Chemical Composition of High Organic Carbon Soil Amendments Affects Fertilizer-Derived N2O Emission and Nitrogen Immobilization in an Oxic Sandy Loam
- 1Forschungszentrum Jülich GmbH, Institute of Bio- and Geosciences, Agrosphere (IBG-3), Jülich, Germany
- 2Empa, Swiss Federal Laboratories for Materials Science and Technology, Laboratory for Air Pollution/Environmental Technology, Dübendorf, Switzerland
- 3General and Theoretical Ecology, Institute of Ecology, University of Bremen, Bremen, Germany
- 4Institute of Crop Science and Resource Conservation, Soil Science and Soil Ecology, University of Bonn, Bonn, Germany
Nitrous oxide (N2O) emission is a negative side effect of modern agriculture and a serious issue for global climate change. The combined application of nitrogen (N) fertilizer and high organic carbon soil amendments (HCA) has been regarded as an alternative to promote fertilizer-related N immobilization and enhance nitrogen use efficiency. The effect of HCA on N2O emission and N immobilization highly depends on its chemical composition, as it controls carbon (C) supply to soil microbes and reactivity of lignin-derived phenols to fertilizer-derived N species. Here we present a 127-d laboratory incubation study to explore the N2O emission and N immobilization after combined application of N fertilizer and HCA (wheat straw, spruce sawdust, and commercial alkali lignin) differing in their chemical composition. The 15N labeling technique was used to trace the transformation of fertilizer-N in ammonium (NH4+), nitrate (NO3–), soil organic nitrogen (SON), and N2O. The amendment of wheat straw and spruce sawdust greatly promoted N immobilization and N2O emission, while lignin amendment enhanced the immobilization of fertilizer N. The chemical composition of HCA explained 26% of the total variance of fertilizer-derived N2O emission and N retention via soil microbial biomass, composition of lignin-derived phenols, and nitrification. The holocellulose/lignin ratio of HCA could be used as an indicator for predicting HCA decomposition, microbial N immobilization and N2O emission. In addition, the composition of lignin-derived phenols was affected by HCA amendment and significantly related to N2O emission and N retention. The varying chemical composition of HCA could thus be a promising tool for controlling N2O emission and N immobilization in environment-friendly and climate-smart agriculture.
Introduction
Since 1920s, the global consumption of industrial N fertilizers in agriculture has increased by 173 million tons in 2013 (FAO, 2015), which has caused serious environmental problems such as nitrous oxide (N2O) emission and nitrate (NO3–) leaching (Zhu and Chen, 2002). Agriculture represents the largest worldwide anthropogenic N2O source (Ravishankara et al., 2009). N2O is not only the third-most important greenhouse gas, but also contributes the most to ozone-depletion in the stratosphere after the successful emission reduction of chlorine- and bromine-containing halocarbons (Ravishankara et al., 2009). It has been proposed that the combined application of N fertilizers and high organic carbon soil amendments (HCA) could strongly promote temporary N retention (Baggs et al., 2000; Millar and Baggs, 2004). Immobilized N can be gradually released into soil through ammonification and taken up by plants, hence, this process may finally improve nitrogen use efficiency of fertilizers and mitigate N2O emission (Burger and Jackson, 2003). Although decreasing N2O emissions after HCA application have been reported (Baggs et al., 2000; Gentile et al., 2008), increased emissions occurred in other cases (Frimpong and Baggs, 2010; García-Ruiz et al., 2012; Chen et al., 2013). Understanding the mechanisms involved in N2O emission and N immobilization upon HCA application is thus mandatory for developing and implementing environmentally friendly agricultural practices.
The quantity and quality of applied HCA play an important role in fertilizer-associated N2O emission and N immobilization in the soil. A C/N ratio of 30 is regarded as a threshold above which net N immobilization occurs (Mooshammer et al., 2014). Nonetheless, the application of HCA did not inhibit N2O emissions in highly anoxic soils even when the C/N ratio exceeded 30 (Chen et al., 2013). Some of this contrasting results can be explained by the different quality of organic matter in HCA. The introduction of labile carbon (C) into oxic soils enhanced N immobilization by promoting soil microbial activity, but simultaneously also promoted N2O emissions due to the activation of nitrifiers and denitrifiers (Chen et al., 2013). Nevertheless, several studies found that fertilizer-related N2O emissions were significantly negatively correlated with the lignin and polyphenol content as well as with the (lignin + polyphenol)-to-N ratio in HCA-amended soils (Millar and Baggs, 2004; Garcia-Ruiz and Baggs, 2007; Frimpong and Baggs, 2010). Hence, the chemical composition of HCA could play a decisive role in controlling N2O emission and N retention rates.
Holocellulose (including cellulose and hemicellulose) and lignin are the most abundant C components in plant residues (Kögel-Knaber and Amelung, 2014). Cellulose is a straight chain polymer derived from D-glucose units which are condensed through β(1→4)-glycosidic bonds, while hemicellulose is a polysaccharide containing many different sugar monomers (Kögel-Knaber and Amelung, 2014). Formed by radical polymerization of guaiacyl (G), syringyl (S), and p-hydroxyphenyl (H) units, lignin is a major component of plant cell walls accounting for 10–30% of lignocellulose (Bugg et al., 2011). Due to the high degree of polymerization, the biological degradation of lignin by soil microorganisms can be very slow (Kögel-Knaber and Amelung, 2014). By contrast, holocellulose is more accessible to soil microbes, and its presence may promote microbial activity (Kiem and Kögel-Knabner, 2003; Chen et al., 2018). Thus, both lignin and holocellulose content affect the rate at which plant residues may be decomposed (Rahman et al., 2013), and thus very likely also the rate and degree of microbial N immobilization and N2O emission. Besides, lignin and its derivatives from the different G, S and H units show distinct reactivity to nitrite (NO2–), suggesting that lignin could play a discriminating role in abiotic N2O emission and N immobilization (Wei et al., 2017a, b).
Based on the distinct microbial availability of holocellulose and lignin, we hypothesized that HCA with higher holocellulose content is more efficient in promoting microbial N immobilization and N2O emission than that containing higher lignin. To test this hypothesis, a laboratory incubation study was conducted with a sandy loam sampled from an agricultural site in Kiel, Germany. Wheat straw, sawdust and pure lignin, whose chemical composition strongly differed from each other, were selected as HCA. After the application of 15N-labeled fertilizer, dynamics of fertilizer-derived N2O, ammonium (NH4+), NO3–, and soil organic nitrogen (SON) were monitored for 114 days. The role of chemical composition of HCA was interpreted based on HCA decomposition, N dynamics, and soil conditions. The present study was complementary to a previous study (Reichel et al., 2018), in which the influence of HCA on microbial N transformations was studied, with a common experimental set and shared samples.
Materials and Methods
Materials
Soil (sandy loam, pHCaCl2 = 6.0 ± 0.1, total C = 1.3 ± 0.1%, total N = 0.15 ± 0.01%) was sampled from a Cambic Luvisol of the Achterwehr field (54°19′05″N, 9°58′38″E), Hohenschulen experimental farm, Kiel, Germany in the summer of 2016 for the laboratory incubation experiment. After sampling, soil was air-dried, sieved at 2 mm and homogenized. Highly 15N-enriched ammonium sulfate [(NH4)2SO4, 98 atom% 15N, VWR, Germany] was diluted to 2.65 atom% 15N by mixing it with (NH4)2SO4 of natural 15N abundance (0.367 atom% 15N, VWR, Germany), and applied to the soil equivalent to 50 mg N kg–1 soil.
Wheat straw (total C = 44.4 ± 0.1%, total N = 0.28 ± 0.02%, lignin content = 17%) was also obtained from the Hohenschulen experimental farm close to Kiel, Germany, and spruce sawdust (without bark, total C = 45.8 ± 0.2%, total N = 0.06 ± 0.01%, lignin content = 25%) was obtained in 2015 from Holz Ruser (Bornhöved, Germany). Both materials were ground to <1 mm in a rotary mill before usage. Alkali lignin (total C = 61.6 ± 0.1%, total N = 0.43 ± 0.01%, VWR, Germany) was suspended in deionized water and allowed to stand for 1 h. The soluble components and micro-particles in the suspension were removed, and the precipitated lignin particles were freeze-dried for usage.
Experimental Setup
For the experiment, as described in Reichel et al. (2018), round stainless steel soil columns were used (20 cm diameter × 30 cm height; workshop of Forschungszentrum Jülich, Jülich, Germany). Each soil column was equally divided into 6 equal sectors using polyvinyl chloride (PVC) boards to allow soil sampling at different time without disturbing the structure of the remaining soil. Three treatments with the combined application of N fertilizer and HCA were established: SWF, soil + wheat straw + fertilizer; SSF, soil + spruce sawdust + fertilizer; SLF, soil + alkali lignin + fertilizer. HCA was applied to the soil at a rate of 1.5 g C kg–1 soil (equivalent to 4.5 t C ha–1). Before filling the soil columns, 90% of HCA had been homogeneously mixed with the soil, while the remaining 10% was buried in litter bags at a depth of 2 cm in the soil at the beginning of the pre-incubation period. The litter bags were made of 0.2 μm nylon mesh and their shape matched the sector surface of each column. Neither fertilizer nor HCA was applied to the blank control (S), while only N fertilizer was applied to the fertilizer control (SF). All treatments were prepared in triplicate.
About 8 kg of soil mixed with or without HCA was equally filled into all the six sectors of each soil column. The soil was compacted to field bulk density (1.5 g cm–3) with a custom-made PVC tool that had the same shape (segment of one sixth of a circle) as each single soil column sector. Afterward, the soil was rewetted to 40% of its water holding capacity (WHC, 35 g H2O/100 g dry soil in this study) and pre-incubated for 2 weeks to activate soil microorganisms. Then, N fertilizer dissolved in deionized water was applied at a rate of 50 mg N kg–1 soil. The final soil moisture of all treatments was about 60% of WHC. Soil columns were incubated at room temperature for 114 days after the application of N fertilizer from 22nd November 2016 to 16th March 2017. The mean temperature during the whole experiment ranged from 20.3 to 22.3°C with mean value of 21 ± 1°C, and deionized water was supplied weekly to keep the soil moisture at about 50–60% of WHC.
Gas Sampling and Analysis
Nitrous oxide flux, as well as N2O isotopic composition, was measured 2–3 times per week. For gas sampling, a gas-tight PVC chamber (20 cm diameter × 20 cm height) with one inlet and one outlet was installed on top of the soil column. The outlet was closed with a screwcap with septum, and the inlet was equipped with a rubber tube (50 mm diameter × 1 m length) closed with a clamp. The gas-tightness of the whole setup had been checked with a helium detector before the experiment. As soon as the PVC chamber was installed, 35 ml of headspace gas was sampled four times in 20–40 min intervals through the septum with a syringe and transferred to a 22.5 ml pre-evacuated gas chromatography vial, thereby creating an overpressure in the GC vial. During gas sampling, the clamp on the inlet tube was opened allowing ambient air to flow into the chamber for pressure equilibration. The N2O mixing ratio was measured with a gas chromatograph equipped with an electron capture detector (GC-ECD, 8610C, SRI Instruments, CA, United States). At the end of each regular gas sampling, 120 ml of gas in the headspace was sampled into a 100-ml pre-evacuated glass bottle, and the 15N enrichment of N2O was analyzed with an isotope ratio mass spectrometer (IRMS, IsoPrime 100, Elementar Analysensysteme, Hanau, Germany) coupled to an online pre-concentration unit (TraceGas, Elementar Analysensysteme) according to Heil et al. (2015).
Soil Sampling and Analysis
Soil in each sector of the soil column was sampled destructively. The first soil sampling was performed 7 days before N fertilizer application as a reference, while the remaining sampling events occurred 8, 22, 50, 78, and 114 days after fertilizer application, respectively. After each sampling, emptied sectors were refilled with appropriately shaped PVC blocks to maintain the remaining soil structure and the air-filled headspace. After sampling, the soil was immediately freeze-dried, and then stored at room temperature until analysis.
Soil pH was determined according to the ISO 10390 method (ISO, 2005). In detail, 1 M potassium chloride (KCl, VWR, Germany) solution was mixed with freeze-dried soil at a ratio of 1:5 (w/v) for 2 h. The mixture was centrifuged at 3500 rpm for 20 min, then the suspension was measured with a pH meter (multi 340i, WTW GmbH, Germany). Soil NO concentration was determined according to an improved colorimetric method (Colman, 2010; Homyak et al., 2015): 5 g of fresh soil was mixed with 25 ml of 0.012 M NaOH solution for 2 h to extract NO2–; after filtered through 0.45 μm, NO2– in the extract was reacted with sulfanilamide and N-(1-napthyl)-ethylenediamine in diethylenetriaminepentaacetic acid buffer; finally the colored product was subsequently measured with a spectrophotometer (DU-800, Beckman Coulter, Fullerton, United States) at 560 nm. Dissolved organic carbon (DOC) and dissolved total nitrogen (DTN) were extracted with 0.01 M CaCl2 solution (Gonet and Debska, 2006), while soil moisture was measured with the classical thermo-gravimetric technique (Lekshmi et al., 2014). Soil microbial biomass was determined with the chloroform fumigation-extraction method as described in Reichel et al. (2018).
The contents of NH4+ and NO3–, as well as their 15N-enrichment, were sequentially measured through micro-diffusion and liquid-liquid extraction methods (Mulvaney et al., 1997; Huber et al., 2012): 80 ml of 1 M KCl solution (pre-treated by heating for 16 h at 550°C to minimize background NH4+) was mixed with 8–9 g of freeze-dried soil. The mixture was shaken for 1 h at 200 rpm, then centrifuged for 20 min at 3000 rpm and filtered through 2–3 μm filter paper (grade 305, VWR Germany). A 60 ml aliquot of KCl extract was transferred to 100-ml polypropylene bottles and adjusted to pH ∼12 with 1 M NaOH solution to deprotonate NH4+ to NH3, which was evaporated and collected from the headspace using an oxalic acid (2 × 15 μl) disk trap (quartz glass, VWR, Germany) for 7 d at room temperature. Afterward, the filter disks were dried in a desiccator over silica gel for 24 h, then packed in Sn capsules (10 × 10 mm) and analyzed using an elemental analyzer coupled to an isotope ratio mass spectrometer (EA-IRMS, Flash EA 2000 and Delta V Plus; Thermo Fisher Scientific, Bremen, Germany). After micro-diffusion, the remaining KCl extract was dried at around 65°C for about 3 d, re-dissolved in 3 ml of 1 M NaOH, and then mixed with 37 ml of acetone for 30 s. The mixture was centrifuged for 20 min at 3000 rpm, and the supernatant acetone phase containing NO3– was transferred into a glass beaker and dried at 30–40°C overnight. The dry residue was re-dissolved in 5 ml of deionized water, freeze-dried, packed in Sn capsules (5 × 9 mm), and analyzed with EA-IRMS. To analyze the immobilized SON, water-soluble N substances, such as NH4+, NO3–, and dissolved organic nitrogen (DON), were removed by washing the soil samples with 1 M KCl solution at a ratio of 1:5 (w/v) for three times. The water-soluble N free soil was collected by centrifugation, freeze-dried, packed in Sn capsules (5 × 9 mm), and analyzed using EA-IRMS. The calculated recovery of 15N in the soil ranged from 71 to 101%. Fertilizer-derived DON was estimated by subtracting fertilizer-derived SON, NH4+, and NO3– from total fertilizer-N in the soil.
The composition of lignin-derived phenols in the soil was characterized according to the cupric oxide (CuO) oxidation method (Hedges and Ertel, 1982; Amelung et al., 1997): aryl ether bonds of lignin were cleaved using CuO under alkaline pH, and phenolic monomers released were separated on a GC (HP 6890, Agilent, CA, United States) equipped with an HP-5 capillary column (30 m × 0.32 mm × 0.25 μm film thickness) and quantified with a flame ionization detector (FID). Lignin G units were calculated as the sum of vanillin, acetovanillone, and vanillic acid, while lignin S units were represented by the sum of acetosyringone, syringaldehyde, and syringic acid. Finally, p-cumaric acid and ferulic acid represented the lignin H units.
HCA Sampling and Analysis
High organic carbon soil amendments in litter bags buried in each soil sector was sampled and freeze-dried together with the soil. Freeze-dried litter bags were carefully cleaned with a brush to remove the soil dust. The remaining organic material was weighed to calculate the decomposition rate. Total lignin in wheat straw and spruce sawdust was extracted according to the Klason method (Schwanninger and Hinterstoisser, 2002; Nicholson et al., 2014): 20–30 mg of HCA was weighed into 50-ml centrifuge tubes, rinsed with acetone, and then stepwise hydrolyzed with 300 μl of 72% H2SO4 for 16–20 h at 5°C and 7.2 ml of 3% H2SO4 for 4 h at 105°C. The lignin precipitate was centrifuged at 3500 rpm for 25 min, washed with hot water (80°C) twice and then freeze-dried for analysis. Holocellulose was extracted according to Wissel et al. (2008): 20–30 mg of HCA was weighed into 50-ml centrifuge tubes, bleached in 7% NaClO2 at pH 3 (adjusted with 100% acetic acid) at 60°C for 10 h, and centrifuged at 3500 rpm for 25 min. Then, the precipitated holocellulose was freeze-dried after having been washed twice with hot water (80°C). The immobilization of fertilizer-N in HCA was determined with EA-IRMS after washing the HCA with 1 M KCl solution at a ratio of 1:5 (w/v) three times. The holocellulose/lignin ratio of HCA was calculated by dividing the holocellulose content with total lignin content.
Calculations and Statistical Analysis
The N2O flux was calculated from the increase of their mixing ratios during the total sampling time and related to the total mass of soil according to the following equation:
E, N2O flux (ng N kg–1 soil h–1); t1:t4, sampling time (h); C1:C4, N2O mixing ratio in the headspace at each sampling time (ppm); V, the volume of the headspace (L); m, the dry weight of the remaining soil (kg); T, air temperature (°C); 22.4, molar volume of ideal gas at 0°C and 101.325 kPa (L); 28, the molecular weight of N in N2O.
Fertilizer-derived N2O, NH4+, NO3–, and SON were calculated according to a two-end-member mixing model based on their 15N-enrichment:
Mfer, Mnat, and Mtot denoted the amount of fertilizer-derived, native, and total N2O (or NH4+, NO3–, SON), respectively; 15Nfer, 15Nloc, and 15Ntot were the 15N enrichment of fertilizer-derived, native, and total N2O (or NH4+, NO3–, SON), respectively. The δ15N value in the blank control served as the value of native 15N enrichment.
Shapiro-Wilk tests showed that data were normally distributed (p < 0.05), therefore, one-way analysis of variance (ANOVA) with Tukey post hoc test at p = 0.05 was used to analyze the significance of difference between treatments. One-way ANOVA, as well as Pearson correlation between N species and HCA parameters, were performed using Origin 8.0 (Originlab Corporation, Wellesley Hills, MA, United States). Redundancy analysis (RDA)-based variation partitioning analysis (VPA) was used to test the contribution of HCA chemical composition to the total variance of fertilizer-derived N2O emission and N immobilization, and the relative response factors of HCA decomposition, holocellulose, and lignin were evaluated by path analysis. Path analysis and VPA were completed using RStudio (Version 1.1.463, R Development Core Team, Vienna, Austria) with packages lavaan 0.6–3, semPlot 1.1, OpenMx 2.12.1, tidyverse 1.2.1, knitr 1.21, kableExtra 1.0.1, GGally 1.4.0, and Vegan 2.5–3. Data were not transformed prior to statistical testing.
Results
Fate of Fertilizer N
Fertilizer-derived NH4+ was quickly converted to NO3–, N2O, or SON after application to the soil; only approximately 23–29% of the initial 15NH4+ was left at day 8, and almost all of the applied 15NH4+ had disappeared 11 weeks later (Figure 1A). Correspondingly, the net NH4+ consumption rate decreased from 5.0–5.5 μg N g–1 soil d–1 to 0.2–0.3 μg N g–1 soil d–1, while there was no significant difference of NH4+ consumption between the four treatments with fertilizer application. Fertilizer-derived NH4+ accounted for more than 90% of the total NH4+ at the beginning of the experiment, but for less than 3% at the end of the incubation period (Figure 2C). In line with the oxidation of NH4+, the concentrations of total NH4+ (the sum of fertilizer-derived and local NH4+) decreased from around 53 μg N g g–1 soil to about 2 μg N g g–1 soil (Supplementary Figure S1E).
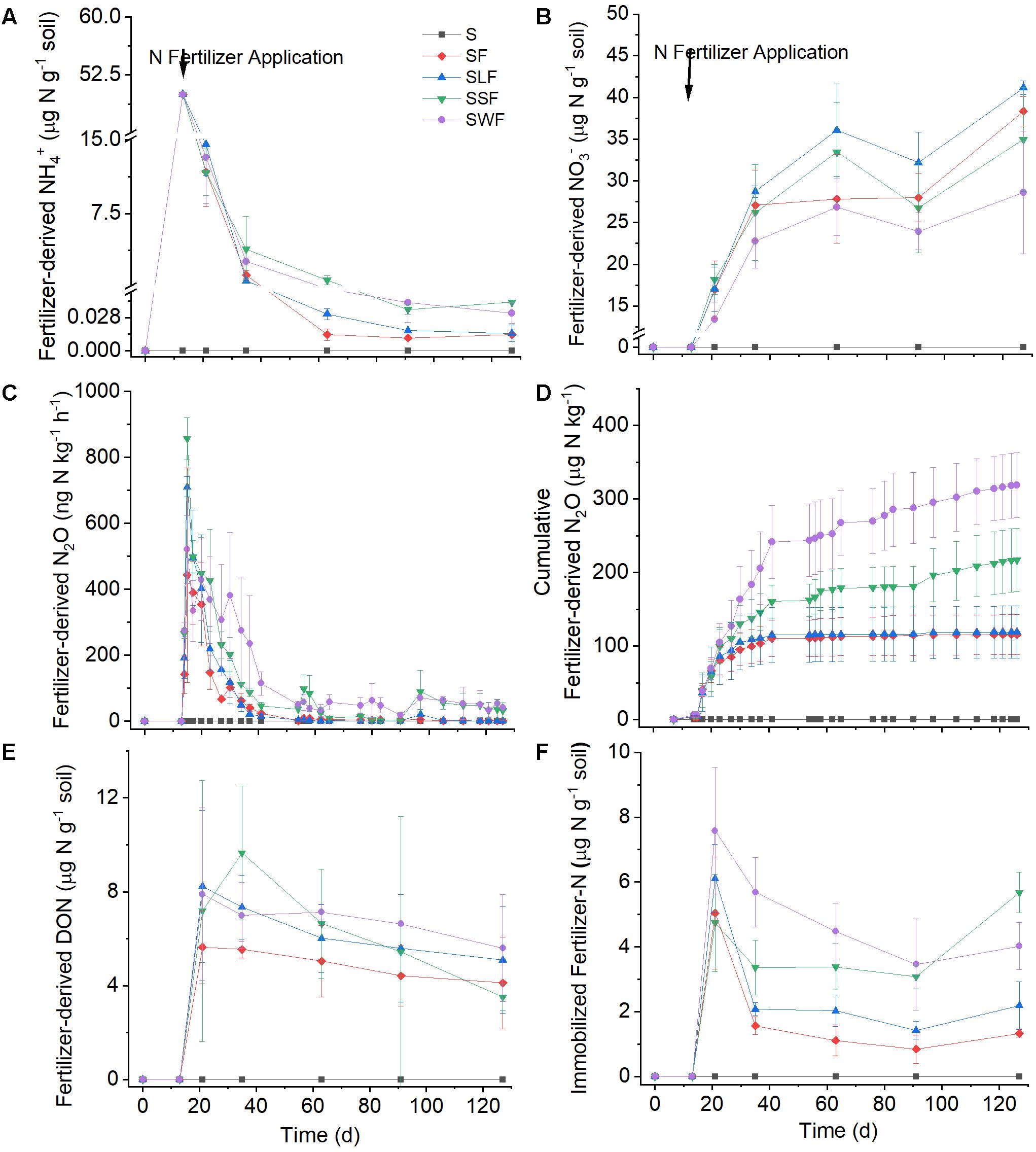
Figure 1. Dynamics of fertilizer-derived NH4+ (A), fertilizer-derived NO3– (B), fertilizer-derived N2O (C), cumulative fertilizer-derived N2O (D), fertilizer-derived DON (E), and immobilization of fertilizer-N in SON (F) in blank control (S, black squares), fertilizer control (SF, red diamonds), as well as after combined application of fertilizer with lignin (SLF, blue up-ward triangles), spruce sawdust (SSF, green down-ward triangles), or wheat straw (SWF, violet dots). Data are shown as mean value ± standard deviation (n = 3).
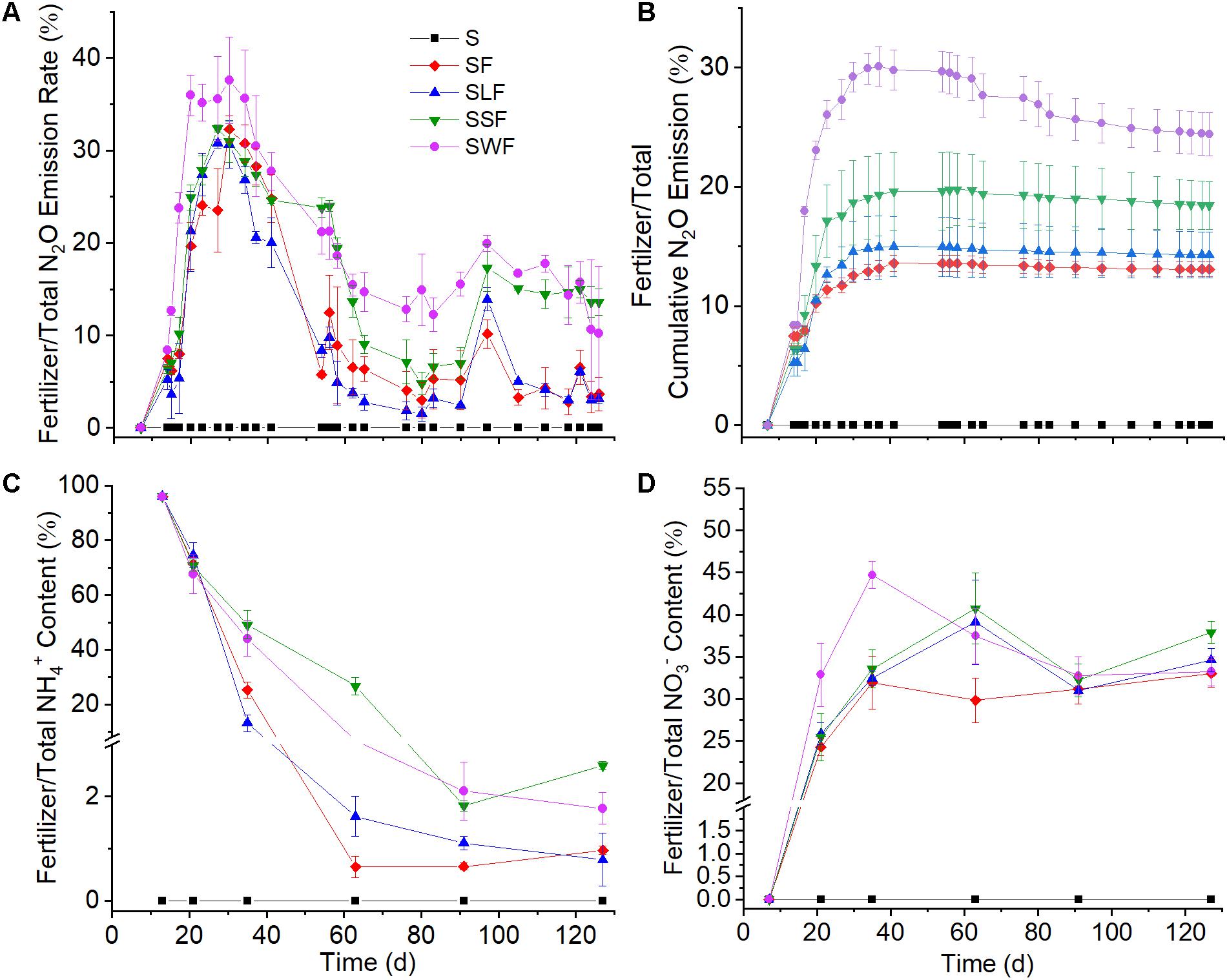
Figure 2. N2O emission dynamics (A), ratios of fertilizer to total cumulative N2O emission (B), NH4+ concentration (C), and NO3– content (D) during the experiment. Blank control (S, black squares), fertilizer control (SF, red diamonds), combined application of fertilizer and lignin (SLF, blue up-ward triangles), combined application of fertilizer and spruce sawdust (SSF, green down-ward triangles), and combined application of fertilizer and wheat straw (SWF, violet dots). Data are shown as mean value ± standard deviation (n = 3).
Fertilizer-Derived N2O Emission
As soon as 15N-NH4+ had been applied, a part of the 15N was immediately emitted to the atmosphere as N2O (Figure 1C). The highest fertilizer-derived N2O emission rate (more than 800 ng N kg–1 h–1) occurred one day after fertilizer application, it then decreased quickly to less than 100 ng N kg–1 h–1 in the following 26 days. The application of wheat straw and spruce sawdust greatly enhanced fertilizer-derived N2O emission compared with the fertilizer control, while there was no significant difference (p > 0.05) of fertilizer-derived N2O emission between SLF and SF treatments. Around 60 days later, the cumulative N2O emission in SWF and SSF treatments were significantly higher compared with SF and SLF treatments (Figure 1D). At the end of the experiment, cumulative fertilizer-derived N2O amounted to 126, 157, 275, and 341 μg N kg–1 in SF, SLF, SSF, and SWF treatments, respectively, accounting for 0.2–0.7% of applied fertilizer-N (Figure 1D). Even though fertilizer-derived N2O peaked one day after fertilizer application, the ratio of fertilizer/total N2O emission rate reached the maximum 16 days later (Figure 2A). Among all the treatments, the ratio of fertilizer-to-total cumulative N2O emission rate was the highest in SWF treatment followed by SSF, and SLF (Figure 2B). Cumulative fertilizer-derived N2O accounted for 12.0% of the total N2O emission in SF, for 15.1% in SSF, for 8.8% in SLF and for 23.6% in SWF treatments at the end of the experiment (Figure 2B).
Immobilization of Fertilizer-N in the Soil
A substantial amount (9.5–15.2%, corresponding to 4.7–7.6 μg N g–1 soil) of fertilizer-N was immobilized in SON within 7 days after the application of N fertilizer, while immobilized fertilizer-N decreased slowly to 0.8–3.5 μg N g–1 soil in the following 9 weeks (Figure 1F). There was no significant difference of N immobilization in SON between different treatments in the first week after fertilizer application. However, the release of immobilized N was much slower in HCA amended soils, resulting in significantly (p < 0.05) larger fertilizer-derived SON in SWF, SSF, and SLF treatments compared with the fertilizer-only control. By the end of the experiment, N immobilization in SON was 2–9% larger in HCA-amended soil than in the fertilizer control.
The retention of fertilizer-N (7.0–19.3% of applied fertilizer-N) in DON was comparable with that of SON, while no significant difference (p > 0.05) between the treatments was observed (Figure 1E). Considering the N retention in both SON and DON, the highest N immobilization (21.7–31.0% of applied fertilizer-N) in the soil occurred 1 week after fertilizer application, followed by a slow release of immobilized N afterward. In addition, N immobilization was most enhanced after wheat straw additions, followed by those of spruce sawdust and lignin.
Fertilizer-Derived NO3–
Concomitant with the quick consumption of 15NH4+, the concentration of fertilizer-derived NO3– increased substantially from 0 to 28.6–41.1 μg N g–1 soil toward the end of the incubation period with the average net NO3– production rate ranging from 1.0 to 1.3 μg N g–1 soil d–1 (Figure 1B). At the end of the incubation experiment, 57–82% of fertilizer-N had been transformed into fertilizer-derived NO3–, which accounted for 33–37% of the total NO3– concentration in soil (Figure 2D). Compared with the fertilizer control, fertilizer-derived NO3– exhibited much lower concentrations in wheat straw amended soils, but higher ones in the lignin amended soils. Pearson correlation analyses revealed that fertilizer-derived NH4+, N2O, and SON were significantly (p < 0.01) positively correlated with each other, but significantly (p < 0.01) negatively correlated with fertilizer-derived NO3– (Supplementary Table S1).
Decomposition and N Immobilization of HCA
Decomposition of HCA
The contents of holocellulose and Klason lignin in spruce sawdust were about 55 and 25%, respectively, with the remaining 20% being other compounds, such as ash, pigment, and proteins (Figure 3B and Supplementary Figure S2B). After 127 days of incubation, 29.7 ± 13.8% of the spruce sawdust had decomposed, most of which was attributed to holocellulose (Figure 3B). Compared with spruce sawdust, wheat straw contained around 8% less Klason lignin and 6% less holocellulose, while about 67% of wheat straw had decomposed at the end of the experiment (Figure 3A and Supplementary Figure S2A). The average decomposition rate of wheat straw (16.7 mg d–1 kg–1 soil) was significantly (p < 0.01) larger than that of spruce sawdust (7.2 mg d–1 kg–1 soil) and lignin (1.3 mg d–1 kg–1 soil), with holocellulose accounting for most of the HCA decomposition (Figure 3). In 127 days, 91.8 ± 1.8% and 39.4 ± 17.0% of the holocellulose in wheat straw and spruce sawdust, respectively, had been decomposed. Consequently, the ratio of holocellulose/lignin in wheat straw and spruce sawdust decreased from 2.2 to 0.4 and from 2.4 to 1.4, respectively (Supplementary Figure S2). Interestingly, the holocellulose/lignin ratio of HCA was significantly (p < 0.01) negatively correlated with its decomposition rate (Figure 3D), but positively (p < 0.01) with the cumulative fertilizer-derived N2O emission (Figure 4D).
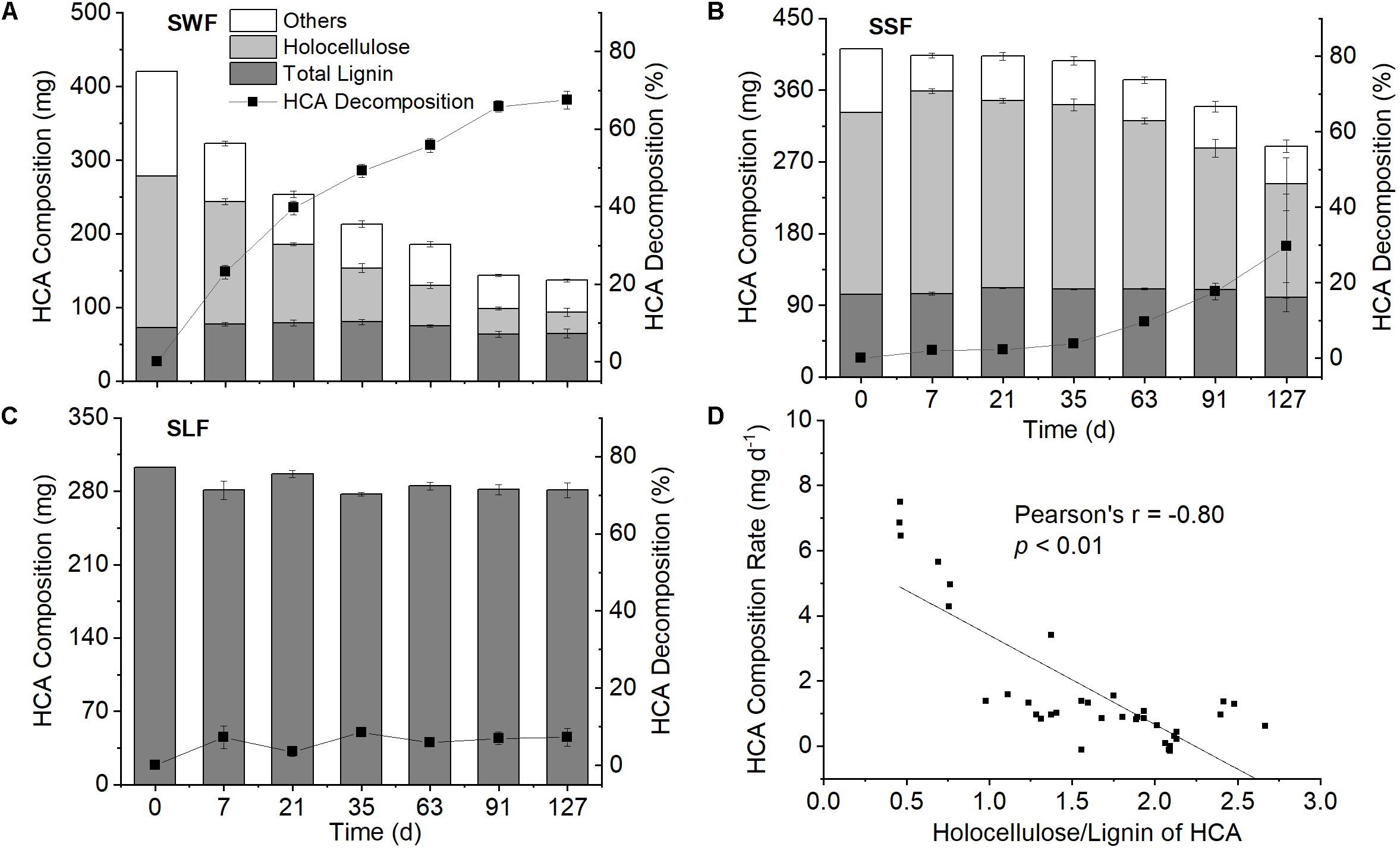
Figure 3. Contents of lignin (dark gray columns), holocellulose (light gray columns), and other compounds (white columns) in high organic carbon soil amendment (HCA), HCA decomposition (black squares) in the wheat straw (SWF, A), spruce sawdust (SSF, B) and lignin treatment (SLF, C), as well as the correlation of HCA decomposition rate with its holocellulose/lignin ratio (D). Data are shown as mean value ± standard deviation (n = 3).
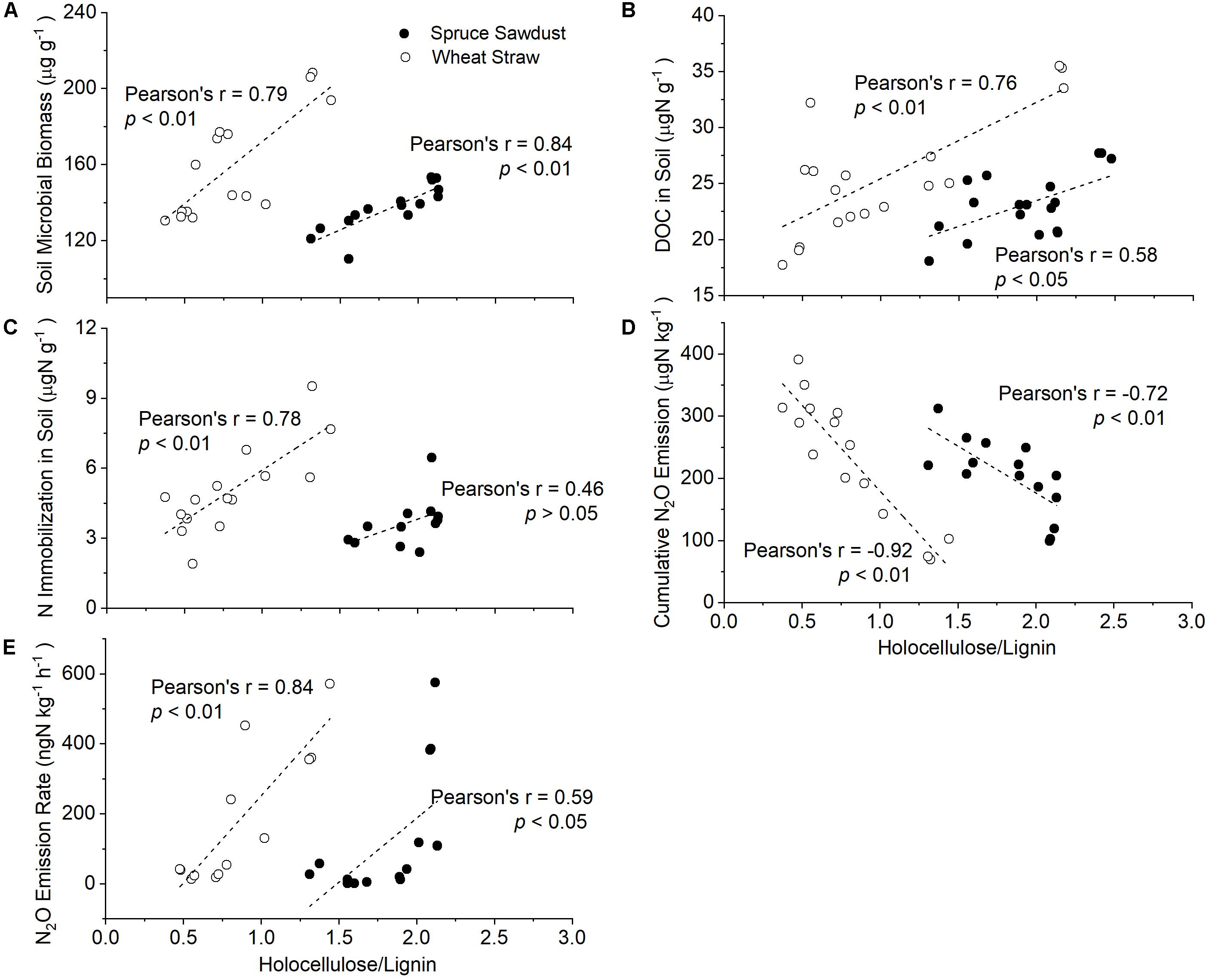
Figure 4. Correlation of the holocellulose/lignin ratio of HCA with soil microbial biomass (A), as well as with the content of dissolved organic carbon (DOC) (B), the N immobilization in the soil (C), cumulative N2O emission (D), and N2O emission rate (E).
N Immobilization in HCA
The immobilization of fertilizer-N in HCA was several magnitudes larger than that in the soil (Table 1). The highest N immobilization was found in wheat straw, which was more than four times larger than that in spruce sawdust and about eight times larger than that in lignin. N immobilization continued to increase in both wheat straw and spruce sawdust during the experiment, however, it started to decrease in lignin 63 days later. At the end of the experiment, 7818 μg N g–1 HCA was immobilized in the wheat straw treatment, 1742 μg N g–1 HCA in the spruce sawdust treatment, and 106 μg N g–1 HCA in the lignin treatment. In addition, a positive correlation between N immobilization and holocellulose/lignin ratio of HCA was observed (Figure 4C).
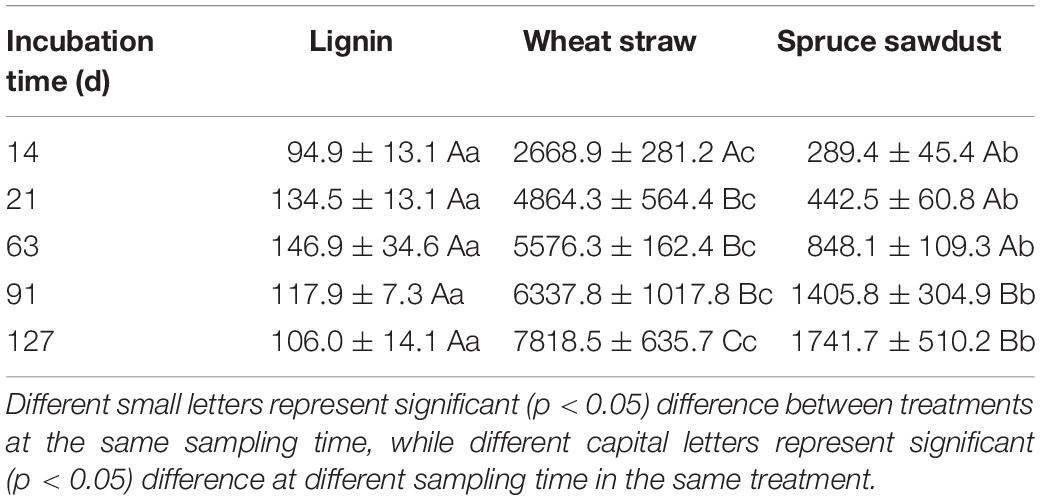
Table 1. N immobilization in HCA (μg N g–1 HCA), data are displayed as mean value ± standard deviation (n = 3).
Soil Conditions
The content of lignin-derived phenols was increased by around 61-100% in the SLF treatment, but only by maximum 33% in the treatments of SWF and SSF compared to the blank control (Figure 5). HCA amendment also largely changed the composition of lignin-derived phenols in the soil. Soil amended with wheat straw was characterized by the highest proportion of lignin S (43-45%) and H (6.8-8.0%) units, but lowest proportion of lignin G (47-49%) units (Table 2). In contrast, lignin-amended soil was characterized by the highest content of lignin G (64-67%) units and lowest content of lignin S (28-31%) and H (3.9-5.9%) units (Table 2). Neither the content nor composition of lignin-derived phenols changed significantly along time (Figure 5). The content of lignin G unit was positively correlated to fertilizer-derived NO3–, it was negatively correlated with fertilizer-derived N2O and SON, and the opposite was found for the lignin S and H units (Supplementary Table S2 and Supplementary Figures S3, S4).
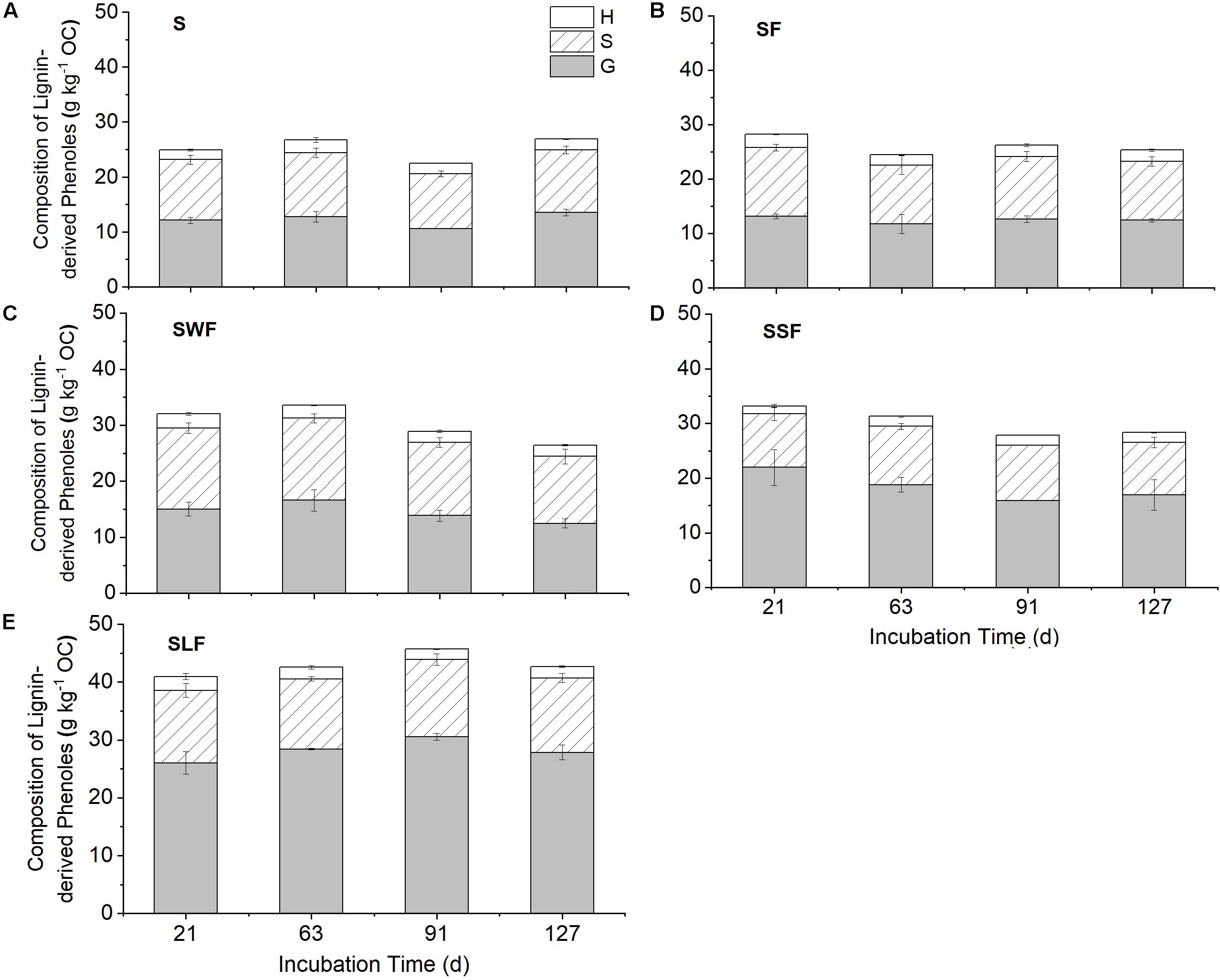
Figure 5. The composition of lignin-derived phenols of guaiacyl (G, gray), syringyl (S, shadowed), and p-hydroxyphenyl (H, white) structural units in blank control (S, A), fertilizer control (SF, B), wheat straw (SWF, C), spruce sawdust (SSF, D), and lignin-amended soil (SLF, E) at days 21, 63, 91, and 127. Data are shown as mean value ± standard deviation (n = 3).
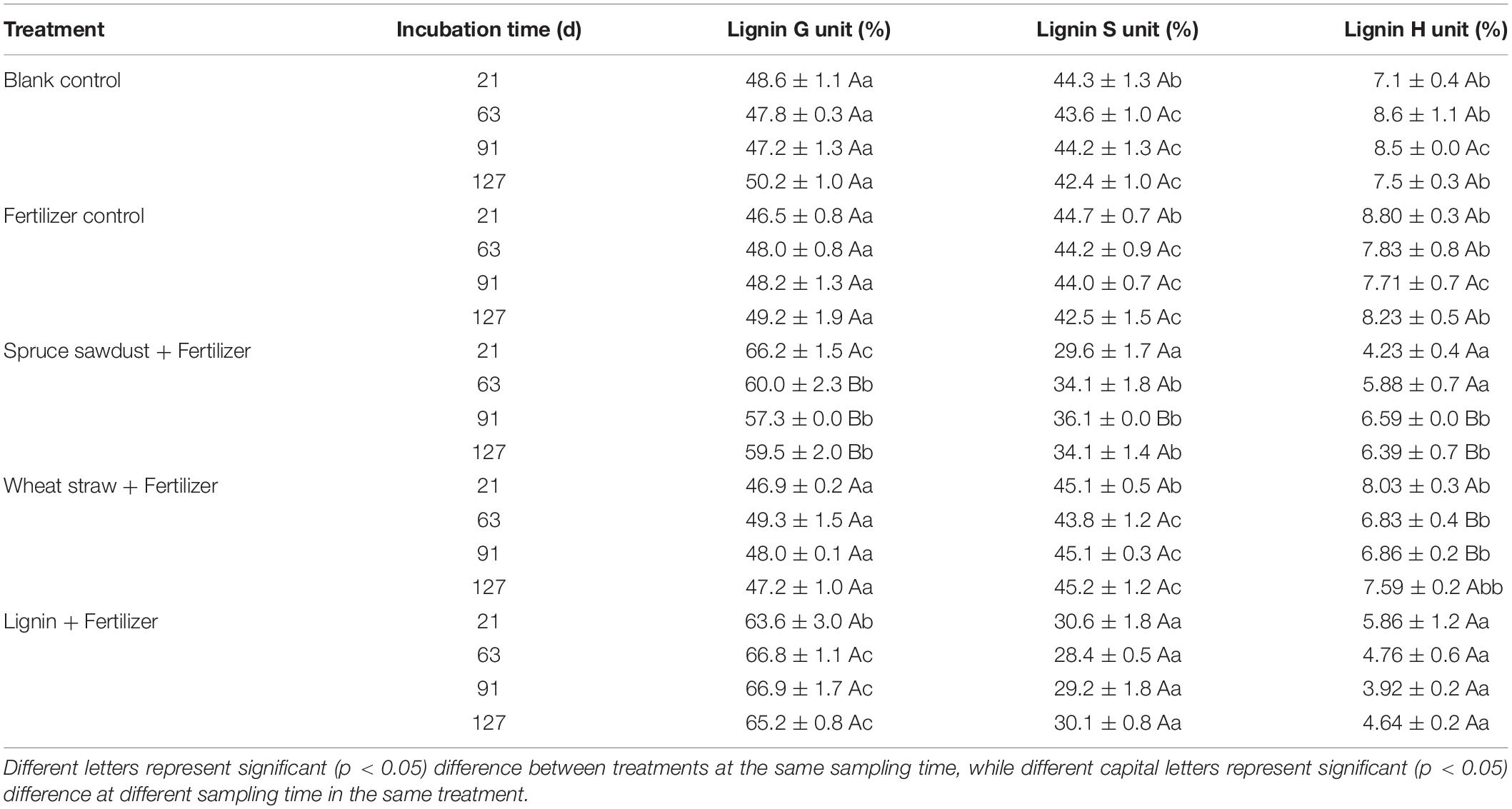
Table 2. Proportions of lignin guaiacyl (G), syringyl (S), and p-hydroxyphenyl (H) units in soils, data are displayed as mean value ± standard deviation (n = 3).
Fertilizer and HCA amendment introduced less than 4% of native N and 10.5% of native C into the soil, resulting in negligible changes of total soil N and C contents. Therefore, no significant (p > 0.05) difference of soil N and C contents between the treatments were observed during the whole experiment, and the soil C/N ratio remained relatively stable at approximately 9. On the other hand, soil pH decreased by about 0.2 units after fertilizer application, accompanied by a decrease of 20-26 μg DOC g–1 dry soil, an increase of 20-70 μg DTN g–1 dry soil, and a decrease of 5-80 μg C g–1 of soil microbial biomass (Supplementary Figure S1). Compared with SF treatment, the amendments of wheat straw and spruce sawdust largely enhanced soil microbial biomass and DOC but decreased total NO3–. By contrast, no significant changes of soil microbial biomass, DOC, nor total NO3– by lignin amendment was observed. In addition, holocellulose/lignin ratio of HCA was significantly (p < 0.01) negatively correlated with soil pH, soil microbial biomass, DOC, and soil nitrite (Figure 4).
Influence of HCA and Soil Conditions on the Fate of Fertilizer-N
Variation partitioning analysis and path analysis were used for sorting the effects of soil-related parameters (soil pH, soil microbial biomass, soil moisture, soil lignin G/S ratio), HCA-related (HCA decomposition rate, holocellulose/lignin ratio, and N immobilization in HCA), and fertilizer-related parameters (fertilizer-derived NH4+ and NO3–) on fertilizer-derived N2O emission and N retention (Figure 6). Fertilizer and HCA alone contributed 8% and 4% to the total variation of fertilizer-derived N2O emission and N retention, respectively (Figure 6A). The combined effect of soil parameters and fertilizer explained 43% of the total variation, while the combined effect of soil parameters and HCA explained 26% of the total variation.
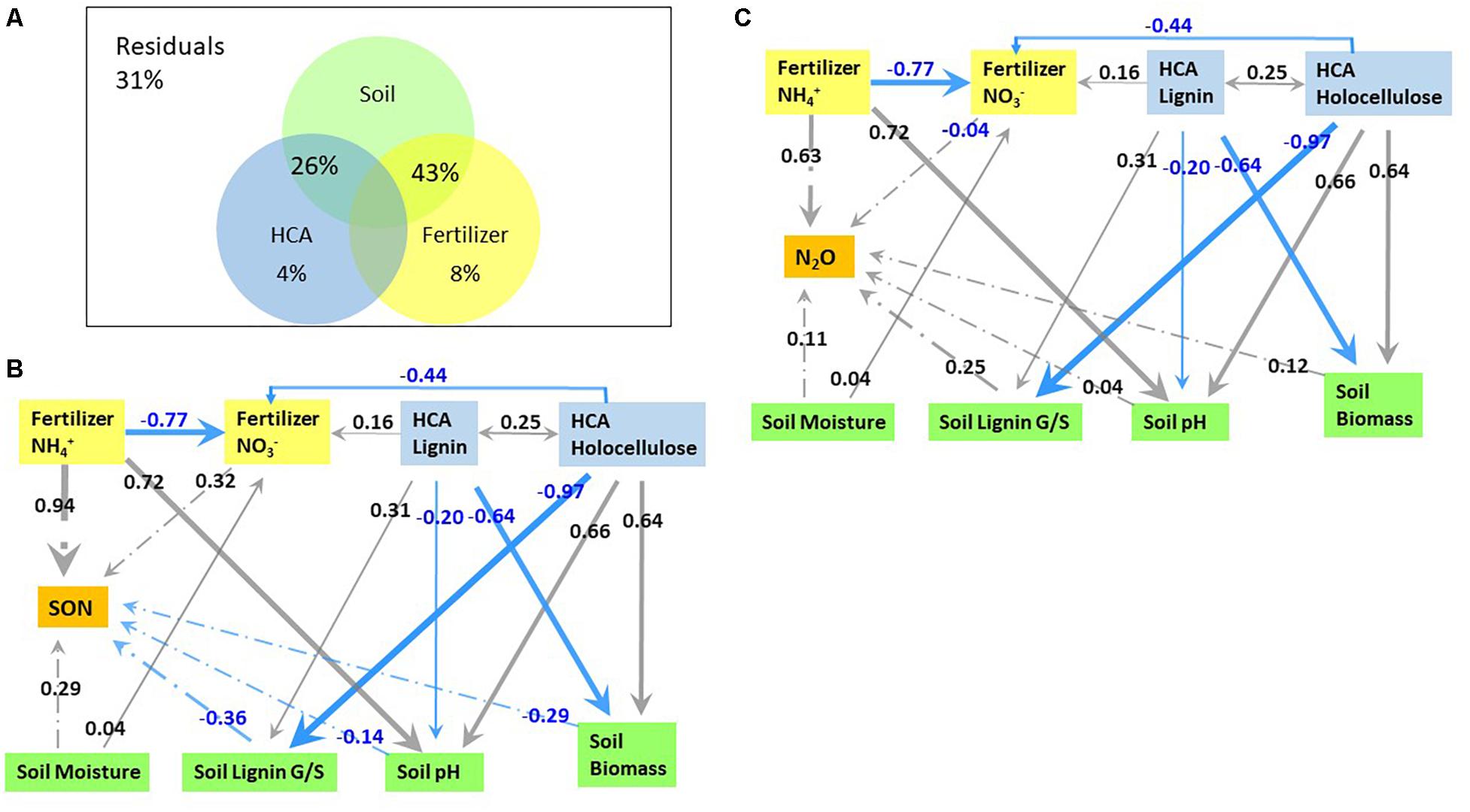
Figure 6. The contribution of high organic carbon soil amendment (HCA) and fertilizer related factors to fertilizer-derived N2O emission and N immobilization in soil (A), as well as the direct and indirect pathways affecting fertilizer-derived N immobilization (B) and N2O emission (C) through path analysis. Numbers in panels B and C are coefficients of each pathway, e.g. a coefficient for the direct effect of fertilizer-derived NH4+ on SON of 0.94 means an increase in fertilizer-derived NH4+ by one unit while keeping all other conditions constant. The effect of indirect pathways can be calculated by multiplying the coefficients of stepwise pathways while adding the coefficients of parallel pathways, e.g. the indirect effect of lignin on N2O emission = –0.64 × 0.12 + –0.20 × 0.25 + 0.31 × 0.25. Only pathways with a coefficient ≥0.03 are illustrated.
Factors directly affecting fertilizer-derived N2O emission included fertilizer-NH4+, soil pH, the composition of lignin-derived phenols, soil microbial biomass, soil moisture, and fertilizer-derived NO3–, with a partial coefficient of 0.63, 0.25, 0.25, 0.12, 0.11, and −0.04, respectively (Figure 6C). The contents of both lignin and holocellulose in HCA acted indirectly on N2O emission by affecting soil pH, fertilizer-derived NO3–, and the soil lignin G/S ratio. Holocellulose affected positively on soil microbial biomass (0.64) and soil pH (0.66), but negatively on soil lignin G/S ratio (−0.97) and fertilizer-derived NO3– (−0.44), resulting in a total correlation coefficient of 0.017 for fertilizer-derived N2O emission. By contrast, lignin influenced negatively on soil microbial biomass (−0.64) and soil pH (−0.20), but positively on the soil lignin G/S ratio (0.31) and fertilizer-derived NO3– (0.16), leading to a total coefficient of −0.056 for fertilizer-derived N2O emission. In addition, fertilizer-derived NH4+ indirectly influenced N2O emission through fertilizer-derived NO3– with an indirect coefficient of 0.03. In summary, the influence of each factor on N2O emission decreased in the order: fertilizer-derived NH4+ (0.66), soil pH (0.25), soil lignin G/S ratio (0.25), soil moisture (0.11), decomposition of HCA lignin (−0.056), fertilizer-derived NO3– (−0.04), and decomposition of HCA holocellulose (0.017).
Fertilizer-derived NH4+ acted on N retention in the soil directly (0.94) and indirectly by affecting fertilizer-derived NO3– (−0.25), resulting in a final coefficient of 0.69 (Figure 6B). By contrast, lignin and holocellulose of HCA affected N immobilization in SON indirectly through soil pH, lignin composition in the soil, fertilizer-derived NO3–, and soil microbial biomass with a coefficient of −0.22 and 0.30, respectively. Soil moisture affected N retention both directly (0.29) and indirectly (0.13), leading to a final coefficient of 0.42. As a result, the immobilization of fertilizer-N was affected mostly by fertilizer-derived NH4+ (0.69), followed by soil moisture (0.42), soil lignin composition (−0.36), fertilizer-derived NO3– (0.32), holocellulose decomposition of HCA (0.30), soil microbial biomass (0.29), lignin decomposition of HCA (−0.22), and soil pH (−0.14).
Discussion
N2O Emission in the Soil
To understand how HCA and fertilizer parameters affect N2O emission and N retention, it is necessary to understand the main pathways involved in N transformation. When applied to the soil, NH4+ is oxidized to NO3– through autotrophic nitrification with N2O as a main side product (Bol et al., 2003), at the meantime, NO3– can be reduced to N2O through denitrification (Russow et al., 2009). It has been reported that N2O emission increased with increasing soil moisture, where 60% of WHC was found to be a threshold below which nitrification acted as the dominant N2O producing process, whereas denitrification would gradually take over as the dominant N2O source above this threshold (Schindlbacher et al., 2004; Bateman and Baggs, 2005). Soil moisture of 50-60% WHC used in the current study is an optimal condition for both autotrophic and heterotrophic nitrification with unrestricted diffusion of N substrates and O2, while autotrophic and heterotrophic nitrification compete for O2 depending on the availability of organic sources and soil pH (Bateman and Baggs, 2005). In this experiment, the fast consumption of fertilizer-NH4+ and accumulation of fertilizer-derived NO3– is a typical nitrification phenomenon (Figure 1), indicating that nitrification dominated N2O emission (Figure 6B). Moreover, the production rate of fertilizer-NO3– was larger than the consumption rate of fertilizer-NH4+, and a decrease of fertilizer-SON in the last 100 days was observed (Figure 1). This decline likely resulted from the oxidation of immobilized SON to NO3– through heterotrophic nitrification (Zhang et al., 2015).
The effects of HCA on fertilizer-derived N2O emission and N retention accounted for 30% of the total variance, mostly via the influence of nitrification, soil microbial biomass, and soil lignin G/S ratio (Figure 6A). Since holocellulose contributed the most to HCA decomposition, while lignin contributed the least (Figure 3), holocellulose is regarded here as the representative of a C source easily accessible to soil microbes and lignin as the representative of microbial resistant C from the HCA. The lignin-containing complex in plant cell walls acts as a natural barrier preventing microbial access to C source in the cell, and biological decomposition of plant residues is in general negatively correlated with their lignin content (Pauly and Keegstra, 2008). A negative effect of HCA lignin content, but positive effect of HCA holocellulose content on soil microbial biomass was found in our study (Figure 6B). Therefore, the holocellulose/lignin ratio is regarded as a factor controlling both C input into the soil and subsequent microbial N2O emission and N immobilization rates. In our study, the HCA decomposition rate largely decreased along decreasing holocellulose/lignin ratio (Figure 3D), resulting in less C input into soil and less soil microbial biomass (Figure 4A). The much larger N2O emission and N retention in SSF and SWF treatments compared with those in the SF treatment can be explained by promoted microbial N2O production and N uptake (Figure 1).
Despite the lower soil microbial biomass (Supplementary Figure S1), N2O emission was slightly larger in the SLF treatment than in the fertilizer control (Figure 1D). We attribute this finding to chemodenitrification (Zhu-Barker et al., 2015; Ostrom et al., 2016; Wankel et al., 2017; Wei et al., 2017a). Phenolic compounds released from lignin decomposition have a strong capability of retaining N substances and chemically reducing NO2– to N2O (Smolander et al., 2012). We found that fertilizer-derived N2O emission was significantly (p < 0.05) related to the composition of lignin-derived phenols in the soil (Supplementary Table S2 and Supplementary Figure S3), which was in accordance with previous studies (Millar and Baggs, 2004; Garcia-Ruiz and Baggs, 2007; Frimpong and Baggs, 2010; Wei et al., 2017a, b). Above all, nitrification, denitrification, and chemodenitrification contributed to N2O emissions, with nitrification dominating the N2O source especially at the beginning of the experiment.
N Immobilization in the Soil
As N is an essential element for the growth of soil microbes, biotic N retention, namely the uptake of mineral N by soil microbes, is a spontaneous way of fertilizer-N incorporation into SON. Dissolved organic carbon, which can be quickly and easily consumed by soil microbes, is very efficient in reducing mineral N content in the short term by promoting soil microbial biomass and biological N uptake (Burke et al., 2013). However, the microbial immobilization of fertilizer-N is a short- to medium-term effect (Burke et al., 2013), as immobilized N will eventually be mineralized or undergo heterotrophic nitrification and released into the soil again (Figure 1). As high holocellulose/lignin ratios correlated with larger soil microbial biomass and high N2O emission rates, they also related to more efficient N retention in the soil (Figure 4). Gentile et al. (2008) reported that mineral N content was lowered by the combined application of crop residues and urea in different soils, most likely by promoting microbial N uptake. In agreement with this study, we also found that the amendment of both wheat straw and spruce sawdust greatly enhanced soil microbial biomass and N immobilization but decreased DTN content compared with fertilizer control (Figure 1 and Supplementary Figure S1).
In addition to microbial N immobilization, abiotic N fixation by DOC as a function of soil pH, redox potential, and ferric iron content has also been reported (Corre et al., 2007; Torres-Canabate et al., 2008). Both NH4+ and NO2– are chemically reactive to various soil organic compounds, such as humic and fulvic acid-like structures as well as lignin derivatives, leading to the formation of SON and to abiotic N retention (Thorn and Mikita, 2000; Fitzhugh et al., 2003). Moreover, it has been proven that NO3– can be incorporated into SON through the so-called ferrous wheel mechanism under various conditions (Davidson et al., 2003; Fitzhugh et al., 2003; Corre et al., 2007; Torres-Canabate et al., 2008). Regardless of the similarity in the contents of soil microbial biomass and DOC in SF and SLF treatments (Supplementary Figure S1), the N retention in the SLF treatment was significantly larger than that in the SF treatment (Figure 1F), which could be explained by abiotic N immobilization. According to Schmidt-Rohr et al. (2004), lignin-derived phenols may chemically react with NH4+, leading to chemical immobilization of fertilizer-N in the soil. The abiotic formation of organic N compounds, e.g. oximes, amides, pyrroles, and nitrophenols, has been found in various chemical reactions of NO2– and NH4+ with SOM (Nommik and Nilsson, 1963; Azhar et al., 1989; Rousseau and Rosazza, 1998; Thorn and Mikita, 2000).
Application of HCA in Agricultural Management
Organic amendments based on locally available plant residues or organic waste is regarded as an alternative that could be an economic way to improve soil quality and fertilizer use efficiency in agricultural management (Gentile et al., 2008). The application of HCA to soil promotes short-term N immobilization by increasing microbial N uptake and chemical N fixation (Baggs et al., 2000; Frimpong and Baggs, 2010; Cucu et al., 2014). Compared with the fertilizer control, the retention of fertilizer-N in HCA-amended soil increased by 50-200% with decreasing lignin content of the HCA in our study (Figure 1F). However, it should be noted that only less than 15% of fertilizer-N was immobilized as SON, and most of the rest prevailed in the form of NO3– (Figure 1B), which could be used by crops, or leached into groundwater or surface water in case of heavy rains or flooding (Schnebelen et al., 2004). Soil moisture was maintained at 50-60% WHC in this study, which favors nitrification instead of denitrification. Therefore, further work is needed to explore the consumption of fertilizer-derived NO3– by crops, as well as N2O emissions under denitrification-favored conditions, before one can state unambiguously if the combined application of HCA and N fertilizer could enhance the nitrogen fertilizer use efficiency in agriculture.
No obvious mitigation of fertilizer-derived N2O emission by HCA was found in our study, instead, the application of wheat straw and spruce sawdust promoted fertilizer-derived N2O emission in the sandy soil by 170 and 120%, respectively (Figure 1D). In agreement with our results, Frimpong and Baggs (2010) reported that the application of leguminous residues (Vigna, Mucuna, and Leucaena) enhanced N2O emission by up to 200% in a reddish brown sandy loam. High availability of amended C can strongly support the growth of microbial communities favoring fast C cycling and N reducing, which finally results in an increase of soil N2O emissions (Rummel et al., 2019). The holocellulose/lignin ratio, acted as an important indicator for soil microbial biomass development, microbial N2O production and N retention. Both N2O emission and N immobilization increased with increasing holocellulose/lignin ratio of HCA. However, no significant increase of N2O emission was observed in lignin-amended soil, whereas N immobilization increased by 50% compared with the fertilizer control (Figure 1). Chemical composition of HCA controls the C availability and quality for N transformation processes, subsequently, acts as the driving force of sustainable agriculture (Rummel et al., 2019). Successive amendment of organics in two olive cultivars for 2 years increased the soil cation exchange capacity by about 2.5 meq 100 g–1 and promoted C assimilation rate of plants by 3.5-3.8 μmol m2 s–1, which finally lead to an increase of plant yield (Roussos et al., 2017). The chemical composition of HCA, especially its holocellulose/lignin ratio, should thus be considered when using HCA amendments for improving climate-smart agriculture practice (Millar and Baggs, 2004; García-Ruiz et al., 2012; Chen et al., 2013; Cucu et al., 2014).
Conclusion
Here, we studied the fate of fertilizer-N after combined application of fertilizer and HCA to an agricultural sandy loam. The chemical composition of HCA, as reflected by different holocellulose/lignin ratio and lignin content and composition, affected largely the fertilizer-derived N2O emission and N immobilization in the soil. The holocellulose/lignin ratio proved to be a useful indicator for C input into the soil and subsequent N2O emission and N immobilization by soil microorganisms. Particularly the amendments with wheat straw and spruce sawdust, both exhibiting elevated holocellulose/lignin ratio, significantly increased the contents of soil microbial biomass and thereby promoted both microbial N2O emission and N immobilization. By contrast, a significant increase of N immobilization and slight increase of N2O emission were found in lignin-amended soil. Biotic pathways dominated during the whole experiment: nitrification together with less pronounced denitrification for N2O emission and microbial N uptake for N immobilization. The composition of lignin-derived phenols also significantly correlated with N2O emissions and N immobilization, indicating lignin-related chemodenitrification and N retention. Lignin-related N immobilization was less pronounced than that of holocellulose-related microbial N uptake, but at the same time caused less climate-relevant N2O emission to an agricultural sandy loam soil.
Data Availability Statement
The raw data supporting the conclusions of this article will be made available by the authors, without undue reservation, to any qualified researcher on request.
Author Contributions
JW, NB, and RR designed the experiment. RR, MI, and JW performed the sampling. JW, MI, and HW performed the δ15N measurements of N2O, NH4+, NO3–, and SON. RR performed the analysis of soil microbial biomass. MI and JW performed the analysis of NO2– and soil pH. HW analyzed the decomposition of holocellulose and lignin of HCA. JW and WA conducted the lignin analysis of soil samples. JW performed the statistical analysis. JW and NB wrote the manuscript. JW, RR, MI, WA, HW, and NB revised the manuscript.
Funding
This study was supported by the BMBF in the frame of the BonaRes project INPLAMINT (FKZ 031A561A). JW acknowledges her funding by the Chinese Scholarship Council (scholarship no. 201406890023).
Conflict of Interest
The authors declare that the research was conducted in the absence of any commercial or financial relationships that could be construed as a potential conflict of interest.
Acknowledgments
All the authors would like to thank Christian Giese and Arne Kappenberg for their kind support during the lignin analysis and Franz Leistner for GC analysis.
Supplementary Material
The Supplementary Material for this article can be found online at: https://www.frontiersin.org/articles/10.3389/fenvs.2020.00015/full#supplementary-material
References
Amelung, W., Zech, W., and Flach, K. W. (1997). Climatic effects on soil organic matter composition in the Great Plains. Soil Sci. Soc. Am. J. 61, 115–123. doi: 10.2136/sssaj1997.03615995006100010018x
Azhar, E. S., Verhe, R., Proot, M., Sandra, P., and Verstraete, W. (1989). Fixation of nitrite nitrogen during the humification of alpha-naphthol in soil suspensions. J. Agric. Food Chem. 37, 262–266. doi: 10.1021/jf00085a060
Baggs, E. M., Watson, C. A., and Rees, R. M. (2000). The fate of nitrogen from incorporated cover crop and green manure residues. Nutr. Cycl. Agroecosyst. 56, 153–163. doi: 10.1023/a:1009825606341
Bateman, E. J., and Baggs, E. M. (2005). Contributions of nitrification and denitrification to N2O emissions from soils at different water-filled pore space. Biol. Fertil. Soils 41, 379–388. doi: 10.1007/s00374-005-0858-3
Bol, R., Toyoda, S., Yamulki, S., Hawkins, J. M. B., Cardenas, L. M., and Yoshida, N. (2003). Dual isotope and isotopomer ratios of N2O emitted from a temperate grassland soil after fertiliser application. Rapid Commun. Mass Spectr. 17, 2550–2556. doi: 10.1002/rcm.1223
Bugg, T. D., Ahmad, M., Hardiman, E. M., and Singh, R. (2011). The emerging role for bacteria in lignin degradation and bio-product formation. Curr. Opin. Biotechnol. 22, 394–400. doi: 10.1016/j.copbio.2010.10.009
Burger, M., and Jackson, L. E. (2003). Microbial immobilization of ammonium and nitrate in relation to ammonification and nitrification rates in organic and conventional cropping systems. Soil Biol. Biochem. 35, 29–36. doi: 10.1016/S0038-0717(02)00233-X
Burke, I. C., Bontti, E. E., Barrett, J. E., Lowe, P. N., Lauenroth, W. K., and Riggle, R. (2013). Impact of labile and recalcitrant carbon treatments on available nitrogen and plant communities in a semiarid ecosystem. Ecol. Appl. 23, 537–545. doi: 10.1890/12-0015.1
Chen, H., Li, X., Hu, F., and Shi, W. (2013). Soil nitrous oxide emissions following crop residue addition: a meta-analysis. Glob. Chang. Biol. 19, 2956–2964. doi: 10.1111/gcb.12274
Chen, X., Hu, Y., Feng, S., Rui, Y., Zhang, Z., He, H., et al. (2018). Lignin and cellulose dynamics with straw incorporation in two contrasting cropping soils. Sci. Rep. 8:1633. doi: 10.1038/s41598-018-20134-5
Colman, B. P. (2010). Understanding and eliminating iron interference in colorimetric nitrate and nitrite analysis. Environ. Monit. Assess. 165, 633–641. doi: 10.1007/s10661-009-0974-x
Corre, M. D., Brumme, R., Veldkamp, E., and Beese, F. O. (2007). Changes in nitrogen cycling and retention processes in soils under spruce forests along a nitrogen enrichment gradient in Germany. Glob. Chang. Biol. 13, 1509–1527. doi: 10.1111/j.1365-2486.2007.01371.x
Cucu, M. A., Said-Pullicino, D., Maurino, V., Bonifacio, E., Romani, M., and Celi, L. (2014). Influence of redox conditions and rice straw incorporation on nitrogen availability in fertilized paddy soils. Biol. Fertil. Soils 50, 755–764. doi: 10.1007/s00374-013-0893-4
Davidson, E. A., Chorover, J., and Dail, D. B. (2003). A mechanism of abiotic immobilization of nitrate in forest ecosystems: the ferrous wheel hypothesis. Glob. Chang. Biol. 9, 228–236. doi: 10.1046/j.1365-2486.2003.00592.x
FAO (2015). Food and Agriculture Organization of the United Nations (FAO). Current World Fertilizer Trends and Outlook to 2018. Rome: FAO.
Fitzhugh, R. D., Lovett, G. M., and Venterea, R. T. (2003). Biotic and abiotic immobilization of ammonium, nitrite, and nitrate in soils developed under different tree species in the Catskill Mountains, New York, USA. Glob. Chang. Biol. 9, 1591–1601. doi: 10.1046/j.1529-8817.2003.00694.x
Frimpong, K. A., and Baggs, E. M. (2010). Do combined applications of crop residues and inorganic fertilizer lower emission of N2O from soil? Soil Use Manag. 26, 412–424. doi: 10.1111/j.1475-2743.2010.00293.x
Garcia-Ruiz, R., and Baggs, E. M. (2007). N2O emission from soil following combined application of fertiliser-N and ground weed residues. Plant Soil 299, 263–274. doi: 10.1007/s11104-007-9382-6
García-Ruiz, R., Gómez-Muñoz, B., Hatch, D. J., Bol, R., and Baggs, E. M. (2012). Soil mineral N retention and N2O emissions following combined application of 15N-labelled fertiliser and weed residues. Rapid Commun. Mass Spectrom. 26, 2379–2385. doi: 10.1002/rcm.6254
Gentile, R., Vanlauwe, B., Chivenge, P., and Six, J. (2008). Interactive effects from combining fertilizer and organic residue inputs on nitrogen transformations. Soil Biol. Biochem. 40, 2375–2384. doi: 10.1016/j.soilbio.2008.05.018
Gonet, S. S., and Debska, B. (2006). Dissolved organic carbon and dissolved nitrogen in soil under different fertilization treatments. Plant Soil Environ. 52, 55–63. doi: 10.17221/3346-PSE
Hedges, J. I., and Ertel, J. R. (1982). Characterization of lignin by gas capillary chromatography of cupric oxide oxidation products. Anal. Chem. 54, 174–178. doi: 10.1021/ac00239a007
Heil, J., Liu, S., Vereecken, H., and Brüggemann, N. (2015). Abiotic nitrous oxide production from hydroxylamine in soils and their dependence on soil properties. Soil Biol. Biochem. 84, 107–115. doi: 10.1016/j.soilbio.2015.02.022
Homyak, P. M., Vasquez, K. T., Sickman, J. O., Parker, D. R., and Schimel, J. P. (2015). Improving nitrite analysis in soils: drawbacks of the conventional 2 M KCl extraction. Soil Sci. Soc. Am. J. 79, 1237–1242. doi: 10.2136/sssaj2015.02.0061n
Huber, B., Bernasconi, S. M., Pannatier, E. G., and Luster, J. (2012). A simple method for the removal of dissolved organic matter and δ15N analysis of NO3– from freshwater. Rapid Commun. Mass Spectrom. 26, 1475–1480. doi: 10.1002/rcm.6243
ISO (2005). ISO 10390:2005 Soil Quality – Determination of pH. Geneva: International Organization for Standardization.
Kiem, R., and Kögel-Knabner, I. (2003). Contribution of lignin and polysaccharides to the refractory carbon pool in C-depleted arable soils. Soil Biol. Biochem. 35, 101–118. doi: 10.1016/S0038-0717(02)00242-240
Kögel-Knaber, I., and Amelung, W. (2014). “Dynamics, Chemistry, and preservation of organic matter in soils,” in Treatise on Geochemistry, Second Edition, eds H. D. Holland, and K. K. Turekian, (Oxford: Elsevier), 157–215. doi: 10.1016/b978-0-08-095975-7.01012-3
Lekshmi, S., Singh, D. N., and Shojaei Baghini, M. (2014). A critical review of soil moisture measurement. Measurement 54, 92–105. doi: 10.1016/j.measurement.2014.04.007
Millar, N., and Baggs, E. M. (2004). Chemical composition, or quality, of agroforestry residues influences N2O emissions after their addition to soil. Soil Biol. Biochem. 36, 935–943. doi: 10.1016/j.soilbio.2004.02.008
Mooshammer, M., Wanek, W., Hämmerle, I., Fuchslueger, L., Hofhansl, F., Knoltsch, A., et al. (2014). Adjustment of microbial nitrogen use efficiency to carbon:nitrogen imbalances regulates soil nitrogen cycling. Nat. Commun. 5:3694. doi: 10.1038/ncomms4694
Mulvaney, R. L., Khan, S. A., Stevens, W. B., and Mulvaney, C. S. (1997). Improved diffusion methods for determination of inorganic nitrogen in soil extracts and water. Biol. Ferti. Soils 24, 413–420. doi: 10.1007/s003740050266
Nicholson, D., Leavitt, A., and Francis, R. (2014). A three-stage klason method for more accurate determinations of hardwood lignin content. Cellul. Chem. Technol. 48, 53–59.
Nommik, H., and Nilsson, K. O. (1963). Fixation of ammonia by the organic fraction of the soil. Acta Agricul. Scand. 13, 371–390. doi: 10.1080/00015126309435663
Ostrom, N. E., Gandhi, H., Trubl, G., and Murray, A. E. (2016). Chemodenitrification in the cryoecosystem of Lake Vida. Victoria Valley, Antarctica. Geobiology 14, 575–587. doi: 10.1111/gbi.12190
Pauly, M., and Keegstra, K. (2008). Cell-wall carbohydrates and their modification as a resource for biofuels. Plant J. 54, 559–568. doi: 10.1111/j.1365-313X.2008.03463.x
Rahman, M. M., Tsukamoto, J., Rahman, M. M., Yoneyama, A., and Mostafa, K. M. (2013). Lignin and its effects on litter decomposition in forest ecosystems. Chem. Ecol. 29, 540–553. doi: 10.1080/02757540.2013.790380
Ravishankara, A. R., Daniel, J. S., and Portmann, R. W. (2009). Nitrous oxide (N2O): the dominant ozone-depleting substance emitted in the 21st century. Science 326, 123–125. doi: 10.1126/science.1176985
Reichel, R., Wei, J., Islam, M. S., Schmid, C., Wissel, H., Schroder, P., et al. (2018). Potential of wheat straw, spruce sawdust, and lignin as high organic carbon soil amendments to improve agricultural nitrogen retention capacity: an incubation study. Front. Plant Sci. 9:900. doi: 10.3389/fpls.2018.00900
Rousseau, B., and Rosazza, J. P. N. (1998). Reaction of ferulic acid with nitrite: formation of 7-hydroxy-6-methoxy-1,2(4H)-benzoxazin-4-one. J. Agric. Food Chem. 46, 3314–3317. doi: 10.1021/jf980130f
Roussos, P. A., Gasparatos, D., Kechrologou, K., Katsenos, P., and Bouchagier, P. (2017). Impact of organic fertilization on soil properties, plant physiology and yield in two newly planted olive (Olea europaea L.) cultivars under Mediterranean conditions. Sci. Horticul. 220, 11–19. doi: 10.1016/j.scienta.2017.03.019
Rummel, P. S., Pfeiffer, B., Pausch, J., Well, R., Schneider, D., and Dittert, K. (2019). Maize root and shoot litter quality controls short-term CO2 and N2O emissions and bacterial community structure of arable soil. Biogeosci. Discuss. 2019, 1–35. doi: 10.5194/bg-2019-2320
Russow, R., Stange, C. F., and Neue, H. U. (2009). Role of nitrite and nitric oxide in the processes of nitrification and denitrification in soil: results from 15N tracer experiments. Soil Biol. Biochem. 41, 785–795. doi: 10.1016/j.soilbio.2009.01.017
Schindlbacher, A., Zechmeister-Boltenstern, S., and Butterbach-Bahl, K. (2004). Effects of soil moisture and temperature on NO, NO2, and N2O emissions from european forest soils. J. Geophys. Res. Atmos. 109:D17302. doi: 10.1029/2004JD004590
Schmidt-Rohr, K., Mao, J.-D., and Olk, D. C. (2004). Nitrogen-bonded aromatics in soil organic matter and their implications for a yield decline in intensive rice cropping. Proc. Natl. Acad. Sci. U.S.A. 101, 6351–6354. doi: 10.1073/pnas.0401349101
Schnebelen, N., Nicoullaud, B., Bourennane, H., Couturier, A., Verbeque, B., Revalier, C., et al. (2004). The STICS model to predict nitrate leaching following agricultural practices. Agronomie 24, 423–435. doi: 10.1051/agro:2004039
Schwanninger, M., and Hinterstoisser, B. (2002). Klason lignin: modifications to improve the precision of the standardized determination. Holzforschung 56, 161–166. doi: 10.1515/HF.2002.027
Smolander, A., Kanerva, S., Adamczyk, B., and Kitunen, V. (2012). Nitrogen transformations in boreal forest soils-does composition of plant secondary compounds give any explanations? Plant Soil 350, 1–26. doi: 10.1007/s11104-011-0895-897
Thorn, K. A., and Mikita, M. A. (2000). Nitrite fixation by humic substances: nitrogen-15 nuclear magnetic resonance evidence for potential intermediates in chemodenitrification. Soil Sci. Soc. Am. J. 64, 568–582. doi: 10.2136/sssaj2000.642568x
Torres-Canabate, P., Davidson, E. A., Bulygina, E., Garcia-Ruiz, R., and Carreira, J. A. (2008). Abiotic immobilization of nitrate in two soils of relic Abies pinsapo-fir forests under mediterranean climate. Biogeochemistry 91, 1–11. doi: 10.1007/s10533-008-9255-y
Wankel, S. D., Ziebis, W., Buchwald, C., Charoenpong, C., de Beer, D., Dentinger, J., et al. (2017). Evidence for fungal and chemodenitrification based N2O flux from nitrogen impacted coastal sediments. Nat. Commun. 8:15595. doi: 10.1038/ncomms15595
Wei, J., Amelung, W., Lehndorff, E., Schloter, M., Vereecken, H., and Brüggemann, N. (2017a). N2O and NOx emissions by reactions of nitrite with soil organic matter of a Norway spruce forest. Biogeochemistry 132, 325–342. doi: 10.1007/s10533-017-0306-300
Wei, J., Zhou, M., Vereecken, H., and Brüggemann, N. (2017b). Large variability of CO2 and N2O emissions and of 15N site preference of N2O from reactions of nitrite with lignin and its derivatives at different pH. Rapid Commun. Mass Spectrom. 31, 1333–1343. doi: 10.1002/rcm.7912
Wissel, H., Mayr, C., and Lücke, A. (2008). A new approach for the isolation of cellulose from aquatic plant tissue and freshwater sediments for stable isotope analysis. Organ. Geochem. 39, 1545–1561. doi: 10.1016/j.orggeochem.2008.07.014
Zhang, J., Müller, C., and Cai, Z. (2015). Heterotrophic nitrification of organic N and its contribution to nitrous oxide emissions in soils. Soil Biol. Biochem. 84, 199–209. doi: 10.1016/j.soilbio.2015.02.028
Zhu, Z. L., and Chen, D. L. (2002). Nitrogen fertilizer use in China – contributions to food production, impacts on the environment and best management strategies. Nutr. Cycl. Agroecosyst. 63, 117–127. doi: 10.1023/a:1021107026067
Keywords: holocellulose, lignin, nitrogen fertilizer, nitrous oxide, nitrogen immobilization
Citation: Wei J, Reichel R, Islam MS, Wissel H, Amelung W and Brüggemann N (2020) Chemical Composition of High Organic Carbon Soil Amendments Affects Fertilizer-Derived N2O Emission and Nitrogen Immobilization in an Oxic Sandy Loam. Front. Environ. Sci. 8:15. doi: 10.3389/fenvs.2020.00015
Received: 10 April 2019; Accepted: 27 January 2020;
Published: 14 February 2020.
Edited by:
Dionisios Gasparatos, Aristotle University of Thessaloniki, GreeceReviewed by:
George Giannopoulos, Virginia Commonwealth University, United StatesFoteini Giannakopoulou, Independent Researcher, Greece
Copyright © 2020 Wei, Reichel, Islam, Wissel, Amelung and Brüggemann. This is an open-access article distributed under the terms of the Creative Commons Attribution License (CC BY). The use, distribution or reproduction in other forums is permitted, provided the original author(s) and the copyright owner(s) are credited and that the original publication in this journal is cited, in accordance with accepted academic practice. No use, distribution or reproduction is permitted which does not comply with these terms.
*Correspondence: Jing Wei, jing.wei@empa.ch