- 1Life and Environmental Sciences Department, School of Natural Sciences, University of California, Merced, Merced, CA, United States
- 2Institut Sénégalais de Recherches Agricoles Rte des Hydrocarbures, Dakar, Senegal
- 3École National Supérieure d'Agriculture (ENSA), Thiès, Senegal
- 4Department of Earth Sciences, University of California, Riverside, Riverside, CA, United States
- 5School of Environment and Natural Resources, The Ohio State University, Columbus, OH, United States
Hydraulic redistribution (HR) by woody vegetation has been proposed as a potential water source for crops in intercropped systems. The native woody shrub, Guiera senegalensis J.F. Gmel, grows in the fields of farmers across the African Sahel and has shown profound yield benefits to associated pearl millet (Pennisetum glaucum) crops, especially in drought years. We tested whether this benefit resulted from the shrubs performing hydraulic redistribution (HR) with pearl millet using some of this HR water. During an experimentally imposed drought, an enriched deuterium (2H) water tracer applied to 1 m deep roots of G. senegalensis shrubs was detected (2H ≥ +300‰) in aboveground stems of intercropped millet within 12–96 h of tracer introduction. The only viable path for the 2H-enriched H2O into millet was via HR by the shrubs, which confirmed active HR during the time when growing millet was under severe drought stress. Millet biomass production when intercropped with shrubs was over 900% greater than crops grown without shrubs present. Improvement of growing conditions previously found near shrubs cannot fully account for the benefit to associated millet under extreme drought stress without considering the positive impact of the transfer of HR water. This finding illuminates HR and water transfer as an important mechanism in a successful agroforestry system in a region where food security is a serious issue.
Introduction
Plant roots spanning spatially disconnected areas of soil moisture can serve as conduits to redistribute moisture within the soil profile. Breazeale (1930) and Breazeale and Crider (1934) performed experiments investigating the ability of catclaw (Acacia gregii), date palm (Phoenix dactylifera), corn (Zea mays), and tomato (Lycopersicon esculentum Mil.) to grow roots into soil below the wilting point and then translocate moisture from disconnected wet soil into dry soil layers over a period of days to weeks in pot and field culture. Further studies by Breazeale and Crider (1934) with tomato plants identified the potential for this translocated moisture to help nearby wheat (Triticum aestivum) seedlings survive otherwise lethal levels of soil moisture stress in a pot-culture. Many years later, the phenomenon was studied in a natural system by Mooney et al. (1980) who found increased soil moisture overnight in the dry shallow soil under phreatophytic Prosopis tamarugo trees growing in the Atacama Desert and proposed that this moisture movement and subsequent release happened at night when the trees were not transpiring. Later Richards and Caldwell (1987) observed the phenomenon of overnight increase in water potential using thermocouple psychrometers in a natural field setting beneath sagebrush (Artemesia tridentata), which has deep tap roots. They coined the term “hydraulic lift,” [later termed “hydraulic redistribution (HR)” by Burgess et al. (1998)] to describe the process.
The stable and radioactive isotopes of hydrogen are extremely useful tools for tracking water movement in ecological studies (Rundel et al., 1989). Studies using natural abundance hydrogen isotopes (Dawson and Ehleringer, 1993), as well as deuterium (Caldwell and Richards, 1989), and tritium (Corak et al., 1987) enriched water, have been used to trace the path of HR water from deeper to shallower soils. HR has been identified across a variety of ecosystem types (Emerman and Dawson, 1996; Warren et al., 2007; Bleby et al., 2010). Hydrogen isotopes have been important tools in observing HR water transfer in natural environments between Acer saccharum trees and multiple nearby species, where the proportion of A. saccharum HR water used correlated linearly to the growth of nearby shallow-rooted species (Dawson and Ehleringer, 1993). Dawson and Pate (1996) continued this work measuring HR in several Australian ecosystems and identified how the dimorphic (i.e., deep and shallow) habit of tree roots in seasonally dry environments can lead to seasonally distinct patterns of water uptake from different elevations within the soil profile.
The presence of HR has been linked to numerous ecosystem processes beyond simple transfer from one plant to another. For example, in greenhouse conditions under severe water stress, Querejeta et al. (2003) showed that HR water can be transferred to mycorrhizal symbionts of oak trees. Furthermore, Cardon et al. (2013) provided evidence that HR promoted nitrogen mineralization and plant nitrogen uptake by Artemesia tridentata. Although HR has been suggested to provide a potential benefit to agroecosystems (Corak et al., 1987; Liste and White, 2008), the transfer of HR water from a deep-rooted woody species to an important food crop species as a mechanism to buffer against a severe, in-season drought that would otherwise cause crop-failure has not been conclusively shown to the best of the authors' knowledge.
To address whether HR can increase crop production, we investigated the existence of this pathway with the native woody shrub, Guiera senegalensis J.F. Gmel, which often grows in the agricultural fields of farmers throughout the Sahel (Figures 1A,C). The Sahel is a transitional climate zone between the arid Sahara desert in the North and the Savannah to the South, where one-third of the 20.2 million inhabitants face food and economic insecurity (United Nations, 2014). The majority population is directly employed in agriculture, which is 97% rain-fed on low-fertility soils where external inputs are not affordable for subsistence farmers (Calzadilla et al., 2013). Furthermore, unsustainable land management such as discontinuation of fallowing, cropping intensification, overgrazing (United Nations, 2014) and scavenging for fuel wood have contributed to severe degradation and no increase in crop yield over the last 50 years (FAO, 2015). Another important agronomic challenge for the Sahel is low and unpredictable rainfall and in-season drought periods, which lead to low yield or crop failure (Figure 1B) (Terrasson et al., 2009). Therefore, water is the most important factor that farmers must use effectively to optimize crop productivity.
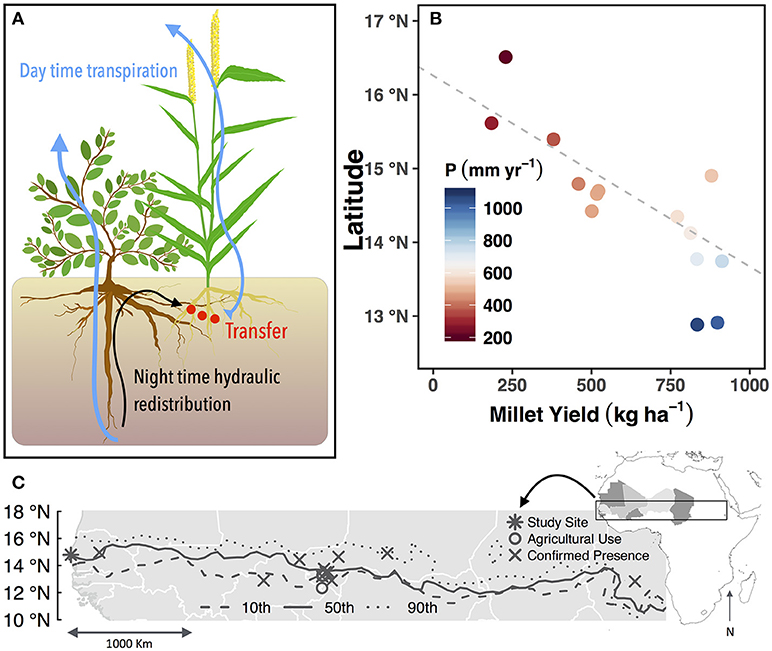
Figure 1. Conceptual figure showing Guiera senegalensis and associated Pennisetum Glaucum (pearl millet) (A). Mean annual millet yield across latitudinal transect in Senegal over the years 1987–2002. Mean annual precipitation for the same time period is indicated by color. Fitted line represents linear relationship of latitude and yield with values below 800 mm of mean annual precipitation (r2 = 0.68) (B). Map of the Sahel showing published locations of G. senegalensis and the median, and 10th and 90th percentiles of the 500 mm isohyet to illustrate the interannual variability of the precipitation (C).
Previously, our team documented benefits to soils and food production when crops are inter-planted with the native woody shrub, G. senegalensis, which is found throughout the Sahel between the 500 and 800 mm isohyets (Figure 1C) (Le Houerou, 1980). This is an optimized system where the density of shrubs is elevated (~1500 shrubs ha−1 or 3 to 4 times greater than the natural density) and coppiced shrub residues are annually returned to the soil. Studies conducted in experimental plots and farmers' fields in the past 15 years concluded that shrubs improve soil quality (Dossa et al., 2009; Diedhiou-Sall et al., 2013) via root and litter inputs that build soil structure, organic matter concentrations, nutrient availability, and water storage (Dossa et al., 2009, 2010).
G. senegalensis has tap roots that that extend beyond 3 m which enable retrieval of water from the subsoil during the dry season and drought periods of the cropping season when the upper 50 cm of soil becomes very dry (Gaze et al., 1998; Wezel and Böcker, 1999; Kizito et al., 2006). Because of this adaptation to the dry climate, G. senegalensis uses minimal amounts of water during the critical early phases of crop germination and growth and does not compete with crops for water (Kizito et al., 2007). Our previous studies (Kizito et al., 2012) also documented elevated soil moisture and diurnal soil water potential fluctuations beneath shrub canopies during drought periods as evidence G. senegalensis performs upward HR of water via roots from wet subsoil to dry surface soil (Caldwell and Richards, 1989; Neumann and Cardon, 2012) due to a soil water potential gradient.
In the coarse soils typically found in the region, water potential ranges widely from near-saturation (−0.01 MPa) to permanent wilting point (−1.50 MPa) with only a 7% change in volumetric water content (Bogie et al., 2018). Rapid drying of the surface soils through crop and shrub evapotranspiration can lead to large gradients in moisture content and water potential, creating the necessary conditions for HR. We hypothesized that HR water could buffer crops against in-season periods of water stress. Furthermore, an 11 year study on P. reticulatum (Bright et al., 2017) and a 4 year study on G. senegalensis (Dossa et al., 2012) showed that crops near shrubs had significantly higher crop yield per mm of rainfall, even in dry years, over non-shrub treatments. In our earlier work, only indirect evidence that HR water from shrubs is available to adjacent crops was documented. To accept or refute this hypothesis, an irrigated field experiment in the dry season was conducted in tandem with a deuterium tracer study during a simulated drought to determine if HR water was taken up by adjacent millet plants.
Materials and Methods
Research Site and Field Experimental Design
This research was performed in the Peanut Basin, Senegal in the village of Keur Matar Arame (14°45' N, 1620°51' W and 54 m above sea level) where the mean annual precipitation is 450 mm yr−1. The farming system is a yearly rotation of Pennisetum glaucum (pearl millet) and Arachis hypogea (groundnut) crops. Daily mean temperatures at the site range from 20° C in December-January to over 33° C from April-June. The soil is a Rubic Arenosol as described in the FAO system, locally described as Dior. The soil has minimal structure except for a periodic crust layer at a depth of approximately 5 cm that disappears once the soil is wet. This crust layer was not reported in previous studies at the site (Badiane et al., 2000; Kizito, 2006; Dossa et al., 2009). There is little horizonation in the top 1 m of soil. The texture of the soil is over 95% sand.
The experimental site was established in 2003. Prior to 2003, for at least 50 years, the area was in a crop rotation between groundnuts and pearl millet under local farmer management with G. senegalensis as the dominant shrub found in farmers' fields with a density of 240 shrubs ha−1. The experiment is a randomized complete block split plot design with shrubs as the main plots and fertilizer rate as the subplot (0, 0.5, 1.0, or 1.5 x the recommended nitrogen-phosphorus-potassium fertilizer rate for millet or groundnut) (see Dossa et al., 2012, for details of the long-term study). For this study the zero fertilizer plots were used.
In 2003 the crop only subplots had shrubs removed manually, which included digging out major roots, and over the course of the experiment received only crop residues as biomass input. The crop+shrub treatment was managed optimally by planting additional shrubs in 2003 to increase shrub density to 1500–1833 shrubs ha−1 followed by annual incorporation of coppiced shrub biomass in May, for the purposes of this dry-season study, additional cuttings and incorporations of biomass occurred on February 27 and April 15, 2014. In contrast to the typical farmer practice of burning coppiced shrub biomass before using a shallow (6 cm) sweep-tillage implement to till the soil prior to planting (traditional, thermal management), the shrub material was chopped (~5 cm lengths) and spread evenly across the crop+shrub subplots after which the same horse drawn sweep tillage implement mentioned above was used to incorporate the shrub stem and leaf material to a depth of about 6 cm (non-thermal management). A study by Dossa et al. (2012) showed improved yield of millet near shrubs with this higher shrub density and non-thermal shrub residue management compared to millet planted on bare soil. A different study by Kizito et al. (2006) showed an insignificant yield increase of millet planted near shrubs at their native density with thermal management compared to millet planted on bare soil. Therefore, the difference between traditional thermal management of shrub residues and this optimized non-thermal management practice may result in higher crop yields compared to a bare soil control where crops are planted alone.
Since 2003 and for the study reported here, (March to June 2014) crop and soil management followed typical farmer practices using animal traction and manual labor. Drip irrigation was used to impose a strong drought stress at early flowering when the crops were highly vulnerable. All shrubs used for the tracer study were the oldest shrubs within the crop+shrub subplots with extensive root systems, present before the addition of new shrubs in 2003.
Plot Layout and Irrigation
In this region, the major field crops of pearl millet and groundnut are entirely rain-fed. To enable full control for inducing drought, the experiment was performed during the dry season. Subplots measuring 10 x 6 m normally cultivated at the site were split to 5 m x 6 m and had drip irrigation installed. Drip tubes were positioned on the surface with 1 m between tubes and along these tubes drip emitters were inserted every 0.5 m. The plots were then sown with pearl millet (Pennisetum glaucum var. Souna III) at a 1 m x 1 m spacing on March 7, 2014. Crops were irrigated beginning on March 5 initially with 24 mm of water and then with 3–30 mm of water every 1–2 days with groundwater from a local water system. For 2 weeks in the early part of the season, plots were irrigated with a sprayer by hand with the amount of water delivered to each plot quantified by a volume counter on the hose, as well as checks in each plot using five buckets to measure water quantity. This was done to avoid excessive surface temperatures since there were no clouds and the climate stayed dry and hot. On days when water was not available, irrigation had to be increased at the following event. Biomass yields obtained in this study were higher than those obtained during the rainy season, particularly for the crop only subplots, though the yields vary widely from season to season (Dossa et al., 2012). As a result of drip irrigation applications that were smaller than the typical large precipitation events during the rain-fed growing season the soil profile did not undergo the large wetting and infiltration events that lead to deep percolation below the rooting zone as seen during the growing season (Kizito et al., 2007; Bogie, 2016). Drip irrigation could have led to higher water use efficiency (g dry matter produced per mm water applied) in this trial than under rain-fed conditions. Therefore under water limited conditions such as this drought treatment, drip irrigation could yield higher biomass and grain production for the same quantity of water entering the soil surface. On May 9, irrigation was cut off during the early flowering stage of the millet and no further water was applied to the crop for the 41 days remaining until harvest. The isotope tracer study occurred from May 24 - May 29.
Millet was harvested on June 19, 2014, six plants per treatment in each of three replicate plots (n = 3 per treatment) were harvested from a 6 m2 area and the total per subplot was used to scale the mass of the six plants to per hectare yield. Panicle yield was used instead of grain yield because many plants were damaged by birds that invaded protective cloth shields placed over panicles and subsequently consumed many of the seeds. Panicles may have been slightly damaged, but the authors observed that the damage was uniform across panicles that had developed through the grain-filling growth stage.
Stable Isotope Tracer Investigation
In order to deliver labeled 2H-water to the deep roots of the shrub, glass vials with rubber septa (4 cm diameter) and screw caps were drilled with holes from 2 to 5 mm in order for them to stretch and create a tight seal around roots that ranged in diameter from 3 to 9 mm. In three separate crop+shrub subplots a hole was dug to ~1 meter and two roots were chosen from one shrub for each subplot (Figure S1). The shrubs were the only plants except for the millet within the study site and had large woody roots compared to the softer roots of the millet that were found above 60 cm depth. Previously sealed vials with ~70 mL of 46 atom % 2H2O (Sigma Aldrich, St. Louis, MO) were attached to two roots cut below 1 m depth for each of three shrubs. Bottles were partially buried under ~20 cm of soil when the tracer study was initiated
Background δ2H millet signals (δ2H-free crop) were determined from millet plants grown in shrub-free plots separated by more than 30 m from the nearest tracer source. Shrub control samples (δ2H-free shrub) were collected from a shrub 100 m away from the nearest tracer source. Tissue samples for δ2H analysis were not collected in the crop only subplots that were used for moisture and millet physiology and phenology measurements (Figure S1). Tracer δ2H exceeding two standard deviations above the control mean were considered as a statistically significant signature of the applied tracer.
Crops and shrubs were sampled separately in the following order at each time interval of during the tracer experiment: (1) shrubs chosen 100 m (δ2H-free shrub) away from the site were sampled, then (2) crops 30 m (δ2H-free crop) from the nearest tracer application location were sampled as a control, (3) then the crops in the sample plots were sampled and followed by, (4) labeled shrub sampling. On May 24 the deuterium label was applied to the shrub roots. On the evening of May 27, the roots attached to the vials were completely severed above the bottle, and both cut ends covered in electrical putty to stop the flow of tracer. After the roots were severed and sealed the bottles were left buried in place in order to minimize disturbance of the soil and reduce the possibility of contamination of the soil with labeled water. From May 24 to 28 sampling of shrub and millet biomass at the above plot locations was done at 8-24 h intervals with shorter intervals in the first 3 days of the tracer study. The bottles were excavated then removed from the holes on May 29 after the last sampling was complete. All of the bottles except one of the two in crop+shrub subplot B (which lost 2.8 g) lost between 33 and 65 g of the tracer water as a result of root uptake.
Extreme caution was taken to avoid contamination, baseline crop and shrub samples were collected in the field before the deuterium solution was ever removed from the lab, and a separate pair of rubber gloves was used to sample each replicate of each type of plant. Shrub biomass was sampled by cutting four stems at the soil surface which then had bark removed. The stripped stems were placed in a glass vial with a polycone cap, sealed, and wrapped with parafilm. The sampled shoots of the shrub were all new growth since the beginning of the study, and were approximately 40 cm long. For the crop sampling, tillers were cut within 5 cm of the soil surface, the first 2 layers of material were removed. The remaining internal shoot material was placed and sealed in glass vials as described for shrub material above. Immediately after sealing, the glass vials were placed in polyethelene bags separated by replicate and treatment and transported in a cooler on ice to a −20° C freezer within 2 h and remained frozen until extraction of the water.
Samples were extracted by cryogenic vacuum distillation as described by West et al. (2006) with the modifications noted below. Samples were removed from the freezer 12 h before extraction to thaw and equilibrate. Most of the extractions were carried out at IRD Dakar and the remaining samples were shipped back to Merced, CA, USA on dry ice to keep them frozen before they were extracted. Once samples were thawed, vials were opened and samples placed into extraction vials with decontaminated dry tweezers and sealed immediately in the previously evacuated vacuum extraction apparatus. Once sealed on the vacuum line, samples were frozen in liquid nitrogen and then drawn down to 30 mTorr using a rotary vane vacuum pump (Max 8C, Fisher Scientific, Pittsburgh, PA). The sample was then isolated from the manifold and monitored for leaks. If no leaks occurred, then the nitrogen was replaced with boiling water over the extraction tube and maintained at boiling throughout the extraction. The nitrogen was then placed on the collection tube to catch the distillate. After 70 min the glass lines were inspected for any condensation and glass and metal parts were heated with a heat gun to make sure no condensation remained inside the lines. Once it was established that there was no condensation in the system, the frozen collection tube was removed from the manifold, sealed with parafilm to thaw, pipetted into 2 mL storage vials with a screwcap and PTFE septa. These sealed vials were wrapped with parafilm and brought back to the Fogel Lab at UC Merced for analysis.
Isotope Analysis
Samples were analyzed for 2H on a Thermo Scientific Temperature Conversion Elemental Analyzer (TCEA) coupled to a Conflo-IV and an isotope ratio mass spectrometer (IRMS) (Delta V- Plus, Thermo Scientific) in continuous flow mode. Isotope ratios are represented using the δ2H notation (Eq. 1) where Rsample is the ratio of 2H/H of the sample, and Rstandard is the ratio of 2H /H of the Vienna Standard Mean Ocean Water (VSMOW) standard.
One μL aliquots were injected manually into the TCEA with a 10 μL syringe. The TCEA furnace was maintained at 1430° C with a constant stream of helium carrier gas. The TCEA reactor column consisted of a graphite crucible housed in a glassy carbon rod where H2O was converted to H2 and CO. Hydrogen and carbon monoxide were separated on a molecular sieve column before entering the Conflo. Samples were run in triplicate with H2 gas standard injected twice before and twice after the sample injections with a mean standard deviation of 1.5‰ among peaks for a given sample. In order to correct for H that is produced during ionization, the H factor was measured with a typical value of 4.71 ppm nV−1. This is introduced because H has the same mass/charge (m/z) value as H2H+ and is read by the Faraday detector for m/z = 3 (Sessions et al., 2001). The voltage signal from the IRMS was processed using ISODAT and calibration regressions were performed using R (R Core Team, 2016). For a sample reading δ2H 23‰ the 95% confidence interval was ±1.4‰. A series of Los Gatos Research working standards were used across the range of −150±1.0 to −40 ±1.0 ‰, as well as an enriched internal standard of 1000 ‰, VSMOW standard, UC Merced distilled water, and SMOW-GL, an internal water standard with a δ2H close to VSMOW.
Soil Moisture and Water Potential
Soil water potential was measured using screen-cage thermocouple psychrometers individually calibrated isothermally in a range of KCl solutions (Brown and Bartos, 1982). Psychrometers used a cooling time of 30 s and were logged hourly with a data logger (PST-55/PSYPRO, Wescor, Logan, UT). The only functional psychrometers were located in the crop+shrub subplot of plot C at depths of 20, 60, and 100 cm. The fluctuations in water potential are synchronized and there is no time lag of the daily maximum and minimum water potential with depth (Figure 2). This is strong evidence that these fluctuations are not caused by diurnal temperature changes, which typically exhibit a depth-wise phase shift in a sinusoidal plot with time series on the x and temperature on the y-axis. Water potential is highly sensitive to changes in temperature (Andraski and Scanlon, 2002) in the range observed at this field site. Therefore, if diurnal temperature fluctuations were driving the changes to water potential that are observed here, the sinusoidal shape of the temperature vs. time plot would exhibit a phase shift with depth. Since there is no phase shift observed in the diurnal water potential fluctuations, we conclude that the changes to water potential are driven by changes to the relative humidity in the soil, which we propose is as a result of transpirational uptake and nighttime efflux of moisture from shrub roots rather than changes in soil temperature, though vapor flux can account for a fraction of water potential change in this system during HR (Kizito et al., 2012).
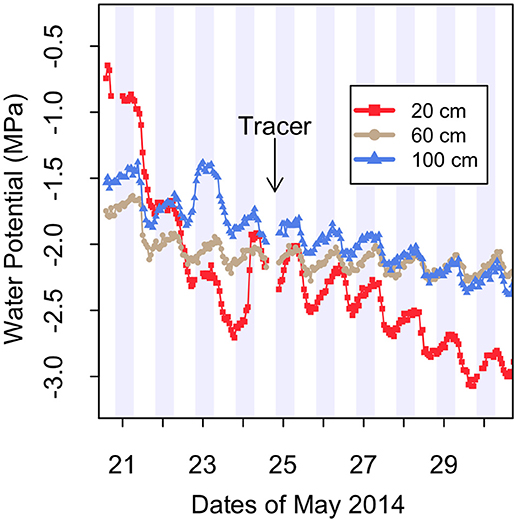
Figure 2. Soil water potential measured by thermocouple psychrometers at three depths in crop+shrub subplot C. “Tracer” indicates the beginning of the stable isotope 2H-tracer study on the evening of May 24. Gray shading represents night time hours. These data represent conditions beginning on day 13 of the imposed drought.
Soil moisture content was measured with a multi-depth profile capacitance probe in access tubes that were installed according to the manufacturer's recommendations with great care taken to avoid air gaps along the tube (PR 2/6, Delta-T Devices, Cambridge, UK). Soil water measurements were taken at three locations: (1) < 0.5 m from canopy in the crop+shrub subplots (crop+shrub “near”); (2) 1 m from the nearest shrub canopy in the crop+shrub subplots (crop+shrub “far”); and (3) >3 m from the nearest shrubs in the crop only subplots (Figure S1). The profile probe measured moisture at depths of 10, 20, 40, 60, and 100 cm. The factory calibration of profile probe was used with an accuracy of ± 0.04 m3m−3. Soil moisture measurements were taken just prior to turning on irrigation every 1–3 days. On May 25 the soil moisture sensor failed, so there are no moisture measurements following this date. Trapezoidal integration was used to calculate the height of water (mm) in the 10–100 cm depth range.
Statistics
Statistical analysis for biomass and panicle production were performed in R (R Core Team, 2016). Two-sided t-tests were used to calculate yield difference on the sum of total millet biomass or panicle masses within each crop+shrub or crop only subplot (n = 3). Statistically significant results were considered at P-values below 0.05. For plant height a non-parametric Kruskal-Wallis test was used to compare between three distances from the shrub on each separate date and a post-hoc Dunn's Test with a Bonferroni correction of the p-value threshold was used (Dinno, 2015).
Regional Precipitation, Yield, and Shrub Locations
The GPCC precipitation and the yield-precipitation data used in Figures 1B,C are adapted from (Mahul et al., 2009) and GPCC precipitation can be found at: http://www.cgd.ucar.edu/cas/catalog/surface/precip/gpcc.html. The reference for GPCC data is specified as (Schneider et al., 2014). The locations of G. senegalensis in Figure 1C are from the following publications, Ringrose and Matheson (1992), Geiger and Manu (1993), Gijsbers et al. (1994), Levy and Jarvis (1998), Wezel et al. (2000), Seghieri et al. (2005), Yelemou et al. (2007), Lufafa et al. (2009), Brandt et al. (2017). Note in some cases where there were multiple sites with G. senegalensis reported within a small region, an approximate location central to the field locations was used.
Results and Discussion
Millet was grown with fully sufficient levels of water for plant growth until early flowering and then water was withheld for 41 days until harvest. Soil water potential rapidly declined during the drought period with soil at the 20 cm depth reaching −2.0 MPa after 12 days, which was drier than the 60 cm and 100 cm depths (Figure 2).
Soil moisture measured at three distances from the closest shrub remained stable until mid-May, when the near-shrub moisture began a steep decline of ~10 mm over the course of a week (Figure S2). Profile soil moisture declined continuously in all subplots after irrigation was stopped on May 9. Declines in the crop+shrub “far” moisture and crop only treatments were less pronounced than the crop+shrub “near” treatments in crop+shrub subplots A and B, in crop+shrub subplot C the declines crop+shrub “far” and “near” were similar (Figure S2). In an earlier study, Kizito et al. (2012) found elevated near-surface moisture in crop+shrub subplots during the early part of the season as compared to crop only treatments. They also found there was movement of moisture upwards toward the shrub and laterally away from the shrub at night indicating G. senegalensis was performing HR during times of extreme drying in the surface soil.
There was little observable difference in moisture content in the upper 1 m of the soil profile between treatments in the early season which does not resemble the pattern observed by Kizito et al. (2007). These authors showed that in the early part of the growing season, crop+shrub treatments had an elevated moisture content of up to 0.03 m3 m−3 in the upper 1.10 m of the profile, integrated over neutron probe measurements at 0.10 m depth increments. The differences Kizito et al. observed may be due to differences in infiltration rates between plots or due to the existence of hydraulic redistribution in the shrub root zone (Kizito et al., 2007). Kizito et al. found higher saturated infiltration rates near the shrub which they attribute to higher macroporosity. Saturated infiltration would then lead to deeper moisture penetration and higher total profile moisture content beneath the shrubs following a large rainfall event. However, the rate of water infiltration by the drip emitters in this study was low enough that there was no ponded infiltration, therefore the role of macropores in infiltration was likely diminished compared to many of the precipitation events during the rain-fed growing season. Smaller, more frequent low-intensity events (such as drip irrigation) could lead to similar infiltration rates across treatments in this study and a more uniform profile moisture depth when compared between treatments. Additionally, the shrub density in this irrigation and tracer study was ~7 times as high as the native density in the field studied by Kizito et al. (2007), so the shrub water uptake was likely higher in this study which could diminish any shrub-mediated increases to profile moisture content due to infiltration or hydraulic redistribution.
Not surprisingly because of the very low soil moisture levels during the drought, millet without shrubs resulted in crop failure with virtually no panicle production and very low biomass (Figure 3 and Figure S3). Although millet is one of the staple crops in the region, biomass yields in fields without the presence of shrubs or additional fertilizer can routinely fall below 200 kg ha−1. Sometimes crops produce no grain at all as they did in 2007 (Dossa et al., 2012) and 2013 at this site (M. Bright, personal communication).
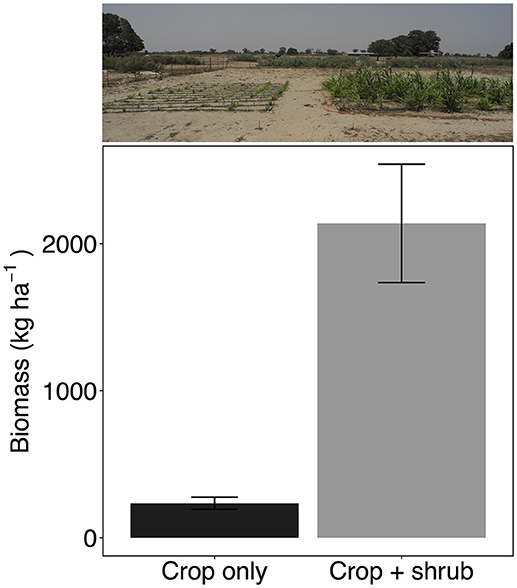
Figure 3. Biomass yield of millet (Pennisetum glaucum) crop grown alone or in association with Guiera senegalensis (n = 3 per treatment). Error bars are one standard error, treatments are significantly different (P < 0.05). Photo is taken in plot C.
However, it was surprising to find that millet in the presence of shrubs, despite experiencing soil water potential well below the wilting point at −3.0 MPa, was not only able to keep growing but actually produced a harvestable product. The millet in crop+shrub subplots produced almost 10 times the biomass and over seven times more panicles than the crop only treatment (Student's t-statistic 15.8, −4.7 for panicle mass and biomass, respectively, P < 0.05 for both) (Figure 3 and Figure S3). These results are consistent with Dossa et al. (2012) who found millet planted near G. senegalensis maintained significantly higher crop yields during low rainfall years.
This raises a fundamental question on how shrubs keep a crop growing when soil moisture is so low. Somehow this shrub is making water available to the crop and apparently very efficiently.
One mechanism for assisting millet could be the higher soil organic matter that has accumulated in the optimized shrub-intercropping system (Dossa et al., 2009, 2010, 2012; Diedhiou-Sall et al., 2013). In particular, Dossa et al. (2012) found that the particulate organic matter fraction (undecomposed plant litter) was significantly higher when soils were under an optimized system which was highly correlated with crop yields. The improved soil quality due to annual incorporation of coppiced shrub biomass and root turnover for 9 years prior to our current study would be expected to increase aggregation and porosity to store water (Carminati, 2012) and make it more readily available for crops. However, the extremely low water potential after the drought was imposed (well below the wilting point), would suggest that improved soil quality and water holding capacity cannot fully account for the positive growth response of millet in the presence of G. senegalensis.
This leaves the second, and reasonably, the most important mechanism, HR, as a plausible explanation for the phenomenal ability of G. senegalensis to keep millet growing through the severe in-season drought that was imposed. Indeed, all sensors recorded the typical signature of HR with an increase of water potential from sunset until sunrise. During this imposed drought period the largest overnight increase in water potential was 0.57 MPa over a 12-h period at 20 cm depth on May 25 (Figure 2). However, these diurnal shifts in water potential do not confirm that hydraulically lifted water by this shrub is actually taken up by adjacent crops.
To address this question the 2H-tracer experiment was done toward the end of the drought period. Figure 4 shows temporal shift of 2H-tracer in shrub tissue increasing in the first 24 h and then declining; whereas millet tissue adjacent to the shrub started showing the signal from 12 h after the tracer was applied. In crop+shrub subplot A and B above-background δ2H was observed in millet with peak δ2H values at 36 and 84 h after tracer application, respectively. In crop+shrub subplot C, above background δ2H was first observed 36 h after 2H tracer application in millet, which was also the time at which it reached its maximum value.
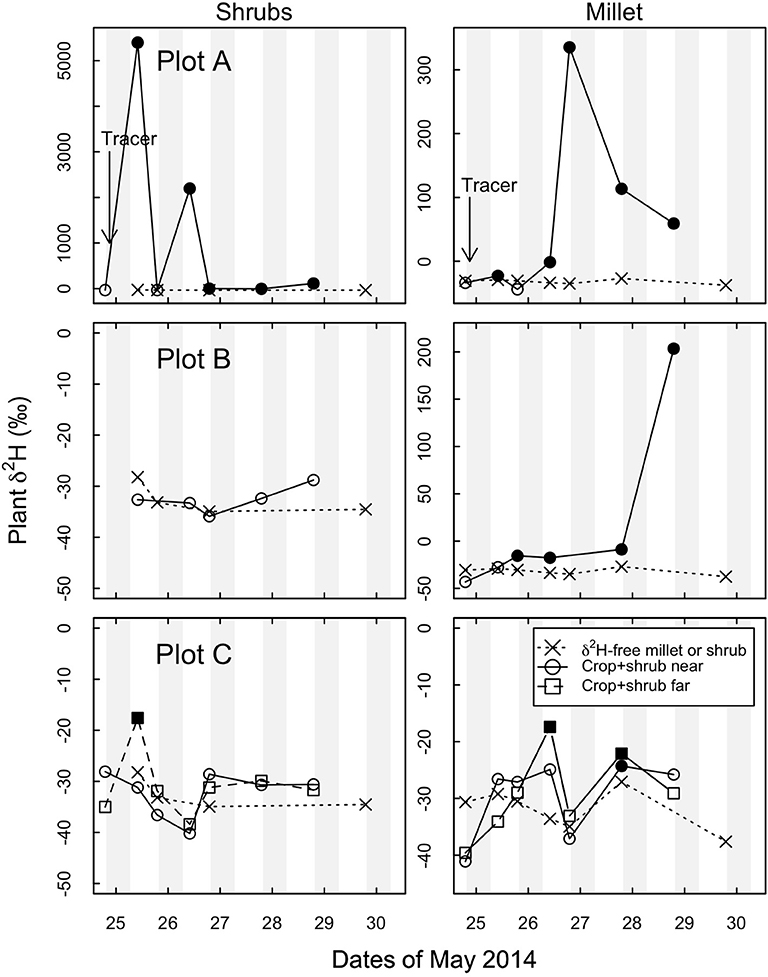
Figure 4. Time evolution in δ2H in shrub (Guiera senegalensis) and crop (Pennisetum glaucum) plant water in each of three crop+shrub subplots and in a separate δ2H-free crop and δ2H-free shrub located at least 30 m from nearest enriched tracer site. “Tracer” indicates the beginning of the stable isotope 2H-tracer study on the evening of May 24. Crop+shrub subplot (A,B,C) “near” were taken < 0.5 m from δ2H labeled shrub. Crop+shrub subplot C “far” was 4.5 m to the East of the δ2H labeled shrub. Shaded portions indicate night time hours. Note different y-axis scales. Open symbols represent background δ2H signal, and filled symbols represent above background δ2H signal, “x” symbols represent data from δ2H-free control millet plot and shrub plot.
The strongest 2H signal in millet was in crop+shrub subplots A and B, which exhibited above-background δ2H at all but one sampling time after the introduction of the tracer on the evening of May 24 (Figure 4). In subplot C of the crop+shrub treatment the tracer was significantly above background levels (δ2H = –18‰) once at the “near” sampling site and twice at the “far” (> 3 m from labeled shrub, but still within shrub subplot near other shrubs) sampling site. The most positive (enriched) δ2H values in the millet in crop+shrub subplots A, B, and C were 335, 208, and −17‰ respectively. The δ2H values in the shrub stems in crop+shrub subplot A showed the greatest enrichment (δ2H = ~5000‰ increase) and an above-background signal in all but one sampling after tracer introduction (Figure 4). These results confirm that G. senegalensis can move water from deep in the profile by HR to surface soil that can be taken up by nearby millet plants.
Although it was not possible to quantify the exact amount of water transferred, the crop yields in crop+shrub vs. crop only subplots indicate the amount of water transferred is important. Sap flow data measured in the crop+shrub subplot of plot C confirms that the millet plants near the shrubs were, in fact, transpiring even after 17 days without added irrigation water, and the moisture content was considerably higher in crop+shrub subplot A where sap flow was measured than in plot C where the water potential was measured (Figures S2, S4).
Despite the fact that the soil moisture nearest to the shrubs was the lowest, the plants closest to the shrubs were 267% taller than the crop only plants, and 142% taller than the millet 1 m away from the shrub (Figure S5). Surprisingly, the largest plants in the field were in the driest conditions, in the presence of shrub roots Thus one can only conclude HR water must have kept millet growing, and done so very efficiently. Additionally, the quantity of water transferred during HR is very small, but the water potential, which can vary widely with a small amount of moisture change, determines the limit to nitrification (Stark and Firestone, 1995) and plant water uptake (Richards and Weaver, 1943). Using an indirect water balance method, Kizito et al. (2012) estimated the quantity of HR to be 35–47 mm annually, which accounts for 8–10% of the mean annual precipitation of 450 mm. With the cessation of irrigation there was no available water to the millet other than that stored in the rooting zone, or HR water.
Water enriched in, 2H was not strongly detected in shrub stems in two of three crop+shrub subplots even though it was applied directly to one of the shrub's tap roots. Guiera senegalensis has an extensive vertical and horizontal rooting system and many aboveground stems (Kizito et al., 2006). It is therefore conceivable that some of the sampled shrub stems were not directly connected to the roots that received the isotopic tracer thus the absence of a 2H signal in the shrubs at some time steps is not unexpected (Figure 4 and Figure S6).
This observation does not, however, explain why all HR water was not completely reabsorbed by the shrubs themselves. One explanation is that a plant may not use its own HR water, as deep water may be easier to take up in wetter conditions of the subsoil due to potential differences in hydraulic conductivity between surface and tap roots (Mcelrone et al., 1999). Alternatively, millet may be better adapted, through its high density of roots in the 0-1 m range, to exploit HR water than a woody species such as G. senegalensis (Brück et al., 2003).
Another mechanism that could contribute to efficient transfer of HR water from shrubs to crops is mycorrhizal hyphae connecting roots of two plant species (Egerton-Warburton et al., 2007). The strong HR signal in the soil water potential (Figure 2) demonstrates the release of moisture into the shallow soil depths, but does not rule out the possibility of a mycorrhizal transfer pathway between species.
We propose three mechanisms by which a relatively small volume of water in an environment of very high potential evaporation can contribute to improved crop performance. First, from soil water potential data (Figure 2) and other similar studies (Richards and Caldwell, 1987; Caldwell and Richards, 1989) HR is particularly strong during times of extreme drought and increases the longevity of shrub roots by maintaining contact between the root and the rhizosphere soil which, if broken, can lead to cavitation and loss of root function (Horton and Hart, 1998). It has been shown that soil with root exudates (extracellular polymers) can retain a greater amount of water than bulk soil under drying conditions (Carminati and Vetterlein, 2013). This mechanism could help prevent drought-induced cavitation of shrub roots when soil water potential is near the wilting point. The same logic could be extended to include the maintenance of root health of the millet roots when they are intermingled with shrub roots. During the rainy season in nearby farmers' fields, the authors observed millet roots growing closely around G. senegalensis roots, supporting the assertion that HR water is involved in the maintenance and functioning of the roots of both species.
Second, in many arid and semi-arid regions shrubs have been shown to create resource “islands” with greater carbon and nutrient contents than in surrounding soils (Dossa et al., 2009, 2012; Hernandez et al., 2015; Diakhate et al., 2016). Improved crop yields near these “islands” are associated with higher microbial activity and abundance in the rhizosphere (Diedhiou-Sall et al., 2013; Debenport et al., 2015). While shrubs provide water during drought stress, they also create higher quality soils and harbor beneficial microbes (Debenport et al., 2015) that improve crop nutrient and health status, all of which can contribute to drought resistance. Indeed, some microbes have the ability to assist plants during water stress (Timmusk and Wagner, 1999).
Third, quantity of water used during grain filling is extremely important to grain yields (Vadez et al., 2013b). Vadez et al. (2013a) showed in a pot study that for every additional kg of moisture used by millet during grain filling, yield can be increased by 4.7 and 3.5 g plant−1. Scaling this result up, at a planting density of 10,000 plants ha−1 this could result in a yield increase of 35–47 kg ha−1 mm−1 additional moisture that plants receive during grain filling. This shows that even a relatively small quantity of moisture, such as that provided by HR from G. senegalensis to millet, can have a strong impact on crop yield.
In areas with less than 800 mm of mean annual precipitation, trees in agroforestry systems will outcompete crops for limited water resources, unless the deep rooted species can access groundwater (Van Noordwijk and Ong, 1999). Additionally, pearl millet yields along a transect in Senegal show 800 mm of annual precipitation as a threshold below which yields begin to decline (Figure 1B) In the presence of shrubs in this study, however, millet continued to grow and was more productive than millet grown alone, and the mean annual rainfall at the site is 450 mm (Kizito et al., 2006). This observation demonstrated that G. senegalensis does not compete with crops for soil water in times of water scarcity.
These results show that shrubs can assist crops through within-season drought periods. This is a particularly critical growth period because at the mid-to-late crop stage as it is no longer possible to replant. Many arid and semi-arid regions including the West African Sahel will experience an increase in drought severity and a decrease in soil moisture with climate change (Dai, 2013), HR from associated shrubs in croplands will increase in their importance for providing adequate food for the population.
Conclusion
Feeding the large and rapidly growing populations in the socioeconomically and environmentally precarious region of the Sahel is a major challenge. For the Sahel, where the majority rural populations depend on drought-tolerant pearl millet, groundnut, sorghum (Sorghum bicolor), and cowpea (Vigna unguiculata), shrub intercropping is a logical and locally available solution for increasing yields and stabilizing productivity in high and low rainfall years.
The findings provide confirmation of an unprecedented agricultural system that performs “bioirrigation” by shrubs, literally transferring hydraulically redistributed water to adjacent crops. Using soil moisture sensors and stable isotope tracing this study provided direct evidence for HR to be the key mechanism of G. senegalensis to sustain millet during extreme water stress. However, further in-depth research is needed to understand how such small amounts of hydraulically lifted water can be so efficiently transferred from shrubs to crops. Also, HR research is needed for Piliostigma reticulatum the other dominant shrub species found in farmers' fields throughout the Sahel.
These results have implications for all semi-arid regions where in-season drought is a common occurrence with increasingly erratic climate patterns owing to global climate change. For highly mechanized semi-arid agroecosystems in other parts of the world, engineered intercropped systems for rain-fed crops should be investigated as a novel approach to manage water for crops to buffer against drought. For the Sahel, optimized shrub intercropping with elevated planting density and the return of coppiced biomass to soils, offers an ecological and locally available solution to buffer against droughts and remediate degraded soils that subsistence farmers can readily utilize.
Data Availability Statement
The data in Figures 1–4 are either the output from the raw data in this manuscript will be made available in a timely manner to any qualified researcher who requests it.
Author Contributions
ID, TG, RD, and NB developed the original idea for the study. NB, TG, RD, RB, ID, MF, and MC Planned the experiment. NB and RB managed lab and field data collection. MF helped with laboratory experimental design and data collection. NB and TG performed data analysis, wrote manuscript, and managed the manuscript editing process. NB, RB, TG, RD, MF, and MC edited manuscript.
Funding
Funding was provided for this project by the US National Science Foundation Partnerships for International Research and Education grant (NSF #0968247) and University of California Merced School of Natural Sciences.
Conflict of Interest Statement
The authors declare that the research was conducted in the absence of any commercial or financial relationships that could be construed as a potential conflict of interest.
Acknowledgments
We thanks to Dame Sy for providing transportation, and Moussa Dione, Boubacar Badji, Matthew Bright, and Mame Balla Ndiaye for help with data collection and field management. Amanda Davey at The Ohio State University was extremely helpful in the logistics and planning of the experiment. Professor Jason Sexton of UC Merced, and Prof. Dr. Andrea Carminati of Georg-August Universitat, Gottingen provided valuable feedback on drafts of this manuscript. Additionally, we would like to thank Dr. Lydie Lardie of IRD Dakar and Dr. Yacine Ndour of ISRA for lab space and field support as well as Ndiaga Cissé and the rest of CERAAS Thiés for laboratory space.
Supplementary Material
The Supplementary Material for this article can be found online at: https://www.frontiersin.org/articles/10.3389/fenvs.2018.00098/full#supplementary-material
References
Andraski, B. J., and Scanlon, B. R. (2002). Thermocouple Psychrometry.Soil Science Society of America Book Series. Madison, WI: Soil Science Society of America, 609–642.
Badiane, A. N., Khouma, M., and Sene, M. (2000). Region de Diourbel, Gestion des Eaux. Crewkerne: Somerset: Drylands Research.
Bleby, T. M., Mcelrone, A. J., and Jackson, R. B. (2010). Water uptake and hydraulic redistribution across large woody root systems to 20 m depth. Plant Cell Environ. 33, 2132–2148. doi: 10.1111/j.1365-3040.2010.02212.x
Bogie, N. (2016). Hydrologic Relations Between Native Woody Shrubs and Food Crops in the Peanut Basin, Senegal. dissertation, Merced, CA: University of California, Merced.
Bogie, N. A., Bayala, R., Diedhiou, I., Dick, R. P., and Ghezzehei, T. A. (2018). Alteration of soil physical properties and processes after ten years of intercropping with native shrubs in the Sahel. Soil Tillage Res. 182, 153–163. doi: 10.1016/j.still.2018.05.010
Brandt, M., Tappan, G., Diouf, A., Beye, G., Mbow, C., and Fensholt, R. (2017). Woody vegetation die off and regeneration in response to rainfall variability in the west african sahel. Remote Sensing 9:39. doi: 10.3390/rs9010039
Breazeale, J. F. (1930). Maintenance of moisture equilibrium and nutrition of plants at and below the wilting percentage. Univ. Ariz. Tech. Bull. 29, 137–174.
Breazeale, J. F., and Crider, F. J. (1934). Plant association and survival, and the build-up of moisture in semi-arid soils. Univ. Ariz. Tech. Bull. 53, 95–123.
Bright, M. B. H., Diedhiou, I., Bayala, R., Assigbetse, K., Chapuis-lardy, L., Ndour, Y., et al. (2017). Long-term piliostigma reticulatum intercropping in the sahel : crop productivity, carbon sequestration, nutrient cycling, and soil quality. Agricult. Ecosys. Environ. 242, 9–22. doi: 10.1016/j.agee.2017.03.007
Brown, R. W., and Bartos, D. L. (1982). A calibration model for screen-caged peltier thermocouple psychrometers. NASA STI/Recon Tech. Rep. 83:16735. doi: 10.2737/INT-RP-293
Brück, H., Piro, B., Sattelmacher, B., and Payne, W. A. (2003). Spatial distribution of roots of pearl millet on sandy soils of Niger. Plant Soil 256, 149–159. doi: 10.1023/A:1026246728095
Burgess, S. S., Adams, M. A., Turner, N. C., and Ong, C. K. (1998). The redistribution of soil water by tree root systems. Oecologia 115, 306–311. doi: 10.1007/s004420050521
Caldwell, M. M., and Richards, J. H. (1989). Hydraulic lift - water efflux from upper roots improves effectiveness of water-uptake by deep roots. Oecologia 79, 1–5. doi: 10.1007/BF00378231
Calzadilla, A., Zhu, T., Rehdanz, K., Tol, R. S. J., and Ringler, C. (2013). Economy-wide impacts of climate change on agriculture in Sub-Saharan Africa. Ecol. Econom. 93, 150–165. doi: 10.1016/j.ecolecon.2013.05.006
Cardon, Z. G., Stark, J. M., Herron, P. M., and Rasmussen, J. A. (2013). Sagebrush carrying out hydraulic lift enhances surface soil nitrogen cycling and nitrogen uptake into inflorescences. Proc. Natl. Acad. Sci. U.S.A. 110, 18988–18993. doi: 10.1073/pnas.1311314110
Carminati, A. (2012). A model of root water uptake coupled with rhizosphere dynamics. Vadose Z. J. 11. doi: 10.2136/vzj2011.0106
Carminati, A., and Vetterlein, D. (2013). Plasticity of rhizosphere hydraulic properties as a key for efficient utilization of scarce resources. Ann. Bot. 112, 277–290. doi: 10.1093/aob/mcs262
Corak, S. J., Blevins, D. G., and Pallardy, S. G. (1987). Water transfer in an Alfalfa/Maize association survival of maize during drought. Plant Physiol. 84, 582–586. doi: 10.1104/pp.84.3.582
Dai, A. G. (2013). Increasing drought under global warming in observations and models. Nat. Clim. Chang. 3, 52–58. doi: 10.1038/nclimate1633
Dawson, T. E., and Ehleringer, J. R. (1993). Isotopic enrichment of water in the woody tissues of plants: implications for plant water source, water uptake, and other studies which use the stable isotopic composition of cellulose. Geochim. Cosmochim. Acta 57, 3487–3492. doi: 10.1016/0016-7037(93)90554-A
Dawson, T. E., and Pate, J. S. (1996). Seasonal water uptake and movement in root systems of Australian phraeatophytic plants of dimorphic root morphology: a stable isotope investigation. Oecologia 107, 13–20. doi: 10.1007/BF00582230
Debenport, S. J., Assigbetse, K., Bayala, R., Chapuis-Lardy, L., Dick, R. P., and McSpadden Gardener, B. B. (2015). Shifting populations in the root-zone microbiome of millet associated with enhanced crop productivity in the Sahel. Appl. Environ. Microbiol. 81, 2841–2851. doi: 10.1128/AEM.04122-14
Diakhate, S., Gueye, M., Chevallier, T., Diallo, N. H., Assigbetse, K., Abadie, J., et al. (2016). Soil microbial functional capacity and diversity in a millet-shrub intercropping system of semi-arid Senegal. J. Arid Environ. 129, 71–79. doi: 10.1016/j.jaridenv.2016.01.010
Diedhiou-Sall, S., Dossa, E. L., Diedhiou, I., Badiane, A. N., Assigbetse, K. B., Samba, S. A. N., et al. (2013). Microbiology and macrofaunal activity in soil beneath shrub canopies during residue decomposition in agroecosystems of the Sahel. Soil Sci. Soc. Am. J. 77, 501–511. doi: 10.2136/sssaj2012.0284
Dinno, A. (2015). R Software Package “dunn.test.” Available online at: https://cran.r-project.org/web/packages/dunn.test/index.html. (Accessed 20 February, 2018).
Dossa, E. L., Diedhiou, I., Khouma, M., Sene, M., Lufafa, A., Kizito, F., et al. (2012). Crop productivity and nutrient dynamics in a shrub-based farming system of the Sahel. Agron. J. 104, 1255–1264. doi: 10.2134/agronj2011.0399
Dossa, E. L., Diedhiou, S., Compton, J. E., Assigbetse, K. B., and Dick, R. P. (2010). Spatial patterns of P fractions and chemical properties in soils of two native shrub communities in Senegal. Plant Soil 327, 185–198. doi: 10.1007/s11104-009-0044-8
Dossa, E. L., Khouma, M., Diedhiou, I., Sene, M., Kizito, F., Badiane, A. N., et al. (2009). Carbon, nitrogen and phosphorus mineralization potential of semiarid Sahelian soils amended with native shrub residues. Geoderma 148, 251–260. doi: 10.1016/j.geoderma.2008.10.009
Egerton-Warburton, L. M., Querejeta, J. I., and Allen, M. F. (2007). Common mycorrhizal networks provide a potential pathway for the transfer of hydraulically lifted water between plants. J. Exp. Bot. 58, 1473–1483. doi: 10.1093/jxb/erm009
Emerman, S. H., and Dawson, T. E. (1996). Hydraulic lift and its influence on the water content of the rhizosphere: An example from sugar maple, Acer saccharum. Oecologia 108, 273–278. doi: 10.1007/BF00334651
Gaze, S. R., Brouwer, J., Simmonds, L. P., and Bromley, J. (1998). Dry season water use patterns under Guiera senegalensis L. shrubs in a tropical savanna. J. Arid Environ. 40, 53–67. doi: 10.1006/jare.1998.0435
Geiger, S. C., and Manu, A. (1993). Soil surface characteristics and variability in the growth of millet in the plateau and valley region of Western Niger. Agric. Ecosys. Environ. 45, 203–211. doi: 10.1016/0167-8809(93)90071-V
Gijsbers, H. J. M., Kessler, J. J., and Knevel, M. K. (1994). Dynamics and natural regeneration of woody species in farmed parklands in the Sahel region (Province of Passore, Burkina Faso). For. Ecol. Manage. 64, 1–12. doi: 10.1016/0378-1127(94)90122-8
Hernandez, R. R., Debenport, S. J., Leewis, M. C. C. E., Ndoye, F., Nkenmogne, K. I. E., Soumare, A. (2015). The native shrub, Piliostigma reticulatum, as an ecological “resource island” for mango trees in the Sahel. Agric. Ecosys. Environ. 204, 51–61. doi: 10.1016/j.agee.2015.02.009
Horton, J. L., and Hart, S. C. (1998). Hydraulic lift: a potentially important ecosystem process. Trends Ecol. Evol. 13, 232–235. doi: 10.1016/S0169-5347(98)01328-7
Kizito, F. (2006). Hydrological Consequences of Two Native Shrubs in Semi-arid Senegal: Patterns, Processes, Concepts and Methods. Corvalis, OR: Oregon State University.
Kizito, F., Dragila, M., Sène, M., Lufafa, A., Diedhiou, I., Dick, R. P., et al. (2006). Seasonal soil water variation and root patterns between two semi-arid shrubs co-existing with Pearl millet in Senegal, West Africa. J. Arid Environ. 67, 436–455. doi: 10.1016/j.jaridenv.2006.02.021
Kizito, F., Dragila, M. I., Senè, M., Brooks, J. R., Meinzer, F. C., Diedhiou, I., et al. (2012). Hydraulic redistribution by two semi-arid shrub species: implications for Sahelian agro-ecosystems. J. Arid Environ. 83, 69–77. doi: 10.1016/j.jaridenv.2012.03.010
Kizito, F., Sène, M., Dragila, M. I., Lufafa, A., Diedhiou, I., Dossa, E., et al. (2007). Soil water balance of annual crop–native shrub systems in Senegal's Peanut Basin: the missing link. Agric. Water Manage. 90, 137–148. doi: 10.1016/j.agwat.2007.02.015
Le Houerou, H.-N. (1980). “Browse in Africa : the current state of knowledge ; papers presented at the Internat,” in Symposium on Browse in Africa, Addis Ababa, April 8-12, 1980, and other submissions. Addis Ababa: Internat Livestock Centre for Africa.
Levy, P. E., and Jarvis, P. G. (1998). Stem CO2 fluxes in two Sahelian shrub species (Guiera senegalensis and Combretum micranthum). Funct. Ecol. 12, 107–116. doi: 10.1046/j.1365-2435.1998.00156.x
Liste, H. H., and White, J. C. (2008). Plant hydraulic lift of soil water - Implications for crop production and land restoration. Plant Soil 313, 1–17. doi: 10.1007/s11104-008-9696-z
Lufafa, A., Diédhiou, I., Ndiaye, N. A. S., Séné, M., Kizito, F., Dick, R. P., et al. (2009). Allometric relationships and peak-season community biomass stocks of native shrubs in Senegal's Peanut Basin. J. Arid Environ. 73, 260–266. doi: 10.1016/j.jaridenv.2008.09.020
Mahul, O., Toure, A., Dick, W., and Muller, B. (2009). Index-based Crop Insurance in Senegal Promoting Access to Agricultural Insurance for Small Farmers. Washington, DC: The World Bank.
Mcelrone, A. J., Pockman, W. T., Martinez-vilalta, J., and Jackson, R. B. (1999). Variation in xylem structure and function in stems and roots. N. Phytol. 163, 507–517. doi: 10.1111/j.1469-8137.2004.01127.x
Mooney, H. A., Gulmon, S. L., Rundel, P. W., and Ehleringer, J. (1980). Further observations on the water relations ofProsopis tamarugo of the northern Atacama desert. Oecologia 180, 177–180.
Neumann, R. B., and Cardon, Z. G. (2012). The magnitude of hydraulic redistribution by plant roots: a review and synthesis of empirical and modeling studies. N Phytol. 194, 337–352. doi: 10.1111/j.1469-8137.2012.04088.x
Querejeta, J. I., Egerton-Warburton, L. M., and Allen, M. F. (2003). Direct nocturnal water transfer from oaks to their mycorrhizal symbionts during severe soil drying. Oecologia 134, 55–65. doi: 10.1007/s00442-002-1078-2
R Core Team (2016). A Language and Environment for Statistical Computing. Vienna: R Foundation for Statistical Computing.
Richards, J. H., and Caldwell, M. M. (1987). Hydraulic lift - substantial nocturnal water transport between soil layers by artemisia-tridentata roots. Oecologia 73, 486–489.
Richards, L. A., and Weaver, L. R. (1943). Fifteen-atmosphere percentage as related to the permanent wilting percentage. Soil Sci. 56, 331–340.
Ringrose, S., and Matheson, W. (1992). The use of landsat MSS imagery to determine the aerial extent of woody vegetation cover change in the west-central Sahel. Glob. Ecol. Biogeogr. Lett. 2, 16–25. doi: 10.2307/2997327
Rundel, P. W., Ehleringer, J. R., and Nagy, K. A. (Eds.) (1989). Stable Isotopes in Ecological Research. New York, NY: Springer-Verlag.
Schneider, U., Becker, A., Finger, P., Meyer-Christoffer, A., Ziese, M., and Rudolf, B. (2014). GPCC's new land surface precipitation climatology based on quality-controlled in situ data and its role in quantifying the global water cycle. Theoret. Appl. Climatol. 115, 15–40. doi: 10.1007/s00704-013-0860-x
Seghieri, J., Simier, M., Mahamane, A., Hiernaux, P., and Rambal, S. (2005). Adaptative above-ground biomass, stand density and leaf water potential to droughts and clearing in Guiera senegalensis, a dominant shrub in Sahelian fallows (Niger). J. Trop. Ecol. 21, 203–213. doi: 10.1017/S0266467404002135
Sessions, A. L., Burgoyne, T. W., and Hayes, J. M. (2001). Correction of H3+ contributions in hydrogen isotope ratio monitoring mass spectrometry. Anal. Chem. 73, 192–199. doi: 10.1021/AC000489E
Stark, J. M., and Firestone, M. K. (1995). Mechanisms for soil-moisture effects on activity of nitrifying bacteria. Appl. Environ. Microbiol. 61, 218–221.
Terrasson, I., Fisher, M. J., Andah, W., and Lemoalle, J. (2009). Yields and water productivity of rain-fed grain crops in the Volta Basin, West Africa. Water Intl. 34, 104–118. doi: 10.1080/02508060802666336
Timmusk, S., and Wagner, E. G. (1999). The plant-growth-promoting rhizobacterium Paenibacillus polymyxa induces changes in Arabidopsis thaliana gene expression: a possible connection between biotic and abiotic stress responses. Molecul. Plant-Microbe Interact 12, 951–959.
Vadez, V., Kholová, J., Yadav, R. S., and Hash, C. T. (2013a). Small temporal differences in water uptake among varieties of pearl millet (Pennisetum glaucum (L.) R. Br.) are critical for grain yield under terminal drought. Plant Soil 371, 447–462. doi: 10.1007/s11104-013-1706-0
Vadez, V., Kholova, J., Zaman-Allah, M., and Belko, N. (2013b). Water: the most important “molecular” component of water stress tolerance research. Funct. Plant Biol. 40, 1310–1322. doi: 10.1071/FP13149
Van Noordwijk, M., and Ong, C. K. (1999). Can the ecosystem mimic hypotheses be applied to farms in African savannahs? Agroforestry Sys. 45, 131–158. doi: 10.1023/A:1006245605705
Warren, J. M., Meinzer, F. C., Brooks, J. R., Domec, J.-C., and Coulombe, R. (2007). Hydraulic redistribution of soil water in two old-growth coniferous forests: quantifying patterns and controls. New Phytol. 173, 753–765. doi: 10.1111/j.1469-8137.2006.01963.x
West, A. G., Patrickson, S. J., and Ehleringer, J. R. (2006). Water extraction times for plant and soil materials used in stable isotope analysis. Rapid Commun. Mass Spectrom. : RCM 20, 1317–1321. doi: 10.1002/rcm.2456
Wezel, A., and Böcker, R. (1999). Mulching with branches of an indigenous shrub (Guiera senegalensis) and yield of millet in semi-arid Niger. Soil Tillage Res. 50, 341–344. doi: 10.1016/S0167-1987(99)00008-2
Wezel, A., Rajot, J.-L., and Herbrig, C. (2000). Influence of shrubs on soil characteristics and their function in Sahelian agro-ecosystems in semi-arid Niger. J. Arid Environ. 44, 383–398. doi: 10.1006/jare.1999.0609
Keywords: agroecology, agroforestry, hydraulic redistribution, hydrology, crop water stress, drought, pearl millet, Sahel
Citation: Bogie NA, Bayala R, Diedhiou I, Conklin MH, Fogel ML, Dick RP and Ghezzehei TA (2018) Hydraulic Redistribution by Native Sahelian Shrubs: Bioirrigation to Resist In-Season Drought. Front. Environ. Sci. 6:98. doi: 10.3389/fenvs.2018.00098
Received: 17 May 2018; Accepted: 20 August 2018;
Published: 18 September 2018.
Edited by:
Urs Feller, Universität Bern, SwitzerlandReviewed by:
Robert Rathbone Pattison, Bureau of Land Management, United StatesClaudio Lovisolo, Università degli Studi di Torino, Italy
Copyright © 2018 Bogie, Bayala, Diedhiou, Conklin, Fogel, Dick and Ghezzehei. This is an open-access article distributed under the terms of the Creative Commons Attribution License (CC BY). The use, distribution or reproduction in other forums is permitted, provided the original author(s) and the copyright owner(s) are credited and that the original publication in this journal is cited, in accordance with accepted academic practice. No use, distribution or reproduction is permitted which does not comply with these terms.
*Correspondence: Nathaniel A. Bogie, bmJvZ2llQHVjbWVyY2VkLmVkdQ==
Richard P. Dick, ZGljay43OEBvc3UuZWR1