- 1Department of Environmental Health Science and Technology, Jimma University, Jimma, Ethiopia
- 2Laboratory of Aquatic Ecology, Evolution and Conservation, KU Leuven, Leuven, Belgium
- 3Department of Civil & Environmental Engineering, University of Connecticut, Storrs, CT, United States
- 4Department of Animal Sciences and Aquatic Ecology, Ghent University, Ghent, Belgium
- 5Provincial Centre of Environmental Research, Ghent, Belgium
Although wetlands in temperate regions have been studied for their pollutant remediation potential, the sediment and nutrient retention capacity of natural wetlands in tropical environments remains understudied. In this study, a mass balance approach was used to estimate the amount of sediment and nutrients retained at 40 different study sites located in four natural riverine wetlands in Southwest Ethiopia. Awetu and Boye wetlands retained a substantial amount of total suspended solids (TSS) and nutrients whereas Kofe and Kito wetlands had a net release. Overall, the amount of TSS retained by the four wetlands was estimated to be 6266.5 kg/ha/day. On the other hand, the net retention of Total Organic Nitrogen (TON) and Total Phosphorous (TP) was estimated to be 37.6 kg/ha/day and 40.9 kg/ha/day, respectively. A stepwise multiple regression analysis revealed that anthropogenic activities such as farming, grazing, waste dumping and clay mining were the main variables that negatively affected the retention of TSS and nutrients. Farming alone explained 58% of the variation in TSS retention and cattle grazing explained 25% of the variation in TON retention. Therefore, watershed management interventions considering the mitigation of anthropogenic activities could be considered to reduce sediment and nutrient input and release.
Introduction
Wetlands provide many ecosystem services such as habitat for plants and animals including endangered species, products (such as fish, reed, timber, fuel, wood, and medicines), mitigation of floods, recharging of aquifers and an improvement in water quality (Millennium Ecosystem Assessment, 2005; Mateos et al., 2009). Water quality functions of wetlands are a composite of many different biogeochemical and mechanical processes, which act collectively to alter and usually improve the quality of surface waters (Mateos et al., 2009). Biogeochemical processes that occur within wetlands can effectively remove a variety of pollutants from the water column (Knox et al., 2008). These processes include microbial transformation to gaseous forms, plant uptake of nutrients, and microbial degradation of pesticides and other organic compounds and sedimentation (Woltemade, 2000; Jordan et al., 2003; Fisher and Acreman, 2004; Knox et al., 2008).
Wetlands can serve both as sink and sources for contaminants (Kadlec and Reddy, 2001). The pollutant retention capacity depends on the structure and functioning of the wetland, and can also be influenced by hydrologic and temperature regimes (Kadlec and Reddy, 2001; Kadlec, 2009). In tropical climates, humidity is high year-round, although there may be a distinct wet and dry season. In addition, ambient temperature does not show substantial temporal variation as in temperate climates, which can impact plant growth and hence also pollutant retention capacity.
Wetland ecosystems are effective sediment traps, generally retaining more suspended sediments than they release (Christopher and David, 2004; Sileshi et al., 2020). Sediment deposition in wetlands is an important mechanism to improve water quality as sediments retain nutrients and toxic substances through sorption processes (Cooper et al., 2000; Noe and Hupp, 2009). The retention of suspended solids in wetlands is controlled by particle size, hydrologic regimes, flow velocity, wetland morphometry and water residence time (Verstraeten et al., 2006). Plants in wetlands can increase the hydraulic resistance and decrease flow velocity, which enhances settling and deposition of suspended solids (Paudel et al., 2013). In addition, aquatic plants retain nutrients through vegetative uptake, create root channels that increase infiltration capacity, provide a large surface area for microbial growth, and transport oxygen to anaerobic layers (Schoonover et al., 2005).
Wetlands can act as sinks and converters of nitrogenous compounds by various mechanisms (Keenan and Lowe, 2001; Jordan et al., 2003). These mechanisms include denitrification, assimilation, and retention by vegetation, converting nitrates to ammonia and organic nitrogen (Jordan et al., 2003). Vegetation plays a crucial role as it affects denitrification and nitrification by influencing oxygen concentrations in wetland substrates within the rhizosphere (Paudel et al., 2013). Phosphorous retention by wetlands is driven by a combination of chemical, biological and physical processes (Reddy et al., 1999). These processes are regulated by vegetation, periphyton, plant litter and detritus accumulation, flow velocity, water depth, hydraulic retention time and hydrologic fluctuations (Jordan et al., 2003; McJannet, 2007).
The loss and degradation of wetlands is a world-wide phenomenon (Russi et al., 2013) and undermines their pollutant mitigation potential (e.g., Mironga, 2005). The major direct drivers of wetland degradation and wetland loss include intensive agriculture, discharge of untreated wastewater, overgrazing and deforestation (Millennium Ecosystem Assessment, 2005). The use of fertilizers to enhance agricultural production often leads to eutrophication of surface waters (Crumpton, 2001; Zhenlou et al., 2002). Moreover, intense agriculture and deforestation can cause runoff and landslides that both increase the concentration of sediments in rivers and wetlands (Broothaerts et al., 2012). In Ethiopia, the occurrences of landslides are facilitated by steep slopes, extreme rainfall events, high human population pressure and severe deforestation (Broothaerts et al., 2012). The resulting increase in sediment loads results in enhanced sedimentation in reservoirs and dams, which reduces their water storage capacity (Devi et al., 2008; Adwubi et al., 2009). Assessments estimated that the volume of the reservoir of the Gilgel Gibe I hydroelectric dam, situated in southwest Ethiopia, will be reduced by half within 12 years (Devi et al., 2008). Although the reservoir was expected to serve for at least 70 years, there is a risk that the reservoir will be completely filled with sediments and characterized by highly eutrophic conditions 24 years after its construction (Devi et al., 2008).
Although constructed wetlands in temperate regions have been extensively studied and are generally considered as effective systems for sediment and nutrient retention, the retention efficiency of natural wetlands in tropical environments is considerably less investigated (McJannet, 2007). The objective of this study was to determine the total suspend solids (TSS) and nutrient retention capacity of natural riverine wetlands in Southwest Ethiopia, and to identify the effect of anthropogenic stressors on this retention capacity. Such information provides pivotal information for the development of sustainable wetland conservation programs in Ethiopia, where wetlands are important biodiversity hotspots and natural resources for food security and rural livelihood, but at the same time are highly threatened by unregulated exploitation and a lack of management.
Materials and Methods
Study Area
This study was conducted in the Awetu sub-catchment, part of the Gilgel Gibe I watershed, situated in Southwest Ethiopia and lying between latitudes 7°37′N and 7°53′N and longitudes 36°46′E and 37°43′E (Figure 1). The total area of the sub-catchment is about 500 km2. Elevation of this sub-catchment area ranges between 1,700 and 2,610 ma.s.l. The mean annual temperature ranges between 15°C and 22°C, and the mean annual precipitation ranges between 1500 mm and 2300 mm, with maximum rainfall from June till early September and minimum precipitation between December and January (National Meteorological Agency, 2013). Four riverine wetlands: Awetu (AW), Boye (BO), Kito (Kt) and Kofe (Kf) were investigated in this study. These wetlands varying in size ranging from 12 to 110 hectares. Streams flowing through these wetlands had an estimated mean discharge of 10.55 m3/sec, contributed to about 25% to the flow of the Gilgel Gibe River, one of the tributary of the Gilgel Gibe I reservoir situated 60 km downstream of the studied wetlands. The major threats to these wetlands include disposal of domestic sewage, drainage, farming, clay mining, removal of riparian vegetation and intensive livestock grazing. Awetu, Boye and some parts of Kito wetlands received untreated wastewater generated by the more than 200,000 inhabitants of Jimma town. Clay mining, drainage, Eucalyptus plantation vegetation clearance and filling were the common practices in Kofe and Kito wetlands (Table 1).
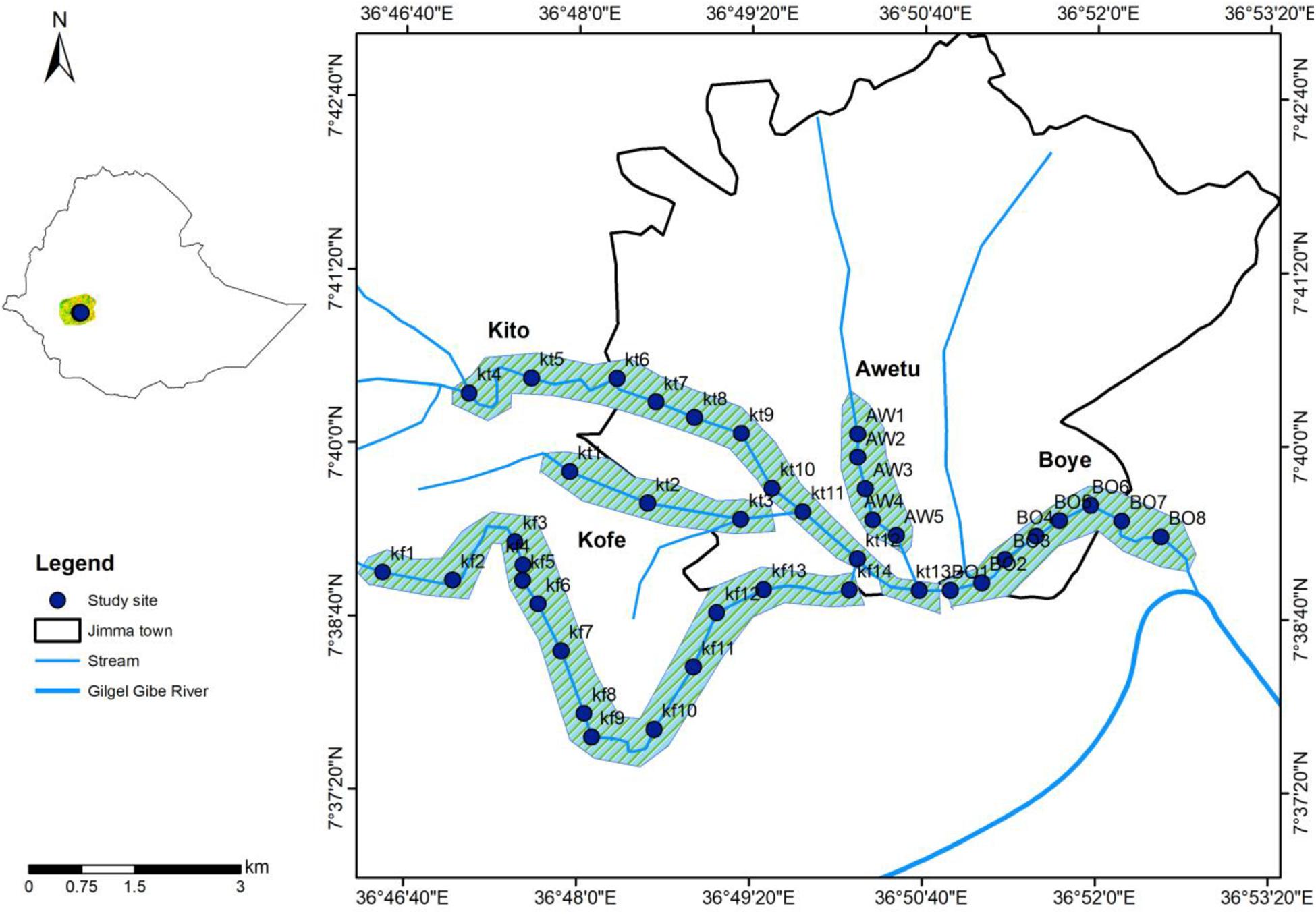
Figure 1. Map of wetland sampling sites in the neighborhoods of Jimma, Southwest Ethiopia (Kf = Kofe, Kt = Kito, AW = Awetu, BO = Boye).
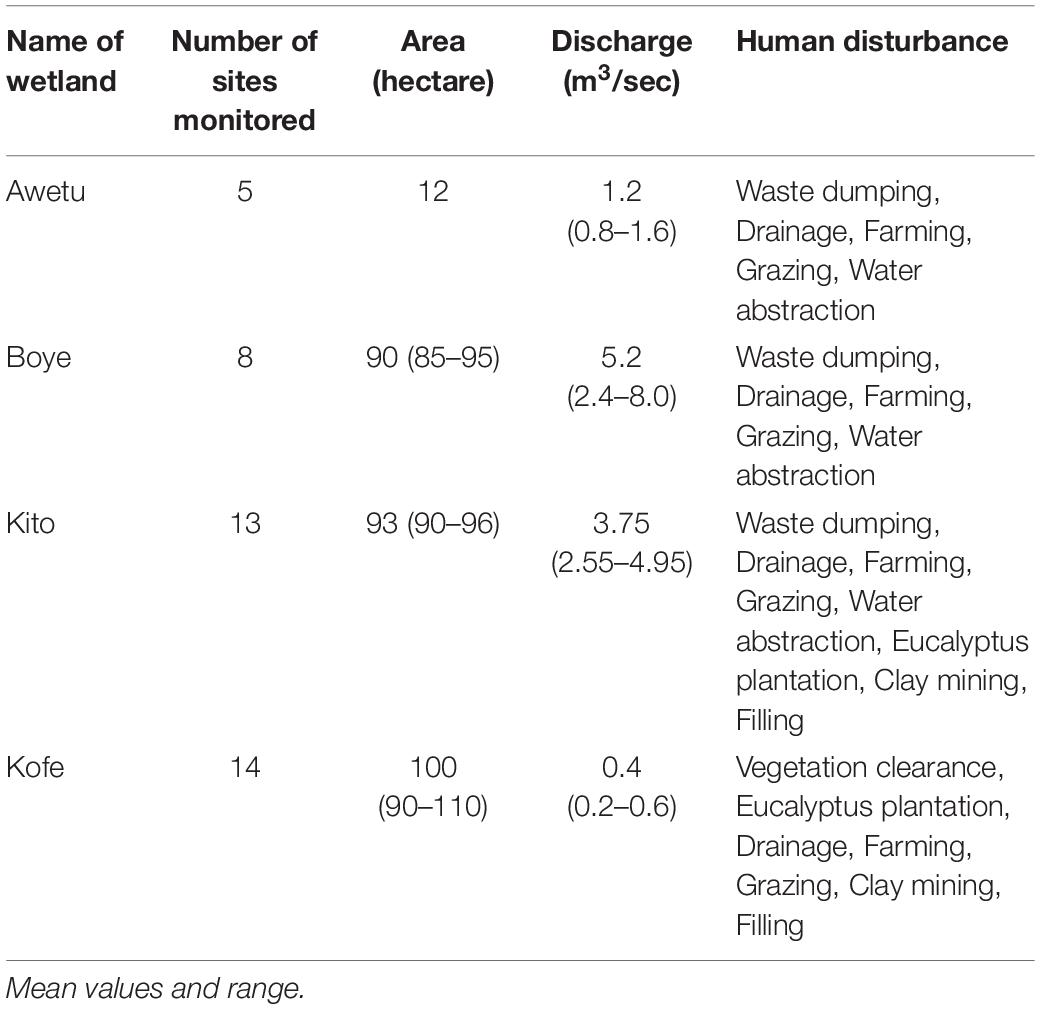
Table 1. Characteristics of the study wetlands located in the Gilgel Gibe I watershed, southwest Ethiopia.
Riverine wetlands in Southwest Ethiopia have been extensively modified during the last years by human activities (Mereta et al., 2013; De Troyer et al., 2016; Chawaka et al., 2018). The major threats from human activities in and around these wetlands include disposal of domestic sewage, drainage, farming, clay mining, removal of riparian vegetation and intensive livestock grazing (Mereta et al., 2013). In addition, river incisions and back erosions as a result of heavy rainfall, steep slopes and deforestation have contributed to landslides in the catchment (Broothaerts et al., 2012). This has led to extensive erosion from the upland areas and to increases in the sediment load in the receiving rivers and siltation problems in the Gilgel Gibe I reservoir, which may interfere with its hydroelectric generating capacity and the provision of other ecosystem services.
Data Collection
A total of 40 sites were selected in four wetlands along a gradient of anthropogenic impacts including both nearly non-impacted and heavily disturbed sites (e.g., presence of point source pollution, eutrophication, hydrological modification, etc.), similar to a previous study conducted by Mereta et al. (2013). The number of sampling sites was evenly distributed among the wetlands depending on their size (Figure 1), with the smallest wetlands having a lower number of sampling sites. Sites up- and downstream of river confluences were included, since this allowed us to assess the impact of the confluence on the receiving wetland or stream. Wetland sampling sites were monitored twice a year: once during the dry (February and March) and once during the wet season (i.e., after the end of rainy season October to November) in 2011. Awetu wetland was sampled only during the wet season of 2011. In total, 75 samples were collected. Geographic coordinate readings were recorded for all sampling sites using a hand-held global positioning system unit (GPS) (Garmin GPS 60, Garmin international Inc., and Olathe, Kansas, United States). Coordinate readings were integrated into a GIS database using Arc MAP 10 GIS software. All digital data in the GIS were displayed in the World Geodetic System (WGS) 1984 Coordinate system.
Habitat Sampling and Classification
Habitat characteristics of the surrounding area were assessed at each sampling station using the USEPA wetland habitat assessment protocol (Baldwin et al., 2005). The degree of hydrological modifications (drainage, ditching and filling), habitat alteration (tree removal, tree plantation and grazing) and land use patterns such as waste dumping, clay mining, and farming were assessed during sampling. Draining refer to water-level drawdown. It is a very common practice in the study area mainly for the cultivation of the dry season maize (Zea mays). Filling refers to placement of materials in the wetlands for conversion to other land-uses such as cultivation, house construction and so on.
Land use, habitat alteration and hydrological modifications were quantified based on their intensity in the studied wetlands according to Mereta et al. (2013). A score of 1 was assigned to no or minimal disturbance, 2 to moderate and 3 to high disturbance (Supplementary Material S1). The final disturbance score was then computed by summing nine disturbance types generating a disturbance score ranging from 9 to 27. This score was then divided into five classes: 9–11 = very low, 12–15 = low, 16–19 = moderate, 20–23 = high, and 24–27 = very high.
Climate data of the year 2011 was used for this study. Daily weather data of the surrounding area was collected from Jimma meteorological station situated near the study wetlands, about 6 km from the farthest wetland site.
Water Sampling and Analysis
Water samples were taken 20 cm below the surface in the water column to avoid scum. A 500 ml sample of unfiltered water was collected for analysis of total organic nitrogen (TON) and total phosphorous (TP). Unfiltered water samples were kept cool and dark during transportation to the laboratory of Environmental Health Science and Technology, Jimma University, for further analysis. Total phosphorus samples were first digested in a block digester using ammonium persulfate and a sulfuric acid reagent (APHA et al., 1995). Total phosphorous was analyzed using the stannous chloride method (APHA et al., 1995). Samples of TON were also digested and measured with photometric kits (Hach Lange) using a Hach DR5000 spectrophotometer.
For total suspended solid (TSS) determination, a known volume of water was filtered through a pre-dried (105°C; 12 h) and pre-weighed Whatman Glass Microfiber filter (GF/C-filters, diameter 47 mm and pore size 1.2 μm). After sampling, the filters were dried in an oven at 105°C for 12 h, cooled in desiccators to balance temperature, and weighed. The TSS concentration was calculated as the difference between the weight of the filter plus the dried residue and the weight of the filter; the value obtained from this difference was divided by the sampled volume. The dried residue was combusted at 550°C for 20 min in a muffle furnace to determine the volatile suspended solids (VSS) (organic fraction). The concentration of volatile suspended solids was calculated as the difference between the weight of the recipient plus the weight of the dried residue before combustion and the weight of the recipient and this difference was divided by the sampled volume. The concentration of fixed suspended solids was calculated as the difference between the total suspended solids and the volatile suspended solids.
Measuring Flow Velocity and Discharge
The volume of water inflow and outflow of the wetland was calculated by multiplying the area of water in the channel cross section by the average flow velocity of the water in that cross section. First, the channel cross section was divided in 5 to 10 subsections depending on the channel size. In each subsection, the area was calculated by measuring the width and depth of the subsection and by multiplying these values. Average flow velocity was computed from three repeated measurement at each cross section using a flow meter (BFM001channel flow meter, Valeport, United Kingdom). The discharge in each subsection was calculated by multiplying the subsection area by the measured average flow velocity. The total discharge was then calculated by summing the discharge of each subsection.
Loading and Flux Calculation
A nutrient and sediment loading rate of the wetland was calculated by multiplying the measured concentrations of nutrients and sediments, respectively, by the instantaneous discharge. The loading rates were corrected for water loss through evaporation and water input from precipitation.
Fluxes (surface loadings) were then calculated by dividing loading rate by wetland surface area (hectare).
Retention rates are typically calculated by subtracting outputs from inputs. This yields positive values when pollutants are retained in the wetland and negative values when pollutants are released. Hence, negative retention refers to release. In this case wetlands act as a source of pollutants.
Data Analysis
Nutrients and TSS data were log transformed [log(x + 1)] prior to analysis to meet normality assumptions. A Principal Component Analysis (PCA) was used to reduce the dimensions of human disturbance by creating a latent variable based on the PCA coordinates. Principal components were computed using the Oblimin rotation method with a Kaiser Normalization. The components were extracted based on eigenvalues greater than 1. Afterward, a stepwise multiple regression analysis was conducted by regressing the component scores computed by the PCA in order to identify which set of disturbance factors best explained the variation in nutrient and TSS retention. The concentration of total suspended solids was the response variable, whereas the habitat disturbances were the explaining variables. In case of nutrients (TON and TP), the response variable was composed of all individual nutrient measurements, whereas the habitat disturbances were the explanatory variables. We used a two-way ANOVA to select the most parsimonious model. The goodness of fit of each regression model for the data was evaluated based on the F-test and adjusted R-squared value.
Box- and Whisker plots were used to visualize the retention of TSS and nutrients at different levels of disturbance in the different wetlands. A non-parametric Kruskal-Wallis test was used to determine whether there were significant differences in the retention of TSS and nutrient concentrations between different levels of disturbance within the wetlands. We used a Wilcoxon rank-sum test to explore differences in retention between the dry and wet season.
Principal Component Analysis (PCA) and multiple regression were performed using Spss Inc (2007) (version 16) (SPSS Inc., Chicago, IL), whereas ANOVA analyses were done with STATISTICA 7.0 (Statsoft Inc, 2004). P-values < 0.05 were considered significant.
Results
Total Suspended Solids Concentration and Retention
From Kf1 to Kf14, the TSS concentration increased from 15 mg/l to 26 mg/l during the wet season and decreased from 37 mg/l to 26 mg/l during the dry season (Figures 2A,B). In kofe wetland the TSS concentration ranges from 4.5 mg/l to 39 mg/l. The net TSS release of Kofe wetland was 221 and 94 kg/ha/day for the wet and dry season, respectively (Table 2). The ratio between volatile suspended solids (VSS) and total suspended solids (TSS) in this wetland ranged from 0.5 to 0.6. The net TSS release during the wet season was significantly higher than during the dry season (p = 0.002).
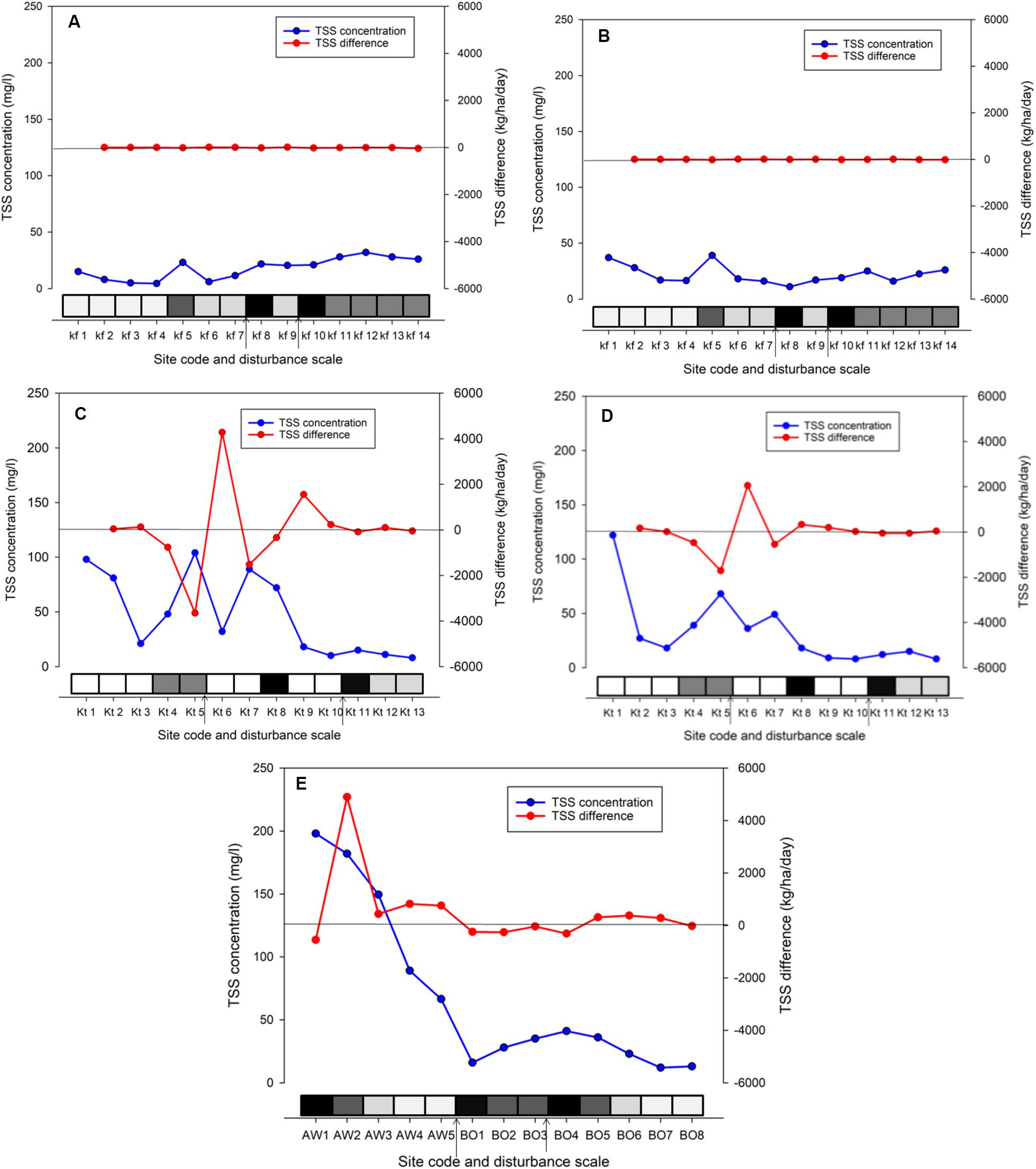
Figure 2. Total Suspended Solids (TSS) concentration and difference in Kofe (A), Kito (C) and Awetu-Boye (E) wetlands during wet season and Kofe (B) and Kito (D) during the dry season. Disturbance condition along the wetland ranged from very low (white) to very high (black). A black arrow indicates a site where a tributary enters into the main stream.
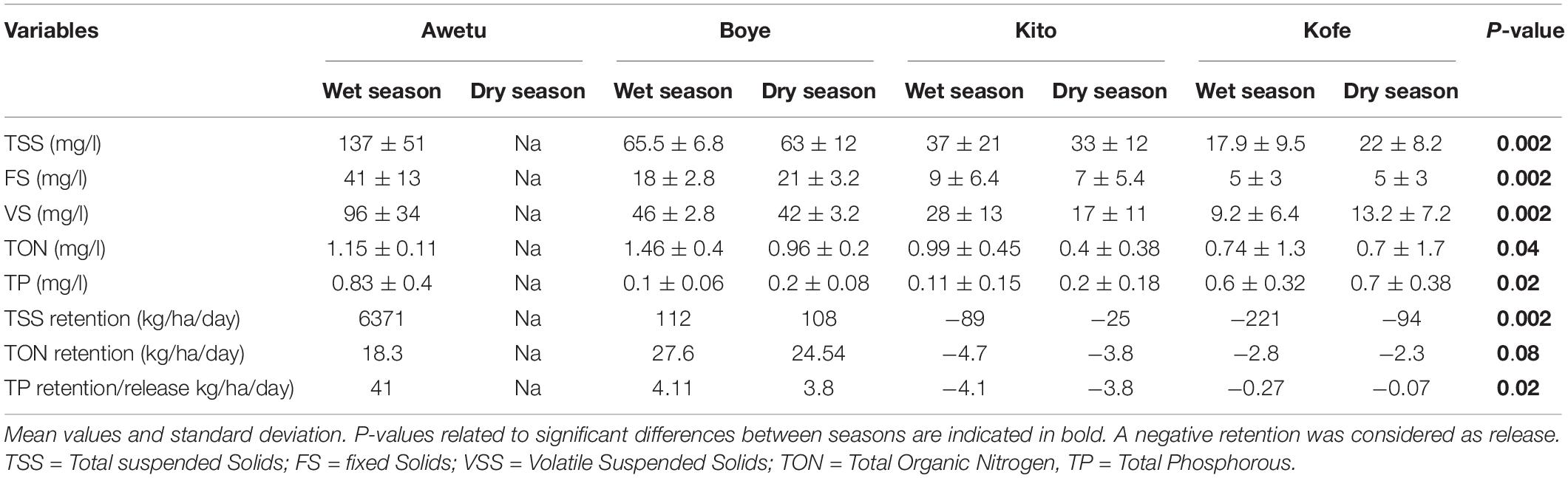
Table 2. Sediment and nutrients retention/release capacity of wetlands in the Gilgel Gibe I watershed.
The highest concentration of TSS was measured at Kt1, 122 mg/l during wet season and at kt5, 104 mg/l during the dry season (Figures 2C,D). Whereas the net TSS release for Kito wetland was 89 and 25 kg/ha/day for the wet and dry season, respectively (Table 2). The ratio between volatile suspended solids (VSS) and total suspended solids (TSS) in this wetland ranged from 0.5 to 0.7. The net release of TSS was significantly higher during the wet season than during the dry season (p = 0.002).
The highest concentration of TSS was recorded in the first upstream site location (AW1) 198 mg/l (Figure 2E). The net TSS retention in Awetu wetland was 6371 kg/ha/day. Kito stream, having an average flow rate of 3.75 m3/s and an average TSS concentration of 8 mg/l, joins the outflow of Awetu below AW5 and flows through Boye wetland. The first upstream sampling point of Boye wetland (BO1) had a measured TSS concentration of 16 mg/l. The highest concentration of TSS (41 mg/l) was measured at BO4, after the entrance of Becho-Bore stream having a flow rate of 0.5 m3/s and a TSS concentration of 88 mg/l. The net TSS retention in Boye wetland was 112 and 108 kg/ha/day during wet and dry season, respectively (Table 2). The ratio between volatile suspended solids (VSS) and total suspended solids (TSS) in this wetland ranged from 0.7 to 0.8.
Nutrient Concentration and Retention
The highest concentration of Total Organic Nitrogen (TON) (5.64 mg/l), was measured at Kf8 during the dry season (Figures 3A,B). The net release of TON in Kofe wetland was 2.8 kg/ha/day and 2.3 kg/ha/day for wet and dry season, respectively (Table 2). Likewise, the highest concentration of Total phosphorous (0.46 mg/l) was recorded at Kf8 during the dry season (Figures 4A,B). The net release of TP in Kofe wetland was 0.27 kg/ha/day and 0.07 kg/ha/day for wet and dry season, respectively. The net input and release of TP was significantly higher during the wet season than during the dry season (p = 0.02) (Table 1).
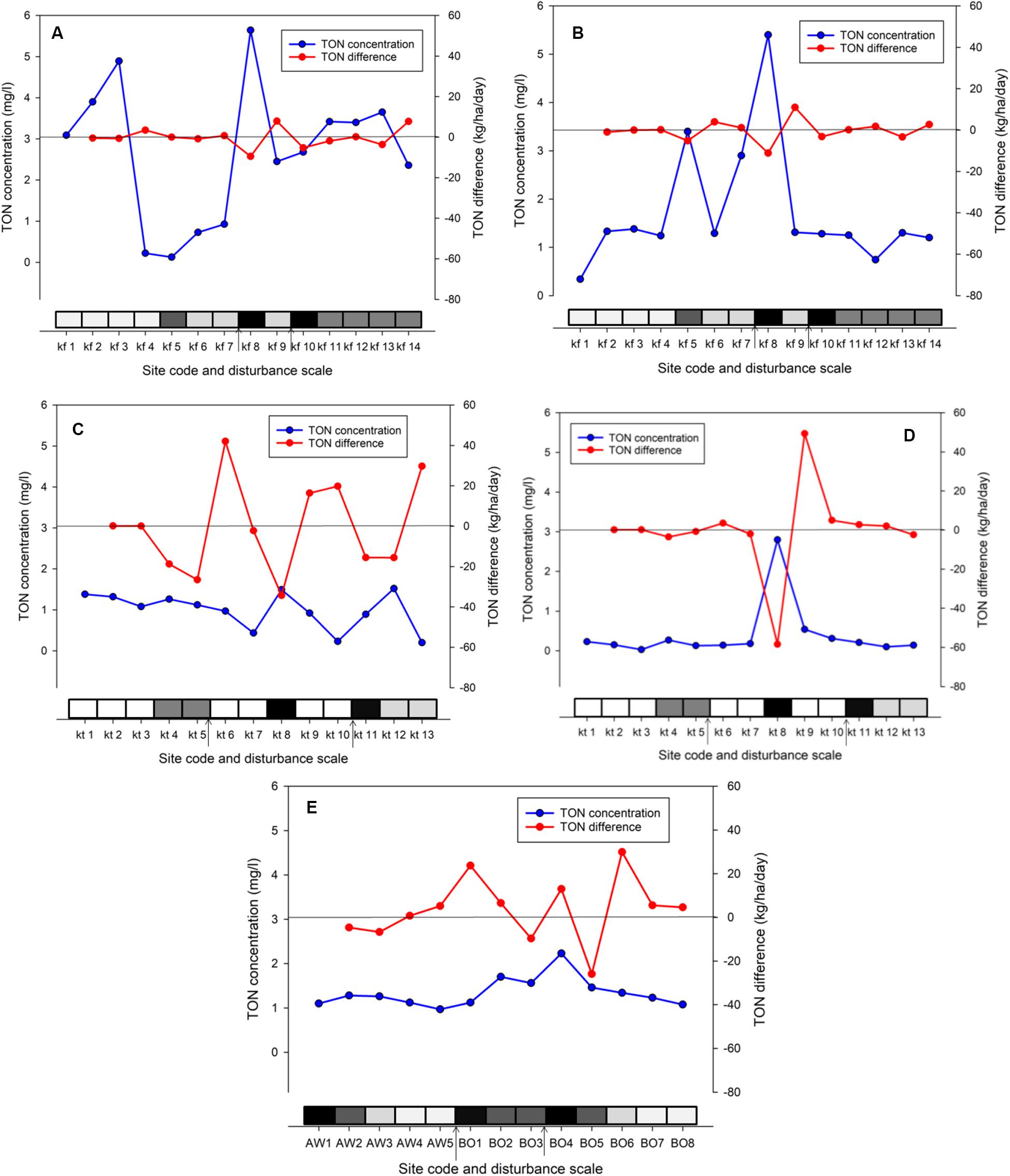
Figure 3. Total Organic Nitrogen (TON) concentration and difference in Kofe (A), Kito (C) and Awetu-Boye (E) wetlands during wet season and Kofe (B) and Kito (D) during the dry season. Disturbance condition along the wetland ranged from very low (white) to very high (black). A black arrow indicates a site where a tributary enters into the main stream.
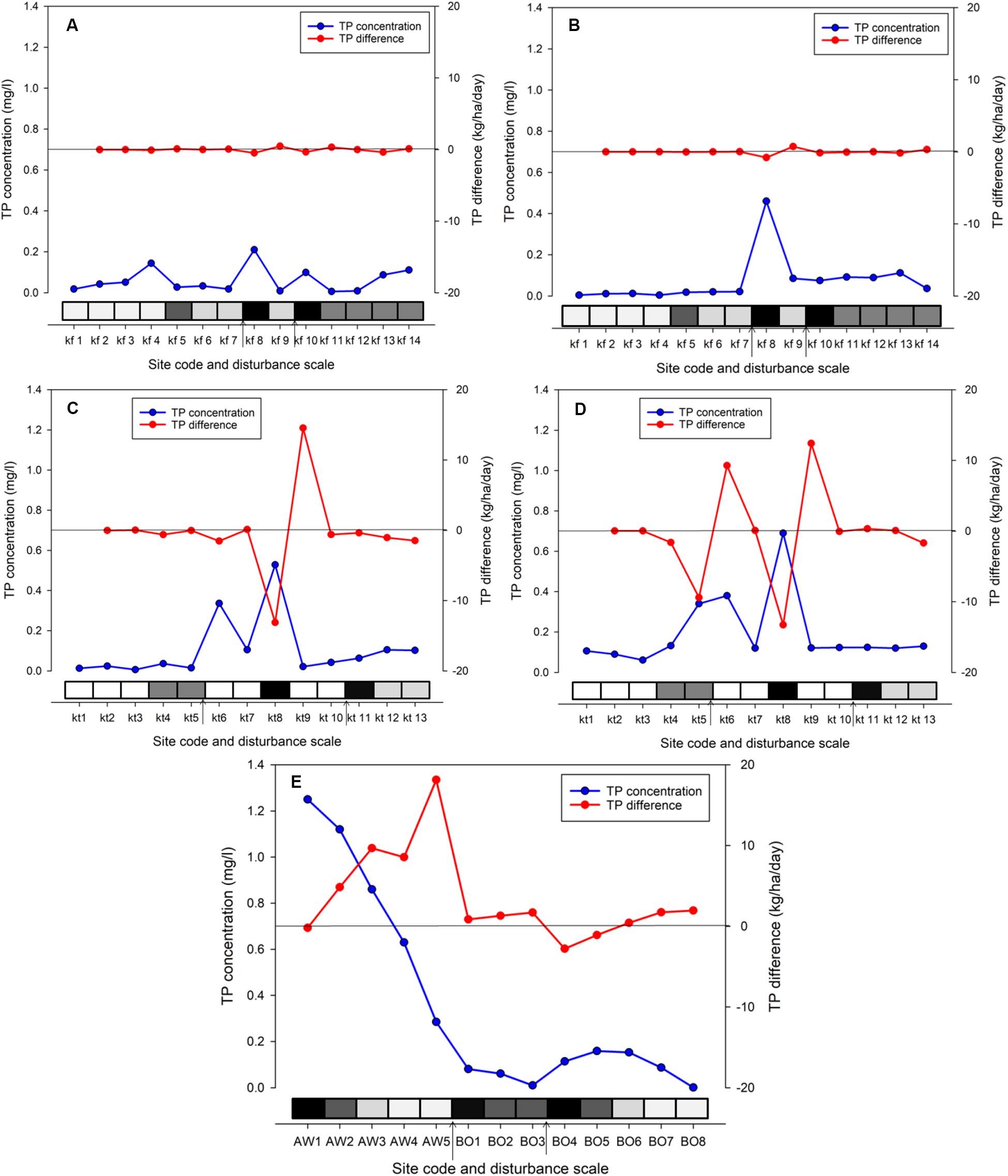
Figure 4. Total Phosphorous (TP) concentration and difference in Kofe (A), Kito (C) and Awetu-Boye (E) wetlands during wet season and Kofe (B) and Kito (D) during the dry season. Disturbance condition along the wetland ranged from very low (white) to very high (black). A black arrow indicates a site where a tributary enters into the main stream.
The highest concentration of TON (2.8 mg/l) was measured at Kt8 during the dry season where as the lowest concentration (0.03 mg/l) was recorded at Kt3 during the dry season (Figures 3C,D). The net release of TON in Kito wetland was 4.7 kg/ha/day and 3.8 kg/ha/day for wet and dry season, respectively. On the other hand, the highest concentration of TP was recorded at Kt8 (0.69 mg/l) during the dry season (Figures 4C,D). The net release of TP in Kito wetland was 4.1 kg/ha/day and 3.8 kg/ha/day for wet and dry season, respectively. There was no statistically significant difference in retention of TON between the dry and wet season (p = 0.08) (Table 2).
In Awetu wetland, the highest concentration of TON (1.28 mg/l) was measured at AW2, whereas the lowest concentration was measured at AW5 (0.97 mg/l) (Figure 3E). The net retention of TON in Awetu wetland was 18.3 kg/ha/day. On the other hand, highest concentration of TON in Boye wetland recorded at Boye 4 (2.23 mg/l). The net retention of TON in Boye wetland was estimated to be 27.6 kg/ha/day and 24.54 kg/ha/day for the wet and dry season, respectively. The net retention of TP in Awetu wetland was estimated to be 41 kg/ha/day whereas; Boye wetland had a net TP retention of 4.11 kg/ha/day and 3.8 kg/ha/day for the wet and dry season, respectively.
Impact of Disturbance on TSS Retention and Nutrient Retention
The first two PCA axes explained 73% of the total variation in human disturbance data. The factor loadings for the different variables are shown in Supplementary Material S2. The disturbance types that contributed most to the variation in PCA1 were: draining, farming, vegetation clearance, clay mining, grazing, filling and waste dumping. The second axis was mainly related to plantation and water abstraction. Among the nine disturbance factors, draining and vegetation clearance were significantly correlated with farming and had a variance inflation factor of 11 and 6, respectively. Accordingly, these two variables were excluded from the final analysis. Out of the seven remaining variables, only four disturbance types contributed to the final linear regression model. Filling, plantation and abstraction had no significant contribution to the regression model and were therefore removed. This model explained 73% of the variation in TSS retention (N = 75; R2 = 0.73; p < 0.001). The retained variables for the final model were: farming, waste dumping, clay mining and grazing. Farming alone explained 58% of the variation (R2 = 0.58) (Supplementary Material S2). The Kruskal-Wallis test indicated that sites with low degree of disturbance had a significantly higher TSS retention than moderately to highly disturbed sites (p < 0.05) (Figures 5A–C).
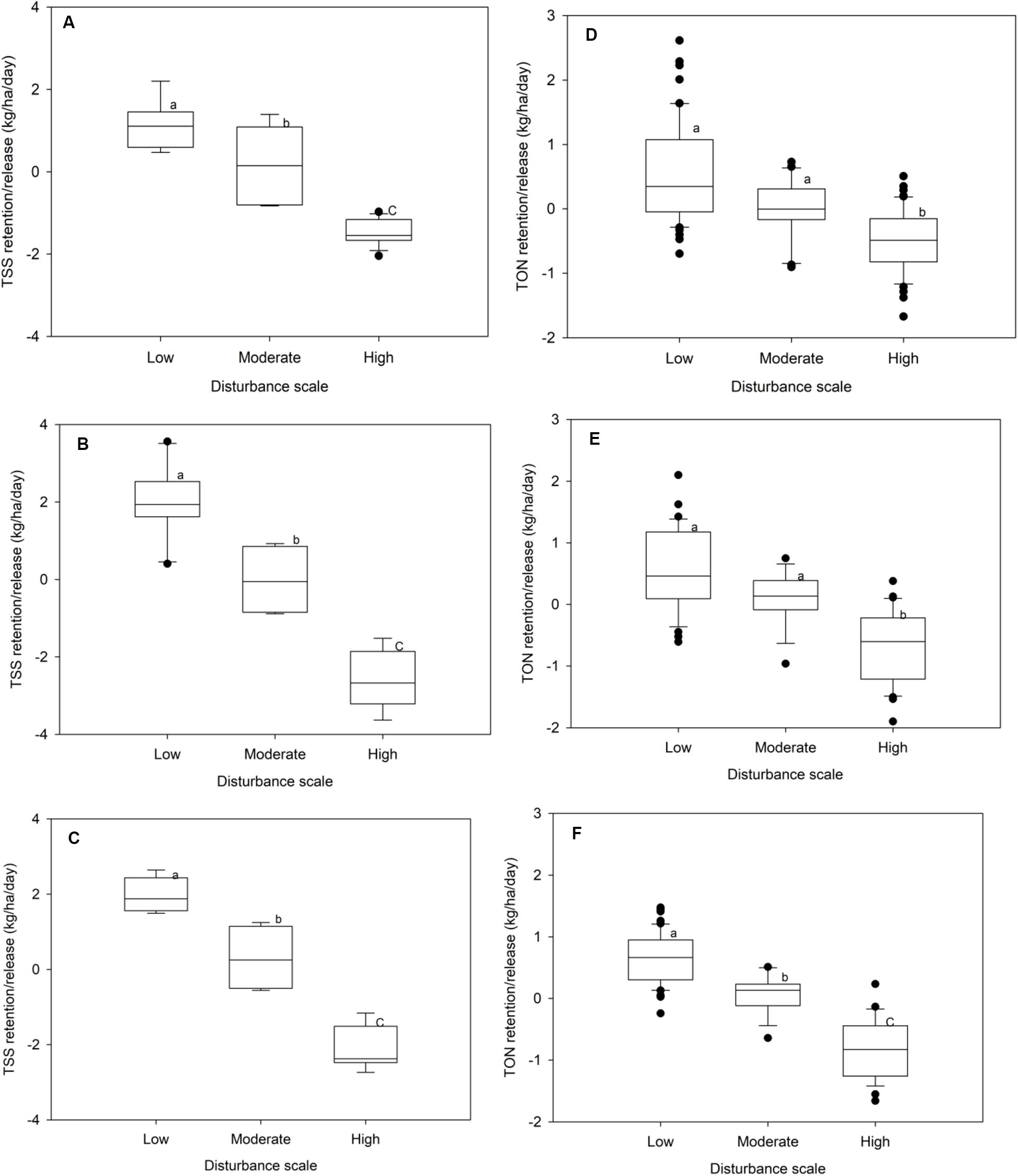
Figure 5. Box- and Whisker plots of the Total Suspended Solids retention log (x + 1) for Kofe (A), Kito (B) and Awetu-Boye (C) and Total Organic Nitrogen (TON) for Kofe (D), Kito (E), and Awetu-Boye (F) in relation to the disturbance classes. The full horizontal line in the box show the median, boxes represent interquartile ranges (25–75% percentiles) and range bars show maximum and minimum values. Statistically significant differences, based on Kruskal-Wallis test (p < 0.05), are indicated by letters (a, b, and C).
The stepwise multiple regression model was able to explain 28% of the variation in TON retention data (N = 75, R2 = 0.28, p < 0.001). The remaining 72% of the variation could be unmeasured wetland specific conditions which might contribute to the retention/release of nutrients. The retained variables for the final model were: grazing, waste dumping and farming. Grazing alone explained 25% of the variation (R2 = 0.25) (Supplementary Material S3). The Kruskal-Wallis test indicated that there was a significant difference in TON retention among different classes of disturbance p < 0.001 (Figures 5D–F).
Discussion
Overall, the TSS and nutrients retention capacity of wetlands was largely governed by the quality of water inflow and human disturbance. This study demonstrates that Awetu and Boye wetlands retained a substantial amount of total suspended solids (TSS) and nutrients, although the outflow concentrations were still higher than the other two wetlands. The higher loading rate of TSS and nutrients to Awetu and Boye wetlands might be attributed the discharge of untreated wastewater and solid wastes generated by more than 200,000 inhabitants of Jimma town into the tributaries of Awetu and Boye wetlands (Haddis et al., 2014). Indeed, a study on constructed wetlands has shown that higher mass loading rates generally result in higher sediment and nutrient retention rates (Redmond et al., 2014).
On the other hand, Kofe and Kito wetlands had relatively good water quality at the upstream sampling locations. However, water quality deteriorates as it flows in the downstream locations mainly due to human induced activities such as clay mining, eucalyptus plantation, farming and grazing. As a result, Kofe and Kito wetlands act as sources of TSS and nutrients (negative retention rate). The release of TSS and nutrients was higher during the wet season than the dry season. This might be the results of seasonality in rainfall that can lead to shifts in hydrologic connectivity between constituent water sources and wetland tributaries. Earlier studies have shown that changes in frequency, timing and intensity of rainfall events do not only alter runoff patterns, but can also affect subsequent pollutant loading (Borris et al., 2014). The total annual precipitation at the study area was calculated to be 1560 mm, two-third of which precipitated during the wet season (between June to September) (Supplementary Material S5). This might contribute to the high release of sediment and nutrients during the wet season.
Despite the high levels of TSS recorded in some wetlands, our results show that 50 to 80% of the TSS consisted of Volatile Suspended Solids (VSS), indicating that a large fraction of the TSS is organic and can be degraded by biological activity. The amount of TSS retained by the investigated wetlands is estimated to be 1848.6 ton per day. Out of which, 35% (647 ton/day) were fixed solids which can be accumulated in the downstream locations including the floodplains and Gilgel Gibe I reservoir. Therefore, the study wetlands play a role in the retention of about 236,161 tons of sediment per annum which potentially occupies 157,441 m3 space per annum. This volume is equivalent to 0.02% of the Gilgel Gibe I reservoir active capacity. The active capacity of Gilgel Gibe I reservoir is estimated to be 717,000,000 m3.
In this study, large variation in TSS and nutrient retention capacity was observed between sampling sites within wetlands. TSS and nutrient retention were strongly and negatively impacted by the degree of habitat disturbance. Habitat disturbances, particularly the conversion of riparian wetlands into cultivated land, livestock grazing, clay mining and waste dumping were found to contribute to the release of TSS and the increase in nutrients in the wetlands. The stepwise regression analysis revealed that farming activities are highly and negatively related to TSS retention. Drainage and vegetation clearing of wetlands for agricultural production results in increased degradation and reduced retention capacity. Similarly, Knox et al. (2008) reported that wetlands drained for agricultural use were characterized by lower retention rates and higher export of nutrients and sediments compared to natural reference wetlands, a phenomenon that was also observed in our study.
Our results indicate that grazing explains 25% of the variation in nutrient retention. Grazing alters the hydrology and the drainage pathways at a site by compacting the topsoil, which in turn decreases the infiltration capacity of the soil (Gathumbi et al., 2004; Pietola et al., 2005) and, consequently, leads to an increase in the release of nutrients and sediments by erosion (Kurz et al., 2005). In addition, grazing may lead to alteration in wetland plant community composition and structure, which changes the capacity to retain sediments and nutrients (Gathumbi et al., 2004). Moreover, the deposition of dung and urine during grazing are important sources of nitrogen and phosphorous to surface water (Edwards et al., 2000). Line et al. (2000) indicated that excluding grazing animals from streams in the United States reduced the TSS by 82%, TON by 55% and TP by 78%. Therefore, avoiding the access of cattle to wetlands would be beneficial for water quality. Although vegetation clearance was not found to be important in this study, earlier investigations report on the importance of wetland vegetation for nutrient retention (Schoonover et al., 2005). It might be that the reduction of vegetation was not strong enough to have an impact, or that vegetation clearance is very local while the impact of vegetation is acting at a large spatial scale.
Land use changes in the catchment, such as deforestation and agricultural intensification, increase runoff and hence increase the occurrence of peak flow discharges in the rivers, which enhances soil erosion (Van Den Eeckhaut et al., 2009). It has been estimated that about 48% of the Gilgel Gibe catchment is agricultural land (Tefera et al., 2002). Vaithiyanathan and Correll (1992) indicated that the flux of phosphorous associated with runoff from an agricultural watershed was found to be 8 to 10 times higher than that from a similar, but forested landscape. Next to intensive agriculture, landslides are an important source of sediment (Broothaerts et al., 2012). Most of the landslides are located near rivers and at the lowest points in the landscape, which can directly increase the input of sediment to the rivers and consequently increase the sediment load in the rivers and wetlands. These land use changes lead to accelerated rates of soil erosion, which might increase the sediment load in surface waters and cause major modifications to terrestrial carbon, nitrogen and phosphorous cycling (Quinton et al., 2010). Besides the overall negative effect on water quality, high input levels of sediment cause sedimentation and eutrophication problems and reduce the life span of reservoirs. For example, the lifespan of the Gilgel Gibe I reservoir, located 60 km downstream of the studied wetlands, is expected to be reduced by one third due to high sediment transport from the catchment (Devi et al., 2008). Therefore, enhancing TSS retention and nutrient retention capacity of wetlands by reducing anthropogenic pressures is an important step to safeguard both river water quality and the lifespan of the Gilgel Gibe I reservoir.
Conclusion and Recommendations
Freshwater wetlands located in the Awetu sub-watershed have the potential to retain a significant fraction of TSS and nutrients. The highest retention rates were generally measured in wetlands receiving high mass loading rates. On the other hand, wetlands that are affected by a wide variety of human disturbances had higher release of TSS and nutrients. The extent and type of human activities in the catchment (i.e., the land use) can influence the amount of TSS and nutrients released to wetlands. Therefore, reducing anthropogenic pressures such as farming, uncontrolled grazing, clay mining and waste dumping could help to enhance TSS and nutrients retention capacities of wetlands. Therefore, watershed management interventions considering land-use practices in the uplands along with processes occurring in the wetlands and tributaries should be considered for nutrient and sediment management options. Sediment and nutrient retention in wetlands could prevent siltation to eutrophication problem to the downstream surface water resources. This in turn could enhance the ecosystem services provided by aquatic resources.
Data Availability Statement
All datasets generated for this study are included in the article/Supplementary Material.
Author Contributions
SM conceived the main idea of the manuscript, collected and analyzed the data, and wrote the manuscript. LD, PL, WL, PG, and PB contributed to writing the manuscript. All authors read and approved the final manuscript.
Conflict of Interest
The authors declare that the research was conducted in the absence of any commercial or financial relationships that could be construed as a potential conflict of interest.
Acknowledgments
The authors are grateful to VLIR-UOS for financing this study. The first author was a recipient of a IUC (Institutional University Cooperation) Ph.D. scholarship from VLIR to carry out this work.
Supplementary Material
The Supplementary Material for this article can be found online at: https://www.frontiersin.org/articles/10.3389/fenvs.2020.00122/full#supplementary-material
References
Adwubi, A., Amegashie, B. K., Agyare, W. A., Tamene, L., Odai, S. N., Quansah, C., et al. (2009). Assessing sediment inputs to small reservoirs in Upper East Region. Ghana. Lake. Reserv. Manag. 14, 279–287. doi: 10.1111/j.1440-1770.2009.00410.x
APHA, AWWA, and WPCF, (1995). Standard Methods for the Examination of Water and Wastewater, 19th Edn. Washington DC: American Public Health Association.
Baldwin, D. S., Nielsen, D. L., Bowen, P. M., and Williams, J. (2005). Recommended Methods for Monitoring Floodplains and Wetlands. 1921038 20 9. MDBC Publication No. 72/04, Canberra: Murray-Darling Basin Commission.
Borris, M., Viklander, M., Gustafsson, A.-M., and Marsalek, J. (2014). Modelling the effects of changes in rainfall event characteristics on TSS loads in urban runoff. Hydrol.Process. 28, 1787–1796. doi: 10.1002/hyp.9729
Broothaerts, N., Kissi, E., Poesen, J., Van Rompaey, A., Getahun, K., Van Ranst, E., et al. (2012). Spatial patterns, causes and consequences of landslides in the Gilgel Gibe catchment. SW Ethiopia. Catena 97, 127–136. doi: 10.1016/j.catena.2012.05.011
Chawaka, S. N., Boets, P., Goethals, P. L. M., and Mereta, S. T. (2018). Does the protection status of wetlands safeguard diversity of macroinvertebrates and birds in southwestern Ethiopia? Biol. Conserv. 226, 63–71. doi: 10.1016/j.biocon.2018.07.021
Christopher, D. A., and David, J. (2004). Analysis of sediment retention in western riverine wetlands: the yampa River Watershed. Colorado, USA. Environ. Manag. 33, 318–330.
Cooper, C. M., Shields, F. D., Testa, S. I., and Knight, S. S. (2000). Sediment retention and water quality enhancement in disturbed watersheds. Int. J. Sediment Res. 15, 121–134.
Crumpton, W. G. (2001). Using wetlands for water quality improvement in agricultural watersheds; the importances of a watershed scale approach. Water. Sci. Technol. 44, 559–564. doi: 10.2166/wst.2001.0880
De Troyer, N., Mereta, S., Goethals, P., and Boets, P. (2016). water quality assessment of streams and wetlands in a fast Growing East African City. Water 8:123. doi: 10.3390/w8040123
Devi, R., Tesfahune, E., Legesse, W., Deboch, B., and Beyene, A. (2008). Assessment of siltation and nutrient enrichment of Gilgel Gibe dam, Southwest Ethiopia. Bioresour. Technol. 99, 975–979. doi: 10.1016/j.biortech.2007.03.013
Edwards, D. R., Hutchens, T. K., Rhodes, R. W., Larson, B. T., and Dunn, L. (2000). Quality of runoff from plots with simulated grazing. J. Am. Water Resour.As. 36, 1063–1073. doi: 10.1111/j.1752-1688.2000.tb05710.x
Fisher, J., and Acreman, M. C. (2004). Wetland nutrient removal: a review of the evidence. Hydrol. Earth. Syst. Sci. 8, 673–685. doi: 10.5194/hess-8-673-2004
Gathumbi, S. M., Bohlen, P. J., and Graetz, D. A. (2004). Nutrient enrichment of wetland vegetation and sediments in subtropical pastures. Soil. Sc. Soc. Am. J. 69, 539–548. doi: 10.2136/sssaj2005.0539
Haddis, A., Getahun, T., Mengistie, E., Jemal, A., Smets, I., and van der Bruggen, B. (2014). Challenges to surface water quality in mid-sized African cities: conclusions from Awetu-Kito Rivers in Jimma, south-west Ethiopia. Water Environ. J. 28, 173–182. doi: 10.1111/wej.12021
Hruby, T. (2004). Washington State Wetland rating System for Eastern Washington - Revised. Washington State Department of Ecology Publication No. 04-06-15, Olympia.
Jordan, T. E., Whigham, D. F., Hofmockel, K. H., and Pittek, M. A. (2003). Nutrient and sediment removal by a restored wetland receiving Agricultural runoff. J. Environ. Qual. 32, 1534–1547.
Kadlec, R. H. (2009). Wastewater treatment at the Houghton Lake wetland: hydrology and water quality. Ecol. Eng. 35, 1287–1311. doi: 10.1016/j.ecoleng.2008.10.001
Kadlec, R. H., and Reddy, K. R. (2001). Temperature effects in treatment wetlands: water environ. Res. 75, 543–557. doi: 10.2175/106143001x139614
Keenan, L., and Lowe, E. (2001). Determining ecologically acceptable nutrient loads to natural wetlands for water quality improvement. Water. Sci. Technol. 44, 289–294. doi: 10.2166/wst.2001.0842
Knox, K., Dahlgren, R. A., Tate, K. W., and Atwill, E. R. (2008). Efficacy of natural wetlands to retain nutrient. sediment and microbial pollutants. J. Environ. Qual. 37, 1837–1846. doi: 10.2134/jeq2007.0067
Kurz, I., Coxon, C., Tunney, H., and Ryan, D. (2005). Effects of grassland management practices and environmental conditions on nutrient concentrations in overland flow. J. Hydrol. 304, 35–50. doi: 10.1016/j.jhydrol.2004.07.022
Line, D. E., Harman, W. A., Jennings, G. D., Thompson, E. J., and Osmond, D. L. (2000). Nonpoint-source pollutant load reductions associated with livestock exclusion. J. Environ. Qual. 29, 1882–1890. doi: 10.2134/jeq2000.00472425002900060022x
Mateos, D., Comín, F. A., Pedrocchi, C., and Causapé, J. (2009). Effect of Wetlands on water quality of an agricultural catchment in a Semi-arid area under land use transformation. Wetlands 29, 1104–1113. doi: 10.1672/08-196.1
McJannet, D. L. (2007). Towards an Understanding of the Filter Function of Tropical Wetlands: Lessons From Temperate Locations, Development of a Conceptual Model and Design of a Field Monitoring Strategy. Canberra: CSIRO.
Mereta, S. T., Boets, P., De Meester, L., and Goethals, P. L. M. (2013). Development of a multimetric index based on benthic macroinvertebrates for the assessment of natural wetlands in Southwest Ethiopia. Ecol. Ind. 29, 510–521. doi: 10.1016/j.ecolind.2013.01.026
Millennium Ecosystem Assessment (2005). Ecosystems and Human Well-Being: Wetlands and Water Synthesis. Washington, DC: World Resources Institute.
Mironga, J. M. (2005). Effect of farming practices on wetlands of Kisii district. Kenya. Appl. Ecol. Environ. Res. 3, 81–91. doi: 10.15666/aeer/0302_081091
National Meteorological Agency, (2013). National Meteorological Agency. Available online at: https://public.wmo.int/en/media/news-from-members/ethiopianational-meteorology-agency (accessed April4, 2019).
Noe, G. B., and Hupp, C. R. (2009). Retention of riverine sediment and nutrient loads by coastal plain floodplains. Ecosystems 19, 726–748. doi: 10.1007/s10021-009-9253-5
Paudel, R., Grace, K. A., Galloway, S., Zamorano, M., and Jawitz, J. W. (2013). Effects of hydraulic resistance by vegetation on stage dynamics of a storm water treatment wetland. J. Hydrol. 484, 74–85. doi: 10.1016/j.jhydrol.2013.01.031
Pietola, L., Horn, R., and Yli-Halla, M. (2005). Effects of trampling by cattle on the hydraulic and mechanical properties of soil. Soil. Till. Res. 82, 99–108. doi: 10.1016/j.still.2004.08.004
Quinton, J. N., Govers, G., Van Oost, K., and Bardgett, R. D. (2010). The impact of agricultural soil erosion on biogeochemical cycling. Nat. Geo. Sci. 3, 311–314. doi: 10.1038/ngeo838
Reddy, K. R., Kadlec, R. H., Flaig, E., and Gale, P. M. (1999). phosphorus retention in streams and wetlands: a review. Crit. Rev. Env. Sci. Tech. 29, 83–146. doi: 10.1080/10643389991259182
Redmond, E. D., Just, C. L., and Parkin, G. F. (2014). Nitrogen removal from wastewater by an aerated subsurface-flow constructed wetland in cold climates. Water Environ. Res. 86, 305–313. doi: 10.2175/106143013x13736496908591
Russi, D., ten Brink, P., Farmer, A., Badura, T., Coates, D., Förster, J., et al. (2013). The Economics of Ecosystems and Biodiversity for Water and Wetlands. Brussels: IEEP.
Schoonover, J. E., Williard, K. W. J., Zaczek, J. J., Mangun, J. C., and Carver, A. D. (2005). Nutrient attenuation in agricultural surface runoff by riparian buffer zones in southern Illinois USA. Agroforest. Syst. 64, 169–180. doi: 10.1007/s10457-004-0294-7
Sileshi, A., Awoke, A., Beyene, A., Stiers, I., and Triest, L. (2020). Water purifying capacity of natural riverine wetlands in relation to their ecological quality. Front. Environ. Sci. 8:39. doi: 10.3389/fenvs.2020.00039
Statsoft Inc (2004). STATISTICA (data Analysis Software System Version 7. Available online at: www.statsoft.com (accessed November 10, 2019).
Tefera, B., Gezahegne, A., Yigezu, A., Jabbar, M., and Paulos, D. (2002). “Nature and cause of land degradation in the Oromiya region: a review,” in Socio-Economic and Policy Research Working Paper 36 ILRI, Nairobi, 82.
Vaithiyanathan, P., and Correll, D. L. (1992). The rhode river watershed: phosphorus distribution and export in forest and agricultural soils. J. Environ. Qual. 21, 280–288. doi: 10.2134/jeq1992.00472425002100020021x
Van Den Eeckhaut, M., Moeyersons, J., Nyssen, J., Abraha, J., Poesen, A., Haile, J., et al. (2009). Spatial patterns of old, deep-seated landslides: a case-study in the northern Ethiopian highlands. Geomorphology. 105, 239–252. doi: 10.1016/j.geomorph.2008.09.027
Verstraeten, G., Poesen, J., Gillijns, K., and Govers, G. (2006). The use of riparian vegetated filter strips to reduce river sediment loads: an overestimated control measure? Hydrol. Process. 20, 4259–4267. doi: 10.1002/hyp.6155
Woltemade, C. J. (2000). Ability of restored wetlands to reduce nitrogen and phosphorus concentrations in agricultural drainage water. J. Soil. Water. Conserv. 55, 303–309.
Keywords: anthropogenic disturbance, multiple regression, nutrients, retention capacity, riverine wetland, total suspended solids
Citation: Mereta ST, De Meester L, Lemmens P, Legesse W, Goethals PLM and Boets P (2020) Sediment and Nutrient Retention Capacity of Natural Riverine Wetlands in Southwest Ethiopia. Front. Environ. Sci. 8:122. doi: 10.3389/fenvs.2020.00122
Received: 28 February 2020; Accepted: 06 July 2020;
Published: 31 July 2020.
Edited by:
Ligang Xu, Nanjing Institute of Geography and Limnology (CAS), ChinaReviewed by:
Joris Eekhout, Spanish National Research Council, SpainJim Wallace, James Cook University, Australia
Copyright © 2020 Mereta, De Meester, Lemmens, Legesse, Goethals and Boets. This is an open-access article distributed under the terms of the Creative Commons Attribution License (CC BY). The use, distribution or reproduction in other forums is permitted, provided the original author(s) and the copyright owner(s) are credited and that the original publication in this journal is cited, in accordance with accepted academic practice. No use, distribution or reproduction is permitted which does not comply with these terms.
*Correspondence: Seid Tiku Mereta, c2VpZHRpa3VAeWFob28uY29t