacterial metabolism at high pH.
A number of studies have addressed the chemical and radiolytic degradation of PVC and common additives under GDF-relevant conditions (Colombani et al., 2009; Shashoua, 2012; Dawson, 2013; Wypych, 2015). The PVC base polymer is widely known to degrade under irradiating conditions, with direct formation of double radicals leading to chain scissions and the formation of hydrochloric acid. The presence of air during irradiation leads to more chain scissions to the polymer (Wypych, 2015). The enhanced nitrate reduction observed in our study with irradiated compared with non-irradiated PVC powder is therefore likely due to the radiolysis of the polymer into breakdown products that can support microbial metabolism. Irradiation of plasticised PVC was found to liberate 100 times more organic compounds than that of pure PVC, the majority of which were found to be additives leached out from the polymer and their degradation products (Colombani et al., 2009). Our results suggest that irradiation, at maximal levels, renders PVC additives less accessible for microbial metabolism, though the mechanism underpinning this remains to be characterized and warrants further study. In particular, long term radiolysis could impact on the pH of the system, resulting in increased microbial activity, especially where electron acceptors that are not energetically favorable at high pH are present (e.g., sulfate reduction; Rizoulis et al., 2012).
Aside from radiolytic damage, PVC and its additives are known to degrade under high pH conditions alone. One experiment compared the effects of aging plasticised PVC in deionized water (pH 7) compared with calcium hydroxide (pH 12), and the latter gave rise to a 30% mass loss compared with 2% in water, attributable to alkaline hydrolysis (Baston and Dawson, 2012). The same mechanism appears to break ester linkages of common phthalate plasticisers, liberating aliphatic alcohols and phthalic acid (Baston and Dawson, 2012), and may explain the increase in DOC observed in experiments reported here regardless of whether materials were irradiated (Figure 5). Indeed, although phthalic acid (supplied to enrichments as phthalate) was found not to support nitrate reduction, it is possible that the aliphatic alcohols liberated from the phthalate esters in the PVC sheet did.
By conducting these experiments under conditions relevant to a nuclear waste geological disposal facility containing cementitious waste, we have demonstrated that additives in plasticised PVC are likely to fuel microbial metabolism, in this case via nitrate reduction. Whilst nitrate is expected to be present in reprocessing wastes, where PVC is commonly used, or in the waste forms themselves within a GDF (McCabe, 1990), microorganisms present in and around a GDF are likely to respire other electron acceptors, such as ferric iron, sulfate, and carbonate. Furthermore, although we used sediment from a high pH environment as our inoculum, the groups of bacteria that were enriched in these experiments are common to soil and water. Therefore, whilst alkalitolerant and alkaliphilic microorganisms will prosper in the early stages of the GDF, plasticised PVC is likely to be bioavailable for a wide range of indigenous microorganisms and over long timescales.
We have shown that additives in plasticised PVC can fuel anaerobic microbial processes at high pH. Our results suggest that irradiated plasticised PVC, subjected to a maximal total dose likely for intermediate level waste, is up to 30% less available for nitrate reduction than its non-irradiated counterpart, likely owing to reduced leachability of additives from this material. An essential next step is to assess which additives, or combinations of additives, are ultimately fueling nitrate reduction, what effect these additives have on radionuclide mobility, whether they can fuel gas generation processes, and therefore constrain their overall impact on the safety case for nuclear waste disposal. This work constitutes the critical first step to elucidate these processes.
Author Contributions
SN, JS, and JL designed the experiments. SN conducted the experiments. CB carried out DNA extractions and sequencing. BvD and SN carried out pyrolysis GC-MS analysis. SN carried out data analysis, prepared figures, and wrote the manuscript, with contributions from all authors.
Funding
This research is part of the European Commission Horizon 2020 MIND (Microbiology in Nuclear waste Disposal) project, and has received funding from the Euratom research and training programme 2014–2018 under Grant Agreement no. 861880. The authors also acknowledge funding from NERC (BIGRAD consortium, grant number NE/H007768/1L) and the Royal Society.
Conflict of Interest Statement
The authors declare that the research was conducted in the absence of any commercial or financial relationships that could be construed as a potential conflict of interest.
Acknowledgments
The authors would like to acknowledge Alastair Bewsher for ion chromatography and total organic carbon analysis.
Supplementary Material
The Supplementary Material for this article can be found online at: https://www.frontiersin.org/articles/10.3389/fenvs.2018.00097/full#supplementary-material
References
Abrahamsen, L., Arnold, T., Brinkmann, H., Leys, N., Merroum, M., Mijnendonckx, K., et al. (2015). A Review of Anthropogenicorganic Wastes and Their Degradation Behavior. Mincrobiology in Nucelar Waste Disposal Deliverable report. Available online at: http://www.mind15.eu/wp-content/uploads/2015/11/MIND-Deliverable-1.1-Review-of-anthropogenic-organic-wastes.pdf.
Anderson, C., Wischer, D., Schmieder, A., and Spiteller, M. (1997). Fate of tripheny phosphate in soil. Chemisohere 27, 869–879. doi: 10.1016/0045-6535(93)90017-Y
Bassil, N. M., Bryan, N., and Lloyd, J. R. (2015). Microbial degradation of isosaccharinic acid and high pH. ISME 9, 310–320. doi: 10.1038/ismej.2014.125
Baston, G., and Dawson, J. (2012). NAPL Generation From PVC Aged in Aqueus Solutions. AMEC Report AMEC/PPE/002834/003.
Booth, G. H., and Robb, J. A. (1968). Bacterial degradation of plasticised PVC-effect on some physical properties. J. Appl. Chem. 18, 194–197.
Brown, A. R., Small, J. S., Pimblott, S. M., Goodacre, R., and Lloyd, J. R. (2017). The Impact of Ionizing Radiation on Microbial Cells Pertinent to the Geological Disposal of Radioactive Waste. Contractor Report to RWM RWM/UManchester/01.
Caporaso, J. G., Kuczynski, J., Stombaugh, J., Bittinger, K., Bushman, F. D., Costello, E. K., et al. (2010). QIIME allows analysis of high-thoughput community sequencing data. Nat. Methods 7, 335–336. doi: 10.1038/nmeth.f.303
Caporaso, J. G., Lauber, C. L., Walters, W. A., Berg-Lyons, D., Huntley, J., Fierer, N., et al. (2012). Ultra-high-throughput microbial community alanysis on the Illumina HiSeq and MiSeq platforms. ISME 6, 1612–1624. doi: 10.1038/ismej.2012.8
Caporaso, J. G., Lauber, C. L., Walters, W. A., Berg-Lyons, D., Lozupone, C. A., Tunrnbaugh, P. J., et al. (2011). Global patterns of 16S rRNA diversity at a depth of millions of sequences per sample. PNAS 108, 4516–4522. doi: 10.1073/pnas.1000080107
Coaker, W. (2003). Fire and flame retardants for PVC. J. Vinyl Add. Technol. 9, 108–115. doi: 10.1002/vnl.10072
Colombani, J., Herbette, G., Rossi, C., JoussotDubien, C., Labed, V., and Gilardi, T. (2009). Leaching of plasticised PVC: effect of irradiation. J. Appl. Polym. Sci. 112, 1372–1377. doi: 10.1002/app.29612
Dawson, J. (2013). The Potenial for Non-aqueous Phase Liquid Production From Irradiated PVC and Ninylesterstyrene (VES). Report to NDA RWMD AMEC/PPE-1008/001 Issue 02.
Edgar, R. C. (2010). Search and clustering orders of magnitude faster than BLAST. Bioinformatics 26, 2460–2461. doi: 10.1093/bioinformatics/btq461
Edgar, R. C. (2013). UPARSE: highly accurate OUT sequences from microbial amplicon reads. Nat. Methods 10, 996–998. doi: 10.1038/nmeth.2604
Engelhardt, G., and Wallnöfer, P. R. (1975). Metabolism of di- and mono-n-butyl phthalate and related dialkyl phthalates. Bull. Environ. Contam. Toxicol. 13, 342–347. doi: 10.1007/BF01685348
Engelhardt, G., and Wallnöfer, P. R. (1978). Metabolism of di- and mono-n-butyl phthalate by soil bacteria. Appl. Environ. Microbiol. 35, 243–246.
Haas, B. J., Gevers, D., Earl, A. M., Feldgarden, M., Ward, D. V., Giannoukos, G., et al. (2011). Chimeric 16S rRNA sequence formation and detection in Sanger and 454-pyrosequences PCR amplicons. Genome Res. 21, 494–504. doi: 10.1101/gr.112730.110
Joshi, N. A., and Fass, J. N. (2011). Sickle: A Sliding-Window, Adaptive, Quality-Based Trimming Tool for FastQ Files (Version 1.33) [Software].
Kaczmarek, H., and Bajer, K. (2007). Biodegradation of plasticized poly(vinyl chloride) containing cellulose. J. Polymer Sci. B Polymer Phys. 45, 903–919. doi: 10.1002/polb.21100
Kozich, J. J., Westcott, S. L., Baxter, N. T., Highlander, S. K., and Schloss, P. D. (2013). Development of a dual-index sequencing strategy and curation pipeline for analysing amplicon sequence data on the MiSeq Illumina sequencing platform. Appl. Environ. Microbiol. 79, 5112–5120. doi: 10.1128/AEM.01043-13
Ma, S., Lu, J., and Gao, J. (2004). Study on the pyrolysis dechlorination of PVC waste. Energy Sour. 26, 387–396. doi: 10.1080/009083197352
Martin, M. (2011). Cutadapt removes adapter sequences from high-throughput sequencing reads. EMBnet J. 17, 10–12. doi: 10.14806/ej.17.1.200
Masella, A. P., Bartram, A. K., Truszkowski, J. M., Brown, D. G., and Neufeld, J. D. (2012). PANDAseq: paired-end assembler for Illumina sequences. BMC Bioinformatics 13:31. doi: 10.1186/1471-2105-13-31
McCabe, A. (1990). The potential significance of microbial activity in radioactive waste disposal. Cell. Mol. Life Sci. 46, 779–787.
Mersiowsky, I., Weller, M., and Ejlertsson, J. (2001). Fate of plasticised PVC products under landfill conditions: a laboratory-scale landfill simulation reactor study. Water Resour. 35, 3063–3070. doi: 10.1016/S0043-1354(01)00027-6
Moy, P. Y. (1998). FR characteristics of phosphate ester plasticizers with inorganic additives in PVC. J. Vinyl Addit. Technol. 4, 22–25. doi: 10.1002/vnl.10004
NDA (2014). Radioactive Wastes in the UK: A Summary of the 2013 Inventory. NDA. Available online at: http://ukinventory.nda.gov.uk/wp-content/uploads/sites/18/2014/02/14D039-NDASTSTY140006-UKRWI-2013-High-Level-Summary.pdf.
Nixon, S. L., Walker, L., Streets, M. D. T., Eden, B., Boothman, C., Taylor, K. G., et al. (2017). Guar gum stimulates biogenic sulfide production at elevated pressures: implications for shale gas extraction. Front. Microbiol. 8:679. doi: 10.3389/fmicb.2017.00679
Nozawa, T., and Maruyama, Y. (1988). Denitrification by a soil bacterium with phthalate and other aromatic compounds as substrates. J. Bacteriol. 170, 2501–2505.
Nurk, S., Bankevich, A., Antipov, D., Gurevich, A. A., Korobeynikov, A., Lapidus, A., et al. (2013). Assembling single-cell genomes and mini-metagenomes from chimeric MDA products. J. Comp. Biol. 20, 714–737. doi: 10.1089/cmb.2013.0084
O'Mara, M. M. (1970). High-temperature pyrolysis of poly(vinyl chloride): gas chromatographic-mass spectrometric analysis of the pyrolysis products from PVC resin and plastisols. J. Polymer Sci. A Polymer Chem. 8, 1887–1899. doi: 10.1002/pol.1970.150080724
Pickard, M. A., Whelihan, J. A., and Westlake, D. W. (1975). Utilisation of triaryl phosphates by a mixed bacterial population. Can. J. Microbiol. 21, 140–145. doi: 10.1139/m75-021
Rizoulis, A., Steele, H. M., Morris, K., and Lloyd, J. R. (2012). The potential impact of anaerobic microbial metabolism during the geological disposal of intermediate-level waste. Mineral. Mag. 78, 3261–3270. doi: 10.1180/minmag.2012.076.8.39
Rout, S. P., Charles, C. J., Dougleris, C., McCarthy, A. J., Rooks, D. J., Loughnane, J. P., et al. (2015). Anoxic biodegradation of isosaccharinic acids at alkaline pH by natural microbial communities. PLoS ONE 10:e0137682. doi: 10.1371/journal.pone.0137682
Sheik, C. S., Reese, B. K., Twing, K. I., Sylvan, J. B., Grim, S. L., Schrenk, M. O., et al. (2018). Identification and removal of contaminant sequences from ribosomal gene databases: lessons from the census of deep life. Front. Microbiol. 9:840. doi: 10.3389/fmicb.2018.00840
Small, J., Nykyri, M., Helin, M., Hovi, U., Sarlin, T., and Itävaara, M. (2008). Experimental and modelling investigations of the biogeochemistry of gas production from low and intermediate level radioactive waste. Appl. Geochem. 23, 1383–1418. doi: 10.1016/j.apgeochem.2007.11.020
Smith, V., Magalhaes, S., and Schneider, S. (2013). The Role of PVC Additives in the Potential Formation of NAPLs. Report to NDA RWMD AMEC/PPE-2834/E-2001.
Vamsee-Krishna, C., and Phale, P. S. (2008). Bacterial degradation of phthalate isomers and their esters. Indian J. Microbiol. 48, 19–34. doi: 10.1007/s12088-008-0003-8
Vazquez, G. J., Dodge, C. J., and Francis, A. J. (2008). Interaction of uranium(VI) with phthalic acid. Inorg. Chem. 47, 10739–10743. doi: 10.1021/ic801230s
Vazquez, G. J., Dodge, C. J., and Francis, A. J. (2009). Bioreduction of U(VI)-phthalate to a polymeric U(VI)-phthalate colloid. Inorg. Chem. 48, 9485–9490. doi: 10.1021/ic900694k
Wang, Q., Garrity, G. M., Tiedje, J. M., and Cole, J. R. (2007). Naïve Bayesian classifier for rapid assignment of rRNA sequences into the new bacterial taxonomy. Appl. Environ. Microbiol. 73, 5261–5267. doi: 10.1128/AEM.00062-07
Willems, A. (2014) “The family comamonadaceae,” in The Prokaryotes, eds E. Rosenberg, E. F. DeLong, S. Lory, E. Stackebrandt, and F. Thompson (Berlin; Heidelberg: Springer), 777–851.
Keywords: PVC, nuclear waste disposal, nitrate reduction, irradiation, bioavailability
Citation: Nixon SL, van Dongen BE, Boothman C, Small JS and Lloyd JR (2018) Additives in Plasticised Polyvinyl Chloride Fuel Microbial Nitrate Reduction at High pH: Implications for Nuclear Waste Disposal. Front. Environ. Sci. 6:97. doi: 10.3389/fenvs.2018.00097
Received: 05 March 2018; Accepted: 17 August 2018;
Published: 11 September 2018.
Edited by:
Bradley M. Tebo, Oregon Health & Science University, United StatesReviewed by:
John Senko, University of Akron, United StatesJohn W. Moreau, The University of Melbourne, Australia
Copyright © 2018 Nixon, van Dongen, Boothman, Small and Lloyd. This is an open-access article distributed under the terms of the Creative Commons Attribution License (CC BY). The use, distribution or reproduction in other forums is permitted, provided the original author(s) and the copyright owner(s) are credited and that the original publication in this journal is cited, in accordance with accepted academic practice. No use, distribution or reproduction is permitted which does not comply with these terms.
*Correspondence: Sophie L. Nixon, c29waGllLm5peG9uQG1hbmNoZXN0ZXIuYWMudWs=