- 1Systems and Process Engineering Centre, College of Engineering, Swansea University, Swansea, United Kingdom
- 2Leibniz-Institut für Ostseeforschung Warnemünde, Rostock, Germany
- 3Cluster of Excellence on Plant Sciences (CEPLAS), Institute of Population Genetics, Heinrich Heine University, Düsseldorf, Germany
- 4CSC – IT Center for Science Ltd., Espoo, Finland
- 5Fera Science Ltd., York, United Kingdom
While the presence of microplastics (MP) has been reported in aquatic habitats across the globe, the pathways through which they enter the environment are still poorly understood. Studies investigating the fate of MP in wastewater are gaining attention but are still scarce, despite the urgent need to understand the role of wastewater treatment plants (WWTP) as point sources of aquatic MP pollution. A likely reason for the limited number of WWTP-associated studies is that working with a biogenic organic matter (BOM)-rich sample matrix like wastewater is challenging. Here, we investigated the presence of MP throughout several stages of a WWTP at multiple depths, employing Fenton’s reagent and focal plane array-based reflectance micro-Fourier-transform infrared spectroscopic (FPA-based reflectance micro-FTIR) imaging, a protocol that allows the automated detection and identification of MP in complex samples with high organic matter content, without the need for previous visual sorting, or reducing considerably the thickness of the sample, or the use of IR-transparent transmission windows. It was found that the number of MP fragments detected at downstream stages of the WWTP notably decreased following the primary settlement stage, with primary settlement stage samples responsible for 76.9% of total MP detected. Despite the marked reduction in the number of MP particles following the primary settlement stage, an average total of 1.5 MP L–1 were identified in the final effluent of the WWTP.
Introduction
While microplastic (MP) pollution is an important environmental concern and its presence has been extensively studied globally (Browne et al., 2011; Cole et al., 2011), the pathways by which microplastics enter aquatic environments remain understudied. Research on the fate of microplastics in wastewater have started to gain more attention only during recent years. However, a likely reason for the still scarcity of such studies is that working with a biogenic organic matter (BOM)-rich sample matrix like wastewater is very challenging. Most aquatic-based investigations have assessed microplastics in seas, rivers and lakes, where the challenges associated with the separation of microplastics from other organic content are often less pronounced (Hidalgo-Ruz et al., 2012).
Despite the difficulty of detecting microplastics in BOM-rich matrices, there is a growing trend to investigate the fate of these pollutants in wastewater to help build a better picture of how wastewater treatment plants (WWTPs) cope with microplastic load and to what extent microplastics enter river systems through WWTPs (Lares et al., 2018; Sun et al., 2019). However, the majority of the studies have focused on the comparison between raw and treated wastewater samples (inlet and outlet only), and only a few have started addressing the different stages within the WWTP. Murphy et al. (2016) investigated microplastic abundances in various wastewater treatment stages and found that the majority of microplastics were removed during the grease removal (settlement stage), yet 0.25 (±0.04) MP L–1 were found in later stages including final effluent, where extrapolation of this data suggested 65 million microplastics could be released into natural waters every day from the WWTP studied. Carr et al. (2016) found differing results, suggesting that tertiary wastewater effluent is not a significant source of microplastics in the environment, finding one microplastic particle per 1,140 L (or 0.0009 MP L–1). Mintenig et al. (2017) sampled 12 WWTPs in Germany and estimated yearly discharges ranging between 9 × 107 to 4 × 109 MP particles and fibers from the WWTPs studied. Simon et al. (2018) estimated that ten of the largest Danish WWTPs discharge around 3 tonnes per year of MP in the size range 10–500 μm. Murphy et al. (2016) collected bulk samples before a sieving step (65 μm mesh size), Carr et al. (2016) fixed stacked sieves (400–445 μm) in a wastewater stream directly without taking bulk samples, Mintenig et al. (2017) applied enzymatic-oxidative purification in combination with focal plane array (FPA)-based transmission micro-FTIR, and Simon et al. (2018) used sieve meshes to eliminate particles and fibers larger than 500 μm in the raw and treated wastewater only. Different studies used diverse techniques to sample, extract, treat and detect microplastic presence in wastewater, and were conducted at different wastewater sites that may use alternate methods for wastewater treatment and support different population sizes and structures. Additionally, as the studies were conducted in areas with differing climate, rainfall and other geographical factors, it is perhaps unsurprising that the results show disparity.
Visual selection has been a commonly used technique for separating microplastics from a sample and relies on the user to visually determine what may be plastic debris before further analysis is undertaken. Visual selection is likely to bias study results, particularly when microplastics occupy the lowest section of the micron range or have a color that is similar to a background or the surrounding medium. The concern that visual selection may be inaccurate is well established (Reddy et al., 2006; Corcoran et al., 2009; Harrison et al., 2012; Hidalgo-Ruz et al., 2012; Rocha-Santos and Duarte, 2015; Tagg et al., 2015, 2017). While it may be possible to avoid analytical bias by treating every particulate solid as a potential microplastic, this may not be possible or practical in complex sample media such as active biologically-treated wastewater.
Over the past years, several studies have investigated the use of spectroscopic imaging as a method to detect microplastics in environmental samples without the need for a visual selection step (Löder et al., 2015; Tagg et al., 2015; Käppler et al., 2016; Mintenig et al., 2017). For example, FPA-based micro-Fourier-transform infrared spectroscopic (FPA-based micro-FTIR) imaging has been used to detect these pollutants in both wastewater and seawater, following their filtration onto membrane filters. Due to its semi-automated nature, this approach is much less user-intensive than approaches involving a visual selection step (Löder et al., 2015; Tagg et al., 2015; Mintenig et al., 2017). An additional advantage is that FPA-based micro-FTIR imaging can be used for the approximate sizing of microplastics using the chemical images produced. There are, however, issues surrounding microplastic sizing, and these are discussed in more detail below.
Infrared imaging can be performed either in transmission or reflectance mode, and both approaches have been successfully used to identify microplastics down to a size of ∼20–25 μm (Löder et al., 2015; Tagg et al., 2015; Mintenig et al., 2017). Although analyses in transmission mode provide comparatively well-resolved spectroscopic and imaging results (Löder et al., 2015), this mode may be unsuitable for samples containing thick and/or opaque plastic fragments. Indeed, in a recent study that employed FTIR imaging in transmission mode to detect microplastics in WWTP effluents, fragments of >500 μm had to be analyzed following a visual sorting step (Mintenig et al., 2017).
Although reflectance micro-FTIR imaging could be used as a stand-alone method to monitor microplastic concentrations in wastewater, this technique has mainly been employed in “proof-of-principle” studies involving either spiked particles or limited volumes of wastewater (Tagg et al., 2015, 2017) or in a sub-set of samples to detect larger particles only (Simon et al., 2018). In this study, we use reflectance micro-FTIR spectroscopy as the sole spectroscopic tool to investigate the presence of microplastics within three different treatment stages of a WWTP. A pre-treatment step using Fenton’s reagent enabled the effective filtration of wastewater for the rapid isolation of microplastics from these BOM-rich samples, while not impacting the size of the microplastics or affecting the presence and positions of the key FTIR absorbance bands for plastic identification (Tagg et al., 2017). By imaging the entire membrane filters directly, with no need for a visual pre-selection step or requirement of IR-transparent transmission windows, we demonstrate that reflectance micro-FTIR can be used as a rapid and reliable tool to detect microplastics in all of the treatment stages examined (including highly challenging sample types such as biologically activated wastewater).
Materials and Methods
Sampling
Wastewater samples were collected from a wastewater treatment facility in the East Midlands (United Kingdom) in summer 2015 between 12 and 4 pm (BST) and in spring 2016 between 12 and 4 pm (GMT). This is a major WWTP serving a population of 200,000. Samples were collected from both the surface (top 5 cm) and subsurface from three different treatment stages (primary settlement, activated biological anoxic treatment, and activated biological aerobic treatment) and the final effluent at point of release. Surface samples were collected using an aluminum telescopic sampling pole, extendable up to 6 m (Telescoop, Waterra Ltd., Solihull, United Kingdom, with a bottle holder scoop container model TSB-0750). Subsurface samples were collected using a hand-operated suction pump and weighted nozzle (Burkle Uni-Sampler, Bad Bellingen, Germany) set at a depth of 2.5 m, with the exception of the final effluent where the sampling depth was ∼60 cm. A total of 10 L was collected at each treatment stage in each visit, giving a total volume sampled of 80 L per visit (4 sampling sites × 2 different depths), with a total of 160 L in the two sampling visits (summer 2015 and spring 2016). Samples were regularly mixed via inversion during storage (no more than a month until analysis). For the activated biological aerobic treatment stage (where aeration occurs on site) a constant air flow was maintained using 0.2 μm membrane filters (VWR, Leicestershire, United Kingdom) on in and out air lines to prevent any contamination.
Sample Preparation
Samples were homogenized (via inversion mixing) and 1 L was extracted for analysis. Each 1 L sample underwent centrifugation at 2,038 g for 2 min in a Thermo Scientific Heraeus 400R Labofuge Refrigerated Centrifuge. The supernatant was retained for filtration and the solid fractions were treated using a 7-day 30% (v/v) H2O2 pretreatment (Tagg et al., 2015) to enable the solids to be effectively filtered afterward. Where this proved ineffective (in samples with very high levels of BOM, i.e., samples from the activated biological treatment: aerated and anoxic tanks), Fenton’s reagent (Fe2+ + H2O2 → Fe3+ + OH + HO–) pretreatment was used for 10 min (see Tagg et al., 2017 for a complete description of the methodology). Filtration was performed using a Millipore vacuum filtering assembly through 47-mm Isopore polycarbonate membrane filters (Millipore Corporation, Billerica, MA, United States), with a pore size of 5 μm at −40 kPa.
Sample Analysis
Membrane filters were imaged using FPA-based reflectance micro-FTIR using a PerkinElmer Spotlight micro-FTIR spectroscope (Beaconsfield, United Kingdom) equipped with a mercury–cadmium–telluride FPA detector (consisting of 16 gold-wired infrared detector elements). A per-pixel aperture size of 25 × 25 μm was used with two co-added scans per pixel and a spectral resolution of 16 cm–1. To identify microplastic types, chemical images of the entire 47 mm (diameter) membrane filter (see Figure 1) were generated for polyethylene (PE), polypropylene (PP), polystyrene (PS), polyvinyl chloride (PVC), and nylon, using the approach described in Tagg et al. (2015). Approximate microplastic size was determined by averaging x and y values (horizontal and vertical cross-section values taken at widest points) of each imaged microplastic using ImageJ v.1.50g (Abràmoff et al., 2004). Further details on the micro-FTIR methodology can be found in the Supplementary Material. Lab coats made of natural fabrics were used at all times during sampling and sample analyses to avoid contamination. Lab materials were carefully cleaned and covered directly with tin foil when not in use. Negative controls using MilliQ water as opposed to wastewater did not show presence of plastic particles or fibers.
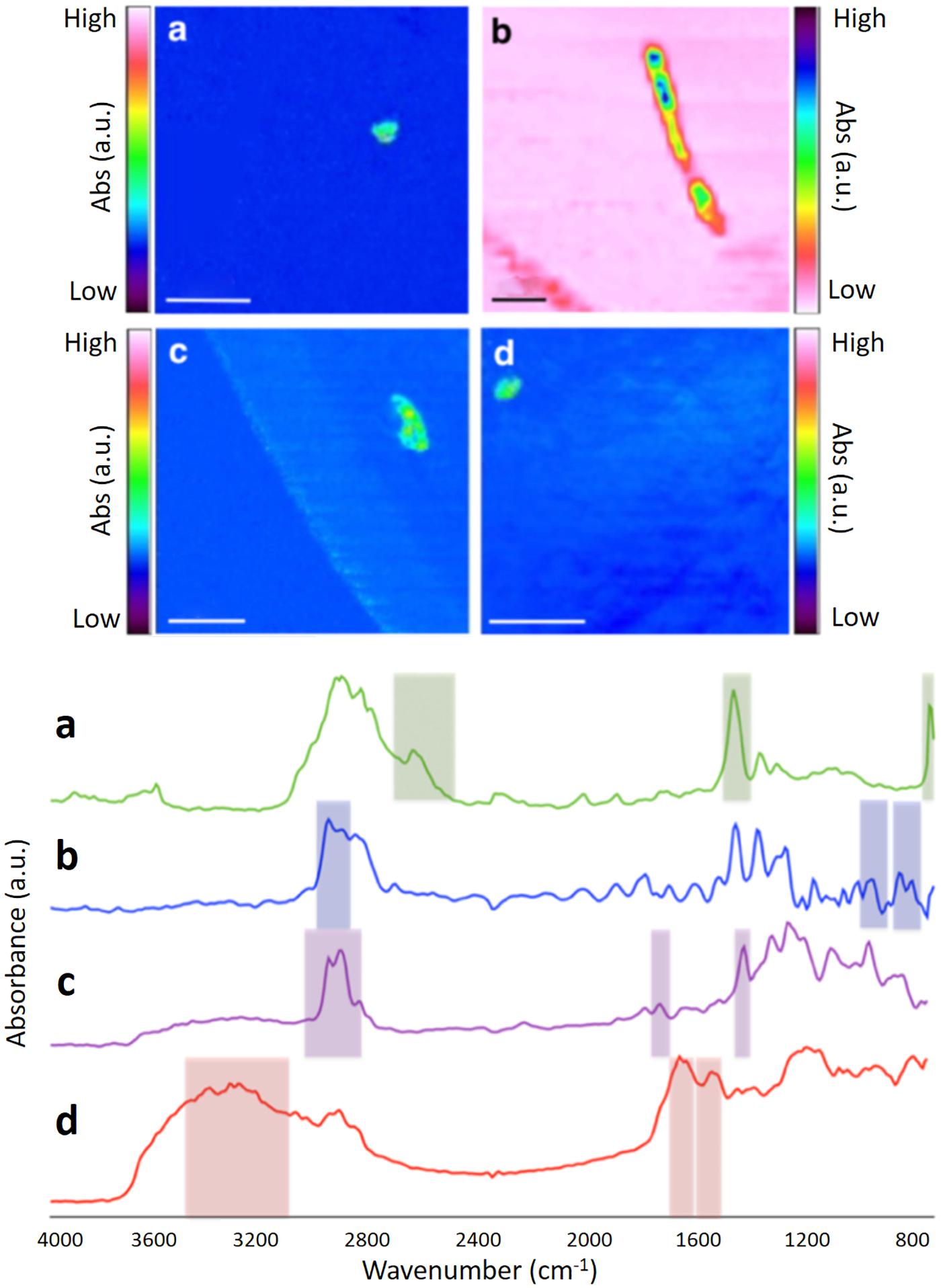
Figure 1. FPA-based micro-FTIR false-color images of microplastics found in the WWTP, generated using key spectral-peak selections (see Supplementary Material for details). Corresponding spectra of different polymer types are shown below the false-color images. (a) PE; (b) PP; (c) PVC; (d) Nylon. Scale bar = 1 mm.
Results and Discussion
Microplastic Count
Examples of the chemical images produced by FPA-based micro-FTIR imaging are shown in Figure 1. Two of the microplastics shown in Figure 1 resemble “microbeads” [PE (A) and nylon (D)], while one microplastic [PP (B)] resembles a fiber. When examining total microplastic counts across the various sampling points, the number of microplastics was found to decline from the first sampling point (primary settlement) to the final sampling point (final effluent; see Figure 2 and Table 1). This reduction was most marked directly following primary settlement and was more pronounced in the surface sample sets. The most likely reason for the reduction in microplastic counts is that the primary settlement (also referred to as the grease-removal stage by Carr et al., 2016) is designed for the removal of floating debris (James, 1971). Many common polymer types (such as PE and PP) have a lower density than water (Hidalgo-Ruz et al., 2012). Therefore, it is expected to see relatively high numbers of microplastics in settlement stage surface samples, where both floating and settling debris collects. Furthermore, microplastic abundance can be anticipated to decline in downstream samples because of the removal of floating debris at this treatment stage. A similar decline (from settlement stage to further downstream aquatic stages) was also observed by other authors (Carr et al., 2016; Murphy et al., 2016).
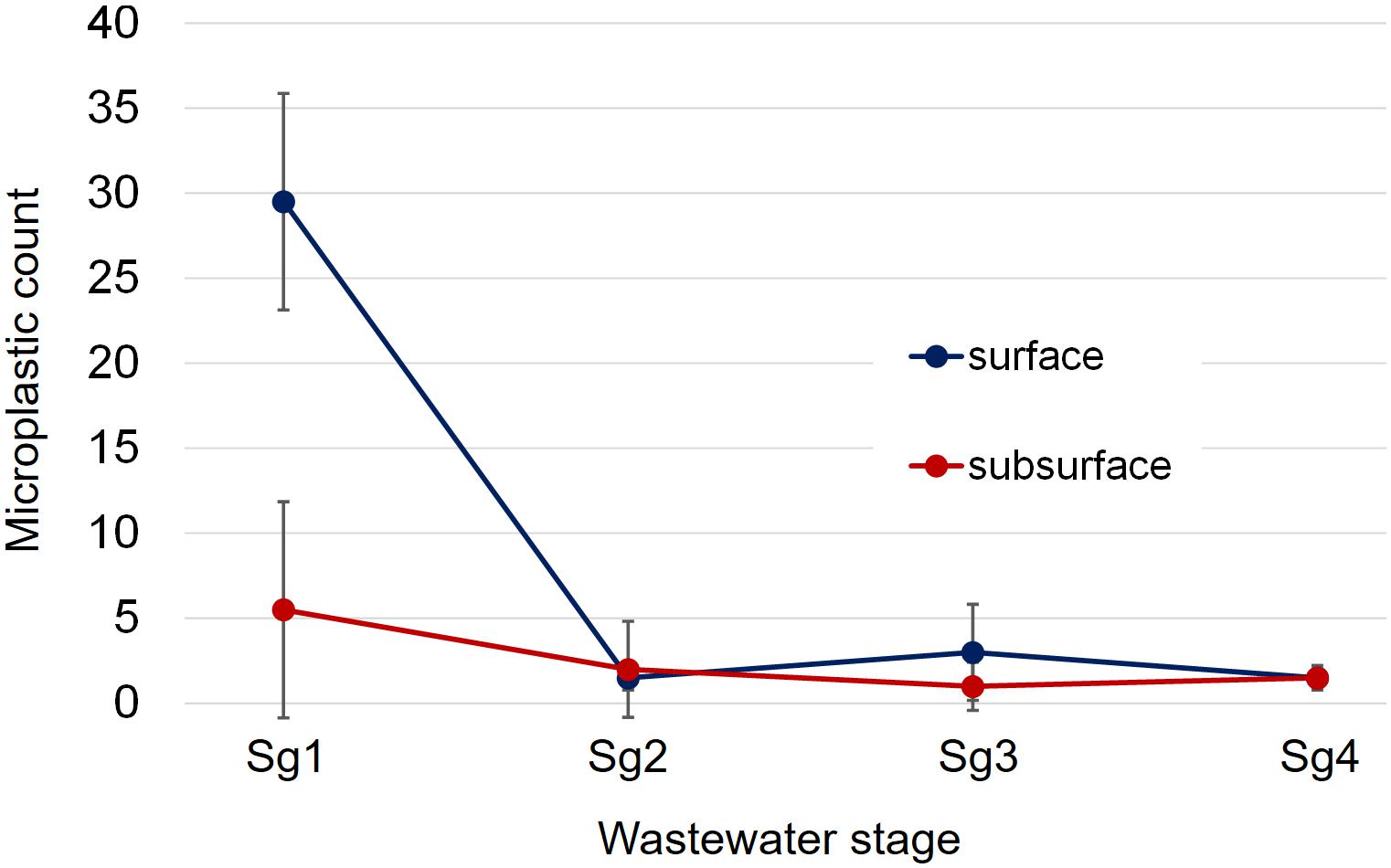
Figure 2. Total microplastic count data across the four wastewater stages sampled in parity with the progress of wastewater treatment from influent to effluent in both surface and subsurface samples. Stage 1 (Sg1) refers to the primary settlement stage, Stage 2 (Sg2) to the activated biological anoxic stage, Stage 3 (Sg3) to the activated biological aerobic stage, and Stage 4 (Sg4) to the final effluent. Reported values are mean microplastic counts based on the two sampling visits, with the error bars being one time the standard deviation.
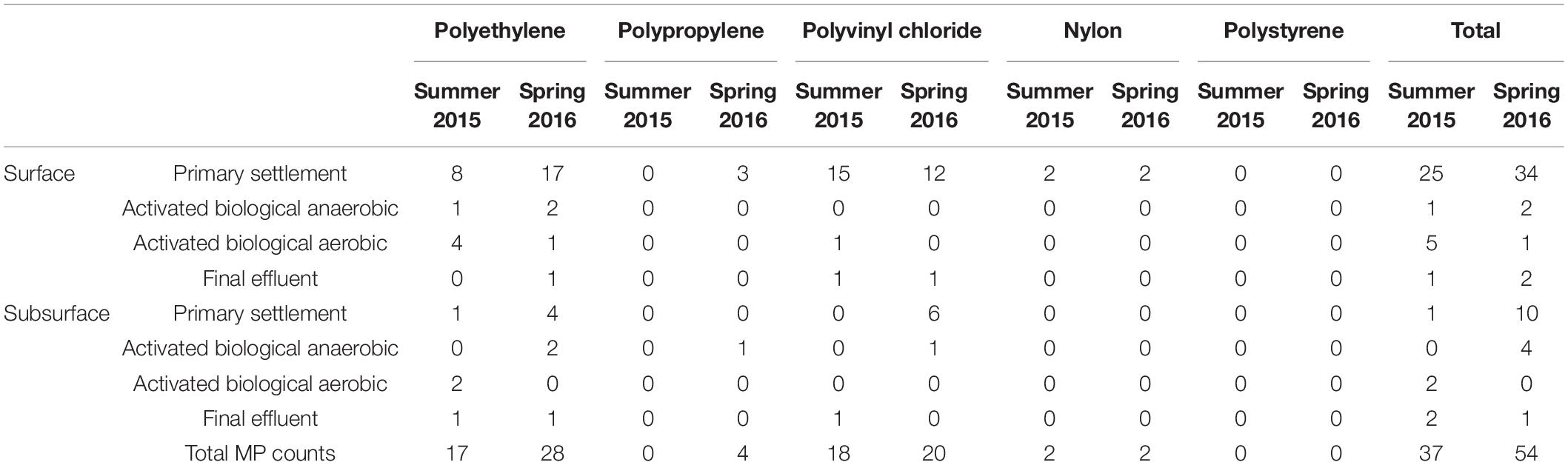
Table 1. Microplastic count data and polymer type across the four wastewater stages, in both surface and subsurface samples. Samples were collected from both the surface (top 5 cm) and subsurface (depth of 2.5 m, except for the final effluent where the sampling depth was ∼60 cm).
An important trend to consider is the difference in count data between surface and subsurface samples. It can be seen in Figure 2 that the surface counts were consistently higher than those for subsurface samples at the settlement stage (Sg 1). This suggests that surface samples are unlikely to give an accurate overall estimate of microplastic abundance within the water column, as this would greatly overestimate the overall microplastic content. However, counts following this point (Figure 2; Sg 2–4) were similar, indicating that the difference between surface and subsurface count data did not continue following the primary settlement stage. When the summer 2015 and spring 2016 sample sets were compared (Table 1), a similar reduction in plastic abundance was observed between both surface samples. However, this reduction, while evident to a lesser extent in the spring 2016 subsurface sample set, was not detected in the summer 2015 sample set. The lower microplastic count in settlement surface samples from summer 2015 compared to spring 2016 (1 compared to 10 microplastics found) may be due to variation within the water column. A possible reason for this variation may be that settlement stages tend not to be vigorously mixed to allow low density particulates and oils to congregate at the surface (James, 1971). However, a more plausible explanation could be lower/higher usage of water between Summer and Spring, or variations in rainfall.
Another observation was that, in multiple samples (surface summer 2015 and subsurface summer 2015 and spring 2016), microplastic count increased slightly between stages 2 and 3 (activated biological anoxic and activated biological aerobic). This difference may be due to the downstream stage being aerated, as the introduction of air streams within the aerobic stage may cause additional mixing and upwelling of particulate matter. This could therefore cause microplastics to be more dispersed.
Microplastic Type
The majority of microplastics identified were PE (50%; see Table 1). This finding is in general agreement with most studies; a meta-analysis of 68 environmental microplastic studies (Hidalgo-Ruz et al., 2012) found PE (along with PP) to be the most commonly identified polymer type. For studies conducted within WWTP, the data are less conclusive. A study of microplastic presence within a relatively small WWTP in Lysekil, Sweden (serving a population of approximate 14,000), conducted by the Swedish Environment Institute (Magnusson and Norén, 2014) identified two out of the total five microplastic particles tested as PE using FTIR. Mintenig et al. (2017) also found PE to be the most prevalent microplastic in the WWTPs sampled in Germany; while Simon et al. (2018) found that the most abundant type in raw wastewater from the largest Danish WWTPs was acrylates, and PE and Polyester for treated wastewater. Murphy et al. (2016) studied a larger WWTP in Glasgow, United Kingdom (serving a population of approximately 650,000), and found alkyds (a type of polyester resins that is often used in paints; Hofland, 2012) to be the most common microplastic in many sample sets. Another study analyzing WWTP effluent from two sites in New South Wales, Australia (2 × 750 mL; five particles) found polyester fibers to be most abundant (Browne et al., 2011).
There are many reasons why different studies investigating microplastic occurrence in wastewater can show different results. Variables pertaining to both time of year and time of day, population size associated with the WWTP, speed and volume of effluent produced per unit time, primary, secondary or tertiary treatment, stages selected for sampling and closely located plastic-producing or -utilizing industries may be attributed to variation in study results. However, the disparity between some of these findings (for microplastic type abundance) may also be partially explained by the fact that, in all studies, differing techniques were used and different amounts of wastewater were sampled. Murphy et al. (2016) used an alternate sampling approach, similar to this study, by bulk sampling rather than sieving the flow over an extended period, and tested 140 L total wastewater per replicate (30 L × 3 stages; 50 L × 1 stage) where samples were collected from surface wastewater only. Similar to Carr et al. (2016), some of these samples could not be fully analyzed, with three out of the four stage samples being partially examined, which makes the total coverage examined difficult to compare. Our study sampled 160 L of wastewater across the different stages and depths (80 L in summer and 80 L in spring), and examined 8 L per sampling trip (1 L surface and subsurface samples for each of the four treatment stages; 16 L total), where each 1 L sub-sample was fully analyzed in all cases. Therefore, disparity between results of these studies (without considering the possible discrepancy associated with experimental procedures such as visual selection, limited use of FTIR and partial sample analysis) may be attributed to differing sampling techniques and sample volumes analyzed. Even if techniques and sample volumes were more comparable, differences in results may well be expected due to the innate differences in treatment approaches of WWTPs and differing populations and industries they serve. Given these potential differences between different WWTPs, variation in microplastic abundance data may be expected to reflect differences in the overall composition of wastewater samples, depending on where the samples were obtained. However, it is still difficult to determine whether differences in results observed between studies analyzing microplastic fate in wastewater are due to differences in methodologies or not, until a standardized approach is adopted and applied across multiple sites.
It is possible that innate differences in wastewater composition (discussed above) may explain the relatively high percentage (42%) of PVC microplastics present in this study, particularly in comparison with nylon (4%) and PP (4%) and a complete absence of PS. Although PVC is a commonly produced and used plastic, it is much less common in the environment than other types of microplastics generally found in environmental microplastic studies (Hidalgo-Ruz et al., 2012). It is unclear why a relatively higher amount of PVC microplastics was found in this study, and additional research would be required to determine why PVC microplastics were common in this specific WWTP during the sampling period. While one origin of these fragments could be due to a high number of PVC-made pipes in the houses’ draining systems adjacent to the WWTP, it is also recommended to sample this WWTP for a longer period of time (years), with more replicates, and at different times of the year. This could provide more robust data and a much longer and continuous description of the type of plastics received by the WWTP, as external factors such as temporary industrial activity (where PVC plastics may be present in high amounts) could also play a role. A recent study by Wagner et al. (2019) found, for example, an unusually high abundance of PS microfragments while monitoring plastic concentrations in the rural subcatchment and downstream of the urban subcatchment of the River Parthe in Leipzig, Germany. It was found that the high PS abundance was due to building construction projects during the sampling period, particularly low-energy modernization, and construction with extensive use of PS building insulation material. Another potential factor to consider is that the approach in this study involved no visual pre-selection step. It is possible that microplastics in complex, BOM-rich substrates which share characteristics similar to non-plastic sample debris, such as color of morphology, would be systematically overlooked when using visual sorting of samples for analysis (Murphy et al., 2016). As a result, the higher PVC presence found in the present study might also be because the use of reflectance micro-FTIR imaging decreases the risk of underestimating microplastics that could otherwise be left unnoticed by visual selection, particularly given the small size of many of the polymer particles that were found.
Microplastics in Effluent
Another important factor to consider is the amount of microplastics released in effluent. In this study, the mean microplastic concentration in the effluent was 1.5 L–1. While these estimates can be useful for comparative purposes with other WWTP-focused microplastic studies (since similar abundances have been reported), the extrapolative approach used to produce such estimates may not be sufficiently accurate. In all current studies which have examined wastewater effluent, no two surveys have produced the same estimate. Our study produced an estimate of 1.5 MP L–1 of effluent, similar to the value of 1 MP L–1 reported by Browne et al. (2011). Within the site studied by Murphy et al. (2016), microplastic abundances were found to be 4× lower than this (0.25 MP L–1). Other studies have reported even lower rates of 0.009 MP L–1 (Magnusson and Norén, 2014) and 0.0009 MP L–1 (Carr et al., 2016). Microplastic counts for several WWTPs in Germany (Mintenig et al., 2017) found between 0 and 0.04 large (<500 μm) MP L–1, 0.08 and 9 small (<500 μm) MP L–1 and 0.1 and 5 MP fibers L–1; whereas at three WWTPs in Charleston Harbor, SC, United States (with different treatment sizes, operations and service compositions) counts ranged between 1 and 30 MP L–1 across all three WWTPs (Conley et al., 2019). As can be seen, this high variability in particle counts between sampling sites and differences in microplastic abundances are likely to be strongly influenced by location-specific factors that affect the overall microplastic load as previously discussed. In addition, each WWTP may have different stages and characteristics, also affecting their MP removal performance. For example, the use of a final filtration step undertaken at tertiary treatment plants, such as granular sand, pile fabric or microfiltration has been reported to reduce microplastic presence in effluent (Michielssen et al., 2016; Mintenig et al., 2017).
Microplastic Size
Determining the size distribution of microplastics in environmental samples is of importance since differently sized microplastics may respond differently to different waste removal treatments (Talvitie et al., 2017). The microplastics detected in our study had a mean size of 392 μm (±27 μm SE), with the smallest particle being 54 μm and largest being 1,277 μm. An interesting trend in the size data was the absence of microplastics >600 μm in samples after the primary settlement stage (Figure 3). This was consistent between both sampling visits and indicates that the primary settlement stage may be responsible for the removal of larger microplastics. It should also be considered that the settlement stage removes most microplastics present at this stage (as suggested by 76.9% of all microplastics identified in this study corresponding to the settlement stage; see Carr et al. (2016) and Murphy et al. (2016) for similar results).
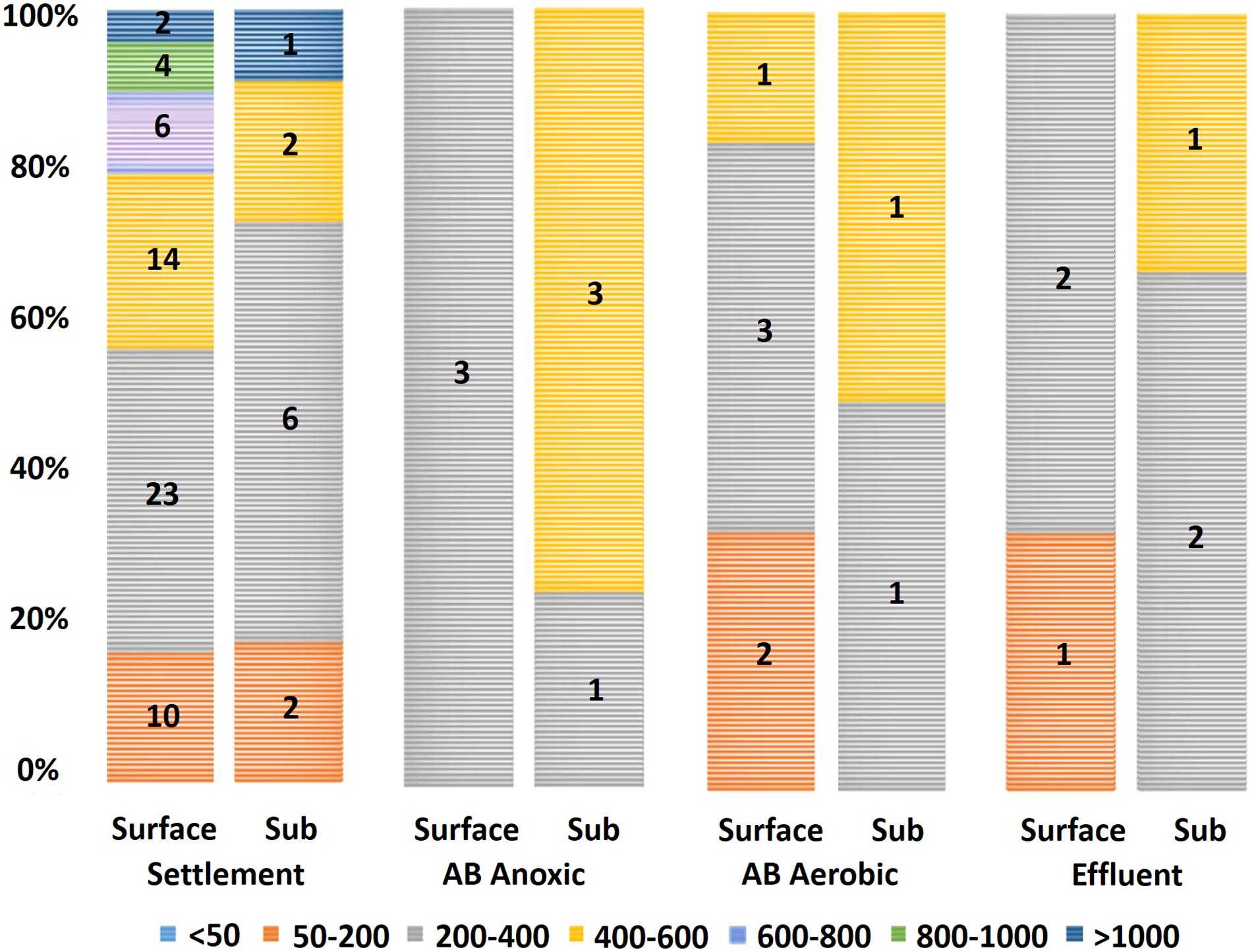
Figure 3. Microplastic size data by wastewater stage (total count of the two sampling visits). Legend entries refer to grouped sizes in micrometers (μm). Microplastics with sizes 200–400 μm had the highest count, with 45% (41/91) falling within this group. No microplastics larger than 600 μm appeared in samples downstream of the settlement stage. Sub: subsurface; AB: activated biological.
Direct comparisons of microplastic size between studies remain challenging. Some earlier wastewater-associated studies do not provide sufficient details on the sizes of the microplastics found in each stage (Browne et al., 2011; Magnusson and Norén, 2014; Carr et al., 2016), and in other publications, microplastics are grouped by size class, with microplastics <1 mm being the minimum reported size group (Doyle et al., 2011; Hidalgo-Ruz et al., 2012). Murphy et al. (2016) found plastic particles within liquid fraction samples to have a mean size of 598 μm (±89 μm). Mintenig et al. (2017) reported the occurrence of large (>500 μm) microplastics (1–5 particles in the range 500–7,200 μm per sample) and a larger amount of small (<500 μm) microplastics (3–12 microplastics per sample, 59% of which were between 50 and 100 μm and 96% of which were below 250 μm) in the WWTPs studied, which is more in agreement with what has been found in the present study.
Sampling protocols may explain why smaller microplastics were more common in both the present study and that of Mintenig et al. (2017) when compared with other studies. For example, Magnusson and Norén (2014) analyzed particles in wastewater collected on a filter with a mesh size of 300 μm. It is possible that microplastics smaller than the sieve apertures could not be retained. Since this mesh size is relatively close to the average particle size found in this study, this might explain why the present survey found a higher frequency of particles per liter of effluent (1.5 MP L–1 compared with 0.009 MP L–1). Studies by Doyle et al. (2011) and Lattin et al. (2004), for example, used plankton net trawls with mesh sizes of 505 and 333 μm, respectively. These sampling methods could also leave out microplastics smaller than the mesh sizes. Although these studies were not related to WWTPs but pelagic ecosystems, and hence have a different scope, it again points out the discrepancy in sampling protocols, and the need of a standardized approach for the analysis of microplastics in the environment.
Similar to our study, Mintenig et al. (2017) also used FPA-based micro-FTIR imaging allowing the authors to accurately identify microplastics down to a size of 20 μm. Although the authors used a visual selection step to identify larger particles and only imaged a portion of 11 mm-diameter filter membranes, this still represents a much more robust approach for identifying small microplastics than using visual selection alone. The use of chemical imaging likely explains why both our study and that by Mintenig et al. (2017) report such comparatively high numbers of small microplastics. However, it must also be noted that in our study, data suggests that larger microplastics (>600 μm) are removed during primary settlement. Therefore, the small fractions found by Mintenig et al. (2017) in treated WWTP effluents may be indicative of the sizes of microplastics typically found in latter treatment and effluent stages.
Limitations and Future Recommendations
While this study has improved our understanding of microplastic presence and composition in wastewater and confirmed the suitability of FPA-based reflectance micro-FTIR imaging for detecting microplastics within multiple wastewater treatment stages, there are several further ways in which research into this topic could be improved. An automated microplastic spectral data processing pipeline has been published for FTIR imaging analyses performed in transmission mode (Primpke et al., 2017; Brandt et al., 2020), and future work could involve extending it to data produced in reflectance mode. In addition, while bulk sampling was used without visual selection or need for physical transfer of microplastics from the membrane filter, there is still a lower detection limit of 25 μm, where microplastics smaller than this may be overlooked due to the minimum spatial resolution currently available for FPA FTIR detectors. However, methods utilizing rapid Raman imaging are now also emerging (Ando et al., 2016; Lares et al., 2018; Wolff et al., 2019) and recent work suggests that the combination of a FPA-based FTIR imaging approach with Raman imaging may allow for analysis of particles down to 1 μm (Käppler et al., 2016). While rapid Raman imaging currently requires specialist equipment, further developments in this research area could eventually make this technique suitable for routine microplastic monitoring purposes.
Finally, this study presents a snapshot of microplastic presence in a specific WWTP, but much more work is still required to obtain an accurate estimate of microplastics likely to be released/prevented from release into aquatic systems. Longer and continuous studies, with multiple replicates and visits throughout the year, comparing multiple stages of different WWTPs with different approaches to treatment, different population sizes and the effect of storm water overflow on microplastic release (with a consistent sampling and analytical protocol) are urgently required. Since no studies focusing on the release of microplastics from WWTPs have yet applied the same methods, more studies are required using a standardized approach to sampling and analyzing microplastic presence and size without the use of visual selection or partial-membrane filter analysis to improve the understanding of the fate of microplastics in wastewater. To improve our understanding of the temporal dynamics of microplastics in wastewater, more complex monitoring schemes would be required. Ideally, such schemes would involve studies conducted over several temporal ranges (investigating changes over the course of hours, days and seasons) in order to more fully understand microplastic load in wastewater effluent.
Conclusion
This study demonstrates the effectiveness of FPA-based reflectance micro-FTIR imaging for detecting microplastic present throughout key stages of wastewater treatment, by imaging the entire membrane filters directly, with no need for a visual pre-selection step or requirement of very thin samples and IR-transparent transmission windows. It was observed that the settlement stage (grease removal stage) was responsible for a considerable reduction in microplastics reaching latter stages in wastewater treatment. It was also found that microplastics >600 μm were particularly likely to be removed at this stage (since no microplastics >600 μm were found downstream of this stage). Microplastic counts at this stage were consistently higher in surface samples than subsurface. Nevertheless, analyzing only surface-samples may underestimate microplastic numbers if subsurface sampling is ignored. In this study, samples were collected at surface and subsurface from three different wastewater treatment stages (primary settlement, activated biological anoxic treatment, and activated biological aerobic treatment) and the final effluent, during Summer 2015 and Spring 2016. Ten liters were collected at each treatment stage in each visit, giving a total volume sampled of 80 L per visit (four sampling sites × two different depths), with a total of 160 L in the two sampling visits. A total of 1.5 MP L–1 was found in the final effluent (average combination of surface and subsurface samples, and the two visits). While these results show a “snapshot” of microplastic presence in a specific WWTP, more accurate or reliable values could be obtained by the inclusion of additional replicates, longer and continuous studies, and additional visits at different times of the year. This study helps to further the insight into the fate of microplastics in WWTPs using FPA-based reflectance micro-FTIR imaging, but further work is needed to obtain an improved understanding of this topic by using consistent and accurate sampling methodologies and extensive temporal-based monitoring schemes at a variety of sites.
Data Availability Statement
The raw data supporting the conclusions of this article will be made available by the authors, without undue reservation.
Author Contributions
AT, JO, and MS designed and performed the sample collection at the WWTP. AT and JO developed the reflectance micro-FT-IR protocol. AT performed the Fenton’s treatment and the reflectance micro-FT-IR analyses. MS, JH, and YJ-N contributed to discussions and improvement in the data collection/analysis and methodology. YJ-N provided extra expertise in Fenton’s treatment. CS and EB contributed to the structure and editing of the manuscript. The manuscript was authored by AT with all authors contributing to manuscript preparation.
Funding
This work was funded by a NERC (Natural Environment Research Council) CASE studentship (NE/K007521) with contribution from industrial partner Fera Science Ltd., United Kingdom.
Conflict of Interest
CS and EB were employed by Fera Science Ltd.
The remaining authors declare that the research was conducted in the absence of any commercial or financial relationships that could be construed as a potential conflict of interest.
Acknowledgments
We thank Peter Vale and Ashley Howkins for their assistance in the acquisition of wastewater samples.
Supplementary Material
The Supplementary Material for this article can be found online at: https://www.frontiersin.org/articles/10.3389/fenvs.2020.00145/full#supplementary-material
References
Abràmoff, M. D., Magalhães, P. J., and Ram, S. J. (2004). Image processing with ImageJ. Biophotonics Int. 11, 36–42.
Ando, J., Palonpon, A. F., Sodeoka, M., and Fujita, K. (2016). High-speed raman imaging of cellular processes. Curr. Opin. Chem. Biol. 33, 16–24. doi: 10.1016/j.cbpa.2016.04.005
Brandt, J., Bittrich, L., Fischer, F., Kanaki, E., Tagg, A., Lenz, R., et al. (2020). High-throughput analyses of microplastic samples using fourier transform infrared and raman spectrometry. Appl. Spectrosc. (in press). doi: 10.1177/0003702820932926
Browne, M. A., Crump, P., Niven, S. J., Teuten, E., Tonkin, A., Galloway, T., et al. (2011). Accumulation of microplastic on shorelines woldwide: sources and sinks. Environ. Sci. Technol. 45, 9175–9179. doi: 10.1021/es201811s
Carr, S. A., Liu, J., and Tesoro, A. G. (2016). Transport and fate of microplastic particles in wastewater treatment plants. Water Res. 91, 174–182. doi: 10.1016/j.watres.2016.01.002
Cole, M., Lindeque, P., Halsband, C., and Galloway, T. S. (2011). Microplastics as contaminants in the marine environment: a review. Mar. Pollut. Bull. 62, 2588–2597. doi: 10.1016/j.marpolbul.2011.09.025
Conley, K., Clum, A., Deepe, J., Lane, H., and Beckingham, B. (2019). Wastewater treatment plants as a source of microplastics to an urban estuary: removal efficiencies and loading per capita over one year. Water Res. X 3:100030. doi: 10.1016/j.wroa.2019.100030
Corcoran, P. L., Biesinger, M. C., and Grifi, M. (2009). Plastics and beaches: a degrading relationship. Mar. Pollut. Bull. 58, 80–84. doi: 10.1016/j.marpolbul.2008.08.022
Doyle, M. J., Watson, W., Bowlin, N. M., and Sheavly, S. B. (2011). Plastic particles in coastal pelagic ecosystems of the Northeast Pacific ocean. Mar. Environ. Res. 71, 41–52. doi: 10.1016/j.marenvres.2010.10.001
Harrison, J. P., Ojeda, J. J., and Romero-Gonzalez, M. E. (2012). The applicability of reflectance micro-Fourier-transform infrared spectroscopy for the detection of synthetic microplastics in marine sediments. Sci. Total Environ. 416, 455–463. doi: 10.1016/j.scitotenv.2011.11.078
Hidalgo-Ruz, V., Gutow, L., Thompson, R. C., and Thiel, M. (2012). Microplastics in the marine environment: a review of the methods used for identification and quantification. Environ. Sci. Technol. 46, 3060–3075. doi: 10.1021/es2031505
Hofland, A. (2012). Alkyd resins: From down and out to alive and kicking. Prog. Org. Coat. 73, 274–282. doi: 10.1016/j.porgcoat.2011.01.014
James, G.V. (ed.) (1971). Water Treatment: A Survey of Current Methods of Purifying Domestic Supplies and of Treating Industrial Effluents and Domestic Sewage, 4th ed. London: Technical Press.
Käppler, A., Fischer, D., Oberbeckmann, S., Schernewski, G., Labrenz, M., Eichhorn, K.-J., et al. (2016). Analysis of environmental microplastics by vibrational microspectroscopy: FTIR. Raman or both? Anal. Bioanal. Chem. 408, 8377–8391. doi: 10.1007/s00216-016-9956-3
Lares, M., Ncibi, M. C., Sillanpää, M., and Sillanpää, M. (2018). Occurrence, identification and removal of microplastic particles and fibers in conventional activated sludge process and advanced MBR technology. Water Res. 133, 236–246. doi: 10.1016/j.watres.2018.01.049
Lattin, G. L., Moore, C. J., Zellers, A. F., Moore, S. L., and Weisberg, S. B. (2004). A comparison of neustonic plastic and zooplankton at different depths near the southern California shore. Mar. Pollut. Bull. 49, 291–294. doi: 10.1016/j.marpolbul.2004.01.020
Löder, M. G. J., Kuczera, M., Mintenig, S., Lorenz, C., and Gerdts, G. (2015). Focal plane array detector-based micro-Fourier-transform infrared imaging for the analysis of microplastics in environmental samples. Environ. Chem. 12, 563–581. doi: 10.1071/en14205
Magnusson, K., and Norén, F. (2014). Screening of Microplastic Particles in and Down-Stream a Wastewater Treatment Plant. Stockholm: Swedish Environment Institute.
Michielssen, M. R., Michielssen, E. R., Ni, J., and Duhaime, M. B. (2016). Fate of microplastics and other small anthropogenic litter (SAL) in wastewater treatment plants depends on unit processes employed. Environ. Sci. Water Res. Technol. 2, 1064–1073. doi: 10.1039/C6EW00207B
Mintenig, S. M., Int-Veen, I., Löder, M. G. J., Primpke, S., and Gerdts, G. (2017). Identification of microplastic in effluents of waste water treatment plants using focal plane array-based micro-Fourier-transform infrared imaging. Water Res. 108, 365–372. doi: 10.1016/j.watres.2016.11.015
Murphy, F., Ewins, C., Carbonnier, F., and Quinn, B. (2016). Wastewater treatment works (WwTW) as a source of microplastics in the aquatic environment. Environ. Sci. Technol. 50, 5800–5808. doi: 10.1021/acs.est.5b05416
Primpke, S., Lorenz, C., Rischer-friesenhausen, R., and Gerdts, G. (2017). An automated approach for microplastics analysis using focal plane array (FPA) FTIR microscopy and image analysis. Anal. Methods 9, 1499–1511. doi: 10.1039/C6AY02476A
Reddy, M. S., Basha, S., Adimurthy, S., and Ramachandraiah, G. (2006). Description of the small plastics fragments in marine sediments along the alang-sosiya ship-breaking yard, India. Estuar. Coast. Shelf Sci. 68, 656–660. doi: 10.1016/j.ecss.2006.03.018
Rocha-Santos, T., and Duarte, A. C. (2015). A critical overview of the analytical approaches to the occurrence, the fate and the behavior of microplastics in the environment. TrAC Trends Anal. Chem. 65, 47–53. doi: 10.1016/j.trac.2014.10.011
Simon, M., van Alst, N., and Vollertsen, J. (2018). Quantification of microplastic mass and removal rates at wastewater treatment plants applying focal plane array (FPA)-based fourier transform infrared (FT-IR) imaging. Water Res. 142, 1–9. doi: 10.1016/j.watres.2018.05.019
Sun, J., Dai, X., Wang, Q., van Loosdrecht, M. C. M., and Ni, B.-J. (2019). Microplastics in wastewater treatment plants: detection, occurrence and removal. Water Res. 152, 21–37. doi: 10.1016/j.watres.2018.12.050
Tagg, A. S., Harrison, J. P., Ju-Nam, Y., Sapp, M., Bradley, E. L., Sinclair, C. J., et al. (2017). Fenton’s reagent for the rapid and efficient isolation of microplastics from wastewater. Chem. Commun. 53, 372–375. doi: 10.1039/C6CC08798A
Tagg, A. S., Sapp, M., Harrison, J. P., and Ojeda, J. J. (2015). Identification and quantification of microplastics in wastewater using focal plane array-based reflectance micro-FT-IR imaging. Anal. Chem. 87, 6032–6040. doi: 10.1021/acs.analchem.5b00495
Talvitie, J., Mikola, A., Koistinen, A., and Setala, O. (2017). Solutions to microplastic pollution - Removal of microplastics from wastewater effluent with advanced wastewater treatment technologies. Water Res. 123, 401–407. doi: 10.1016/j.watres.2017.07.005
Wagner, S., Klöckner, P., Stier, B., Römer, M., Seiwert, B., Reemtsma, T., et al. (2019). Relationship between discharge and river plastic concentrations in a rural and an urban catchment. Environ. Sci. Technol. 53, 10082–10091. doi: 10.1021/acs.est.9b03048
Keywords: microplastics, reflectance micro-FTIR, wastewater, Fenton’s reagent, infrared imaging
Citation: Tagg AS, Sapp M, Harrison JP, Sinclair CJ, Bradley E, Ju-Nam Y and Ojeda JJ (2020) Microplastic Monitoring at Different Stages in a Wastewater Treatment Plant Using Reflectance Micro-FTIR Imaging. Front. Environ. Sci. 8:145. doi: 10.3389/fenvs.2020.00145
Received: 11 May 2020; Accepted: 29 July 2020;
Published: 25 August 2020.
Edited by:
André Ricardo Araújo Lima, Center for Marine and Environmental Sciences (MARE), PortugalReviewed by:
Joao Pinto Da Costa, University of Aveiro, PortugalTeresa Bottari, Istituto per l’Ambiente Marino Costiero (IAMC), Italy
Copyright © 2020 Tagg, Sapp, Harrison, Sinclair, Bradley, Ju-Nam and Ojeda. This is an open-access article distributed under the terms of the Creative Commons Attribution License (CC BY). The use, distribution or reproduction in other forums is permitted, provided the original author(s) and the copyright owner(s) are credited and that the original publication in this journal is cited, in accordance with accepted academic practice. No use, distribution or reproduction is permitted which does not comply with these terms.
*Correspondence: Jesús J. Ojeda, ai5qLm9qZWRhbGVkb0Bzd2Fuc2VhLmFjLnVr