- 1Aquatic Ecosystems Group, IHE Delft Institute for Water Education, Delft, Netherlands
- 2National Water and Sewerage Corporation, Kampala, Uganda
- 3Institute for Land, Water and Society, Charles Sturt University, Albury, NSW, Australia
- 4VITUKI Hungary Plc., Budapest, Hungary
- 5National University of Public Service, Budapest, Hungary
- 6Aquatic Ecology and Water Quality Management, Wageningen University & Research, Wageningen, Netherlands
The Namatala Wetland in Uganda faces severe degradation from agricultural development and urbanization. Besides the Namatala River and tributary rural streams, the wetland receives surface water from Mbale town and wastewater from two sets of wastewater stabilization ponds. The objective of this study was to examine water quality, and sediment and nutrient retention in different land use zones. Five hydrogeomorphic units (HGMUs) were distinguished on the basis of soil, hydrology and land use. HGMUs 1 and 2 in the upstream part of the wetland are characterized by drainage channels and mixed agriculture. HGMU 3 is a wet floodplain with intensive rice farming. HGMU 4 and 5 are permanently wet units in the downstream part of the wetland with moderate rice farming and partly intact papyrus (Cyperus papyrus L.) vegetation. Stream discharge was measured, and surface water samples collected, monthly from the river channel, the tributaries, and the five HGMUs from April 2015 to October 2016. Significant differences in total nitrogen (TN), phosphorus (TP) and total suspended solids (TSS) were observed among the streams and among the five HGMUs, with highest concentrations in urban streams and lowest in the main river channel and rural streams. Among the HGMUs, nutrients and TSS were highest within HGMU 3 and lowest in HGMU 1 and 5. Loads of nutrients and sediment into the wetland were greater from the main river channel compared with urban and rural streams. Regressions of net TN, TP, and TSS yields for each HGMU against river discharge showed a net loss of nutrients and sediments in HGMU 3 with the most intensive agriculture, and net retention in HGMUs 4 and 5 which mostly maintain their wetland character. This study shows that sediment and nutrient retention in the downstream part of the wetland compensate for increased export caused by agricultural and urban land use in the middle and upper zones of the wetland, thus maintaining net nutrient retention of Namatala Wetland. However, there is a trade-off between economic development and wetland protection and future management planning should incorporate more sustainable farming practices and improved wastewater treatment.
Introduction
Conversion of wetlands for agriculture and settlements, and their degradation through pollution with wastewater, are a persistent and global environmental problem (Davidson, 2014). This leads to loss of ecosystem services with negative consequences for people and wildlife (Verhoeven et al., 2006; Díaz et al., 2015; Ramsar Convention on Wetlands, 2018). In Sub-Saharan Africa, although many countries are signatories to the Ramsar Convention on Wetlands and have national policies for wetland protection (Gardner et al., 2009; Dini and Everard, 2018), loss and degradation of wetlands continue. Agriculture is one of the most important drivers of wetland loss (van Asselen et al., 2013; Ramsar Convention on Wetlands, 2018), with most countries having policies for expanding agricultural production (OECD/FAO, 2016). The need to produce food is increasing, and a projected growth of the population on the African continent to 2.1 billion people by 2050 drives a policy conflict between protecting wetlands and achieving food security.
Uganda was one of the first African countries to recognize the importance of wetland ecosystem services by developing a wetland policy and designating Ramsar sites. The policy aims at ensuring that there is no drainage of wetlands, that activities in and around wetlands are non-destructive, and that developments are subject to environmental impact assessment and audits (Mafabi, 2000, 2018). Despite this, sustainable management of Ugandan wetlands has often floundered in the face of local pressures. Striking a balance and accepting trade-offs between protection and exploitation of wetlands is often restricted by lack of knowledge on wetland function. More information is needed on land use that contributes to nutrient and sediment loads (Namaalwa et al., 2013) and the capacity of wetlands to remove nutrients from wastewater (Kansiime and Nalubega, 1999; Bateganya et al., 2015). A lack of government capacity in implementing policy exacerbates the problem (Ostrovskaya et al., 2013). More knowledge about the impact of economic development and land use change would help wetland users and policy makers view patterns and impacts more clearly, prioritize vulnerable zones of the catchment, and implement sustainable land use planning and practice.
Several processes contribute to retention of sediment and nutrients in wetlands, including sedimentation, uptake and storage in vegetation, adsorption to soil, and gaseous losses to the atmosphere. Their relative importance depends on environmental conditions, soil characteristics, and hydrology (Burt and Pinay, 2005; Noe and Hupp, 2007; Lohse et al., 2009; Pärn et al., 2012). Variable land use and seasonal climatic patterns in small wetland areas provide scale-dependent effects on biophysical processes (Jung et al., 2008; Park et al., 2011; Uwimana et al., 2017). Translating knowledge of hydro-ecological processes into maintenance of ecosystem services and conservation of biodiversity is needed as an evidence-based approach to managing pressures from wastewater, food production and livelihoods (Biggs et al., 2015).
Different zones of a wetland catchment that are subject to different intensities of anthropogenic pressure can be expected to vary in their contributions to nutrient and sediment retention (Baker et al., 2006; Noe and Hupp, 2007). This raises questions about the overall functioning of the wetland. Can the loss of retention in one zone be compensated by retention in another? How does this overall functionality depend on hydrological connectivity and flow paths? Can these functions be quantified to find a sustainable balance between the use of the wetland ecosystem (e.g., for agricultural production) and a desirable level of ecological functioning? For Namatala Wetland in Uganda, we hypothesize that retention in the zones influenced by agriculture and wastewater is reduced compared with zones where the integrity of the original wetland and its natural processes (with papyrus vegetation and regular flooding) are still intact. The overall objective of this study was to examine water quality and estimate sediment and nutrient loads across upstream and downstream zones with different anthropogenic pressures (wastewater discharge and agriculture) in a tropical wetland and river system. Specific objectives were: (1) to describe discharge and water quality in river and wetland in relation to seasonal and land use variation; (2) to estimate loads of sediment and nutrients (nitrogen, phosphorus) from the river and its tributaries; and (3) to compare different wetland land use zones in terms of sediment and nutrient retention.
Materials and Methods
Study Area
The Namatala Wetland is part of the Doho-Namatala Wetland System within the Lake Kyoga Basin. It lies along the main Namatala River with a stretch of about 31 km from upstream to downstream, and a surface area of 113 km2 (Figure 1). The average rainfall in the wetland is 1,300 mm per year in a bi-modal pattern, with peaks in April-June and September-November. Ambient air temperature is in the range of 20–30°C. The wetland has an elevation range from 1,150 m upstream to 1,060 m downstream. The geology of the area comprises Pre-Cambrian rock, mainly granites (WMD, 2008). The wetland soils consist of clay loam in the central area and sandy loam in the swampy lowlands (WMD, 2008; Kayendeke and French, 2019). The major tributaries are three perennial streams, Nabuyonga, Nashibiso, and Nambale/Ndukwe, which receive water from other intermittent streams and ephemeral channels (Figure 1). In addition, the wetland receives surface water from two urban streams (Budaka and Nashibiso) draining the upstream catchment and the urban center of Mbale town, including the wastewater discharge from two sets of wastewater stabilization ponds (WSPs) operated by National Water and Sewerage Corporation (NWSC), Uganda.
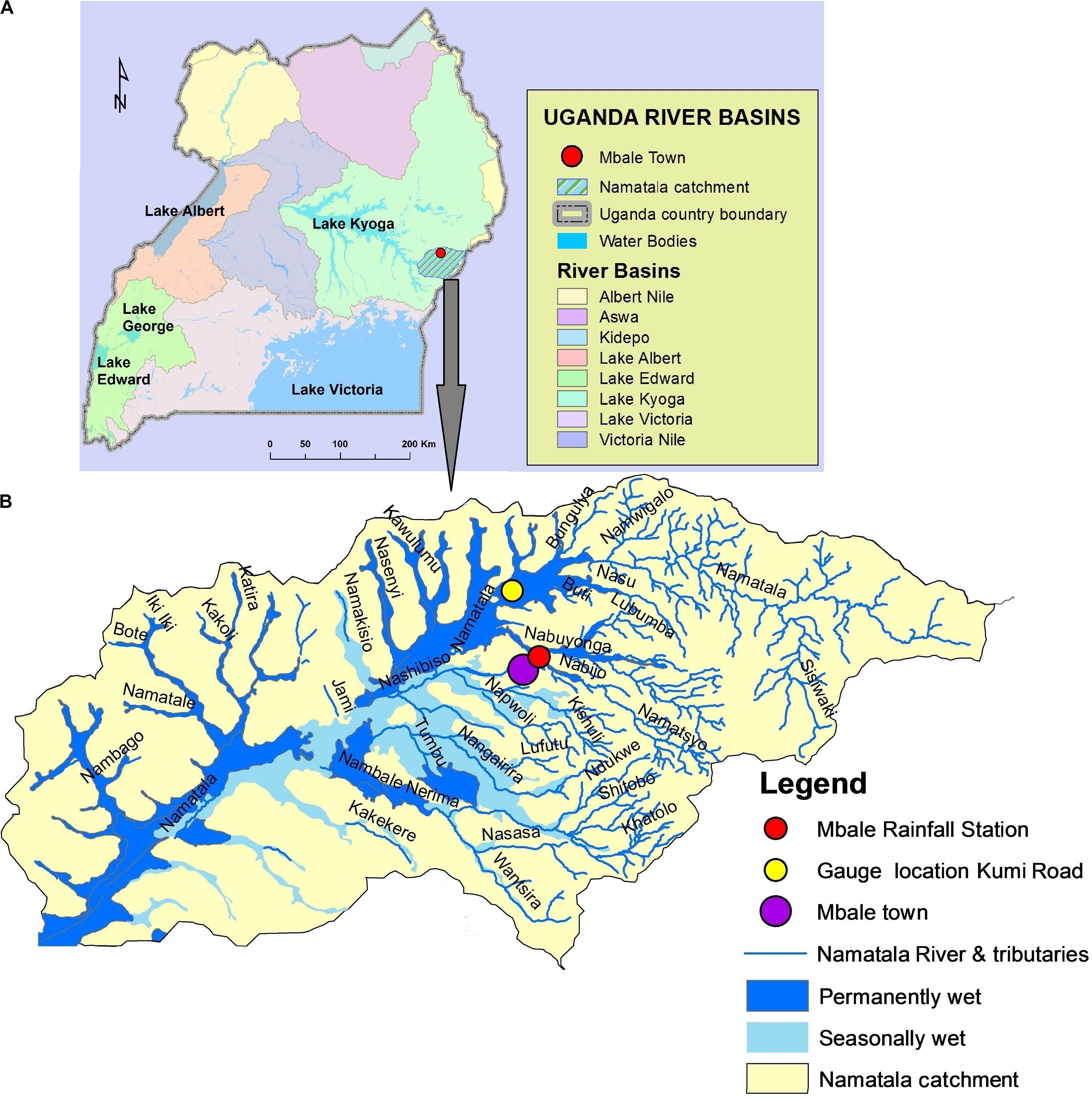
Figure 1. (A) Location of Mbale town and Namatala Wetland within Uganda; (B) Drainage system of Namatala Wetland. Data source: Mapping and Inventory Centre, National Forestry Authority Uganda, 2005. Maps produced by authors.
The wetland is located in a densely populated region (average 577 inhabitants km–2) within the districts of Mbale, Butaleja, Budaka, Bududa, Sironko, and Pallisa (UBOS, 2014). Mbale district has a population of 488,900, with 93,000 people residing in Mbale town which has an expanse of informal settlements along Namatala River and Nabuyonga, Budaka and Nashibiso streams (Cities Alliance, 2015). Open waste disposal is common within these settlements. Only 3.7% of the population is served by the sewer network. Because of old sewer mains, ingress of storm water in the pipe network is common, resulting in overloading of the WSPs. Final effluent has been reported to have a COD of 300 mg L–1, BOD of 180 mg L–1, and fecal coliforms of 26,000 CFU (100 mL)–1 (NWSC, 2017).
The population around the wetland depends on agriculture as the main source of livelihood. The extent of wetland area cleared for farming has been increasing over the years. In the upper part of the wetland, about 100 km2 was cleared for wetland farming between 1990 and 2010 (NFA, 2005; Namaalwa et al., 2013). Agricultural practices include surface drainage, soil compaction and removal of wetland vegetation. The original papyrus vegetation (Cyperus papyrus L.), which is known for its high productivity and storage capacity for nutrients (Kansiime et al., 2007; van Dam et al., 2014), has declined. This has created concerns about the impact of the land use change on sediment and nutrient retention in the wetland and on downstream water quality.
Currently, the seasonally wet zones in the upper wetland comprise subsistence agriculture with cultivation of rice, yams, maize, bananas, and sweet potatoes. The middle part of the wetland is used mainly for commercial rice farming in two seasons (February-July and August-January). Land preparation at the beginning of each season includes tillage, creation of channels and ditches for irrigation of the nursery beds, and construction of compacted dikes to ease access to the farms and regulate water flows. The wettest lower part of the wetland still has a considerable area with intact papyrus vegetation.
Field Sampling Design and Selection of Sampling Points
The wetland was divided into five hydrogeomorphic units (HGMUs) according to soil, hydrology and land use (Figure 2; Namaalwa et al., 2013). HGMUs 1 and 2 are located in the upstream part of the wetland, with a catchment that includes Mbale town and settlements in the neighboring areas of Mutoto, Bungokho, Bumageni, Nambale, and Nakaloke. HGMUs 1 and 2 have straightened river channels, compacted surface soils, high surface water flow rates, and low connectivity with, or inundation from, the main river. HGMU 3 is the mid-floodplain of the wetland, characterized by intensive rice farming. The major surface inflow into HGMU 3 is the Namatala River channel flowing out of HGMU 1 and 2. Within HGMU 3, intensive rice farming involves excessive tillage, tile drainage, diversion of surface water and high surface water flow rates. HGMUs 4 and 5 are permanently wet units in the downstream part of the wetland with moderate rice farming, some soil tillage in rice fields, and diversion of surface water flow. Partly intact wetland vegetation maintains a stronger wetland character compared with upstream HGMUs (see Table 1).
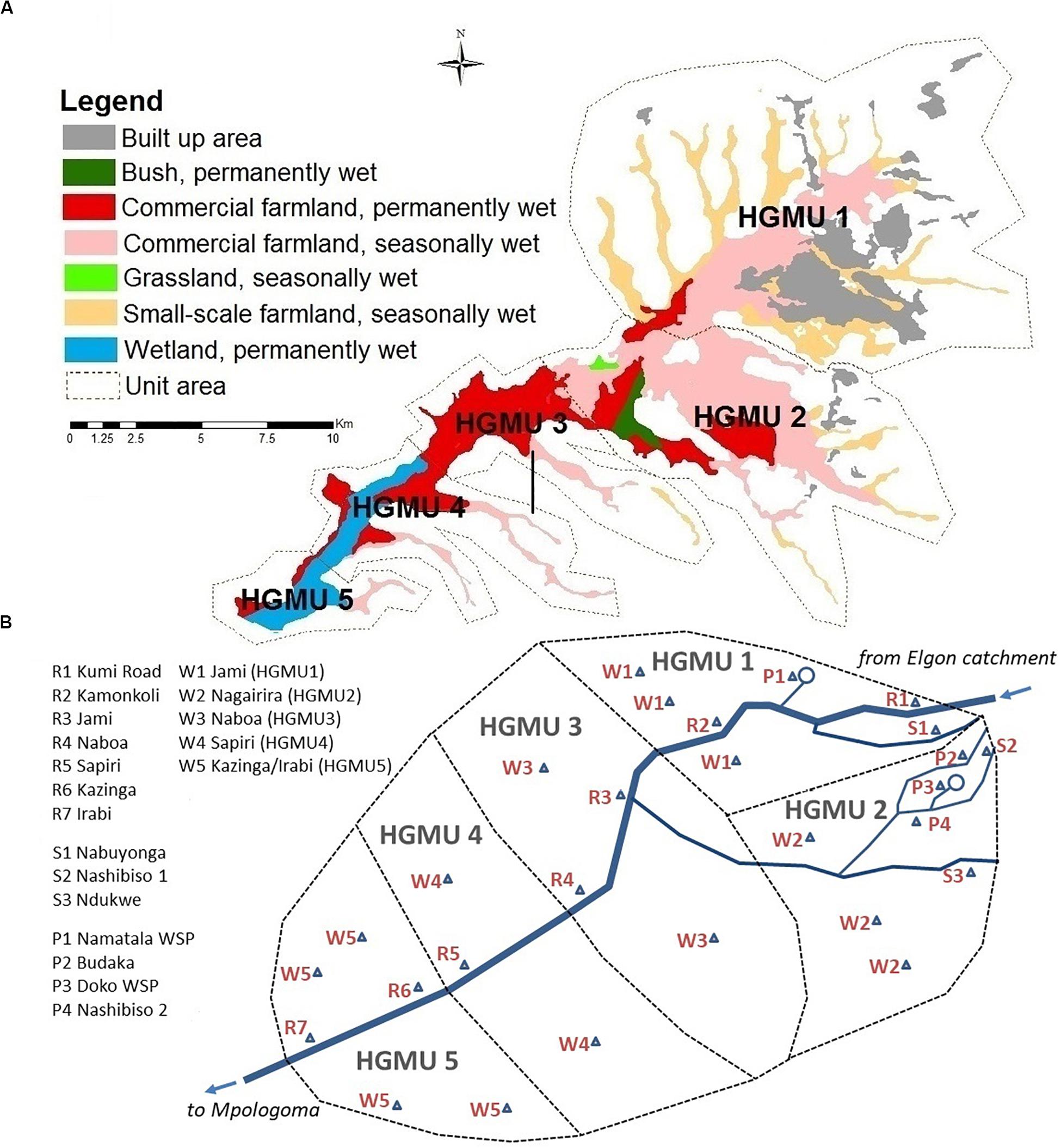
Figure 2. (A) Layout of hydrogeomorphic units (HGMUs); and (B) schematic map of sampling points in Namatala Wetland, Uganda. Inflow and outflow points for calculation of net yields were: HGMU 1: P1, R1, and S1 (in) and R2 (out); HGMU 3: R3 (in) and R4 (out); HGMU4: R4 (in) and R5 (out); and HGMU5: R5 (in) and R7 (out) (see legend for codes; R, river; S, rural streams; P, polluted streams; W, wetland). For HGMU 2, no net yield calculation was done because no outflow location was available. WSP, wastewater stabilization pond. For further explanation, see text. Data source for (A) Mapping and Inventory Centre, National Forestry Authority Uganda, 2005. Map produced by authors.
The wetland surface inflows were grouped into three categories, determined by the immediate catchment: (1) Namatala River, with seven sampling points (Kumi Road, Kamonkoli, Jami, Naboa, Sapiri, Kazinga, and Irabi) along the main river channel as it flows from upstream to downstream; (2) rural streams, Nabuyonga, Nashibiso-1, and Ndukwe, which are natural tributaries of Namatala River fed by smaller, often ephemeral streams; and (3) urban streams, also tributaries of the river, two of which (Budaka and Nashibiso-2) receive untreated domestic and industrial wastes from Mbale town, and the other two (Namatala WSP and Doko WSP channels) that discharge effluent from the two sets of WSP systems. A detailed layout and characteristics of the HGMUs are given, respectively, in Figure 2 and Table 1.
Precipitation and Discharge
Daily precipitation data recorded at the weather station in Mbale town, and discharge data recorded from the automatic gauge station (Figure 2) were obtained from the Directorate of Water Resources Management, Entebbe, for the period 2003–2016. Data was processed to generate mean monthly total rainfall and discharge. To estimate the contribution of groundwater to the wetland’s water resources, baseflow separation was carried out using the method developed by WMO (2008), and a 16 years long daily time series (period 2000–2015) of discharge at the Kumi Road gauge (Figure 2). The long-term average value of the Base Flow Index (BFI), which is the ratio of the cumulative volumes of baseflow and total discharge, was estimated. The quality of the groundwater was investigated by comparing the separated hydrograph with the monthly variations in water quality (especially nutrients) at Kumi Road.
Data to support calculation of discharge in the other sampling stations in Namatala River channel and in the tributary streams were collected monthly from April 2015 to October 2016. Flow velocity was measured at 25, 50, and 75% of the total stream width using a portable velocity flow meter with electromagnetic sensor (model FH950, Hach Company, Colorado, United States). At the same time, width and depth of the wetted perimeter were measured. Discharge was calculated using the velocity-area method (Herschy, 2009).
Water Quality
Samples for measuring water quality were collected monthly from April 2015 to October 2016 at the sampling points (Figure 2). For the river and tributaries, samples were taken using an alpha horizontal water sampler (Science First/Wildco, Yulee, Florida, United States) to make a composite sample from different depths (surface, middle and bottom). Because of the low water levels at the wetland points, grab samples were taken by filling the bottle beneath the surface of the water.
A photometric method was used to determine total suspended solids (TSS) of unfiltered samples using a spectrophotometer (DR 6000, Hach Company, Colorado, United States). Temperature, pH, electrical conductivity (EC), and dissolved oxygen concentration (DO) were measured in situ using a multi-parameter water quality meter with probe (Hydrolab Quanta, Hydrolab Corporation, Texas, United States). Water samples were placed in cool boxes kept at below 4°C and transported to the NWSC central laboratory in Bugolobi, Kampala, for analysis of nitrogen and phosphorus following standard methods (APHA, 2005). For nitrogen, ammonium (NH4-N; sodium salicylate and hypochloride method), nitrate (NO3-N; sodium salicylate method), nitrite (NO2-N; sulfanilamide and N-naphthyl-(1)-ethylendiamin-dihydrochlorid method), and total nitrogen (TN; alkaline persulfate digestion) were determined. For phosphorus, soluble reactive phosphorus (PO4-P; ascorbic acid method) and total phosphorus (TP; acidic persulfate digestion) were determined.
Sediment and Nutrient Loads in Surface Inflows
Total monthly sediment and nutrient loads at each river and stream sampling point were calculated by relating monthly discharge and water quality measurements to the river discharge measurements at the Kumi Road gauging station (based on Wollheim et al., 2005). First, we used regression equations to relate the measured discharge to corresponding discharge recorded at the gauging station for each sampling occasion. Regression equations (covering samples from the whole 19 month period, R2 in the range 0.82–0.97; Supplementary Table 3) were used to estimate discharge at each sampling point for every day of the sampling period (except for the months August-November 2015, when the gauging station was not working). Then, regressions were calculated between measured concentrations of TSS, TP and TN and measured discharge at each sampling point. These regressions (R2 in the range 0.71–0.96 for TSS; 0.80–0.93 for TP; 0.61–0.90 for TN; Supplementary Table 4) were then used to estimate daily concentrations of TSS, TN, and TP from the estimated daily discharge values at each sampling point. Finally, monthly TSS, TP, and TN loads (metric tonnes month–1) were calculated for all river and stream sampling points by multiplying daily discharge and concentration estimates, and summing for each month. The significant linear regressions across a wide range of discharge values with high R2-values provided confidence in these monthly load estimates.
Net Yields as Estimate of Sediment and Nutrient Retention
From the loads at each stream sampling point, net yields (in tonnes month–1km–2) were calculated for each month in HGMUs 1, 3, 4, and 5 as the difference between total incoming and outgoing load (based on the sampling points in the inlets and outlets of these HGMUs; see Figure 2), divided by the surface area of the HGMU (in km2), as follows:
HGMU1: [Load (Kumi Rd) + Load (NamatalaWSP) + Load (Nabuyonga) – Load(Kamonkoli)]/38.74
HGMU3: [Load (Jami) – Load (Naboa)]/13.87
HGMU4: [Load (Naboa) – Load (Sapiri)]/15.09
HGMU5: [Load (Sapiri) – Load (Irabi)]/6.79
The calculation could not be done for HGMU 2 because it did not have a suitable outflow point (Figure 2). Positive values of these net yields are interpreted as a measure of retention, whereas negative values suggest export of sediment or nutrients from the HGMU.
Data Analysis
To compare HGMUs and stream types, linear mixed effects ANOVA models were formulated using HGMU or stream type, month and their interaction as fixed variables, and individual sampling points as a random effect. The random effect accounted for the dependence of the repeated measurements (Everitt and Hothorn, 2010). Random intercept models were compared with models without the random component, and the model with the lowest AIC (Akaike Information Criterion) was selected. Mean values of water quality variables were compared among the HGMUs and among stream types (only the tributaries, as the river sampling points were not replicated) using least square means with Tukey adjustment. River water quality samples were compared from upstream to downstream using a non-parametric pairwise test (Wilcoxon signed rank test). Monthly net yields in HGMUs 1, 3, 4, and 5 were related to mean inflow into the respective HGMU using simple linear regression. All calculations were done using function wilcox.test for the signed rank test, function lme for estimating the mixed models, function lsmeans for post-hoc analysis, and function lm for the regression (for the load and net yield calculations), all part of R software version 3.3.1 (R Core Team, 2018). Significance is reported at p < 0.05, unless stated otherwise.
Results
Precipitation and Discharge
During the study period, precipitation ranged from almost zero in the very dry months of February and July of 2016 to peaks of 130-150 mm in May-June 2015, 230 mm in October 2015, and 160-170 mm in April-May 2016 (Figure 3). These rainfall peaks were reflected in the highest discharge observed in Namatala River between April and June, and between August and October in both years (Figure 4A). The highest discharge of about 22,000 m3 h–1 was recorded at Naboa in HGMU 3, and at Jami after the confluence of Namatala River with the Nabuyonga and Ndukwe streams (Figure 5). This discharge was about three times higher than that recorded at Kumi Road, the upstream point of the river. Maximum discharge in the tributaries was on average 1,000–1,100 m3 h–1 in the rural streams during the rainy periods in 2016. The urban streams had the lowest peak discharge, at 200–300 m3 h–1 (Figure 4B).
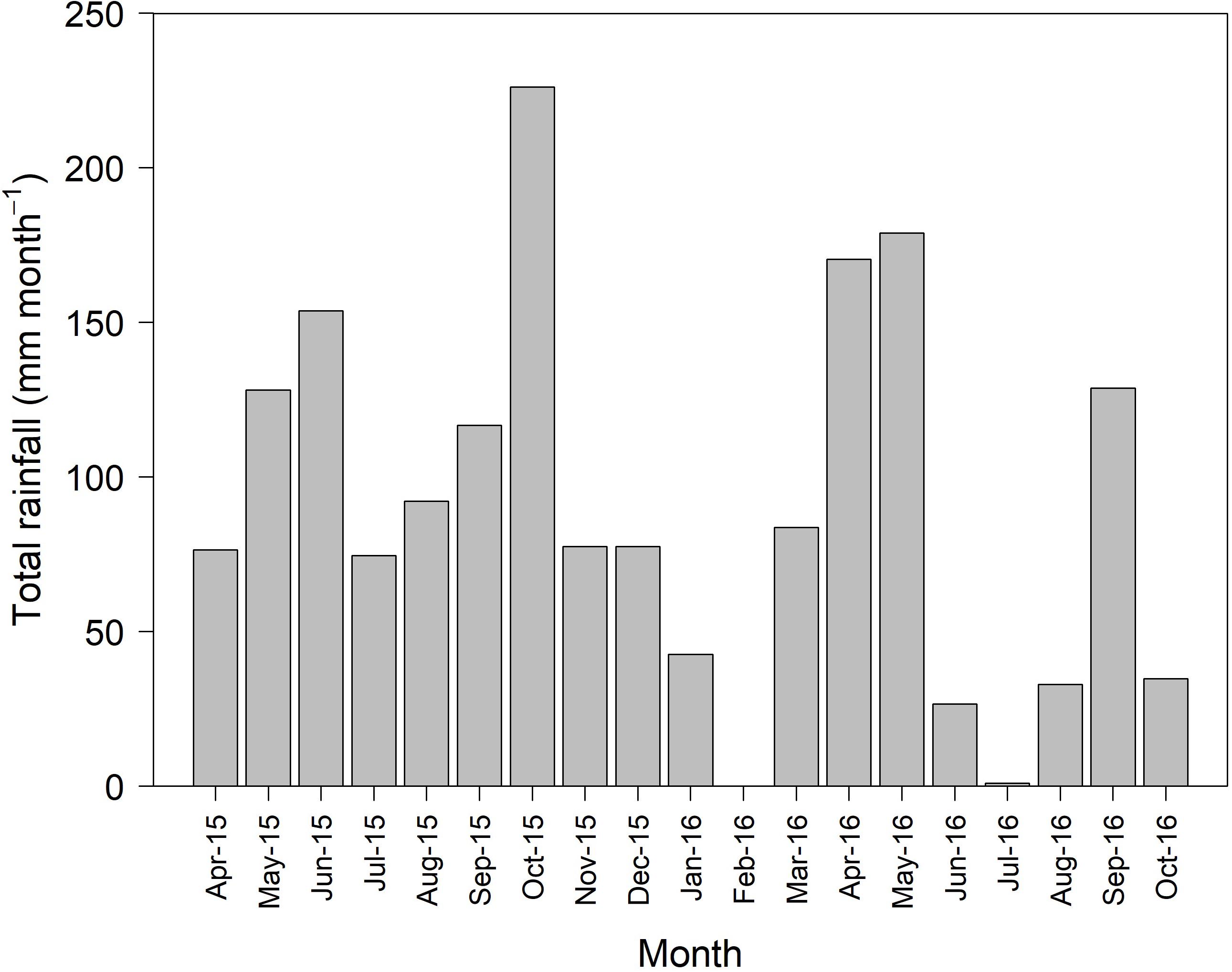
Figure 3. Total monthly rainfall at the weather station in Mbale, Uganda, during the study period April 2015–October 2016. No or very little rainfall occurred in the area in February and July 2016. Data source: Directorate of Water Resources Management, Entebbe.
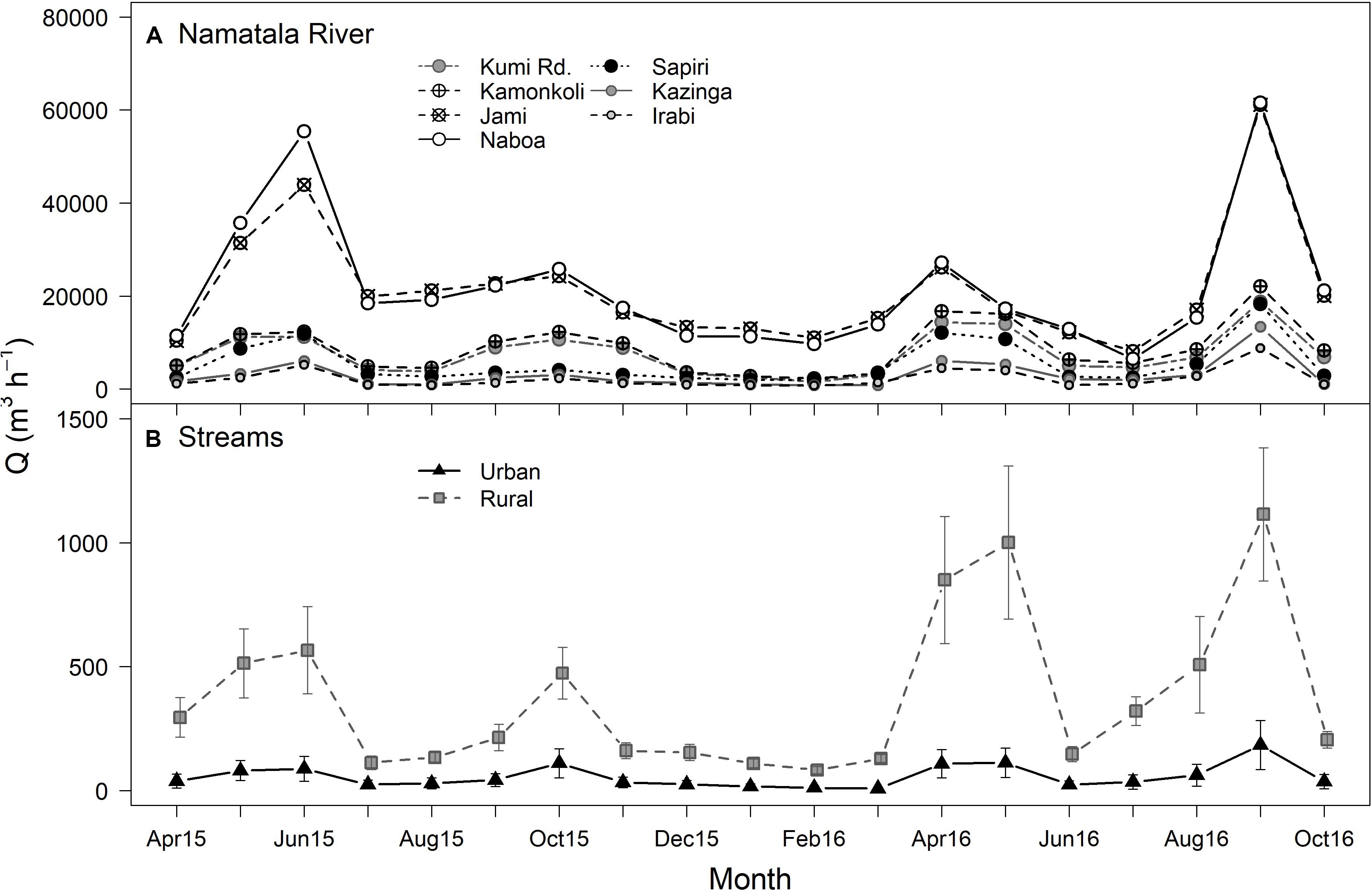
Figure 4. Discharge in m3 h–1 measured monthly in Namatala Wetland for the period April 2015–October 2016, with (A) seven sampling points in Namatala River; and (B) mean values for the four urban streams (n = 4) and three rural streams (n = 3). Error bars indicate standard error.
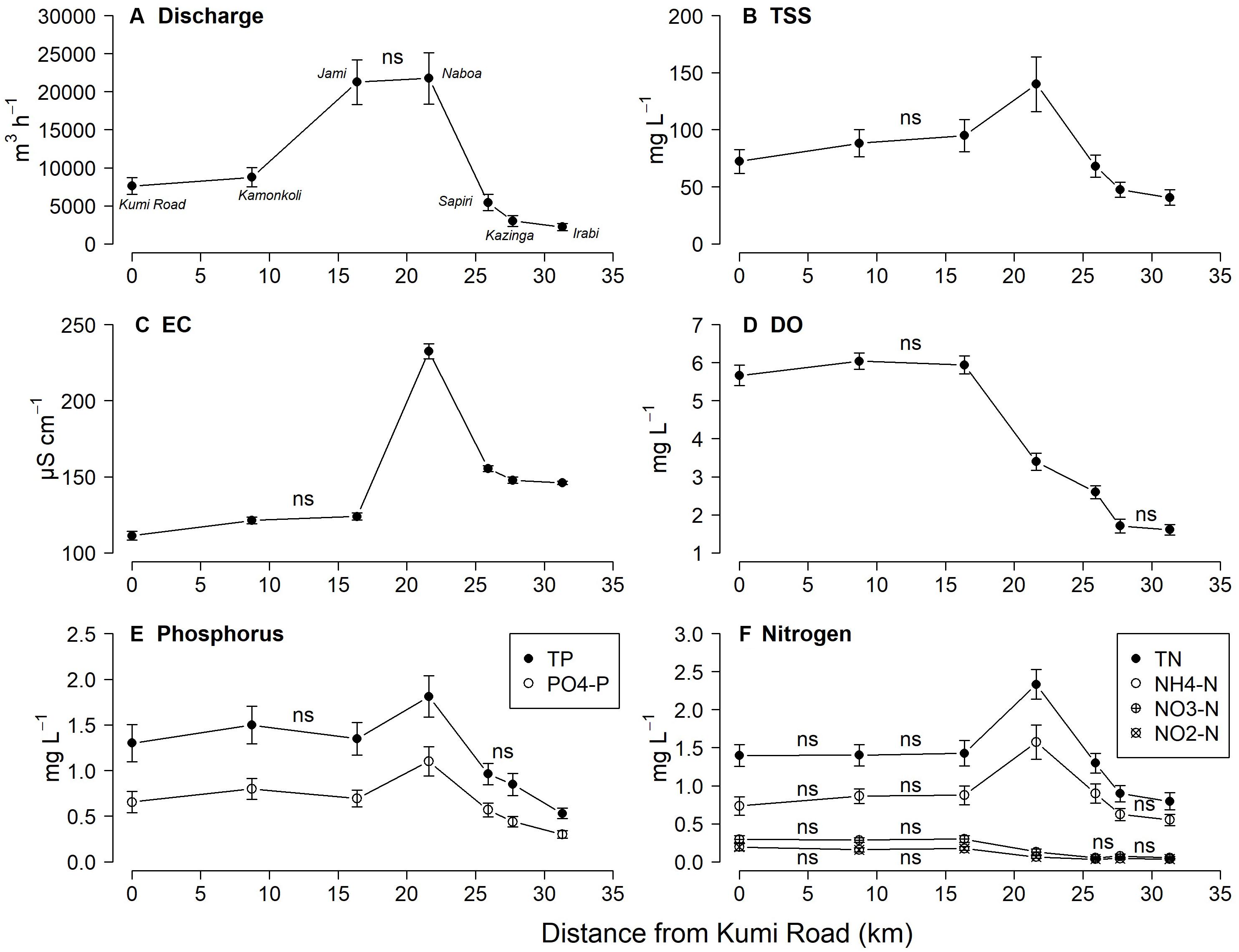
Figure 5. Variation of discharge and water quality in the main channel of Namatala River from upstream to downstream for the period April 2015 – October 2016. (A) Discharge; (B) Total suspended solids (TSS); (C) Electrical conductivity (EC); (D) Dissolved oxygen (DO); (E) Total and soluble reactive phosphorus; (F) Total nitrogen, and ammonium, nitrate and nitrite nitrogen. Names of the sampling points are indicated in panel (A). Data points are means of 19 monthly measurements; error bars indicate standard error. Differences between consecutive sampling points are significant (Wilcoxon signed rank test, P at least < 0.05) unless indicated by “ns” (not significant).
Figure 6 shows the separated hydrograph of Namatala River at Kumi Road for the experimental period, along with concentrations of nitrogen and phosphorus. The BFI value estimated for the Namatala River at Kumi Road was 0.605, which means that, in the long run, about 60% of the inflow into Namatala Wetland at Kumi Road originates from the groundwater of the upstream catchment. Peaks in nutrient concentrations coincided with waves of surface runoff which washed organic and inorganic substances into the river, mainly from agricultural lands. By contrast, nutrient concentrations were low during baseflow-dominated periods.
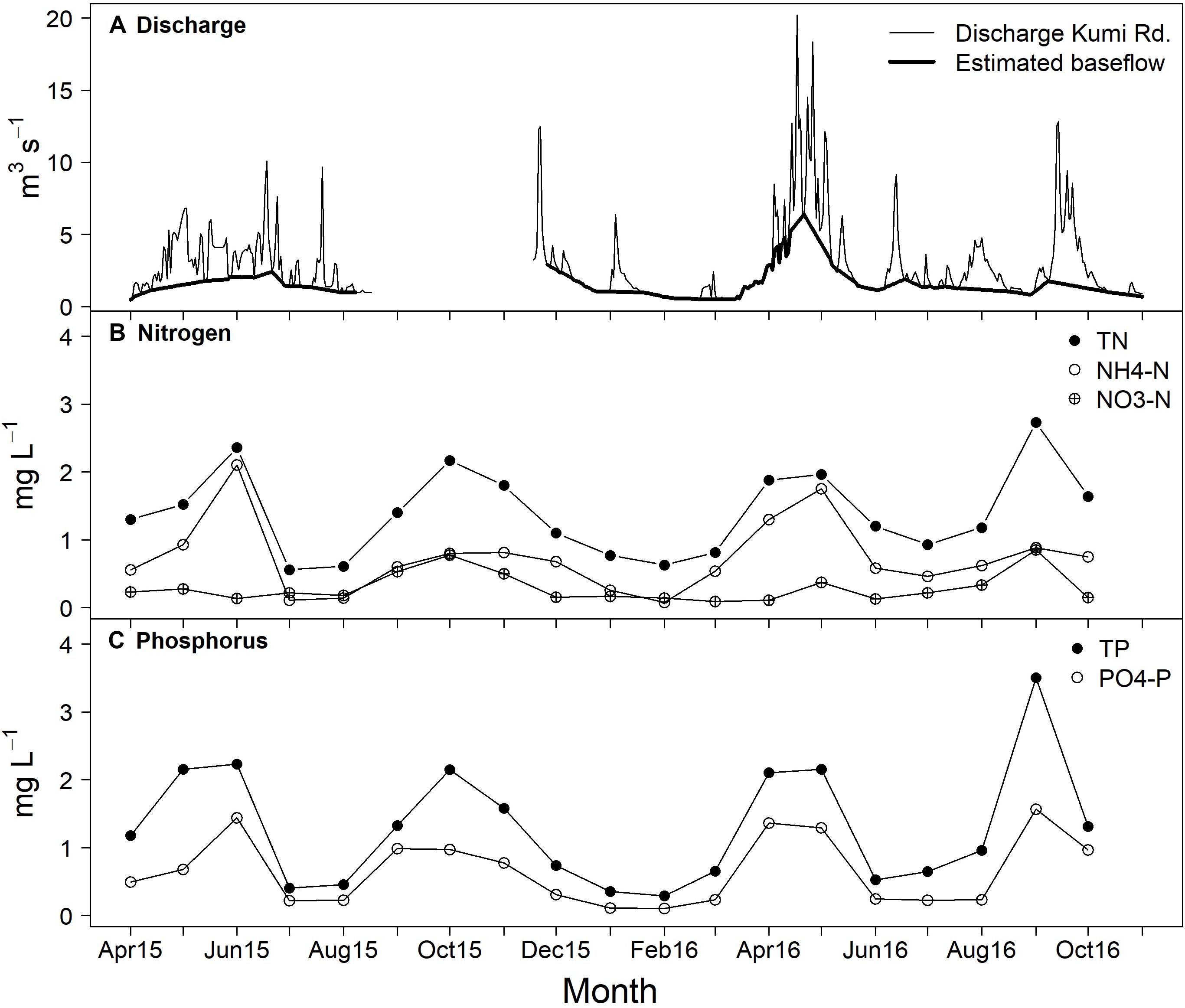
Figure 6. (A) Separation of baseflow for the Namatala River; (B) nitrogen concentration; and (C) phosphorus concentration; all at Kumi Road, Mbale, during the experimental period. Daily discharge data from the gauge at Kumi Road were used from the period 2000–2015 using the method from WMO (2008). Estimated Base Flow Index (BFI) for Namatala River was 0.605. Owing to a technical problem at the gauging station, there was a gap in discharge data in the period August–November 2015. For further explanation, see text.
Spatial and Temporal Water Quality Variation of Streams
Water quality in the Namatala River changed significantly from upstream to downstream sampling points, with the strongest changes observed from Jami to Naboa (the sampling point just downstream of the rice farming area in HGMU 3), and from Naboa to Sapiri (where the more natural part of the wetland starts). TSS and EC (Figures 5B,C) were significantly higher in Naboa than in Jami (upstream) and Sapiri (downstream). For nutrients (TP, PO4-P, TN, NH4-N), a similar significant peak in concentrations at Naboa occurred (Figures 5E,F). DO had the opposite pattern, with a significant drop from around 6 mg L–1 in Kamonkoli and Jami to 3.4 mg L–1 in Naboa, and further significant decreases in Sapiri and Kazinga (all comparisons Wilcoxon paired t-test, p < 0.001; see Supplementary Table 1). Nitrite (NO2) and nitrate (NO3) concentrations decreased significantly from Jami to Naboa and from Naboa to Sapiri.
Water quality in the tributaries exhibited clear differences among stream types and seasons (Figure 7), but with significant interactions between stream type and month for EC and nutrients (ANOVA; see Supplementary Table 2 for all means and variation). Mean EC over the 19-month sampling period was 111 and 235 μS cm–1 in river (Kumi Road station) and rural streams, respectively, with low seasonal variation. In the urban streams, EC was significantly higher (mean value 898 μS cm–1), with peaks in the dry seasons (July 2015, February 2016, and June-August 2016). Mean TSS ranged from 25 to about 250 mg L–1, with differences in mean TSS not significant among tributaries. Variation in TSS was mostly related to seasonal differences, with peaks in the periods of high discharge (May-June 2015, October 2015, April-May 2016, and September 2016). Similarly, nutrient concentrations (NH4-N, TN, PO4-P, and TP) showed seasonal peaks during the wet periods, with significantly higher nutrient concentrations in the urban streams. Mean DO concentrations were lowest in the urban streams (1.3 mg L–1), compared with the river (5.7 mg L–1 at Kumi Road station) and rural streams (3.2 mg L–1). pH was highest in urban streams (mean 8.4) and lowest in the river (mean 7.7). Mean temperature ranged from 24.7°C in rural streams, to 25.2°C in both the river and in urban streams. There was a slight seasonal range of 2–3°C (see Supplementary Table 6).
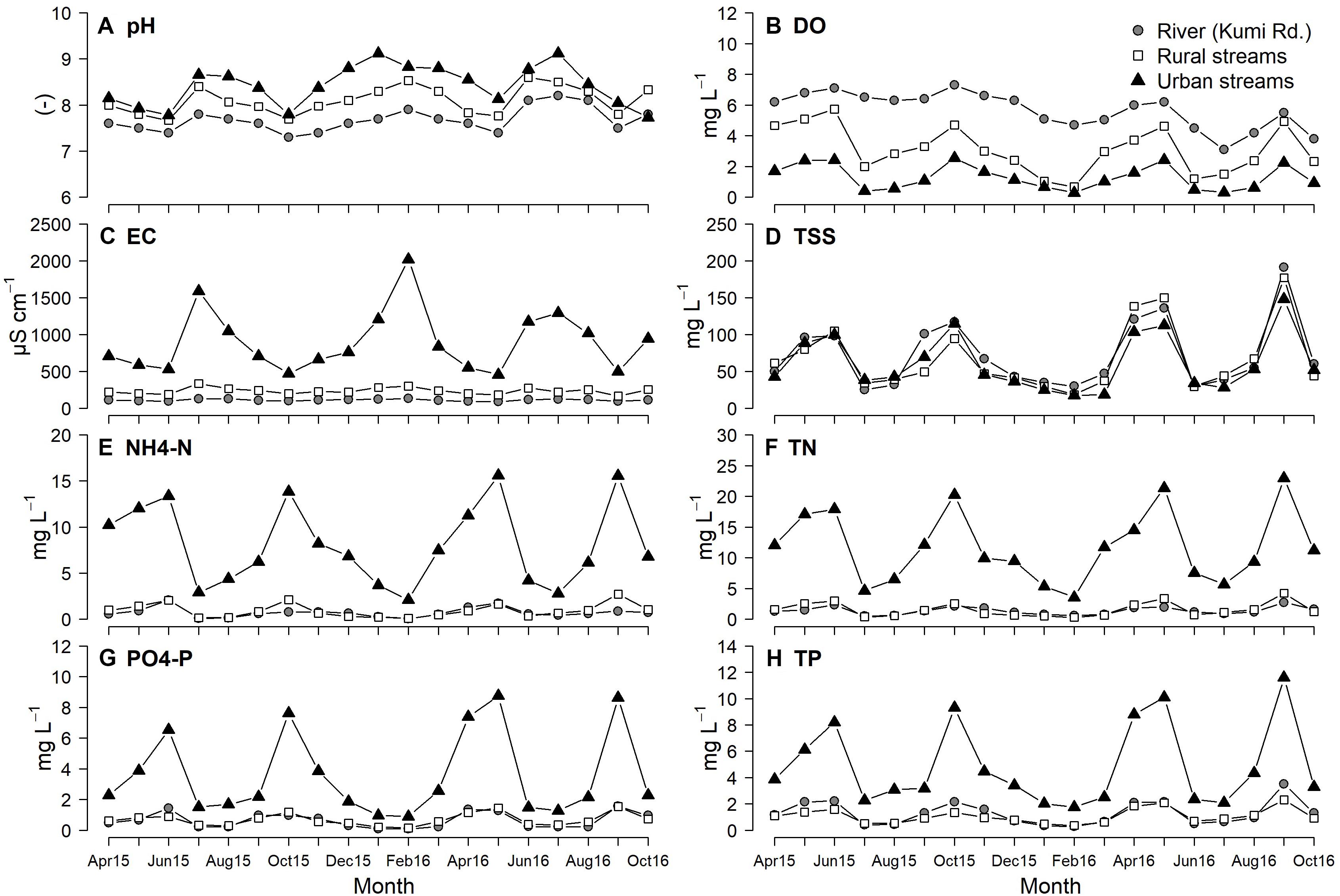
Figure 7. Variation of water quality parameters in Namatala River (at the Kumi Road sampling point), urban streams (mean value of four streams) and rural streams (mean value of three streams) of Namatala Wetland, Uganda, in the period April 2015–October 2016. (A) pH; (B) dissolved oxygen (DO); (C) electrical conductivity (EC); (D) total suspended solids (TSS); (E) ammonium nitrogen (NH4-N); (F) total nitrogen (TN); (G) soluble reactive phosphorus (PO4-P); (H) total phosphorus (TP).
Spatial and Temporal Water Quality Variation Among HGMUs
High spatial variation in DO, EC, pH, and TP was observed among the wetland units (Figure 8). Mean DO decreased significantly from upstream to downstream HGMUs, with highest DO in HGMU 1 and lowest values in HGMUs 4 and 5. For EC and TP, the lowest mean values were observed in HGMUs 1 and 5, and highest values in HGMU 3. TSS, pH and nutrients were significantly higher in HGMUs 3 and 4 than in the other HGMUs, except for N03-N which was significantly lower in HGMUs 3 and 4 than in the other HGMUs. In HGMUs 3, 4, and 5, total nitrogen consisted of NH4-N for about 70% of the samples, whereas in HGMUs 1 and 2 this was only 30%. A slight seasonal effect was observed with a decrease of pH in the wet seasons (April-June, October-December), possibly due to increased discharge. Fluctuations in EC were observed over the entire sampling period; however, values were consistently higher for HGMUs 2 and 3. EC values ranged from 174 to 243 μS cm–1 in HGMU 2 and from 180 to 338 μS cm–1 in HGMU 3. Within each HGMU, peak values of EC were recorded during the dry months (July-August, January-February). Mean temperatures were slightly lower in HGMU 4 (23.9°C) and 5 (23.8°C) and higher in HGMU 1 and 2 (25.0°C), with an average of 24.7°C for HGMU 3.
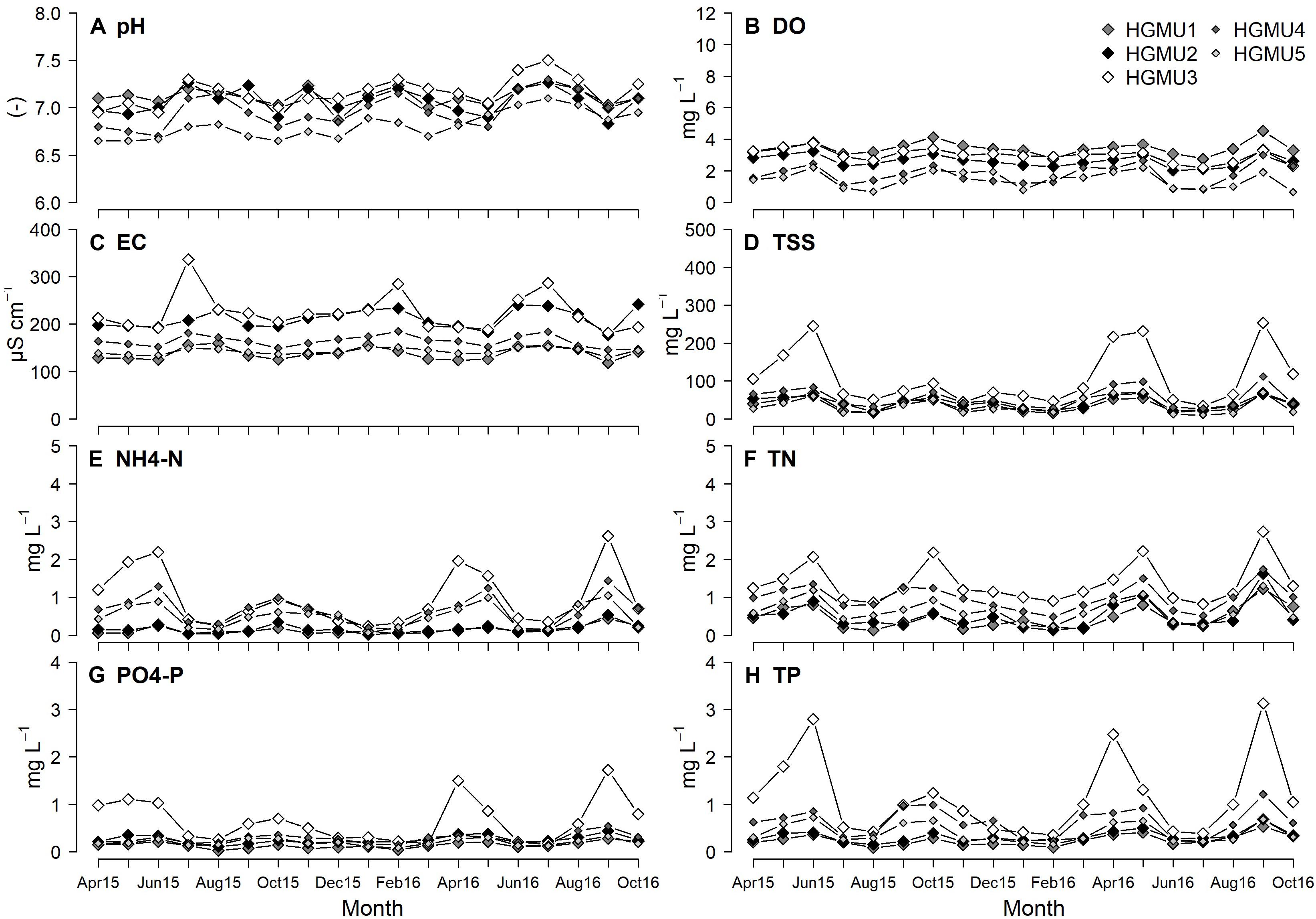
Figure 8. Seasonal variation of water quality parameters in HGMUs 1–5 of Namatala Wetland, for the period April 2015–October 2016. (A) pH; (B) dissolved oxygen (DO); (C) electrical conductivity (EC); (D) total suspended solids (TSS); (E) ammonium nitrogen (NH4-N); (F) total nitrogen (TN); (G) soluble reactive phosphorus (PO4-P); (H) total phosphorus (TP).
Sediment and Nutrient Loads
Seasonal trends were also observed in TSS, TP, and TN loads of all surface inflows with high loads during the peak rainfall period of April-June (especially in 2016), and a reduction in loads during other months of the year (Figure 9). The loads of TSS, TP and TN in the main river were one (TP, TN) to two (TSS) orders of magnitude greater compared with rural and urban streams, with the lowest loads recorded among urban streams for the entire period. Along the main river channel, TSS loads decreased from the upstream point (Kumi Road) through the mid-stream sub-catchment (Naboa, Sapiri) to the downstream points (Kazinga, Irabi). Nutrient (TN and TP) loads, however, were highest in Jami (after the confluence of Ndukwe stream with the river), and especially Naboa (just downstream of HGMU 3).
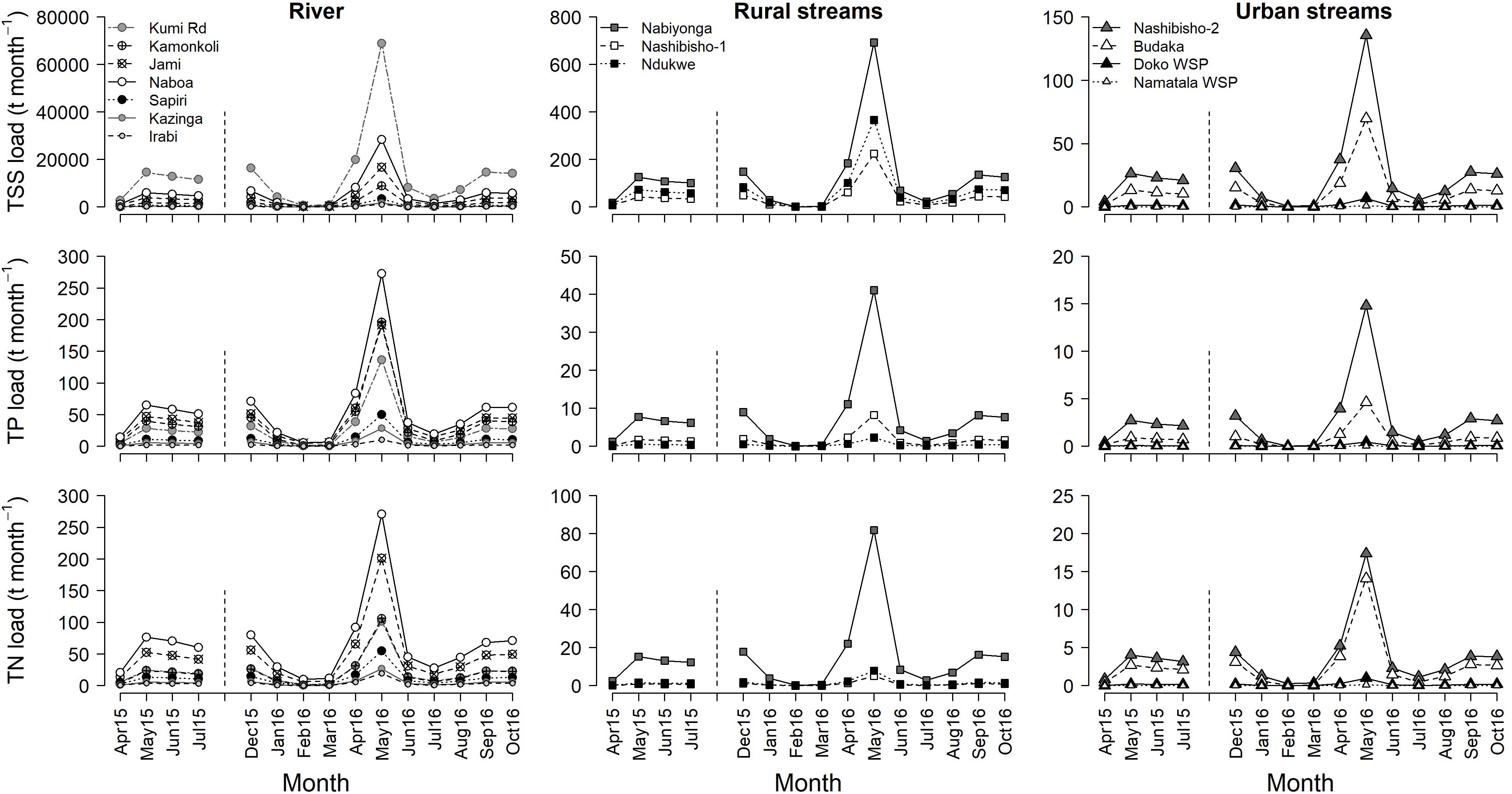
Figure 9. Total suspended solids (TSS), total phosphorus (TP), and total nitrogen (TN) loads (tonnes month–1) from the different streams of Namatala Wetland in the period April 2015–October 2016. For details of load calculations, see text. Loads between August and November 2015 could not be calculated because discharge data from the Kumi Road gauging station were not available.
Net Yields for HGMUs With Different Land Use
Positive net yields were observed mainly in downstream HGMUs 4 and 5, and negative to near-zero yields in the upstream HGMU 1 and midstream HGMU 3. The highest mean net TSS yields were found in HGMU 4 (331 t km–2 month–1) and HGMU 5 (72 t km–2 month–1), and the lowest in HGMU 3 (-160 t km–2 month–1). Similarly, mean net yields of TP and TN were highest in HGMU 4 (3.3 t km–2 month–1 for TP and 3.7 t km–2 month–1 for TN) and lowest in HGMU 3 (-1.3 t km–2 for TP and -1.5 t km–2 for TN). Net yields in HGMUs 4 and 5 showed significant positive linear relationships with mean monthly inflow (all R2 = 0.85 or higher), suggesting retention of sediment and nutrient in these downstream HGMUs. In HGMUs 1 and 3, this relationship had a significant negative slope suggesting export of sediment and nutrients in these highly converted HMGUs (except for net TN yield in HGMU1 where R2 = 0.38; Figure 10, see also Supplementary Table 5).
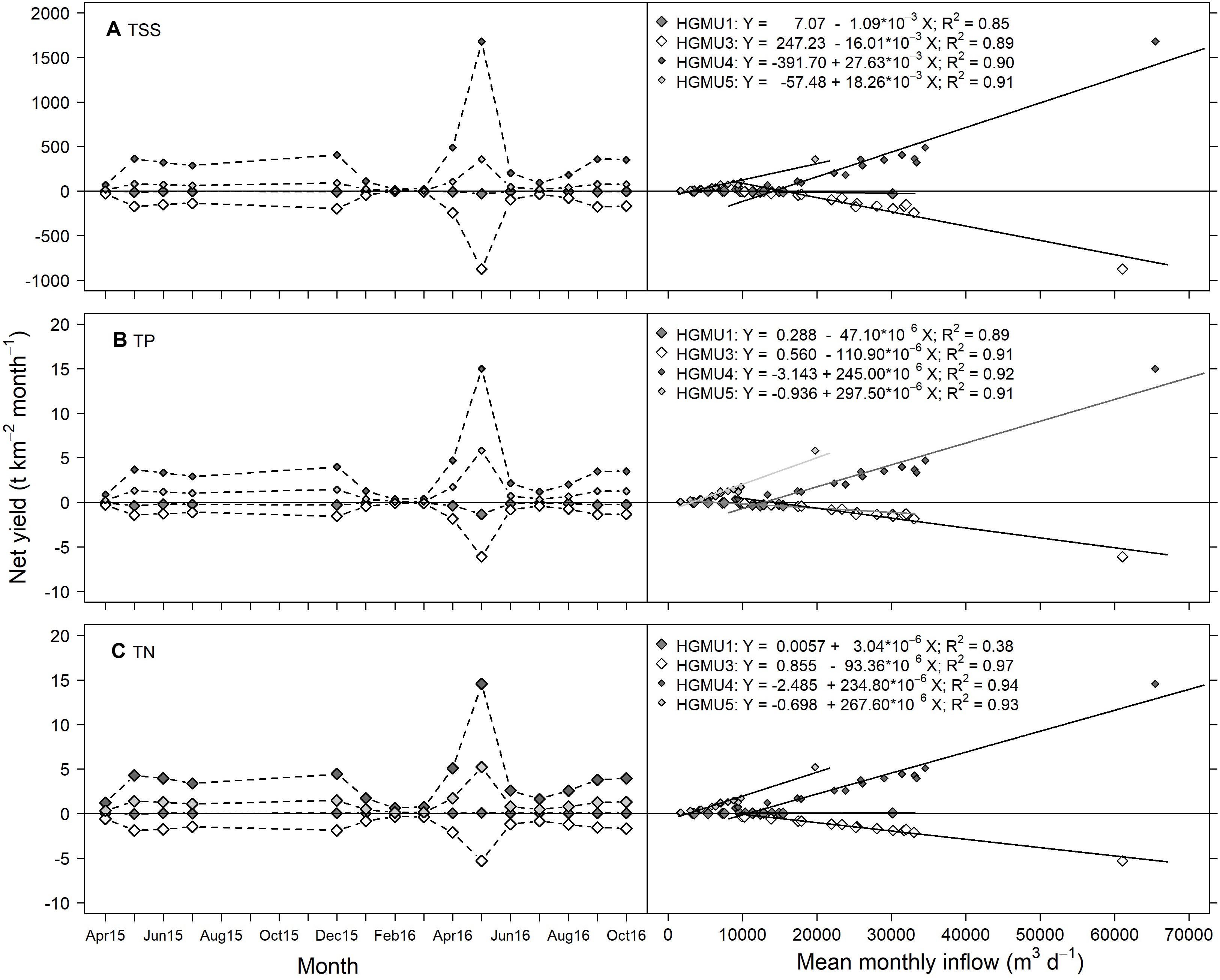
Figure 10. Net yield (tonnes km–2 month–1) (left panel), and regression of net yield (Y) on mean monthly inflow (X) (right panel). (A) Total suspended solids (TSS); (B) Total phosphorus (TP); (C) Total nitrogen (TN). Data are from the period April 2015–October 2016 in HGMUs 1, 3, 4, and 5 of Namatala Wetland. For further explanation, see text.
Discussion
Lake Kyoga and its connected wetlands drain the groundwater bodies underneath the surrounding hills and high grounds, and water flow in the opposite direction is unlikely (Owor et al., 2011; Kayendeke and French, 2019). The estimated BFI value in this study was practically identical to the BFI calculated for the Namatala River by Rugumayo and Ojeo (2006), who also calculated BFIs for other rivers in the Kyoga basin and derived similar, or even higher, values (e.g., BFI was 0.875 for Mpologoma River). The high BFI values mean that groundwater inflows from the hills and mountains into the wetlands are high compared with surface runoff inflows. To assess the influence of these groundwater inflows on surface water quality in the Namatala Wetland, we checked the water quality of the river during baseflow periods, such as February 2016. Concentrations of dissolved inorganic nutrients (NH4-N and PO4–P), as well as concentrations of TSS, TN, and TP were the lowest in this month compared with the rest of the monitoring period. This means that groundwater, although contributing significantly to the wetland’s water resources in quantitative terms, does not carry considerable amounts of nutrients into the wetland. The bulk of organic and inorganic nutrient inputs come with surface runoff during the flood periods, as shown by the coincidence of flood periods with the peaks of nutrient concentrations.
There were clear differences in water quality among the Namatala River and its rural and urban tributaries, with a strong influence from the different types of land use in the wetland. The highest TSS and nutrient concentrations and EC were observed in the urban streams in HGMUs 1 and 2, which also showed strong seasonal differences in EC and nutrient concentrations. This seasonal dynamic of nutrient flow from urban streams is influenced by the characteristics of their catchment. Open waste disposal, limited sewer network coverage, and storm water and sewage overflow during rainfall periods lead to overflow of nutrients into the streams. The runoff resulting from soil compaction, stream widening, diversion, and straightening in the upper catchment of Namatala Wetland mobilizes sediments and nutrients from impervious surfaces and farms into receiving streams (Recha et al., 2012; Uwimana et al., 2017). The presence of inorganic nitrogen (mainly NH4-N) and orthophosphate as main forms of TN and TP, respectively, is characteristic of the influence from the urban catchment, as shown in other studies on the impact of land use on water quality (Nyenje et al., 2010; Haidary et al., 2013). However, these higher concentrations in the urban streams did not have a strong impact on the nutrient concentrations after the confluence with the river in Jami, which were not significantly different from those in Kamonkoli. This was confirmed by the load calculations which showed much higher sediment and nutrient loads in the river than in the tributaries. While their impact on river water quality is limited, locally these polluted streams may lead to environmental and health risks, especially if they also carry high amounts of heavy metals or other contaminants (K’oreje et al., 2016; Sereyrath et al., 2016). With a growing urban population, the load from these streams may increase in the future. On the other hand, the riparian communities also utilize these nutrient-rich streams to cultivate maize and vegetables (Namaalwa, pers. obs.).
Compared with the polluted urban streams, the rice farming in HGMU 3 had a much stronger effect on sediment and nutrient concentrations and loads entering the river. Significantly higher sediment and nutrient concentrations and EC were observed in Naboa, downstream of HGMU 3, compared with sampling points upstream and downstream of Naboa. Also, DO concentration decreased significantly between Jami and Naboa, and concentrations of TN, TP, and TSS within HGMU 3 were significantly higher than in the other HGMUs. These high nutrient and sediment concentrations likely originate from the commercial rice farming in HGMU 3, which involves a high degree of tillage erosion and drainage that lead to elevated concentrations of TSS, accompanied by NH4-N and PO4-P that are known to adsorb to soil particles (Shore et al., 2015; Weigelhofer et al., 2018). Leaching from agricultural soil can also lead to the increase in EC and the lower DO concentrations at Naboa. It is also likely that fertilizers are applied in these rice farms, although interviews with farmers reported no use of fertilizers (Namaalwa, unpublished results). This can be expected to increase NH4-N and PO4-P concentrations from mineralization under the prevailing high temperatures, slightly alkaline pH and the alternate soil drying and wetting between seasons (Manguiat et al., 1996; Sahrawat, 2010).
The combination of land use in the wider catchment and agricultural practices within the wetland provide a complex seasonal dynamic, leading to loss of the retention function in the agricultural zones of Namatala Wetland. Increased discharge during the months of greater precipitation (March-June; Sept-November) increased the nutrient concentrations and loads from all surface inflows. Farming practices in HGMU 1 have permanently changed the ecological character of this area, which is now mostly disconnected from the river. Similar long term changes to surface water flow patterns caused by agriculture have been reported in headwater catchments in western Kenya (Recha et al., 2012). Plowing (between January and March) and weeding (in April and October) take place just before the onset of the rainy season, increasing sediment suspension and the release of sediment-bound nutrients into the water column. This results in a pulse of sediment and nutrients that is carried downstream. The modified agricultural zones (HGMUs 1 and 3) cannot retain this pulse. A similar synchronicity of seasonal rainfall and agricultural practices was observed in the Migina catchment in Rwanda (Uwimana et al., 2018).
Downstream of Naboa in HGMUs 4 and 5, sediment and nutrient concentrations in the river gradually decreased. Values in Irabi, the most downstream sampling point, were lower than those at the Kumi Road station. Here discharge was also much lower and, as the river connects laterally with the wetland, the water quality is typical of papyrus wetlands found elsewhere (Kansiime et al., 2007; van Dam et al., 2014). DO concentrations in these wetlands are low because of accumulation of organic matter from decaying vegetation, and pH decreases from accumulation of humic acids Whereas the decrease in DO concentration in the river after HGMU 3 can be seen as a negative impact of farming activities, the low DO in HGMUs 4 and 5 is partly a natural feature of the papyrus wetland.
The yield calculations show that HGMUs 4 and 5 retain sediment and nutrients, whereas HGMU 3 exports them. The net yields calculated for each HGMU can be interpreted as the retention of sediment or nutrients within that area. Because of the location of the sampling stations, it was not possible to calculate net yield for HGMU 2, and the calculations for the other HGMUs should be seen as crude indications for sediment and nutrient retention. Nevertheless, there were clear differences in net yield with HGMUs 4 and 5, showing mostly positive net yields throughout the year, while HGMU 3 generally had negative net yields. HGMU 1 had net yields close to 0. The significant regressions of net yield with discharge show that retention increased with higher discharge in HGMUs 4 and 5, while it decreased in HGMU 3. The positive slopes of these regressions in HGMUs 4 and 5 were all greater (about double for TSS and TP, and almost triple for TN) than the negative slopes in HGMU3, suggesting that retention capacity of the two downstream HGMUs still compensated for the increased export of sediment and nutrients from HGMU 3 during period with high discharge.
The retention function of the lower Namatala Wetland is likely the result of the intact lateral connectivity with the river, with high flows dispersing and slowing down in the downstream zone of the wetland, where there is ample opportunity for sedimentation, adsorption and uptake. Sediment and nutrient retention in floodplains and buffer zones are influenced by many factors, including length of the inundation period, hydraulic characteristics of the soil, relative contributions of groundwater and surface water, and characteristics of vegetation and microbial communities (Noe and Hupp, 2007; Weigelhofer et al., 2018). The retention of phosphorus is strongly linked to sedimentation of particulate matter (Shore et al., 2015), and in papyrus wetlands adsorption of phosphorus to sediment can be important (Kelderman et al., 2007). For nitrogen, sub-surface transport and nitrification-denitrification are generally more important pathways (Pärn et al., 2012; Weigelhofer et al., 2018) although in Namatala Wetland, NH4-N adsorption to sediment may also play a role. In headwater streams, nitrification of NH4-N and uptake of NO3-N by benthic periphyton dominate N removal (Peterson et al., 2001). In more downstream river reaches impacted by agriculture, the relative importance of coupled nitrification-denitrification is, however, likely reduced (Kemp and Dodds, 2002). In Namatala Wetland, most of the TN consisted of NH4-N, which is more likely to be taken up by the papyrus vegetation and accumulate in peat than to be removed by nitrification (Hes and van Dam, 2019). Nevertheless, NO3-N concentrations also decreased toward the downstream stations. Partly this could reflect uptake by biota in the wetlands, but the conditions for denitrification are good with low DO and high organic matter availability. Experiments on potential denitrification in Namatala Wetland showed much higher potential in the intact wetland zones than in agriculturally converted soils (Namaalwa et al., unpublished results).
Results from this study demonstrate that the Namatala Wetland delivers important regulating ecosystem services for the downstream Mpologoma River and the Lake Kyoga catchment. However, this function of the wetlands is at risk. The connectivity and integrity of the downstream wetland zone are threatened by encroachment and further development of agriculture, accompanied by overharvesting and removal of papyrus. Similar developments have been observed in the East African region (Maclean et al., 2013; Bateganya et al., 2015; Uwimana et al., 2018). Future management strategies of Namatala Wetland should include sustainable agriculture in the upper wetland, including introduction of buffer strips along the main river channel, conservation of the remaining papyrus swamp and its connectivity with the river in the lower wetland, and improved wastewater treatment (including integrated nutrient management) around Mbale town. Options for reducing erosion and nutrient runoff caused by rice farming in HGMU 3 should be considered. Support among stakeholders for integrated management solutions and more sustainable farming practices seems to be present (Zsuffa et al., 2014) and can be used, together with the results of this study, to strengthen wetland management planning for Namatala Wetland and similar wetlands in Uganda.
Land use change has a strong impact on landscape sediment and nutrient retention (Burt and Pinay, 2005; Jung et al., 2008; Uwimana et al., 2018). This study shows that the agricultural and urban development pressure in the upstream zones of the wetland can be mitigated to a large extent by the natural functioning of the downstream zones of the wetland ecosystem. This delicate balance between economic development and wetland protection is representative of many wetlands in Africa and other parts of the world, and determining a sustainable level of wetland use is a challenge for many wetland managers and governments (Ramsar Convention on Wetlands, 2018). Detailed knowledge of water and nutrient flows in relation to natural variation, local conditions and land use is important, but more studies on rivers and wetlands in Africa are needed. Besides water quality regulation, other ecosystem services such as fish production, tourism, and biodiversity conservation should be taken into account. Practical methods for quantifying different ecosystem services and determining optimum management strategies are needed (Wood et al., 2013; Zsuffa et al., 2014). A lot of useful information could be obtained from regular monitoring of hydrology and water quality, but this is often lacking. Economic valuation studies show that the monetary value of water quality regulation by wetlands often exceeds the value of the provisioning services (Emerton et al., 1999; Russi et al., 2013). Allowing agricultural and urban development to gradually replace natural wetlands is, therefore, also economically undesirable. Lost regulating services need to be replaced through capital investment in water treatment facilities.
Conclusion
Urban streams had significantly higher concentrations of sediment and nutrients than the Namatala River and its tributaries. However, the largest loads of sediment and nutrients were carried into the wetland by the Namatala River. The largest nutrient loads were observed downstream of the main rice growing area in the central part of the wetland. Sediment and nutrient loads were strongly related to seasonal variation in rainfall and river discharge, and to the corresponding peaks in agricultural practices. Peak loads coincided with the rainy seasons and consisted of accumulated material from densely populated urban areas, as well as sediment and nutrients from intensively cultivated parts of the wetland. The capacity for sediment and nutrient retention was 2–3 times higher in the downstream part of the wetland with more intact papyrus vegetation and lateral hydrological connectivity. Retention in the downstream part of the wetland increased at higher river flow rates. On balance, the sediment and nutrient retention in the intact parts of Namatala Wetland absorbed the export from the converted parts. It can be concluded that Namatala Wetland still performs its sediment and nutrient regulating ecosystem services in the Lake Kyoga catchment. However, further conversion to agriculture puts this function of the wetland at risk. To maintain the regulating ecosystem services of Namatala Wetland in the future, improved wastewater treatment, sustainable agricultural practices and conservation of the remaining intact wetland zone are needed. Detailed knowledge of the sediment and nutrient retention processes can be used for determining trade-offs in sustainable wetland management.
Data Availability Statement
The datasets generated for this study are available on request from the corresponding author.
Author Contributions
SN designed the research, performed field and laboratory measurements, and took the lead in writing the manuscript. AD and GG contributed to research design, data analysis, and manuscript editing. IZ advised on the hydrological aspects and contributed the hydrograph separation analysis. All co-authors provided research guidance and critical reviews of the manuscript.
Funding
This study was funded by the EU-FP7 WETwin project (project no. 212300), the Netherlands Fellowship Programme (NFP) through Nuffic (grant no. CF9174/2013), and the International Foundation for Science (IFS Sweden, individual grant no. W5416).
Conflict of Interest
IZ was employed by VITUKI Hungary Plc., Budapest, in addition to his affiliation to National University of Public Service, Budapest Hungary.
The remaining authors declare that the research was conducted in the absence of any commercial or financial relationships that could be construed as a potential conflict of interest.
Acknowledgments
We would like to thank the Directorate of Water Resources Management (DWRM), Entebbe, Uganda, for the river discharge, water level and rainfall data. Special appreciation is expressed to the NWSC Central Laboratory Bugolobi for the space and laboratory equipment provided during the research. Special thanks to the field assistants Bright Twesigye, Robert Katungi, and Aloysius Bukenya.
Supplementary Material
The Supplementary Material for this article can be found online at: https://www.frontiersin.org/articles/10.3389/fenvs.2020.00148/full#supplementary-material
References
APHA (2005). Standard Methods for the Examination of Water and Wastewater, 21st Edn, Washington, DC: Centennial Edition.
Baker, M. E., Weller, D. E., and Jordan, T. E. (2006). Improved methods for quantifying potential nutrient interception by riparian buffers. Landsc. Ecol. 21, 1327–1345. doi: 10.1007/s10980-006-0020-0
Bateganya, N. L., Nakalanzi, D., Babu, M., and Hein, T. (2015). Buffering municipal wastewater pollution using urban wetlands in sub-Saharan Africa: a case of Masaka municipality, Uganda. Environ. Technol. 36, 2149–2160. doi: 10.1080/09593330.2015.1023363
Biggs, E. M., Bruce, E., Boruff, B., Duncan, J. M., Horsley, J., Pauli, N., et al. (2015). Sustainable development and the water-energy-food nexus: a perspective on livelihoods. Environ. Sci. Policy 54, 389–397. doi: 10.1016/j.envsci.2015.08.002
Burt, T. P., and Pinay, G. (2005). Linking hydrology and biogeochemistry in complex landscapes. Prog. Phys. Geogr. 29, 297–316. doi: 10.1191/0309133305pp450ra
Cities Alliance (2015). Mbale City Profiling Report. Cities Alliance Project Report in Partnership With Uganda Slum Dwellers Federation, ACTogether-Uganda and the Municipality of Mbale. Brussels: Cities Alliance.
Davidson, N. C. (2014). How much wetland has the world lost? Long-term and recent trends in global wetland area. Mar. Freshw. Res. 65, 934–941. doi: 10.1071/MF14173
Díaz, S., Demissew, S., Carabias, J., Joly, C., Lonsdale, M., Ash, N., et al. (2015). The IPBES conceptual framework—connecting nature and people. Curr. Opin. Environ. Sustain. 14, 1–16.
Dini, J. A., and Everard, M. (2018). “National wetland policy: South Africa,” in The Wetland Book Vol. 1: Wetland Structure And Function, Management, and Methods, eds N. C. Davidson, B. Middleton, R. McInnes, M. Everard, K. Irvine, A. A. van Dam, et al. (Dordrecht: Springer), 795–800. doi: 10.1007/978-90-481-9659-3_157
Emerton, L., Iyango, L., Luwum, P., and Malinga, A. (1999). The Present Economic Value of Nakivubo Urban Wetland, Uganda. Kampala: IUCN.
Everitt, B. S., and Hothorn, T. (2010). A Handbook Of Statistical Analyses Using R, 2nd Edn, Boca Raton, FL: CRC Press.
Gardner, R. W., Connolly, K. D., and Bamba, A. (2009). African wetlands of international importance: assessment of benefits associated with designations under the Ramsar convention. Georgetown Intern. Environ. Law Rev. 21, 257–294.
Haidary, A., Amiri, B. J., Adamowski, J., Fohrer, N., and Nakane, K. (2013). Assessing the impacts of four land use types on the water quality of wetlands in Japan. Water Resour. Manag. 27, 2217–2229. doi: 10.1007/s11269-013-0284-5
Hes, E. M. A., and van Dam, A. A. (2019). Modelling nitrogen and phosphorus cycling and retention in Cyperus papyrus dominated natural wetlands. Environ. Model. Softw. 122:104531. doi: 10.1016/j.envsoft.2019.104531
Jung, K. W., Lee, S. W., Hwang, H. S., and Jang, J. H. (2008). The effects of spatial variability of land use on stream water quality in a coastal watershed. Paddy Water Environ. 6, 275–284. doi: 10.1007/s10333-008-0122-1
Kansiime, F., and Nalubega, M. (1999). Natural treatment by Uganda’s Nakivubo swamp. Water Q. Intern. 1999, 29–31.
Kansiime, F., Saunders, M. J., and Loiselle, S. A. (2007). Functioning and dynamics of wetland vegetation of Lake Victoria: an overview. Wetlands Ecol. Manag. 15, 443–451. doi: 10.1007/s11273-007-9043-9
Kayendeke, E., and French, H. K. (2019). “Characterising the hydrological regime of a tropical papyrus wetland in the Lake Kyoga Basin, Uganda,” in Agriculture and Ecosystem Resilience in Sub Saharan Africa, Climate Change Management, ed. Y. Bamutaze (Cham: Springer).
Kelderman, P., Kansiime, F., Tola, M. A., and van Dam, A. A. (2007). The role of sediments for phosphorus retention in the Kirinya wetland (Uganda). Wetlands Ecol. Manag. 15, 481–488. doi: 10.1007/s11273-007-9048-4
Kemp, M. J., and Dodds, W. K. (2002). Comparisons of nitrification and denitrification in prairie and agriculturally influenced lotic ecosystems. Ecol. Appl. 12, 998–1009. doi: 10.1890/1051-0761(2002)012[0998:conadi]2.0.co;2
K’oreje, K. O., Vergeynst, L., Ombaka, D., De Wispelaere, P., Okoth, M., Van Langenhove, H., et al. (2016). Occurrence patterns of pharmaceutical residues in wastewater, surface water and groundwater of Nairobi and Kisumu city, Kenya. Chemosphere 149, 238–244. doi: 10.1016/j.chemosphere.2016.01.095
Lohse, K. A., Brooks, P. D., McIntosh, J. C., Meixner, T., and Huxman, T. E. (2009). Interactions between biogeochemistry and hydrologic systems. Annu. Rev. Environ. Resourc. 34, 65–96. doi: 10.1146/annurev.environ.33.031207.111141
Maclean, I. M. D., Bird, J. P., and Hassall, M. (2013). Papyrus swamp drainage and the conservation status of their avifauna. Wetlands Ecol. Manag. 22, 115–127. doi: 10.1007/s11273-013-9292-8
Mafabi, P. (2000). The role of wetland policies in the conservation of water birds: the case of Uganda. Ostrich 71, 96–98. doi: 10.1080/00306525.2000.9639880
Mafabi, P. (2018). “National wetland policy: Uganda,” in The Wetland Book: I: Structure and Function, Management and Methods, ed. C. Finlayson (Dordrecht: Springer), 807–812. doi: 10.1007/978-90-481-9659-3_154
Manguiat, I. J., Watanabe, I., Mascarina, G. B., and Tallada, J. G. (1996). N Mineralization in Tropical Wetland Rice Soils. I. relationship with temperature and soil properties. Soil Sci. Plant Nutr. 42, 229–238.
Namaalwa, S., van Dam, A. A., Funk, A., Guruh Satria, A., and Kaggwa, R. C. (2013). A characterization of the drivers, pressures, ecosystem functions and services of Namatala Wetland, Uganda. Environ. Sci. Policy 34, 44–57. doi: 10.1016/j.envsci.2013.01.002
NFA (2005). National Biomass Study Report 2005. Kampala: Mapping and Inventory Centre, National Forestry Authority.
Noe, G. B., and Hupp, C. R. (2007). Seasonal variation in nutrient retention during inundation of a short-hydroperiod floodplain. River Res. Appl. 23, 1088–1101. doi: 10.1002/rra.1035
NWSC (2017). Water Quality Annual Report 2016/2017. Uganda: National Water and Sewerage Corporation.
Nyenje, P. M., Foppen, J. W., Uhlenbrook, S., Kulabako, R., and Muwanga, A. (2010). Eutrophication and nutrient release in urban areas of sub-Saharan Africa: a review. Sci. Total Environ. 408, 447–455. doi: 10.1016/j.scitotenv.2009.10.020
OECD/FAO (2016). Agriculture in Sub-Saharan Africa: Prospects and Challenges for the Next Decade, in OECD-FAO Agricultural Outlook 2016-2025. Paris: OECD Publishing.
Ostrovskaya, E., Douven, W., Schwartz, K., Pataki, B., Mukuyu, P., and Kaggwa, R. C. (2013). Capacity for sustainable management of wetlands: lessons from the WETwin project. Environ. Sci. Policy 34, 128–137. doi: 10.1016/j.envsci.2012.08.006
Owor, M., Taylor, R., Mukwaya, C., and Tindimugaya, C. (2011). Groundwater/surface-water interactions on deeply weathered surface of low relief: evidence from Lakes Victoria and Kyoga, Uganda. Hydrogeol. J. 19, 1403–1420. doi: 10.1007/s10040-011-0779-1
Park, S. R., Lee, H. J., Lee, S. W., Hwang, S. J., Byeon, M. S., Joo, G. J., et al. (2011). Relationships between land use and multi-dimensional characteristics of streams and rivers at two different scales. Intern. J. Limnol. 47, 107–116.
Pärn, J., Pinay, G., and Mander, Ü (2012). Indicators of nutrient transport from agricultural catchments under temperate climate: a review. Ecol. Indic. 22, 4–15. doi: 10.1016/j.ecolind.2011.10.002
Peterson, B. J., Wollheim, W. M., Mulholland, P. J., Webster, J. R., Meyer, J. L., Tank, J. L., et al. (2001). Control of nitrogen export from watersheds by headwater streams. Science 292, 86–90. doi: 10.1126/science.1056874
R Core Team (2018). R: A Language and Environment for Statistical Computing. Vienna: R Foundation for Statistical Computing.
Ramsar Convention on Wetlands (2018). Global Wetland Outlook: State of the World’s Wetlands and Their Services to People. Gland: Ramsar Convention Secretariat.
Recha, J. W., Lehmann, J., Walter, M. T., Pell, A., Verchot, L., and Johnson, M. (2012). Stream discharge in tropical headwater catchments as a result of forest clearing and soil degradation. Earth Interact. 16, 1–18. doi: 10.1175/2012EI000439.1
Rugumayo, A. I., and Ojeo, J. (2006). “Low flow analysis in Lake Kyoga basin Eastern Uganda,” in Proceedings of the International Conference on Advances in Engineering and Technology, eds J. A. Mwakali, and G. Taban-Wani (Amsterdam: Elsevier), 739–755. doi: 10.1016/b978-008045312-5/50078-9
Russi, D., ten Brink, P., Farmer, A., Badura, T., Coates, D., Förster, J., et al. (2013). The Economics of Ecosystems and Biodiversity for Water and Wetlands. Brussels: Ramsar Secretariat.
Sahrawat, K. L. (2010). Nitrogen mineralization in lowland rice soils: the role of organic matter quantity and quality. Archiv. Agron. Soil Sci. 56, 337–353. doi: 10.1080/03650340903093158
Sereyrath, L., Irvine, K. N., Murphy, T. P., and Wilson, K. (2016). Sediment-associated metals levels along the sewer-natural treatment wetland continuum, Phnom Penh, Cambodia. Urban Water J. 13, 819–829. doi: 10.1080/1573062x.2015.1036088
Shore, M., Jordan, P., Mellander, P. E., Kelly-Quinn, M., and Melland, A. R. (2015). An agricultural drainage channel classification system for phosphorus management. Agric. Ecosyst. Environ. 199, 207–215. doi: 10.1016/j.agee.2014.09.003
Uwimana, A., van Dam, A. A., Gettel, G. M., Bigirimana, B., and Irvine, K. (2017). Effects of river discharge and land use and land cover (LULC) on water quality dynamics in Migina Catchment, Rwanda. J. Environ. Manag. 60, 496–512. doi: 10.1007/s00267-017-0891-7
Uwimana, A., van Dam, A. A., Gettel, G. M., and Irvine, K. (2018). Effects of agricultural land use on sedimentand nutrient retention in valley-bottom wetlands of Migina catchment, southern Rwanda. J. Environ. Manag. 219, 103–114. doi: 10.1016/j.jenvman.2018.04.094
van Asselen, S., Verburg, P. H., Vermaat, J. E., and Janse, J. H. (2013). Drivers of wetland conversion: a global meta-analysis. PLoS One 8:e081292. doi: 10.1371/journal.pone.0081292
van Dam, A. A., Kipkemboi, J., Mazvimavi, D., and Irvine, K. (2014). A synthesis of past, current and future research for protection and management of papyrus (Cyperus papyrus L.) wetlands in Africa. Wetlands Ecol. Manag. 22, 99–114. doi: 10.1007/s11273-013-9335-1
Verhoeven, J. T., Arheimer, B., Yin, C., and Hefting, M. M. (2006). Regional and global concerns over wetlands and water quality. Trends Ecol. Evol. 21, 96–103. doi: 10.1016/j.tree.2005.11.015
Weigelhofer, G., Hein, T., and Bondar-Kunze, E. (2018). “Phosphorus and nitrogen dynamics in riverine systems: human impacts and management options,” in Riverine Ecosystem Management, Aquatic Ecology Series 8, eds S. Schmutz, and J. Sendzimir (London: Springer), 187–202. doi: 10.1007/978-3-319-73250-3_10
WMD (2008). Framework Management Plan for Doho-Namatala Wetland System. Wetlands Management Department Support Project (WSSP-SP). Kampala: Wetlands Management Department.
WMO (2008). Manual on Low-flow Estimation and Prediction. Operational Hydrology Report no. 50. Geneva: World Meteorological Organization.
Wollheim, W. M., Pellerin, B. A., Vörösmarty, C. J., and Hopkinson, C. S. (2005). N retention in urbanizing headwater catchments. Ecosystems 8, 871–884. doi: 10.1007/s10021-005-0178-3
Wood, A., Dixon, A., and McCartney, M. (eds) (2013). Wetland Management and Sustainable Livelihoods in Africa. New York, NY: Routledge.
Keywords: Namatala Wetland, papyrus, sustainable use, water quality, agriculture in wetlands, integrated wetland management
Citation: Namaalwa S, van Dam AA, Gettel GM, Kaggwa RC, Zsuffa I and Irvine K (2020) The Impact of Wastewater Discharge and Agriculture on Water Quality and Nutrient Retention of Namatala Wetland, Eastern Uganda. Front. Environ. Sci. 8:148. doi: 10.3389/fenvs.2020.00148
Received: 01 November 2019; Accepted: 30 July 2020;
Published: 19 August 2020.
Edited by:
Rebecca Elizabeth Tharme, Riverfutures Ltd., United KingdomReviewed by:
Elizabeth Almeida Duarte, University of Lisbon, PortugalRenato Tavares Martins, Universidade Federal de Goiás, Brazil
Copyright © 2020 Namaalwa, van Dam, Gettel, Kaggwa, Zsuffa and Irvine. This is an open-access article distributed under the terms of the Creative Commons Attribution License (CC BY). The use, distribution or reproduction in other forums is permitted, provided the original author(s) and the copyright owner(s) are credited and that the original publication in this journal is cited, in accordance with accepted academic practice. No use, distribution or reproduction is permitted which does not comply with these terms.
*Correspondence: Susan Namaalwa, c3VzYW4ubmFtYWFsd2FAbndzYy5jby51Zw==; bmFtYWFsd2FzdWVAeWFob28uY29t