- 1Department of Biological and Environmental Sciences, University of Gothenburg, Gothenburg, Sweden
- 2School of Design, University of Leeds, Leeds, United Kingdom
Synthetic microfibers have been reported in most aquatic environments and represent a large proportion of environmental microplastics. However, they remain largely under-represented in microplastic ecotoxicity studies. The present study aims to investigate particle interaction with, and retention time in, aquatic organisms comparing microfibers, and microbeads. We used brine shrimp (Artemia sp.) and fish (Gasterosteus aculeatus) as invertebrate and vertebrate models, respectively. Organisms were exposed to a mixture of microbeads (polyethylene, 27–32 μm) and microfibers (dope dyed polyester; 500 μm-long) for 2 h, at high concentrations (100,000 part./L) in order to maximize organism-particles interaction. Artemia were exposed in the presence or absence of food. Fish were exposed either via the trophic route or directly via water, and water exposures were performed either in freshwater or seawater. In the absence of food, Artemia ingested high numbers of microbeads, retained in their digestive tract for up to 96 h. Microfiber ingestion was very limited, and its egestion was fast. In the presence of food, no microfiber was ingested, microbead ingestion was limited, and egestion was fast (48 h). Limited particle ingestion was observed in fish exposed via water, and particle retention time in gut did not exceed 48 h, both for direct and trophic exposure. However, water exposures resulted in a higher number of particles present in gills, and average retention time was higher in gills, compared to gut. This suggests that gills are organs susceptible to microplastic exposure and should be taken into account in fish exposure and effect studies. Our results show that particle ingestion and retention by organisms differ between microbeads and microfibers, suggesting particle selection based on size, shape, and/or color and species-specific selective feeding. We also showed that the presence of food results in limited particle ingestion and retention in Artemia and that microbeads are more likely to be transferred to organisms from upper trophic levels than microfibers. Finally, fish exposure to particles was not significantly different between freshwater and seawater conditions.
Introduction
The presence of synthetic microfibers has been reported in most aquatic environments—from deep sea to intertidal sediments (Mathalon and Hill, 2014; Woodall et al., 2014; Sanchez-Vidal et al., 2018), and in every ocean (Desforges et al., 2014; Lusher et al., 2015). In marine environments, synthetic fibers mostly originate from the degradation of discarded maritime equipment (e.g., nets and ropes), and release during textile production and use (Carney Almroth et al., 2018; Henry et al., 2019; Cai et al., 2020). The highest fiber concentrations have been observed close to urban areas, with up to 200–800 fibers/kg of sediment and up to 4100 fibers/m3 in rivers (Mathalon and Hill, 2014; Zhao et al., 2015). Overall, synthetic fibers represent a significant proportion of microplastics in aquatic environments. Based on a literature review, Burns and Boxall (2018) found that 52% of microplastics are fibers [marine water and fresh water (FW) combined]. Other studies found that 75% (Desforges et al., 2014) and more than 95% (Lusher et al., 2014, 2015) of microplastics found in oceanic waters are fibers. Synthetic microfibers have also been shown to be ingested by organisms. Field studies report their presence in zooplankton, polychaetes, bivalves, crustaceans (Phuong et al., 2016; Bour et al., 2018; Avio et al., 2020), deep-sea organisms (Taylor et al., 2016), fish (Halstead et al., 2018; Avio et al., 2020) and FW fish species (Sanchez et al., 2014; Pazos et al., 2017; Silva-Cavalcanti et al., 2017; Horton et al., 2018). Moreover, many authors do not include fibers in their analyses since they could result from air-borne contamination. Therefore, the presence of fibers in field organisms could currently be underestimated.
Effects studies showed that exposure to microfibers can impact growth, reproduction and survival of FW water fleas, and amphipods (Au et al., 2015; Jemec et al., 2016; Ziajahromi et al., 2017). Decreased food consumption, energy and body condition were observed in shore crabs and langoustine (Watts et al., 2015; Welden and Cowie, 2016). Damaged gill epithelium was also observed in Japanese medaka following long-term exposure to microfibers (Hu et al., 2020). The effects of fiber exposure to soil invertebrates was also investigated, with lower cast production observed for exposed earthworms (Prendergast-Miller et al., 2019), while limited effects were observed on enchytraeids, springtails, isopods and oribatid mites (Selonen et al., 2020). Studies focusing on fiber retention and gut residence time showed that retention time for synthetic microfiber varies from a few hours to a couple of days for small invertebrates, like daphnids, gammarids, sea anemones, and shrimps (Blarer and Burkhardt-Holm, 2016; Jemec et al., 2016; Gray and Weinstein, 2017; Romanó de Orte et al., 2019), and from a few hours to up to 3 weeks for crabs and fish species (Watts et al., 2015; Grigorakis et al., 2017; Ory et al., 2018).
Retention time is an important parameter that directly influences microplastic accumulation in organisms. High retention time and accumulation can lead to increased effects over time, increased chemical transfer from particles to organisms (Koelmans, 2015; Rochman, 2015), and increased potential for trophic transfer. Although the number of studies providing data on microplastic ingestion and retention is continuously growing, most studies use microbeads as model particle. However, there is currently no evidence that organisms present similar ingestion patterns and retention times for microbeads and microfibers. Trophic transfer is also an important parameter to consider, since it can result in increased fiber accumulation in species of higher trophic levels, and can also lead to adverse effects occurring at the food web scale. Despite the relevance of this parameter, the number of studies investigating the trophic transfer of microplastics, all shapes considered, is still limited (Farrell and Nelson, 2013; Setälä et al., 2014; Batel et al., 2016; Au et al., 2017; Rochman et al., 2017; Tosetto et al., 2017).
The present study aims to compare exposure to and retention time of plastic microfibers and microbeads in fish and invertebrate species under different exposure scenarios. Specifically, we tested the following hypotheses: (i) exposure and retention by organisms are different between microbeads and microfibers; (ii) the presence of food influences particle ingestion and retention by invertebrates; (iii) particle ingestion and filtration through the gills are different between FW and seawater (SW) fish; and (iv) microbeads and microfibers can be transferred through the trophic chain. Previous studies suggest that the presence of food can influence microplastic ingestion and retention by invertebrates (Wang et al., 2019; Chae and An, 2020). FW and SW fish present different physiologies, especially concerning the functions, and organizations of gill epithelia and the volumes of water swallowed (Eckert et al., 1988). Marine fish ingest larger volumes of water, potentially resulting in higher particle ingestion (Rochman, 2018). It can therefore be expected that fish gills and digestive tracts present different exposure patterns to contaminants, depending on the environment. For this study, we selected the three-spined stickleback Gasterosteus aculeatus as a model species for its ability to adapt its physiology to different salinity conditions (Bell and Foster, 1994). This species is also broadly used in ecotoxicology and naturally occupies a wide range of aquatic habitats (Jutfelt et al., 2013; Marchand et al., 2017). To test our hypotheses, we exposed brine shrimps (Artemia sp.) to identical concentrations of microbeads and microfibers in the presence or absence of food and quantified particle ingestion and depuration time. We also exposed sticklebacks in FW or SW to the same particle concentrations, and quantified the presence of particles in gills and gut following exposure and over depuration time. In a second step, we combined Artemia and stickleback in the same experiment to study particle trophic transfer. Since microfiber ingestion by Artemia was not concluding, trophic transfer was assessed with microbeads only.
Materials and Methods
Organisms
Three-spined sticklebacks (Gasterosteus aculeatus) were collected in a reference site (Skaftö, Sweden; water salinity: 16–18‰) with a hand-operated net and immediately brought to the laboratory in aerated, thermally isolated boxes containing water from the sampling site. They were then randomly divided in two groups, acclimatized to and kept in either artificial SW (13°C ± 1°C, 30‰) or FW (13°C ± 1°C, 0‰) for a month prior to the start of the experiment. Fish were fed daily with red mosquito larvae. Continuous water flow and aeration ensured good water quality, and environmental enrichment was provided.
Brine shrimps (Artemia sp.) were obtained from commercially available dry cysts (HOBBY Aquaristik, Germany). Cysts were placed in artificial SW (35‰) for 48 h and supplied with strong aeration. After hatching, nauplii were transferred in 30‰ artificial SW and fed everyday with dried phytoplankton powder (HOBBY Aquaristik, Germany). Artemia reached their adult size after 3 weeks.
Microparticles and Suspension Preparation
Microbeads (opaque blue polyethylene microspheres) were purchased from Cospheric (Santa Barbara, United States; lot #120328-2–1). According to the manufacturer, they are spherical (>90%), with a 27–32 μm diameter (>90% of particles in size range), and a density of 1.00 g/cc. The polyester microfibers were extracted from a commercially manufactured yarn. This yarn is a solution dyed black, textured, filament yarn, which is very similar to yarns used in a wide range of clothing products. The yarn has a linear density of 75 denier, with 72 filaments in its cross section. The individual filaments within the cross section are approximately 12 μm in diameter. Black microfibers were used to improve identification and tracking during experimentation. The yarn was further manually cut into 500 μm-long fibers. Microscopic measurement of microfibers (n = 100) indicates median length of 483 ± 13 μm. Particle suspensions were prepared in artificial SW (salinity 30‰) or in FW (salinity 0‰), and specific concentrations were obtained either by weighing microbeads and diluting suspension or by counting microfibers while cutting. Gentle sonication ensured suspensions homogeneity.
Organismal Exposure and Retention Time Determination
Brine Shrimps (Artemia)
Before exposure, Artemia were starved for 24 h. Adult Artemia were exposed in artificial SW to a combination of microbeads and microfibers (50,000 part./L each, 100,000 part./L in total) for 2 h, in the presence or absence of food. These concentrations are not environmentally relevant and were selected to maximize interaction between organisms and particles. Exposures were carried out in triplicate, each replicate comprising 10 individuals, in 100 ml glass flasks.
For the exposure in the absence of food, an initial suspension was prepared (300 ml total volume, comprising 15,000 microfibers and 15,000 microbeads), homogenized by gentle sonication, and distributed between replicates. Artemia were then added to the flasks. After 2 h of exposure, individuals were gently picked, rinsed in clean medium and placed on a petri dish for microscopic observation (Leica EZ4HD stereomicroscope). The number of ingested particles was manually counted, and individuals were placed in clean SW for depuration (grouped by replicate). The number of particles present in Artemia digestive tract was determined at 2, 6, 24, 48, 72, and 96 h of depuration. Individual microbeads and microfibers could easily be detected through the Artemia’s transparent cuticle (Figure 1).
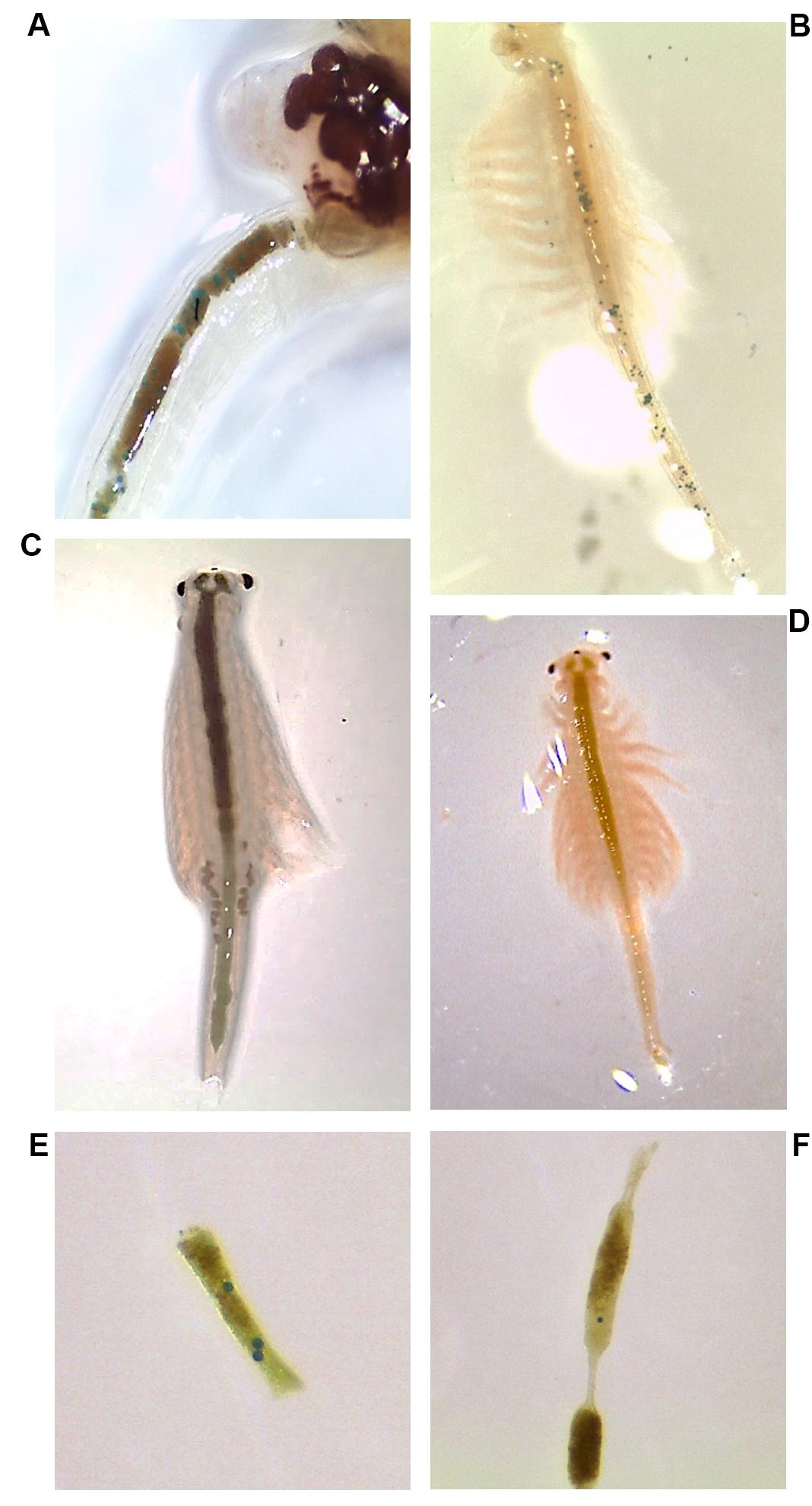
Figure 1. Particle ingestion and egestion by Artemia. (A) Particles present in the Artemia digestive tract after 2 h of exposure. One black microfiber can be seen close to blue microbeads. (B) Trophic chain experiment: Artemia after exposure to microbeads and before being fed to fish. (C) Artemia exposed to particles in the presence of food: the upper digestive tract is filled with food, preventing the observation of microbeads, and microfibers potentially ingested. (D) Artemia after 96 h of depuration. (E,F) Microbeads observed in Artemia feces.
For the exposure in the presence of food, a similar initial suspension was prepared and supplemented with 100 mg of Artemia food (phytoplankton powder, final concentration 333.3 mg/L) before homogenization and distribution between replicates. After 2 h of exposure, the presence of food in Artemia’s digestive tract prevented assessing the presence of microparticles. Therefore, individuals were gently picked, rinsed and individually placed in petri dishes containing clean SW supplemented with food (333.3 mg/L). Microparticles were then quantified in depuration SW and fecal pellets by microscopic observation at 2, 6, 24, and 48 h. Depuration medium was changed after every observation. Individual particles could easily be detected in SW and fecal pellets (Figure 1). Fecal pellets that were too dense to be fully transparent were mechanically torn to ensure the absence of particle. For each individual, the number of ingested microbeads was expressed as the sum of microbeads egested, counted at each observation time.
For both experiments, after 2 h of exposure the water column was sampled from each replicate in order to quantify microparticles in suspension. In the case of the absence of food, visual observation indicated that no particle was present at the bottom of the flasks; therefore the whole volume was sampled. In the case of the presence of food, sedimented microfibers and food were observed at the bottom of the flasks; therefore, only the water column was sampled, excluding the solid deposit. Water samples were filtered on gridded cellulose nitrate filters (12 μm pore size). Particles were counted in five representative cells, and the total number of particles was estimated based on average numbers per cell.
Fish Exposed via Water
Sticklebacks were exposed to a combination of beads and fibers (50,000 part./L each, 100,000 part./L in total) either in SW or FW (20 cm × 12 cm × 13 cm tanks; 4 L). Fish from the same exposure condition (SW: n = 27; FW: n = 26) were grouped in a single replicate. Control conditions (clean SW and FW) were also included (SW: n = 4; FW: n = 8). After 2 h of exposure, fish were transferred to clean water (SW or FW, respectively) for 3 min to allow removal of particles potentially present on their body. Fish from control (SW: n = 1; FW: n = 2) and exposed (SW: n = 6; FW: n = 7) groups were immediately euthanized, and the digestive tracts and gills were sampled. The remaining fish were transferred in new clean water for depuration. Fish from exposed groups were euthanized, and organs were sampled after 2, 24, or 48 h depuration (n = 7 per condition and sampling time). Fish from control groups were euthanized, and organs were sampled after 2 h of depuration. Samples were stored at 4°C before further analysis (see section Fish Samples Processing and Analysis). Water samples (two replicates per condition; 15 ml per sample) were taken from exposure waters immediately after fish exposure, filtered on gridded cellulose nitrate filters (12 μm pore size), and particles were counted.
Fish Exposed via Trophic Chain
Due to the very limited ingestion of microfibers by Artemia, trophic exposure was carried out with microbeads only. As a first step, Artemia were placed for 5 min in 15 ml flasks (six Artemia/flask) containing either microbeads suspension (500,000 particle/L in SW) or SW only (control group). Individuals were then gently rinsed in SW and photographed under microscopic condition for further microbead quantification: pictures were analyzed with ImageJ® software to count the number of ingested microbeads, easily detected through the organisms’ cuticles. Each Artemia was attributed a number, corresponding to the fish it would be fed. In a second step, fish were individualized to ensure equal prey ingestion between individuals. Every fish was fed three Artemia, either pre-exposed to microbead suspension (n = 18 fish) or to SW only (n = 12 fish). All the Artemia were eaten within 5 min, so microbead egestion by Artemia is considered negligible. Three fish from control and exposed groups were immediately euthanized and their digestive tract was sampled. The remaining fish were allowed to depurate in clean SW. Fish from the microbead group were taken at 2, 8, 12, 24, and 48 h of depuration. Fish from the control group were taken at 2, 24, and 48 h of depuration. At each sampling time, three fish per condition were taken and their gills and gut were sampled and stored at 4°C before further analysis (see section Fish Samples Processing and Analysis).
Fish Samples Processing and Analysis
Samples were digested overnight in 10% KOH at 50°C then filtered on 10 μm nylon mesh. This digestion method has been validated for different plastic polymers (Dehaut et al., 2016), and a pilot study was conducted in our laboratory to ensure that it would not alter the microbeads or microfibers’ physical properties (Supplementary Figure 1). Filters were observed under a stereomicroscope, and particles were counted. The polyester fibers studied here have a specific shape, color, and size and were easily distinguished from potential air-borne fibers.
Statistics
All statistical analyses were performed using GraphPad Prism 8 software. For Artemia exposure, ANOVAs were performed to compare the number of ingested microbeads between replicates at each observation time. When the required assumptions were not satisfied, ANOVAs on ranks (Kruskal–Wallis test) were performed instead. For fish water exposure, four factors were considered to compare the number of particles found in samples: depuration time, particle shape (i.e. fiber or bead), organ (i.e. gills or gut) and water salinity (i.e. FW or SW). Since data did not satisfy the assumptions required to perform multi-factor analyses, each factor was analyzed separately in all possible combinations of other factors. ANOVA on ranks (Kruskal–Wallis test) was performed to compare the number of particles found in samples based on depuration time. Particle shape, organ and water salinity were analyzed with t-test or the corresponding non-parametric test (Mann-Whitney) when the required assumptions were not met. Levels of significance were set at p < 0.05.
Results
Artemia
Exposure in the Absence of Food
Ingestion of microfibers was very limited, with only four individuals presenting one fiber each in their gut. Egestion of microfiber was fast, occurring between 2 h and 72 h of depuration. Microbead ingestion rate was much higher, with 100% of individuals having microbeads in their gut (30 ± 3 microbeads/individual on average) after 2 h of exposure. The number of microbeads ingested per individual did not significantly differ between replicates. Complete microbead egestion occurred within 96 h for most individuals, and was almost complete for others: only one or two beads were still present in gut for seven individuals at 96 h of depuration. On average, more than 50% of the ingested microbeads were excreted in less than 24 h (Figure 2A). Detailed particle counts are presented in Supplementary Table 1.
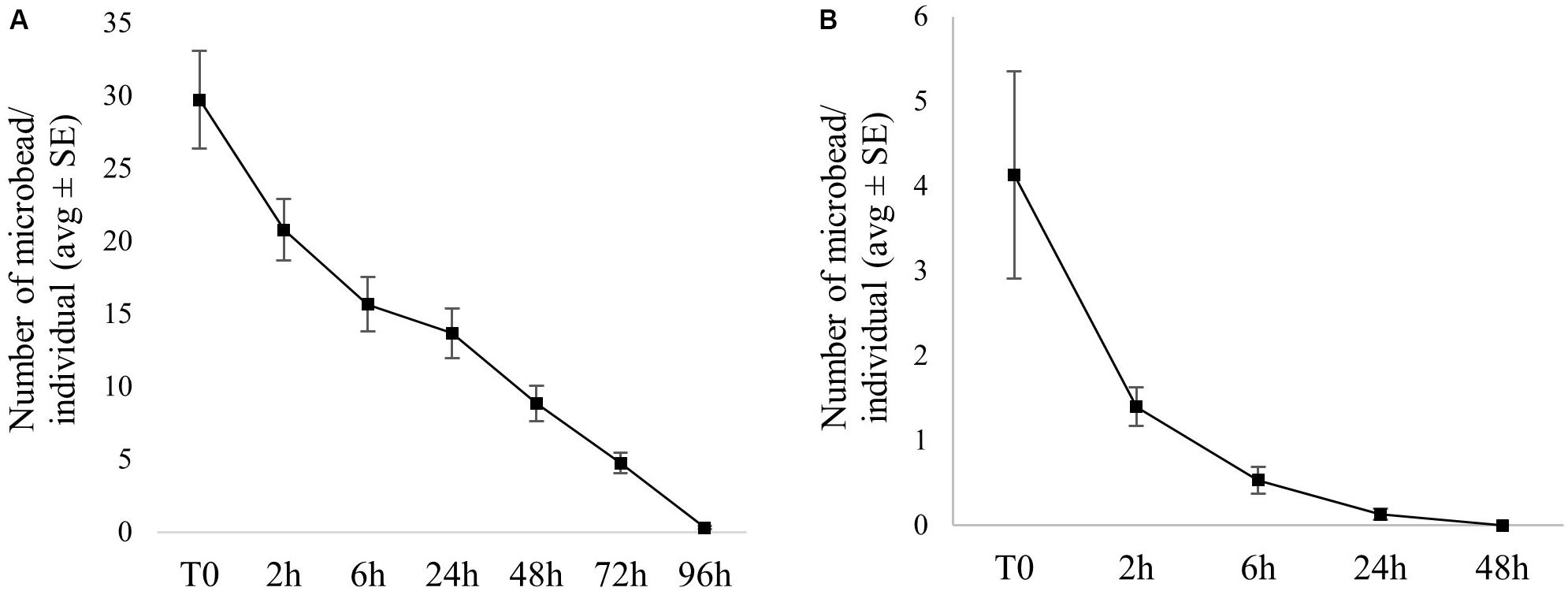
Figure 2. Microbead uptake (T0) and depuration over time in Artemia exposed (A) without food and (B) in the presence of food (average number/individual ± SE).
Following filtration of exposure water, 5,220 ± 765 (average ± SE) microbeads and 5,069 ± 64 (average ± SE) microfibers were recovered, indicating concentrations of approximately 52,200 beads/L and 50,690 fibers/L.
Exposure in the Presence of Food
No fiber ingestion was observed when particles were presented in the presence of food. Microbead ingestion was low, with 4 ± 1 part./individual (average ± SE), and 10% of individuals not having ingested any particle. The number of microbeads ingested per individual did not significantly differ between replicates. Microbead egestion was complete in 48 h for all the individuals, and more than 50% of the ingested microbeads were excreted in less than 2 h on average (see Figure 2B and Supplementary Table 1). Following filtration of exposure water (water column), 4,439 ± 178 (average ± SE) microbeads and 339 ± 53 (average ± SE) microfibers were recovered.
Fish Exposed via Water
In gills, microfibers were more abundant than microbeads: an average of 31 and 18 microfibers/individuals were observed for SW and FW fish, respectively, while only 18 and 0.2 microbeads/individuals were observed for SW and FW fish, respectively. Contrary to gills, very few particles were observed in the gut: samples containing fibers had a maximum of two fibers (1 and 0.6 fibers/individual on average for SW and FW fish, respectively), and only one sample contained microbeads (two beads present in one SW fish). In both FW and SW conditions, most particles were depurated in 2 h, and complete depuration was achieved in 48 h (Figure 3 and Supplementary Table 2). Group comparison based on particle shape indicates that in FW, a significantly higher number of fibers was found in both gills and gut immediately after exposure, compared to microbeads (p = 0.02, both in gills and gut). More specifically, microbeads were totally absent from gut samples and nearly totally absent from gills samples (only two FW fish were positive for the presence of beads in the gills). From 2 h of depuration, no significant difference between shapes was observed (Figures 3C,D). In SW, beads were nearly totally absent from gut samples (only one SW fish was positive for beads) but were found in gills samples (Figures 3A,B). However, the difference between shapes was not statistically significant in SW fish (p = 0.37 and 0.25 at T0 in gills and gut, respectively, and p = 0.06 in both gills and gut, at 2 h).
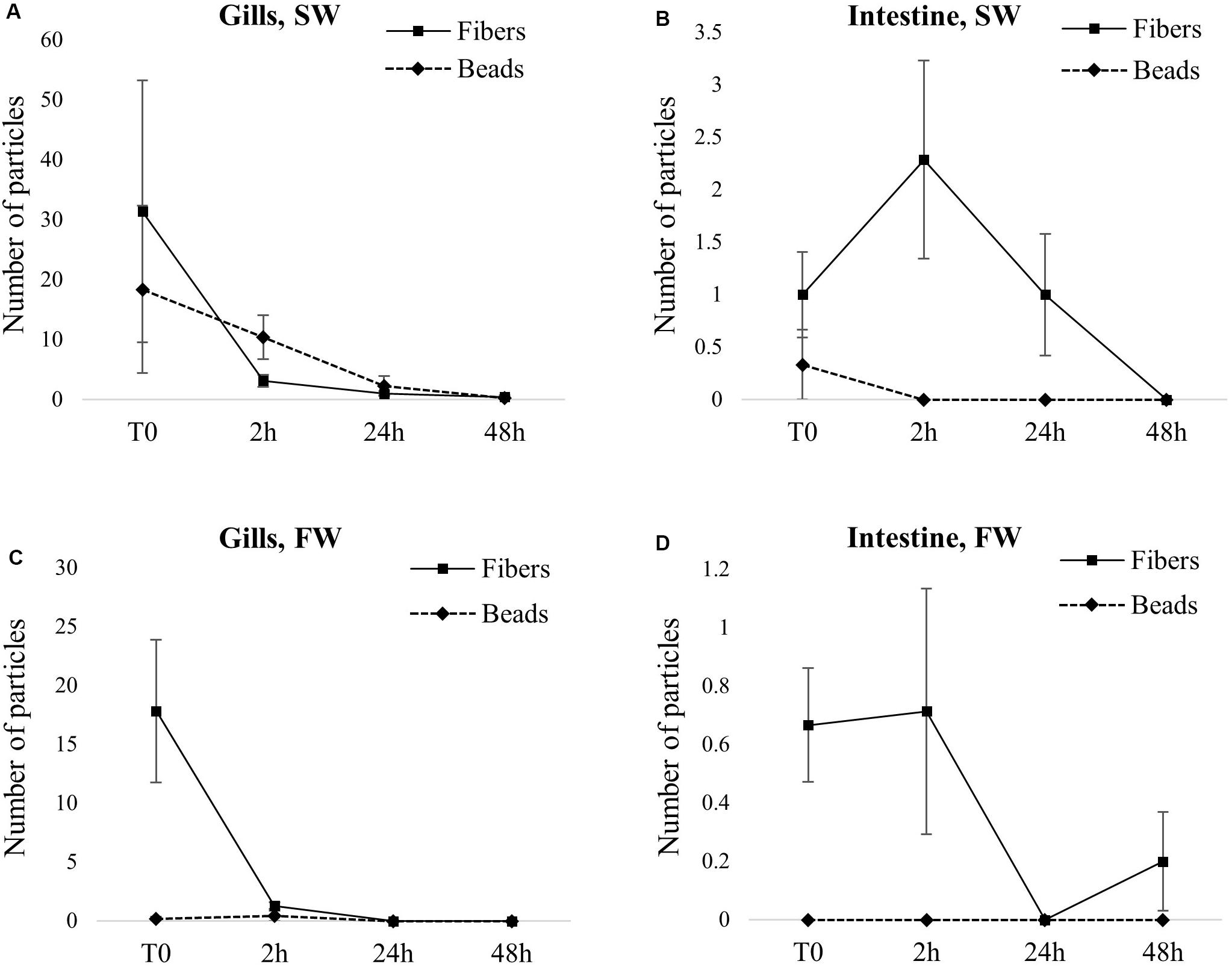
Figure 3. Particle uptake (T0) and depuration over time in fish exposed via water [seawater (SW) or freshwater (FW)]. Results are expressed as the average number of particles found in tissue ± SE. (A) gills from SW fish. (B) Intestine from SW fish. (C) Gills from FW fish. (D) Intestine from FW fish.
Group comparison based on fish organs (i.e., gills and gut) indicated significantly higher numbers of particles found in gills, compared to gut, both in FW and SW conditions and until 2 h of depuration (SW: p < 0.03 and p < 0.02 at 0 and 2 h of depuration, respectively; FW: p < 0.02 and p < 0.05 at 0 and 2 h of depuration, respectively).
No difference in the number of particle was observed when groups were compared based on water salinity (i.e. SW and FW).
Following filtration of exposure waters, 646 ± 43 (average ± SE) microbeads and 646 ± 23 (average ± SE) microfibers were recovered in SW conditions, and 373 ± 26 (average ± SE) microbeads and 486 ± 43 (average ± SE) microfibers in FW conditions. This indicates approximate concentrations of 43,033 part./L for both microbeads and microfibers in SW and 24,867 microbeads/L, and 32,367 microfibers/L in FW. Although these concentrations are below the theoretical concentration of 50,000 part./L, they confirm fish exposure to both microbeads and microfibers, in SW and FW.
Following processing and filtration of samples from the control groups, eight microfibers were observed in the case of one gut sample from an SW fish. No microbead or microfiber was observed in any other sample from control groups neither in SW nor in FW, gut or gills, and at any sampling time.
Fish Exposed via Trophic Chain
The method used to perform trophic exposures allowed us to quantify the number of ingested microbeads for each fish. Detailed numbers are presented in Supplementary Table 3. The presence of microbeads in fish gut is therefore expressed as the percentage of ingested particle. Similarly to water exposure, fast depuration was observed: more than 50% depuration was achieved within 2 h and complete depuration was observed in 48 h (Figure 4). Detailed numbers of microbeads recovered over time are presented in Supplementary Table 3. No microbead was observed in fish from the control group.
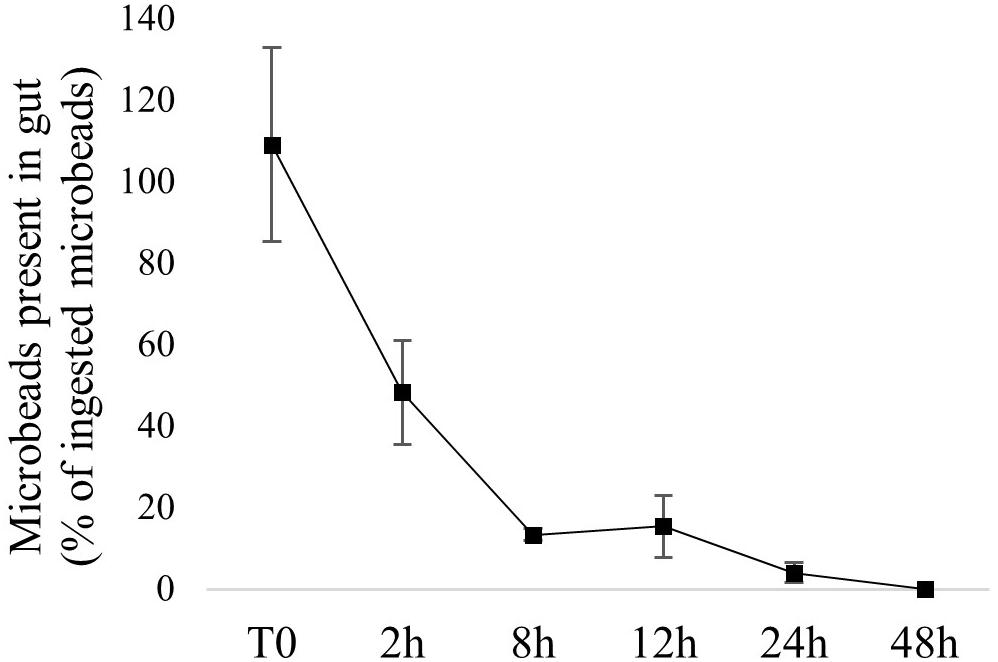
Figure 4. Microbead depuration over time in fish exposed via trophic chain. Values are numbers of microbeads recovered in fish gut, expressed as a percentage of ingested microbeads (average ± SE; n = 3).
Discussion
Particle Ingestion and Retention Time in Artemia
Artemia are filter-feeders that can feed on a wide range of particle sizes (Bergami et al., 2016): they continuously ingest particles present in the surrounding water. The very high particle concentration used for this study is not representative of environmental concentrations but was chosen to maximize interactions between particles and organisms. The microbead ingestion (30 particle/individual on average) observed in the absence of a food source is therefore not surprising and has previously been observed (Batel et al., 2016; Peixoto et al., 2019). The observed microbead retention time was also longer than normal retention time for Artemia (48 h, determined with food). Despite the high particle concentration, fiber ingestion was very low. Although microfiber diameter (12.5 μm) is much smaller than that in Artemia (∼1 cm), the fibers can be long enough to limit their ingestion. Other studies observed higher ingestion rates for microbeads than for fibers, by gammarids (Blarer and Burkhardt-Holm, 2016), shrimps (Gray and Weinstein, 2017), and daphnids (Ziajahromi et al., 2017). One study found higher ingestion rates for fibers, in Hyalella azteca (Au et al., 2015).
In the presence of food, no microfiber ingestion was observed and microbead ingestion was very limited (approximately four microbeads/individual), while food ingestion was fast. In the exposure water, visual observation indicated microfiber flocculation with food and sedimentation, which was further confirmed by particle quantification: a low proportion of fibers was left in the water column (<7%). However, the number of microfibers in suspension was still high enough to allow interaction with Artemia. The number of microbeads in the suspension was high (89% of nominal concentration) and similar to concentrations measured in the absence of food. Therefore, the limited particle ingestion by Artemia in the presence of food is not likely to be due to limited numbers of particles, and suggests instead selective feeding and avoidance of plastic microparticles. Complete microbead egestion was faster in the presence of food, corresponding to the normal food retention time (48 h). Similarly to our result, limited ingestion of plastic microparticles in presence of food has been observed in Artemia parthenogenetica (Wang et al., 2019). On the contrary, a similar study conducted with blue mussel showed that plastic microbead retention time increased in the presence of food (Chae and An, 2020), suggesting species-specific ingestion, and egestion patterns.
These results also highlight the importance of the experimental design when assessing microplastic ingestion and retention time. The total absence of food in natural environments seems unlikely, and very high microplastic concentrations are not expected, except in specific hotspots (Van Cauwenberghe et al., 2013). Our results show that microbeads can be ingested by Artemia and retained for a couple of days and are therefore likely to be transferred to organisms from upper trophic levels.
Microbead Trophic Transfer to Fish
Microbead egestion by fish exposed via trophic chain was fast, with more than 50% depuration achieved within 2 h and complete depuration was achieved in 48 h. This corresponds to stickleback’s normal gut retention time (estimated at 48 h) and suggests that plastic microbeads do not accumulate when transferred from contaminated preys. Similarly to our results, a short retention time of microplastic was previously observed in goldfish exposed via food (33 h), with no significant differences between microplastic and natural food (Grigorakis et al., 2017). The short microplastic retention time in fish, seen in light of field studies’ results, highlights an important feature of environmental exposure. Currently, most field studies report high proportions of fish positive for the presence of microplastics. Short microplastic retention time implies that the probability of fish ingesting microplastic shortly before sampling is high, suggesting that fish are continuously, or at least very frequently, exposed to microplastics in the environment.
Particle Interaction With Fish Exposed via Water
Particle quantification in exposure waters showed concentrations lower than nominal concentrations, especially in FW. This could be due to initial suspension preparation. However, the concentrations measured in Artemia exposure waters were very close to nominal concentrations, suggesting that our methods enable accurate preparation of both microbead and microfiber suspensions. Moreover, we performed pilot studies to ensure that particle suspension in water, ingestion by organism and processing with 10% KOH (cf. section Fish Samples Processing and Analysis) would not alter particles’ physical properties (e.g., color loss) and result in lowered recovery rates (see Supplementary Figure 1). It is therefore more likely that after 2 h of exposure, particles are not homogeneously distributed in the water column. Since the water samples were taken at mid-depth of the water column, it can be hypothesized that plastic microparticles tend to float (case of SW) or sink, potentially flocculating with fish feces (case of FW). The quantities of particles recovered from water samples indicate that fish were still exposed to high particle concentrations and that concentrations were similar for microbeads and microfibers.
However, significant differences between microbeads and microfibers recovered from fish samples were observed in FW. This difference was not significant in SW, but the important individual variations at T0 and the low p-values (0.06) at 2 h of depuration suggest that the non-significance could be due to a low statistical power. Eight microfibers were found in a gut sample from one control SW fish. This is by far the highest number of microfibers observed in all gut samples and suggests a contamination of the filter during sample processing rather than contamination of fish during exposure. Since this is the only case of control contamination, it was decided to not include it in the calculations, by removing it as background contamination for instance. The almost complete absence of microbeads in the gut in both SW and FW conditions suggests that fish could selectively avoid microbeads. Stickleback are capable of actively selecting their prey based on shape and prefer straight prey over globular ones (Ibrahim and Huntingford, 2010), which supports the hypothesis of fibers selection over beads in our experiment. However, prey selection is also based on other factors, such as size, color, and movement, and color has been shown to be the predominant one, followed by movement, shape, and size (Ibrahim and Huntingford, 2010). Especially, stickleback can perceive blue colors (Rick and Bakker, 2008) and could have therefore distinguished the microbeads and actively avoided them. In another study where fish were exposed to microplastics of different colors, authors observed that fish avoided colored particles, whereas they ingested black particles that looked more similar to their food (Ory et al., 2018). Although the blue color of microbeads may have resulted in their selective avoidance by fish, it is important to note that in environmental conditions, rapid biofouling of microplastics (Harrison et al., 2014) can alter their physical appearance, including color. Color-based selection of microparticles may therefore not be as relevant in environmental conditions.
The significantly higher numbers of particles found in gills, compared to gut, show that gills are the most exposed organ when fish are directly exposed via water. Therefore, gills should be considered a highly relevant organ when investigating microplastic exposure and effects in fish. Currently, the vast majority of field studies conducted on fish focus solely on gut. Based on our results, we recommend that future field studies include gills when assessing the presence of microplastics in fish. Similarly, when considering effect studies at the organ level following water exposure, data on gills are under-represented, compared to those of gut (Karami et al., 2016, 2017; Choi et al., 2018; Jin et al., 2018; Lei et al., 2018). More effect studies on gills are therefore necessary to fill this gap.
Finally, our results indicate that in our specific exposure conditions, water salinity (i.e. SW or FW) do not influence exposure to and retention time of particles, therefore invalidating our initial hypothesis. Although the present results are unambiguous and represent valuable data on the comparison of FW and SW systems, they should not be extrapolated to the whole FW and SW ecosystems, and complementary studies comprising different species would be needed.
Conclusion
Our results confirmed that ingestion and retention by organisms are different between microbeads and microfibers, suggesting particle selection based on size, shape, and/or color. Invertebrates and fish exhibited different patterns—Artemia ingesting large quantities of microbeads but very few microfibers, while stickleback ingested more microfibers than microbeads. For Artemia, selective feeding was also confirmed with decreased particle ingestion when exposed in the presence of food. Our results also show that microfiber trophic transfer from Artemia to predator species is not likely, contrarily to microbeads. In fish, particle ingestion directly via water is limited, and ingested particles, either directly from water or from contaminated prey, have a short gut retention time. On the contrary, gills are more susceptible to microparticle exposure, with higher numbers of particles- and longer retention times. This highlights the importance of gills in fish exposure and effect studies. We therefore recommend that both field and laboratory studies include gills in their experimental design. Finally, we initially hypothesized that fish exposure to particle would differ between FW and SW conditions, but our results invalidated this hypothesis.
Data Availability Statement
All datasets generated for this study are included in the article/Supplementary Material.
Ethics Statement
The animal study was reviewed and approved by Swedish Board of Agriculture (Jordbruks Verket). Animal husbandry and experiments were conducted in compliance with ethical practices from the Swedish Board of Agriculture (Ethical permit number #15986-2018).
Author Contributions
AB and BC conceived the study. AB and SH conducted the experiments. MT and MS provided the yarn samples and textile expertise. AB wrote the manuscript, which all authors approved before submission.
Funding
This research was supported by the Swedish Research Council FORMAS (2016-00895).
Conflict of Interest
The authors declare that the research was conducted in the absence of any commercial or financial relationships that could be construed as a potential conflict of interest.
The reviewer SR declared a past co-authorship with one of the authors BC to the handling Editor.
Acknowledgments
The authors are grateful to the reviewers for their thorough review of this work and their constructive comments which helped improve and clarify this manuscript.
Supplementary Material
The Supplementary Material for this article can be found online at: https://www.frontiersin.org/articles/10.3389/fenvs.2020.00083/full#supplementary-material
References
Au, S. Y., Bruce, T. F., Bridges, W. C., and Klaine, S. J. (2015). Responses of Hyalella azteca to acute and chronic microplastic exposures. Environ. Toxicol. Chem. 34, 2564–2572. doi: 10.1002/etc.3093
Au, S. Y., Lee, C. M., Weinstein, J. E., van den Hurk, P., and Klaine, S. J. (2017). Trophic transfer of microplastics in aquatic ecosystems: identifying critical research needs. Integr. Environ. Assess. Manag. 13, 505–509. doi: 10.1002/ieam.1907
Avio, C. G., Pittura, L., d’Errico, G., Abel, S., Amorello, S., Marino, G., et al. (2020). Distribution and characterization of microplastic particles and textile microfibers in Adriatic food webs: general insights for biomonitoring strategies. Environ. Pollut. 258:113766. doi: 10.1016/j.envpol.2019.113766
Batel, A., Linti, F., Scherer, M., Erdinger, L., and Braunbeck, T. (2016). Transfer of benzo[a]pyrene from microplastics to Artemia nauplii and further to zebrafish via a trophic food web experiment: CYP1A induction and visual tracking of persistent organic pollutants: trophic transfer of microplastics and associated POPs. Environ. Toxicol. Chem. 35, 1656–1666. doi: 10.1002/etc.3361
Bell, M. A., and Foster, S. A. (1994). The Evolutionary Biology of the Threespine Stickleback. New York, NY: Oxford University Press.
Bergami, E., Bocci, E., Vannuccini, M. L., Monopoli, M., Salvati, A., Dawson, K. A., et al. (2016). Nano-sized polystyrene affects feeding, behavior and physiology of brine shrimp Artemia franciscana larvae. Ecotoxicol. Environ. Saf. 123, 18–25. doi: 10.1016/j.ecoenv.2015.09.021
Blarer, P., and Burkhardt-Holm, P. (2016). Microplastics affect assimilation efficiency in the freshwater amphipod Gammarus fossarum. Environ. Sci. Pollut. Res. 23, 23522–23532. doi: 10.1007/s11356-016-7584-2
Bour, A., Avio, C. G., Gorbi, S., Regoli, F., and Hylland, K. (2018). Presence of microplastics in benthic and epibenthic organisms: influence of habitat, feeding mode and trophic level. Environ. Pollut. 243, 1217–1225. doi: 10.1016/j.envpol.2018.09.115
Burns, E. E., and Boxall, A. B. A. (2018). Microplastics in the aquatic environment: evidence for or against adverse impacts and major knowledge gaps: microplastics in the environment. Environ. Toxicol. Chem. 37, 2776–2796. doi: 10.1002/etc.4268
Cai, Y., Yang, T., Mitrano, D. M., Heuberger, M., Hufenus, R., and Nowack, B. (2020). Systematic study of microplastic fiber release from 12 different polyester textiles during washing. Environ. Sci. Technol. 54, 4847–4855. doi: 10.1021/acs.est.9b07395
Carney Almroth, B. M., Åström, L., Roslund, S., Petersson, H., Johansson, M., and Persson, N.-K. (2018). Quantifying shedding of synthetic fibers from textiles; a source of microplastics released into the environment. Environ. Sci. Pollut. Res. 25, 1191–1199. doi: 10.1007/s11356-017-0528-7
Chae, Y., and An, Y.-J. (2020). Effects of food presence on microplastic ingestion and egestion in Mytilus galloprovincialis. Chemosphere 240:124855. doi: 10.1016/j.chemosphere.2019.124855
Choi, J. S., Jung, Y.-J., Hong, N.-H., Hong, S. H., and Park, J.-W. (2018). Toxicological effects of irregularly shaped and spherical microplastics in a marine teleost, the sheepshead minnow (Cyprinodon variegatus). Mar. Pollut. Bull. 129, 231–240. doi: 10.1016/j.marpolbul.2018.02.039
Dehaut, A., Cassone, A.-L., Frère, L., Hermabessiere, L., Himber, C., Rinnert, E., et al. (2016). Microplastics in seafood: benchmark protocol for their extraction and characterization. Environ. Pollut. 215, 223–233. doi: 10.1016/j.envpol.2016.05.018
Desforges, J.-P. W., Galbraith, M., Dangerfield, N., and Ross, P. S. (2014). Widespread distribution of microplastics in subsurface seawater in the NE Pacific Ocean. Mar. Pollut. Bull. 79, 94–99. doi: 10.1016/j.marpolbul.2013.12.035
Eckert, R., Randall, R., and Augustine, G. (1988). Animal Physiology: Mechanisms and Adaptations. New York, NY: W. H. Freeman and Co.
Farrell, P., and Nelson, K. (2013). Trophic level transfer of microplastic: Mytilus edulis (L.) to Carcinus maenas (L.). Environ. Pollut. 177, 1–3. doi: 10.1016/j.envpol.2013.01.046
Gray, A. D., and Weinstein, J. E. (2017). Size- and shape-dependent effects of microplastic particles on adult daggerblade grass shrimp (Palaemonetes pugio): uptake and retention of microplastics in grass shrimp. Environ. Toxicol. Chem. 36, 3074–3080. doi: 10.1002/etc.3881
Grigorakis, S., Mason, S. A., and Drouillard, K. G. (2017). Determination of the gut retention of plastic microbeads and microfibers in goldfish (Carassius auratus). Chemosphere 169, 233–238. doi: 10.1016/j.chemosphere.2016.11.055
Halstead, J. E., Smith, J. A., Carter, E. A., Lay, P. A., and Johnston, E. L. (2018). Assessment tools for microplastics and natural fibres ingested by fish in an urbanised estuary. Environ. Pollut. 234, 552–561. doi: 10.1016/j.envpol.2017.11.085
Harrison, J. P., Schratzberger, M., Sapp, M., and Osborn, A. M. (2014). Rapid bacterial colonization of low-density polyethylene microplastics in coastal sediment microcosms. BMC Microbiol. 14:232. doi: 10.1186/s12866-014-0232-4
Henry, B., Laitala, K., and Klepp, I. G. (2019). Microfibres from apparel and home textiles: prospects for including microplastics in environmental sustainability assessment. Sci. Total Environ. 652, 483–494. doi: 10.1016/j.scitotenv.2018.10.166
Horton, A. A., Jürgens, M. D., Lahive, E., van Bodegom, P. M., and Vijver, M. G. (2018). The influence of exposure and physiology on microplastic ingestion by the freshwater fish Rutilus rutilus (roach) in the River Thames, UK. Environ. Pollut. 236, 188–194. doi: 10.1016/j.envpol.2018.01.044
Hu, L., Chernick, M., Lewis, A. M., Ferguson, P. L., and Hinton, D. E. (2020). Chronic microfiber exposure in adult Japanese medaka (Oryzias latipes). PLoS One 15:e0229962. doi: 10.1371/journal.pone.0229962
Ibrahim, A. A., and Huntingford, F. A. (2010). The Role of Visual Cues in Prey Selection in Three-spined Sticklebacks (Gasterosteus aculeatus). Ethology 81, 265–272. doi: 10.1111/j.1439-0310.1989.tb00772.x
Jemec, A., Horvat, P., Kunej, U., Bele, M., and Kržan, A. (2016). Uptake and effects of microplastic textile fibers on freshwater crustacean Daphnia magna. Environ. Pollut. 219, 201–209. doi: 10.1016/j.envpol.2016.10.037
Jin, Y., Xia, J., Pan, Z., Yang, J., Wang, W., and Fu, Z. (2018). Polystyrene microplastics induce microbiota dysbiosis and inflammation in the gut of adult zebrafish. Environ. Pollut. 235, 322–329. doi: 10.1016/j.envpol.2017.12.088
Jutfelt, F., Bresolin de Souza, K., Vuylsteke, A., and Sturve, J. (2013). Behavioural Disturbances in a Temperate Fish Exposed to Sustained High-CO2 Levels. PLoS One 8:e0065825. doi: 10.1371/journal.pone.0065825
Karami, A., Groman, D. B., Wilson, S. P., Ismail, P., and Neela, V. K. (2017). Biomarker responses in zebrafish (Danio rerio) larvae exposed to pristine low-density polyethylene fragments. Environ. Pollut. 223, 466–475. doi: 10.1016/j.envpol.2017.01.047
Karami, A., Romano, N., Galloway, T., and Hamzah, H. (2016). Virgin microplastics cause toxicity and modulate the impacts of phenanthrene on biomarker responses in African catfish (Clarias gariepinus). Environ. Res. 151, 58–70. doi: 10.1016/j.envres.2016.07.024
Koelmans, A. A. (2015). “Modeling the role of microplastics in bioaccumulation of organic chemicals to marine aquatic organisms. a critical review,” in Marine Anthropogenic Litter, eds M. Bergmann, L. Gutow, and M. Klages (Cham: Springer International Publishing), 309–324. doi: 10.1007/978-3-319-16510-3_11
Lei, L., Wu, S., Lu, S., Liu, M., Song, Y., Fu, Z., et al. (2018). Microplastic particles cause intestinal damage and other adverse effects in zebrafish Danio rerio and nematode Caenorhabditis elegans. Sci. Total Environ. 619–620, 1–8. doi: 10.1016/j.scitotenv.2017.11.103
Lusher, A. L., Burke, A., O’Connor, I., and Officer, R. (2014). Microplastic pollution in the Northeast Atlantic Ocean: validated and opportunistic sampling. Mar. Pollut. Bull. 88, 325–333. doi: 10.1016/j.marpolbul.2014.08.023
Lusher, A. L., Tirelli, V., O’Connor, I., and Officer, R. (2015). Microplastics in Arctic polar waters: the first reported values of particles in surface and sub-surface samples. Sci. Rep. 5:14947. doi: 10.1038/srep14947
Marchand, A., Porcher, J.-M., Turies, C., Chadili, E., Palluel, O., Baudoin, P., et al. (2017). Evaluation of chlorpyrifos effects, alone and combined with lipopolysaccharide stress, on DNA integrity and immune responses of the three-spined stickleback, Gasterosteus aculeatus. Ecotoxicol. Environ. Saf. 145, 333–339. doi: 10.1016/j.ecoenv.2017.07.025
Mathalon, A., and Hill, P. (2014). Microplastic fibers in the intertidal ecosystem surrounding Halifax Harbor, Nova Scotia. Mar. Pollut. Bull. 81, 69–79. doi: 10.1016/j.marpolbul.2014.02.018
Ory, N. C., Gallardo, C., Lenz, M., and Thiel, M. (2018). Capture, swallowing, and egestion of microplastics by a planktivorous juvenile fish. Environ. Pollut. 240, 566–573. doi: 10.1016/j.envpol.2018.04.093
Pazos, R. S., Maiztegui, T., Colautti, D. C., Paracampo, A. H., and Gómez, N. (2017). Microplastics in gut contents of coastal freshwater fish from Río de la Plata estuary. Mar. Pollut. Bull. 122, 85–90. doi: 10.1016/j.marpolbul.2017.06.007
Peixoto, D., Amorim, J., Pinheiro, C., Oliva-Teles, L., Varó, I., de Medeiros Rocha, R., et al. (2019). Uptake and effects of different concentrations of spherical polymer microparticles on Artemia franciscana. Ecotoxicol. Environ. Saf. 176, 211–218. doi: 10.1016/j.ecoenv.2019.03.100
Phuong, N. N., Zalouk-Vergnoux, A., Poirier, L., Kamari, A., Châtel, A., Mouneyrac, C., et al. (2016). Is there any consistency between the microplastics found in the field and those used in laboratory experiments? Environ. Pollut. 211, 111–123. doi: 10.1016/j.envpol.2015.12.035
Prendergast-Miller, M. T., Katsiamides, A., Abbass, M., Sturzenbaum, S. R., Thorpe, K. L., and Hodson, M. E. (2019). Polyester-derived microfibre impacts on the soil-dwelling earthworm Lumbricus terrestris. Environ. Pollut. 251, 453–459. doi: 10.1016/j.envpol.2019.05.037
Rick, I. P., and Bakker, T. C. (2008). Color signaling in conspicuous red sticklebacks: do ultraviolet signals surpass others? BMC Evol. Biol. 8:189. doi: 10.1186/1471-2148-8-189
Rochman, C. M. (2015). “The complex mixture, fate and toxicity of chemicals associated with plastic debris in the marine environment,” in Marine Anthropogenic Litter, eds M. Bergmann, L. Gutow, and M. Klages (Cham: Springer International Publishing), 117–140. doi: 10.1007/978-3-319-16510-3_5
Rochman, C. M. (2018). Microplastics research—from sink to source. Science 360, 28–29. doi: 10.1126/science.aar7734
Rochman, C. M., Parnis, J. M., Browne, M. A., Serrato, S., Reiner, E. J., Robson, M., et al. (2017). Direct and indirect effects of different types of microplastics on freshwater prey (Corbicula fluminea) and their predator (Acipenser transmontanus). PLoS One 12:e0187664. doi: 10.1371/journal.pone.0187664
Romanó de Orte, M., Clowez, S., and Caldeira, K. (2019). Response of bleached and symbiotic sea anemones to plastic microfiber exposure. Environ. Pollut. 249, 512–517. doi: 10.1016/j.envpol.2019.02.100
Sanchez, W., Bender, C., and Porcher, J.-M. (2014). Wild gudgeons (Gobio gobio) from French rivers are contaminated by microplastics: preliminary study and first evidence. Environ. Res. 128, 98–100. doi: 10.1016/j.envres.2013.11.004
Sanchez-Vidal, A., Thompson, R. C., Canals, M., and de Haan, W. P. (2018). The imprint of microfibres in southern European deep seas. PLoS One 13:e0207033. doi: 10.1371/journal.pone.0207033
Selonen, S., Dolar, A., Jemec Kokalj, A., Skalar, T., Parramon Dolcet, L., Hurley, R., et al. (2020). Exploring the impacts of plastics in soil – The effects of polyester textile fibers on soil invertebrates. Sci. Total Environ. 700:134451. doi: 10.1016/j.scitotenv.2019.134451
Setälä, O., Fleming-Lehtinen, V., and Lehtiniemi, M. (2014). Ingestion and transfer of microplastics in the planktonic food web. Environ. Pollut. 185, 77–83. doi: 10.1016/j.envpol.2013.10.013
Silva-Cavalcanti, J. S., Silva, J. D. B., de França, E. J., de Araújo, M. C. B., and Gusmão, F. (2017). Microplastics ingestion by a common tropical freshwater fishing resource. Environ. Pollut. 221, 218–226. doi: 10.1016/j.envpol.2016.11.068
Taylor, M. L., Gwinnett, C., Robinson, L. F., and Woodall, L. C. (2016). Plastic microfibre ingestion by deep-sea organisms. Sci. Rep. 6:33997. doi: 10.1038/srep33997
Tosetto, L., Williamson, J. E., and Brown, C. (2017). Trophic transfer of microplastics does not affect fish personality. Anim. Behav. 123, 159–167. doi: 10.1016/j.anbehav.2016.10.035
Van Cauwenberghe, L., Vanreusel, A., Mees, J., and Janssen, C. R. (2013). Microplastic pollution in deep-sea sediments. Environ. Pollut. 182, 495–499. doi: 10.1016/j.envpol.2013.08.013
Wang, Y., Mao, Z., Zhang, M., Ding, G., Sun, J., Du, M., et al. (2019). The uptake and elimination of polystyrene microplastics by the brine shrimp, Artemia parthenogenetica, and its impact on its feeding behavior and intestinal histology. Chemosphere 234, 123–131. doi: 10.1016/j.chemosphere.2019.05.267
Watts, A. J. R., Urbina, M. A., Corr, S., Lewis, C., and Galloway, T. S. (2015). Ingestion of plastic microfibers by the crab carcinus maenas and its effect on food consumption and energy balance. Environ. Sci. Technol. 49, 14597–14604. doi: 10.1021/acs.est.5b04026
Welden, N. A. C., and Cowie, P. R. (2016). Long-term microplastic retention causes reduced body condition in the langoustine, Nephrops norvegicus. Environ. Pollut. 218, 895–900. doi: 10.1016/j.envpol.2016.08.020
Woodall, L. C., Sanchez-Vidal, A., Canals, M., Paterson, G. L. J., Coppock, R., Sleight, V., et al. (2014). The deep sea is a major sink for microplastic debris. R. Soc. Open Sci. 1, 140317–140317. doi: 10.1098/rsos.140317
Zhao, S., Zhu, L., and Li, D. (2015). Microplastic in three urban estuaries. China Environ. Pollut. 206, 597–604. doi: 10.1016/j.envpol.2015.08.027
Keywords: microplastic, fish, invertebrates, ingestion, depuration, trophic transfer, ecotoxicity
Citation: Bour A, Hossain S, Taylor M, Sumner M and Carney Almroth B (2020) Synthetic Microfiber and Microbead Exposure and Retention Time in Model Aquatic Species Under Different Exposure Scenarios. Front. Environ. Sci. 8:83. doi: 10.3389/fenvs.2020.00083
Received: 23 January 2020; Accepted: 26 May 2020;
Published: 26 June 2020.
Edited by:
Denise M. Mitrano, Swiss Federal Institute of Aquatic Science and Technology, SwitzerlandReviewed by:
Alice Horton, University of Southampton, United KingdomSinja Rist, Technical University of Denmark, Denmark
Gerardo Pulido-Reyes, Swiss Federal Institute of Aquatic Science and Technology, Switzerland
Copyright © 2020 Bour, Hossain, Taylor, Sumner and Carney Almroth. This is an open-access article distributed under the terms of the Creative Commons Attribution License (CC BY). The use, distribution or reproduction in other forums is permitted, provided the original author(s) and the copyright owner(s) are credited and that the original publication in this journal is cited, in accordance with accepted academic practice. No use, distribution or reproduction is permitted which does not comply with these terms.
*Correspondence: Agathe Bour, YWdhdGhlLmJvdXJAYmlvZW52Lmd1LnNl