- Department of Marine Sciences, Kristineberg Marine Research Station, University of Gothenburg, Fiskebäckskil, Sweden
Coastal seas and oceans receive engineered nanoparticles that are released from nano-enabled consumer and industrial products and incidental nanoparticles that are formed as byproducts of combustion and friction. The marine environment is often perceived as a rapid sink for particles, because of the high salinity promoting the attachment between particles producing heavy agglomerates that sediment on the seafloor. In this work the effect of seasonal production of extracellular polymeric substances (EPS) on particle stability is tested using seawater collected from the Gullmarn fjord in the winter, spring, and summer. A novel approach is used that is based on light scattering of the bulk particle population for tracking agglomerates and of single particles for tracking particles smaller than approximately 300 nm. Results show that organic particles formed from EPS during algal blooms are capable of stabilizing nanoparticles in marine waters for at least 48 h. In contrast, particles agglomerate rapidly in the same seawater that has previously been filtered through 0.02 μm pore size membranes. Furthermore, particles with fibrillar shape have been detected using atomic force microscopy, supporting the argument that organic particles from EPS are responsible for the stabilization effect. These results suggest that seasonal biological activity can act as an intermittent stabilization factor for nanoparticles in marine waters.
Introduction
Nanotechnology has enabled novel solutions in several sectors, from consumer products to high-tech industries and transportation. While these nano-enabled solutions are increasingly introduced in everyday life, there are more than 3,000 nano-enabled consumer products in the market1 and numerous industrial and commercial products and parts currently in use2. The use and disposal of nano-enabled products and the incidental production of nanomaterials (e.g., through combustion or friction) will inevitably lead to their release in the environment and may pose a risk to human health and the ecosystem (Wiesner et al., 2006). Risk is a factor of the hazard assessment of a material to a certain organism and the level of exposure of the organism to the material. The former is an inherent parameter of the material, while the latter is largely dependent on the surrounding environment. For example, photocatalytic titania nanoparticles produce reactive oxygen species (ROS) when exposed to sunlight, which can be hazardous, but the amounts of ROS produced will depend on the factors determining the efficiency of photoexcitation (e.g., surface exposed or quenching effects) of particles exposed to sunlight (Sharma, 2009). In natural aquatic systems, such as seas and oceans, adsorption of organics and aggregation may drastically suppress the production of ROS (Carp et al., 2004). In addition, aggregation with suspended particulate matter will produce large aggregates that sink toward the seabed, thus reducing exposure to sunlight (Praetorius et al., 2020). It is therefore important to determine the fate of nanomaterials in the aquatic environment in order to assess their risk.
Engineered nanoparticles (ENPs) from nano-enabled consumer and industrial products may enter coastal seas and oceans through indirect routes after their use and disposal, e.g., effluent discharge from wastewater treatment plants and long-range atmospheric deposition (Linders et al., 2018). In this case, ENPs are likely to be significantly altered prior to entering the aquatic environment (Kaegi et al., 2011). Direct routes, such as emissions from shipping, water-based leisure activities, release of untreated wastewater, and other anthropogenic activities are more likely to release ENPs closer to their engineered form (Kaegi et al., 2017). When ENPs enter the environment, their fate will eventually determine their potential toxicity, a factor that has been ignored by many toxicological studies (Baalousha and Lead, 2013). It is therefore necessary to develop a clear understanding of those processes that regulate the fate of ENPs in the environment (Frimmel et al., 2007; Wiesner and Bottero, 2007). Several studies have been conducted on the fate and impact of ENPs in natural waters, such as rivers (Reed et al., 2017), lakes (Gondikas et al., 2014), and estuaries (Stolpe and Hassellöv, 2007). Fewer studies have focused on marine waters, because it is expected that ENPs would quickly aggregate and settle on the sediments.
Due to the high ionic strength of seawater, which suppresses the electrostatic repulsive forces between particles, it is often perceived that nanomaterials are likely to rapidly aggregate in marine waters. Aggregates are heavier than single nanoparticles and tend to sediment faster, and therefore sediments in seas and oceans are often perceived as sinks for the fate of ENPs. However, particle aggregation is strongly regulated by dissolved organic matter (Aiken et al., 2011) and marine waters host large scale biogeochemical processes in the earth’s carbon cycle (Hedges, 1992), which involve massive amounts of organic compounds. Nutrient availability combined with increased sunlight and temperature in the spring trigger a rapid growth of phytoplankton and microorganisms (algal bloom), which in turn triggers zoo plankton grazing and viral activity, leading to dissolved organic matter release through exudates, sloppy feeding, and cell lysis (Tiselius and Kuylenstierna, 1996; Calliari and Tiselius, 2009). A fraction of the excreted organic matter forms extracellular polymeric substances (EPS) and may form larger entities that are operationally defined as transparent exopolymer particles (TEP), which is known to be correlated with the export of carbon when large structures are formed and sediment, sometimes called marine snow (Stolpe and Hassellöv, 2010). Such seasonal production and transformations of dissolved and colloidal organic matter has been shown to influence particle stability in the studied fjord (Waite et al., 2005) and may impact the fate of ENPs, as shown in lake water, where ionic strength is significantly lower than marine water (Ellis et al., 2018).
Another factor that is critical for the stability of particles in suspension is that the aggregation rate is proportional to the number concentration of the particles in the aqueous medium (Elimelech et al., 1995; Mylon et al., 2004). Marine waters contain significantly less suspended particles than river and estuary waters and aggregation may not be the primary process regulating the fate of ENPs. For example, Toncelli et al. (2017) found that it is dissolution and not aggregation that governs the fate of silver nanoparticles in seawater, at environmentally relevant particle concentrations. Terrestrial sources of ENPs in the sea include effluents of wastewater treatment plants, transport and deposition through wind, etc., while marine sources include cargo, leisure, and transportation ships. Maritime transport, in particular, is responsible for 90% of the world trade and release of ENPs from shipping (e.g., from antifouling paints) is likely to be gradual and in small amounts but impacting very large areas.
In this work, the hypothesis is tested that organic matter produced during the algal bloom may act as steric stabilizers for nanomaterials and overcome the suppression of electrostatic repulsion caused by the high ionic strength of seawater. Combined with the low concentration of natural suspended particles, the result is an extended stability of ENPs in the water column. In order to test this hypothesis, seawater was sampled and filtered sequentially through membranes with decreasing pore size to remove larger and smaller particles and organic matter that are naturally present in the seawater. Gold ENPs were then spiked in the different water fractions and the aggregation behavior of the particles over long periods, up to 2.5 days was monitored. Although sedimentation is an important process for the fate of ENPs in aquatic systems, in this work focus is given to aggregation processes.
Materials and Methods
Seawater Sampling and Treatment
Seawater samples were collected from the Gullmarn Fjord in Sweden (approximate coordinates: 58°15′19.8″N 11°25′44.0″E) using a 2 L Ruttner water sampler (KC Denmark A/S). The sampler was filled sequentially with seawater at 1 m depth and decanted in pre-cleaned Nalgene barrel until 10 L were collected. The barrel was rinsed twice with seawater prior starting sample collection. Historical monitoring data from the sampling location have shown that there are elevated values of Chlorophyll-a during the spring bloom (typically late February to mid March), and summer blooms (more spread out in time, typically from July to September). Sampling took place during three separate seasons: (i) spring (March 21, 2016) representing the end of the spring bloom, (ii) winter (February 17, 2017) representing the beginning of the spring bloom following winter inactivity, and (iii) summer (June 27, 2017) representing the more spread out summer blooms. No later than 1 h after sampling, the seawater was transferred to the lab and 5 L were gravity filtered through 5 μm mixed cellulose membrane (SMWP Millipore) to remove most microorganisms that may alter the composition of the organic matter in the seawater during storage. Prior to this first filtration step, the filters were rinsed by flowing 1 L of MQ water across the membrane. The seawater filtrate (labeled “micro-seawater,” μSW) was stored at 5°C until further use. From the μSW, 2 L were filtered through 0.45 μm hydrophilic polyvinylidene fluoride (PVDF) membrane (HVLP Millipore) and this filtrate was labeled “submicron seawater” (sμSW) and was collected in polycarbonate containers (Nalgene). Subsequently, 0.5 L from the sμSW were filtered through a 0.02 μm alumina based (Anopore) membrane in a polypropylene housing (Whatman) and the filtrate was labeled “nano seawater” (nSW) and was collected in polycarbonate bottles.
ENP Aggregation Studies
The three seawater fractions (μSW, sμSW, and nSW) were spiked and thoroughly mixed with 30 nm Au ENPs to reach a total Au concentration in the test suspensions of 56 μg/L. This concentration was chosen to represent the higher end of the expected concentrations of nanomaterials in surface waters (Gottschalk et al., 2009). Detailed characterization of the Au ENPs used are given in Gallego-Urrea et al. (2016). Artificial seawater (ASW) was synthesized in the laboratory and spiked with Au ENPs, under the same conditions as for the filtered seawater fractions. Preliminary tests conducted under continuous stirring in a jar test apparatus showed no effect of stirring on aggregation, thus experiments were conducted in idle 50 ml polypropylene centrifuge tubes. Two replicates were prepared for each type of water matrix. Aggregation in the spiked waters was monitored by measuring the intensity-based hydrodynamic diameter with dynamic light scattering (DLS), (Zetasizer S, beam wavelength 532 nm, Malvern instruments) and the number concentration and hydrodynamic diameter of particles (including individual scattering intensity) in suspension using nanoparticle-tracking analysis (NTA), (NanoSight LM10, Malvern instruments; software NanoSight 2.3, threshold 18, gain 7). Prior to each measurement, the ENP suspension was gently mixed end over end and 1 mL was removed for DLS and NTA analysis, each.
The diffusion tracks recorded by the NanoSight software were analyzed and 2D histograms of particle size distribution and particle intensity distribution were obtained and normalized by the number of recordings (Gallego-Urrea et al., 2016) in order to allow comparison (Figure S1 in the SI). From Figure 3, the intensity-size signal from AuNP is distinguishable from the signal coming from other natural particles or aggregates, although there is not such a strong difference in intensity as observed in other works (Gallego-Urrea et al., 2016); this is probably due a combination of two factors: (i) the settings chosen during measurements to maximize the number of particles tracked and (ii) the presence of particles with relatively high scattering efficiency in the natural waters used here as compared to model particles used in Gallego-Urrea et al. (2016). Nevertheless, after visual identification of areas in the 2D histogram, it was possible to divide the histograms in three zones: the sum of all tracks encompassed by an ellipse between 10 and 60 nm hydrodynamic diameter and 0–4 intensity units (called “original”), a bigger ellipsoid covering the area not overlapping with the “original” ellipsoid between 10 and 300 nm and 2–9 intensity units (“Aggregates”) and a third one summing the rest of particles called “Large” (Figure 3).
Atomic force microscopy (AFM) (Nanoscope IIIA, Digital Instruments) was used to image the physicochemical structure of the aquatic colloids and especially applied to investigate the presence of fibrillary biopolymers. Prior to analysis, 50 mL of the seawater fraction were placed in a polypropylene tube and a thin sheet of mica glass (approximate area 5 mm × 5 mm) was inserted for 2 h, allowing organics and particles to attach on the mica surface. The mica sheet was then removed and dipped in MQ water three times to rinse off the salts. Excess water was removed by touching a fiber-free paper (Kimwipe) and the mica sheet was stored in pre-cleaned polypropylene container. During analysis with AFM, random areas of the mica sheet were scanned.
Results and Discussion
The intensity of scattered light measured with DLS (counts per second) on the collected seawater indicates that filtration with decreasing membrane pore size resulted in the removal of natural particles and organic matter that are larger than the membrane pore size. The largest difference was observed in seawater collected during the summer. Seawater filtered through 5 μm membranes (μSW) gave the highest background signal intensity in DLS (represented by the mean count rate in Figure 1, left) compared to seawater filtered through 0.45 μm (sμSW) and 0.02 μm (nSW). Background signal from nSW was at the same level as the instrument noise, indicating the absence of natural particles smaller than 20 nm that may scatter light. The same was observed for nSW, sμSW, and μSW of winter and spring water. The background signals from sμSW and μSW indicate the presence of natural particles from the algal bloom, most likely TEP precursors and other organic matter that are results of the seasonal biological activity. Spiking with Au ENPs more than doubled the scattering intensity of the suspension and, independently of the seawater fraction, the size of Au ENPs rapidly increased immediately after spiking. The intensity-based hydrodynamic diameter increased from 40 nm (ENPs in MQ water) up to 120 nm as soon as the particles got in contact with the seawater medium, likely caused by homoaggregation of the Au ENPs and attachment of natural particles and organic compounds on their surface. In summer nSW, the hydrodynamic diameter of the Au ENPs is gradually increasing over the time-span of 5 h (Figure 1, right), indicating a slow homoaggregation process that is the result of the high ionic content of the water and the low number of particles present. On the contrary, the hydrodynamic diameter of the Au ENPs in sμSW and μSW increase to approximately 120 nm within the first 10 min (not captured by the DLS measurements) and then increase with a slower rate compared to nSW. This behavior is indicative of a heteroaggregation process resulting in particles coated with organic compounds (corona formation) that are more stable than the bare particles (Liu et al., 2013).
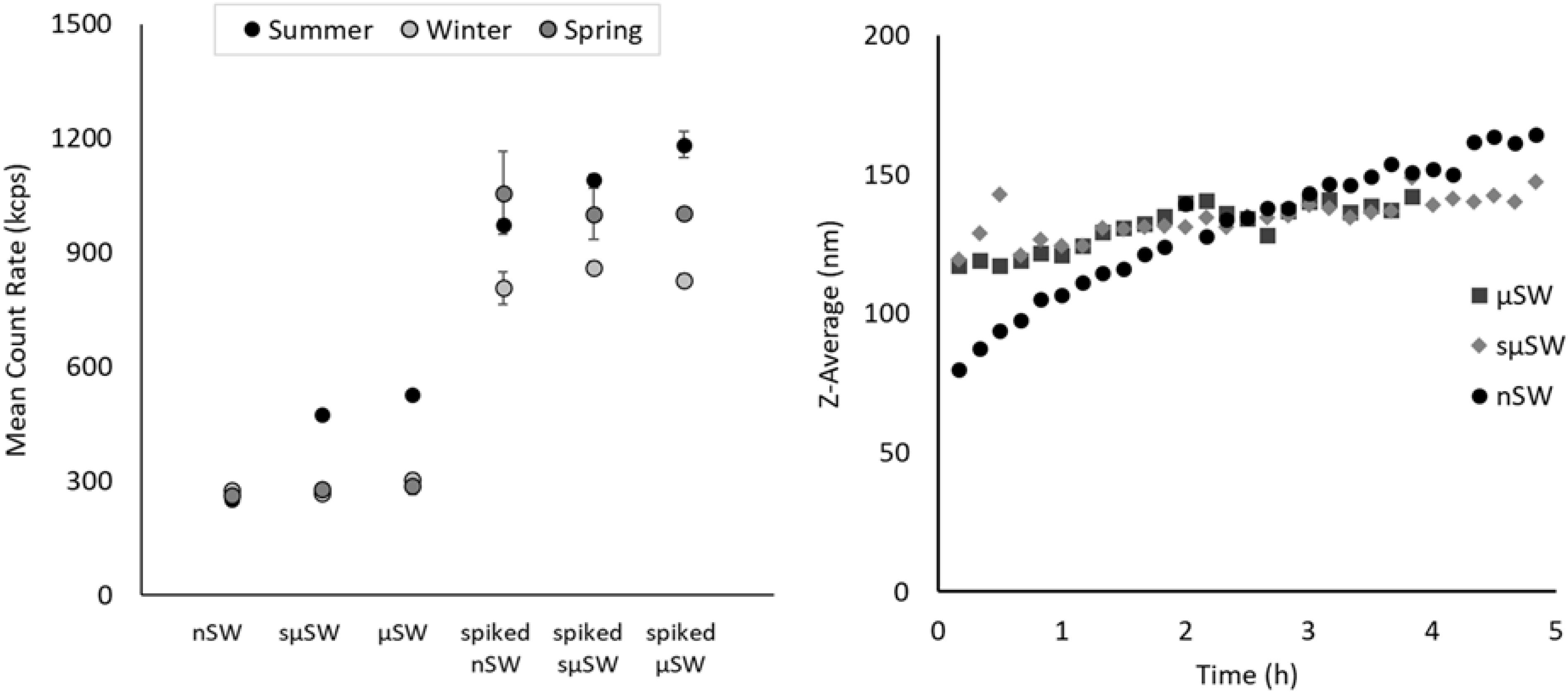
Figure 1. Intensity of scattered light (measured with DLS) for nSW, sμSW, and μSW before and after spiking with Au ENPs in seawater collected during the summer, winter, and spring season (left). Marker and error bars for non-spiked samples represent the average and standard deviation of three measurements, while marker and error bars for spiked samples represent the average and deviation between duplicate measurements. Intensity-based hydrodynamic diameter (Z-average) of summer seawater spiked with Au ENPs within 5 h from the time of spiking (right).
Prolonged aggregation studies (up to 58 h after spiking with Au ENPs) showed that the hydrodynamic diameter of the suspensions continues to increase (Figure 2). Due to the absence of stabilizing factors, aggregation rates observed in nSW from all seasons were higher than those observed for the other fractions and similar to ASW. DLS is a technique measuring the size distribution of the bulk suspension and is highly sensitive to the presence of larger particles, since the intensity of scattered light is dependent on the particle diameter to the power of six (Schurtenberger and Meredith, 1993; Hassellov et al., 2008; Gallego-Urrea et al., 2014; Gondikas et al., 2019). As aggregation proceeds, the formation of larger aggregates will dominate the Z-Average values reported by DLS, overshadowing the original monomer particles and smaller aggregates. The aggregation process that was observed with DLS (Figure 2) is therefore indicative of the rate of formation of large aggregates (up to 1 μm). On the other hand, NTA is a single particle technique and is thus sensitive to the number concentration of the suspended particles. The larger aggregates reported by DLS are few in number and are thus rarely detected by NTA. Therefore, this technique was used to follow the particle population of smaller particles (10–300 nm), i.e., the monomer Au ENPs that were spiked in the seawater and small aggregates. In addition to the particle size and number concentration that is typically reported from NTA measurements, the intensity of scattered light and particle size was used (Figure 3) to differentiate between the Au NP monomers smaller than 60 nm (original), small aggregates of Au ENPs and natural particles less than 300 nm (aggregates), and larger particles and aggregates (large), as shown in Figure 3. Since the intensity and size are reported on a single particle basis, the bias toward larger particles posed by DLS is overcome.
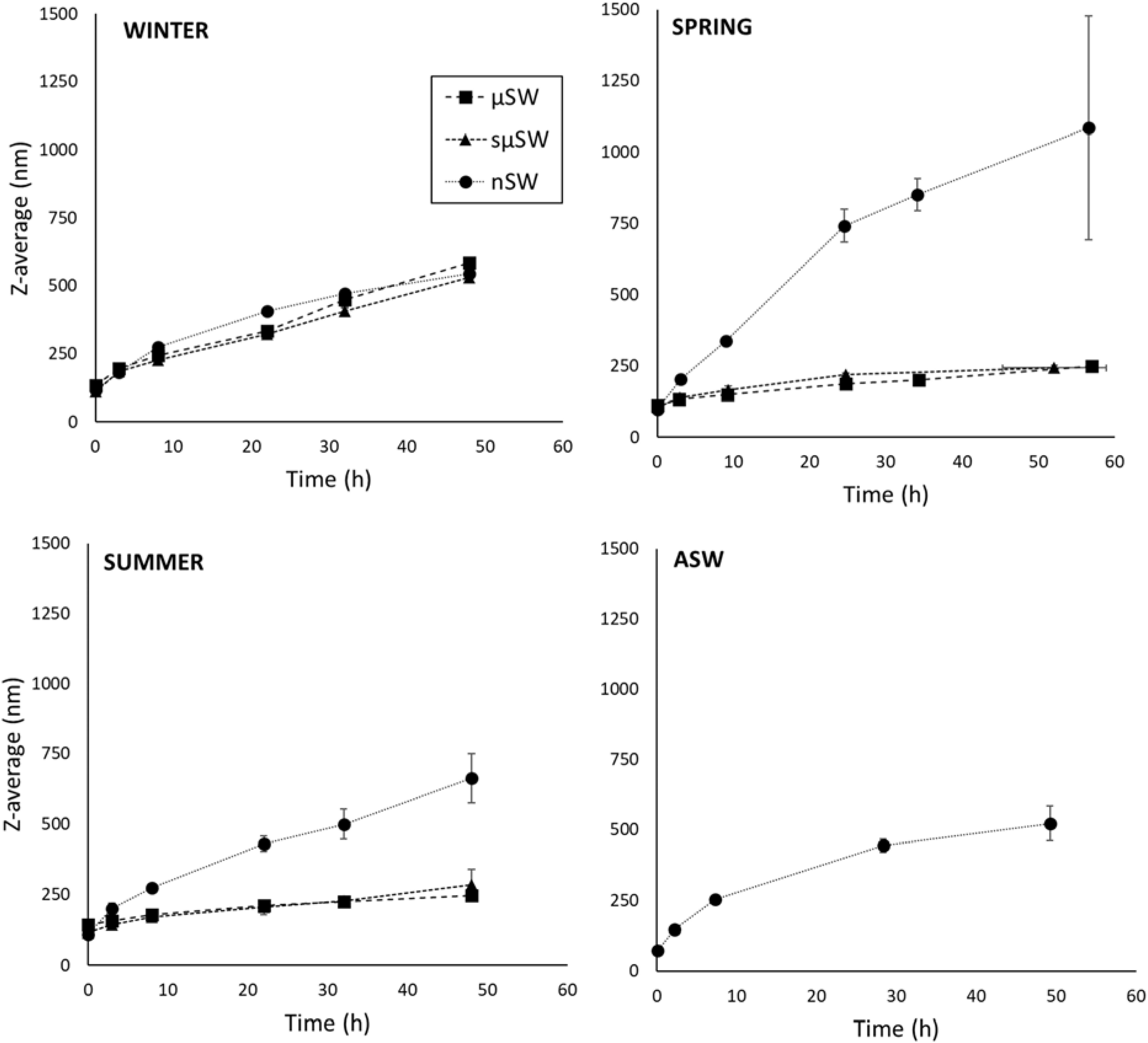
Figure 2. Z-average measured with DLS over time for Au ENPs in seawater collected during the winter (A), spring (B), and summer (C) and filtered through 5 μm (μSW), 0.45 μm (sμSW), and 0.02 μm (nSW) pore size membrane. Identical experiments in artificial seawater (ASW) are also shown (D).
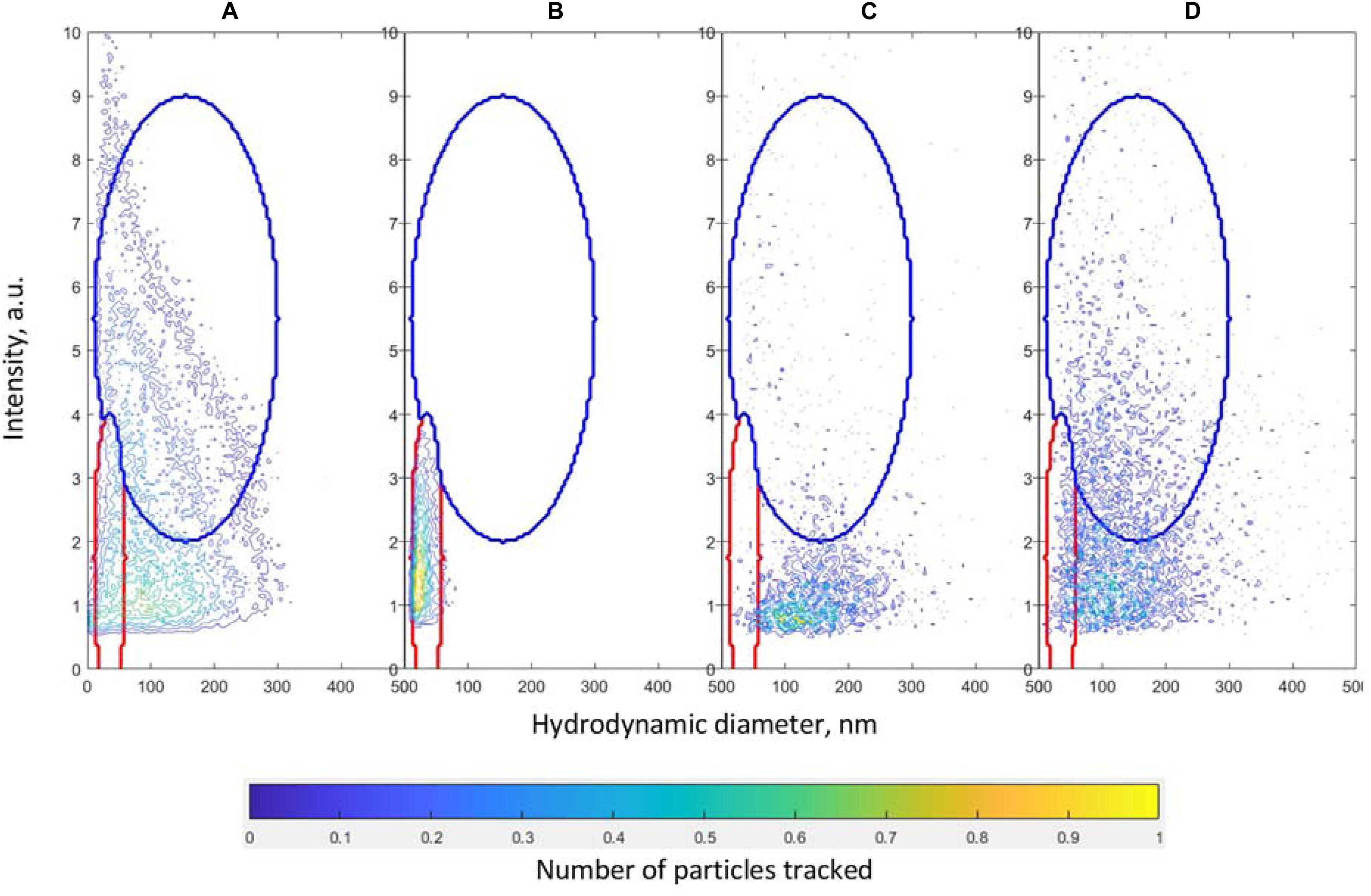
Figure 3. Areas selected for estimating the concentration of particles from NTA measurements according to size and intensity. The inner red ellipse corresponds to “original” particles; the outer purple ellipse corresponds to “aggregates” particles, as described in the text. The contour plots correspond to the following samples, discriminated by size and intensity: (A) the average of all particles measured in the experiments (154 samples), (B) one replicate from the AuNP spiked in milli-Q water (number of particles tracked scaled by 10 to facilitate comparison), (C) one replicate of the summer sample μSW (background signal) and (D) one replicate for the spiked summer sample μSW taken at time 0.
A higher number of background particles (prior to spiking) was found in the summer samples, compared to the spring and winter (Figure 4, background), which is in agreement with DLS measurements. These particles are for the most part removed in the nSW. In ASW (Figure 4, left column) there is an increase of particle size with time, which plateaus after 7 h. The simultaneous decrease of the number concentration of particles (original, aggregates, and large) indicates that particles continue to aggregate into large particles, not captured by NTA.
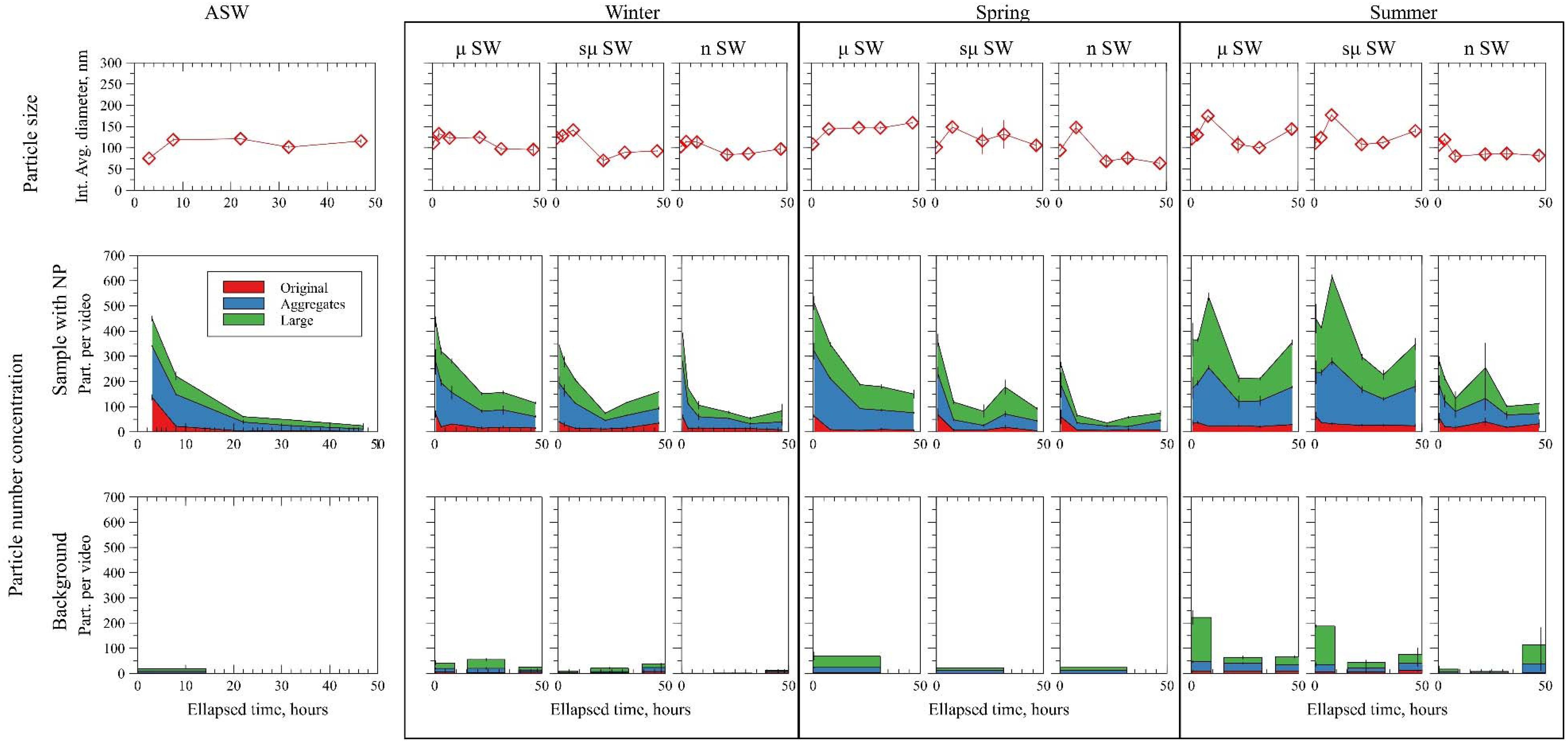
Figure 4. Evolution in time of the intensity averaged diameter from NTA, concentration of particles from NTA in samples with AuNP and in the background. Original, aggregates and large particles are described in the text and Figure 3. Two replicates of the fractionated samples (nSW, suSW, and uSW as explained in text) are presented. Background samples (without AuNP spike) are presented when available.
In μSW and sμSW of the summer water (Figure 4, right column) populations of original, aggregate, and large particles were observed that are fluctuating around higher values, both in terms of number and size. Only in nSW a similar behavior to ASW was observed, where the number concentration decreases with time, indicating the formation of larger aggregates. This was observed in nSW from all seasons. In the μSW and sμSW of spring and winter water a similar behavior as in nSW and ASW was observed, with the number concentrations of all groups of particles decreased with time.
Overall, the NTA data shows that particles in the size range of 10–300 nm tend to disappear with a faster rate in the winter than in spring and they may remain suspended for periods of time longer than 2 days in the summer. The experiments were conducted in three aquatic colloid size fractions (μSW, sμSW, and nSW) show that background particles are responsible for the slower aggregation rates of Au ENPs. In order to elucidate the shape and size of these particles, AFM analysis was conducted on spiked and non-spiked waters. Several fibrils with lengths of 200–500 nm and width of 1.5–2.5 nm were detected in sμSW samples, along with some longer fibrils with lengths of more than 2 μm (Figure 5). These particles are likely products of the algal bloom, such as TEP and its precursors. Fibrillar shaped biopolymers have been reported with AFM previously (Santschi et al., 1998; Verdugo et al., 2004), and also from the same location (Stolpe and Hassellöv, 2010). The physicochemical structure and dynamics of such biopolymers from marine algae and bacteria has been proposed to conform to the dynamics of a gel system (Chin et al., 1998). Nanoparticle aggregates attached to fibrils were also observed due to their spherical shape and size. Physicochemical conditions regulate how free nanoparticles and homoagglomerates can adhere to microgels and large gel structures leading to the sedimentation process known as TEP or marine snow (Passow, 2002). The observations here provide plausible explanations that depending on season in any given marine biogeochemical domain, the rate of homoagglomeration for specific nanomaterials in the high ionic strength conditions is not only driving the ENP fate but as important is the interplay of free and agglomerated ENP with the marine biopolymers and its dynamics.
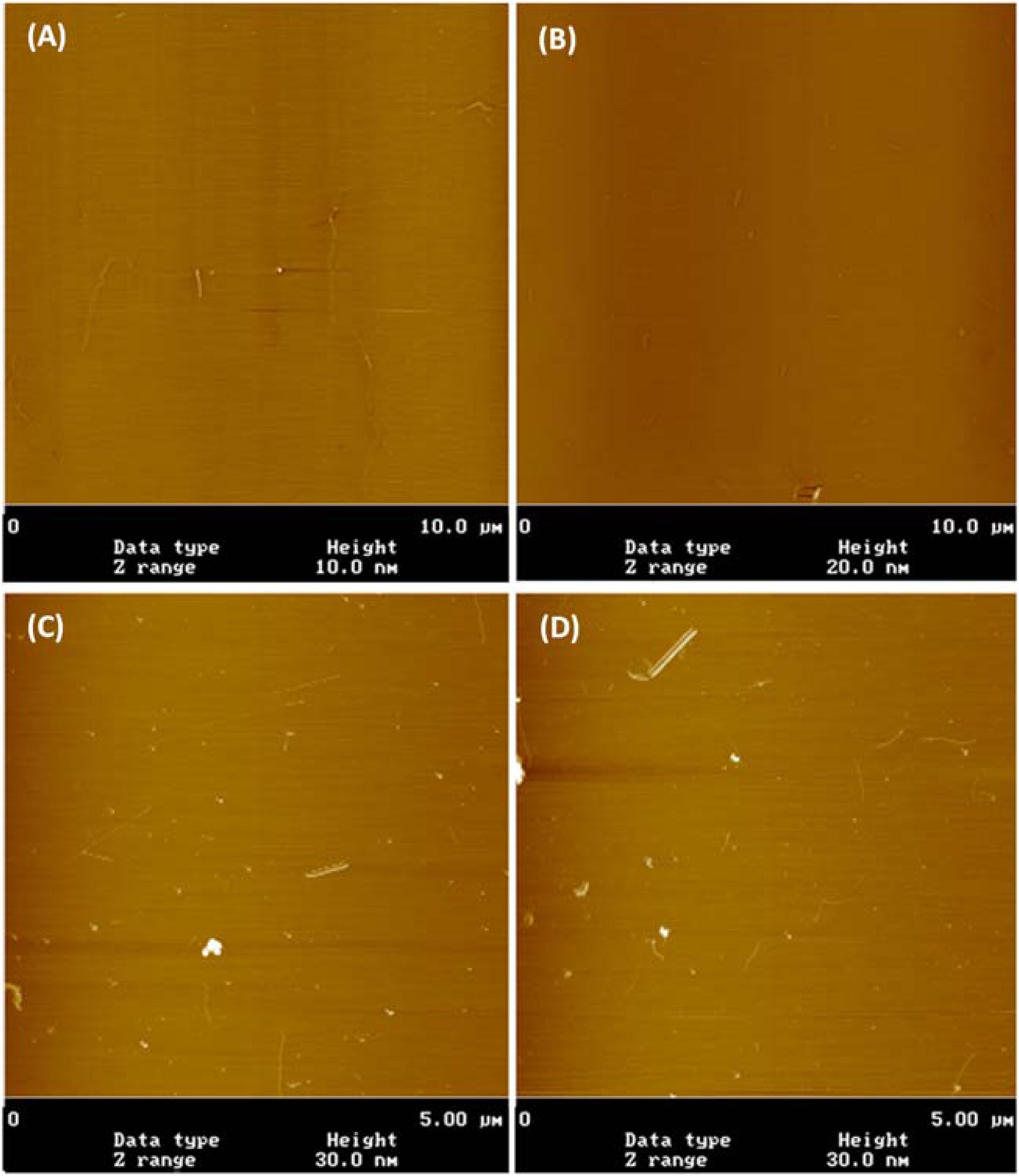
Figure 5. Atomic force microscopy pictures of TEP molecules in unspiked summer sμSW (A,B) and TEP molecules with Au ENPs in spiked summer sμSW (C,D).
Conclusion
Here, a novel approach was used to study the aggregation process of ENPs in surface water collected from the field, combining DLS to detect the formation of large aggregates and NTA to track the number concentration and size distribution of particles, while distinguishing between ENPs, natural particles, and their aggregates based on their scattering intensity. The occurrence, shape and size of organic fibrils that has been reported in the literature was verified in our samples with AFM. The chemical composition of seawater induces rapid aggregation in nano-filtered and ASWs, due to the high ionic strength and suppression of the electric double layer (EDL) on the particles surface. It is therefore often perceived that ENPs will rapidly aggregate and sediment to the seafloor. Our work shows that this may be true when biological activity is suppressed by seasonal conditions, but when algal blooms occur seawaters are enriched with a variety of organic compounds that may stabilize ENPs for several days. It is therefore likely that organic matter, mostly TEP and precursors induce a steric stabilization mechanism that is counteracting the suppression of the EDL and the suspended particles maintain their numbers and particle size. A similar finding has been reported by Ellis et al., for seasonal variations of river water chemistry and its effect on nanoparticle stability (Ellis et al., 2018).
Our study shows that organic fibrils present in the fraction passing through the 5 μm filter and between 0.02 and 0.45 μm were able to stabilize Au ENPs for several days. Since this effect was only observed in the larger fractions (>0.02 μm), it is likely that these substances are responsible for the stabilization of AuNP in SW observed in this work. This material is present during spring and summer primarily due to biological activity. Biochemical properties of the seawater at the sampling location show that the biological activity may be significantly variable both temporally and spatially (Table S1 in SI). For example, Chlorophyll-a values in the spring were higher than winter and summer, indicating a spring bloom event; however, elevated values were also reported in the winter and spring in the upper water layer (0–1 m depth), which is where our sampling took place. The winter sampling time co-occurred with an unusual early start of the spring bloom, so it corresponds to the early stage of the spring bloom that followed the winter inactivity, rather than truly winter conditions with no biological activity. A potential solution to the temporal and spatial variability has been recently proposed by Santschi et al., suggesting that the protein/carbohydrate ratio of EPS may be used as a proxy for their aggregation propensity (Santschi et al., 2020). Although not currently measured in routine monitoring, this approach may simplify predictions for the fate of ENPs in seawater.
Exopolymeric substances, dominated by acidic polysaccharides are exudated from phytoplankton during algal blooms (Alldredge et al., 1993). EPS form biotically in marine waters and have high affinity toward solids surfaces and are thus influenced by the presence of particles (Chen et al., 2011; Chiu et al., 2017). EPS are forming a gel network that may act as a stabilizing factor for nanomaterials in seawater, but in high concentrations can become the glue to form marine snow aggregates that lead to scavenging of suspended particles and depositing them on the sediments, a process that is critical for the biogeochemical cycling of elements (Zetsche and Ploug, 2015). Algal blooms are therefore acting as an intermittent stabilizing factor for ENPs in marine waters. This study calls for more studies on the fate of ENP under realistic conditions (in situ field studies or mesocosms) with complementary advanced minimum perturbing analytical techniques. For example, the settling fate of nanoparticles and intermediate buoyancy in the nepheloid benthic boundary layers of coastal seas under seasonal variations and the consequence on ecological exposure should be investigated.
Data Availability Statement
The raw data supporting the conclusions of this article will be made available by the authors, without undue reservation.
Author Contributions
MHas conceived the idea, developed the experimental plan, and contributed to writing of the manuscript. AG co-developed the experimental plan, carried out the sampling, sample analysis, data analysis, and wrote the manuscript. JG-U helped with the sampling, carried out data analysis, and contributed to writing of the manuscript. MHal and ND helped with the sampling, carried out sample analysis, and data analysis. All authors contributed to the article and approved the submitted version.
Funding
AG, JG-U, and MHas were partially supported by the Mistra Environmental Nanosafety Program, phases 1 and 2, financed by the Swedish Foundation for Strategic Environmental Research (grant no. DIA 2013/48) and the NANOFASE project funded under the European Union Horizon 2020 framework (grant no. 646002). AG has also received funding from the NanoIsland project, financed by the Hellenic Foundation for Research and Innovation (HFRI) and the General Secretariat for Research and Technology (GSRT), under grant agreement No. 1317. JG-U was also financed by the Centre for Future Chemical Risk Assessment and Management Strategies (FRAM), University of Gothenburg, Sweden.
Conflict of Interest
The authors declare that the research was conducted in the absence of any commercial or financial relationships that could be construed as a potential conflict of interest.
Acknowledgments
The authors would like to thank Anders Mårtensson for assisting with the AFM measurements and the reviewers for their insightful comments.
Supplementary Material
The Supplementary Material for this article can be found online at: https://www.frontiersin.org/articles/10.3389/fenvs.2020.00151/full#supplementary-material
Footnotes
References
Aiken, G. R., Hsu-Kim, H., and Ryan, J. N. (2011). Influence of dissolved organic matter on the environmental fate of metals, nanoparticles, and colloids. Environ. Sci. Technol. 45, 3196–3201. doi: 10.1021/es103992s
Alldredge, A. L., Passow, U., and Logan, B. E. (1993). The abundance and significance of a class of large, transparent organic particles in the ocean. Deep. Res. Part I. 40, 1131–1140. doi: 10.1016/0967-0637(93)90129-Q
Baalousha, M., and Lead, J. R. (2013). Nanoparticle dispersity in toxicology. Nat. Nanotechnol. 8, 308–309. doi: 10.1038/nnano.2013.78
Calliari, D., and Tiselius, P. (2009). Organic carbon fluxes through the mesozooplankton and their variability at different time-scales in the Gullmarsfjord. Sweden. Estuar. Coast. Shelf Sci. 85, 107–117. doi: 10.1016/j.ecss.2009.06.016
Carp, O., Huisman, C. L., and Reller, A. (2004). Photoinduced reactivity of titanium dioxide. Prog. Solid State Chem. 32, 33–177. doi: 10.1016/j.progsolidstchem.2004.08.001
Chen, C. S., Anaya, J. M., Zhang, S., Spurgin, J., Chuang, C. Y., Xu, C., et al. (2011). Effects of engineered nanoparticles on the assembly of exopolymeric substances from phytoplankton. PLoS One 6:e21865. doi: 10.1371/journal.pone.0021865
Chin, W. C., Orellana, M. V., and Verdugo, P. (1998). Spontaneous assembly of marine dissolved organic matter into polymer gels. Nature 391, 568–572. doi: 10.1038/35345
Chiu, M. H., Khan, Z. A., Garcia, S. G., Le, A. D., Kagiri, A., Ramos, J., et al. (2017). Effect of engineered nanoparticles on exopolymeric substances release from marine phytoplankton. Nanoscale Res. Lett. 12:620. doi: 10.1186/s11671-017-2397-x
Elimelech, M., Gregory, J., Jia, X., and Williams, R. (1995). Particle Deposition and Aggregation. Woburn, MA: Butterworth-Heinemann.
Ellis, L. J. A., Baalousha, M., Valsami-Jones, E., and Lead, J. R. (2018). Seasonal variability of natural water chemistry affects the fate and behaviour of silver nanoparticles. Chemosphere 191, 616–625. doi: 10.1016/j.chemosphere.2017.10.006
Frimmel, F. H., von der Kammer, F., and Flemming, H.-C. (2007). Colloidal Transport in Porous Media. Berlin: Springer-Verlag.
Gallego-Urrea, J. A., Hammes, J., Cornelis, G., and Hassellöv, M. (2014). Multimethod 3D characterization of natural plate-like nanoparticles: Shape effects on equivalent size measurements. J. Nanoparticle Res. 16:2383. doi: 10.1007/s11051-014-2383-5
Gallego-Urrea, J. A., Hammes, J., Cornelis, G., and Hassellov, M. (2016). Coagulation and sedimentation of gold nanoparticles and illite in model natural waters: influence of initial particle concentration. NanoImpact 3–4, 67–74. doi: 10.1016/j.impact.2016.10.004
Gondikas, A., Von Der Kammer, F., Reed, R. B., Wagner, S., Ranville, J. F., and Hofmann, T. (2014). Release of TiO2 nanoparticles from sunscreens into surface waters: a one-year survey at the old danube recreational lake. Environ. Sci. Technol. 48, 5415–5422. doi: 10.1021/es405596y
Gondikas, A., Wagner, S., and Navratilova, J. (2019). “Nanomaterials in water: detection and characterization,” in Encyclopedia of Water: Science, Technology, and Society, ed. P. A. Maurice (Hoboken, NJ: JohnWiley & Sons, Inc), doi: 10.1002/9781119300762.wsts0078
Gottschalk, F., Sonderer, T., Scholz, R. W., and Nowack, B. (2009). Modeled environmental concentrations of engineered nanomaterials (TiO2, ZnO, Ag, CNT, fullerenes) for different regions. Environ. Sci. Technol. 43, 9216–9222. doi: 10.1021/es9015553
Hassellov, M., Readman, J. W., Ranville, J. F., and Tiede, K. (2008). Nanoparticle analysis and characterization methodologies in environmental risk assessment of engineered nanoparticles. Ecotoxicology 17, 344–361. doi: 10.1007/s10646-008-0225-x
Hedges, J. I. (1992). Global biogeochemical cycles: progress and problems. Mar. Chem. 39, 67–93. doi: 10.1016/0304-4203(92)90096-S
Kaegi, R., Englert, A., Gondikas, A., Sinnet, B., von der Kammer, F., and Burkhardt, M. (2017). Release of TiO2 – (Nano) particles from construction and demolition landfills. NanoImpact 8, 73–79. doi: 10.1016/j.impact.2017.07.004
Kaegi, R., Voegelin, A., Sinnet, B., Zuleeg, S., Hagendorfer, H., Burkhardt, M., et al. (2011). Behavior of metallic silver nanoparticles in a pilot wastewater treatment plant. Environ. Sci. Technol. 45, 3902–3908. doi: 10.1021/es1041892
Linders, T., Infantes, E., Joyce, A., Karlsson, T., Ploug, H., Hassellöv, M., et al. (2018). Particle sources and transport in stratified Nordic coastal seas in the Anthropocene. Elem Sci Anth 6:29. doi: 10.1525/elementa.149
Liu, W., Rose, J., Plantevin, S., Auffan, M., Bottero, J.-Y., and Vidaud, C. (2013). Protein corona formation for nanomaterials and proteins of a similar size: hard or soft corona? Nanoscale 5, 1658–1668. doi: 10.1039/c2nr33611a
Mylon, S. E., Chen, K. L., and Elimelech, M. (2004). Influence of natural organic matter and ionic composition on the kinetics and structure of hematite colloid aggregation: implications to iron depletion in estuaries. Langmuir 20, 9000–9006. doi: 10.1021/la049153g
Passow, U. (2002). Transparent exopolymer particles (TEP) in aquatic environments. Prog. Oceanogr. 55, 287–333. doi: 10.1016/S0079-6611(02)00138-6
Praetorius, A., Badetti, E., Brunelli, A., Clavier, A., Gallego-Urrea, J. A., Gondikas, A., et al. (2020). Strategies for determining heteroaggregation attachment efficiencies of engineered nanoparticles in aquatic environments. Environ. Sci. Nano 7, 351–367. doi: 10.1039/c9en01016e
Reed, R. B., Martin, D. P., Bednar, A. J., Montaño, M. D., Westerhoffd, P., and Ranville, J. F. (2017). Multi-day diurnal measurements of Ti-containing nanoparticle and organic sunscreen chemical release during recreational use of a natural surface water. Environ. Sci. Nano 4, 69–77. doi: 10.1039/c6en00283h
Santschi, P. H., Balnois, E., Wilkinson, K. J., Zhang, J., Buffle, J., and Guo, L. (1998). Fibrillar polysaccharides in marine macromolecular organic matter as imaged by atomic force microscopy and transmission electron microscopy. Limnol. Oceanogr. 43, 896–908. doi: 10.4319/lo.1998.43.5.0896
Santschi, P. H., Xu, C., Schwehr, K. A., Lin, P., Sun, L., Chin, W. C., et al. (2020). Can the protein/carbohydrate (P/C) ratio of exopolymeric substances (EPS) be used as a proxy for their ‘stickiness’ and aggregation propensity? Mar. Chem. 218:103734. doi: 10.1016/j.marchem.2019.103734
Schurtenberger, P., and Meredith, N. (1993). “Characterization of biological and environmental particles using static and dynamic light scattering,” in Environmental Particles, Vol. 2, eds J. Buffle and H. van Leeuwen (Boca Raton, FL: Lewis), 37–115. doi: 10.1201/9781351270809-2
Sharma, V. K. (2009). Aggregation and toxicity of titanium dioxide nanoparticles in aquatic environment–a review. J. Environ. Sci. Health. A Tox. Hazard. Subst. Environ. Eng. 44, 1485–1495. doi: 10.1080/10934520903263231
Stolpe, B., and Hassellöv, M. (2007). Changes in size distribution of fresh water nanoscale colloidal matter and associated elements on mixing with seawater. Geochim. Cosmochim. Acta 71, 3292–3301. doi: 10.1016/j.gca.2007.04.025
Stolpe, B., and Hassellöv, M. (2010). Nanofibrils and other colloidal biopolymers binding trace elements in coastal seawater: significance for variations in element size distributions. Limnol. Oceanogr. 55, 187–202. doi: 10.4319/lo.2010.55.1.0187
Tiselius, P., and Kuylenstierna, M. (1996). Growth and decline of a diatom spring bloom: phytoplankton species composition, formation of marine snow and the role of heterotrophic dinoflagellates. J. Plankton Res. 18, 133–155. doi: 10.1093/plankt/18.2.133
Toncelli, C., Mylona, K., Kalantzi, I., Tsiola, A., Pitta, P., Tsapakis, M., et al. (2017). Silver nanoparticles in seawater: a dynamic mass balance at part per trillion silver concentrations. Sci. Total Environ. 601–602, 15–21. doi: 10.1016/j.scitotenv.2017.05.148
Verdugo, P., Alldredge, A. L., Azam, F., Kirchman, D. L., Passow, U., and Santschi, P. H. (2004). The oceanic gel phase: a bridge in the DOM-POM continuum. Mar. Chem. 92, 67–85. doi: 10.1016/j.marchem.2004.06.017
Waite, A. M., Gustafsson, Ö, Lindahl, O., and Tiselius, P. (2005). Linking ecosystem dynamics and biogeochemistry: sinking fractionation of organic carbon in a Swedish fjord. Limnol. Oceanogr. 50, 658–671. doi: 10.4319/lo.2005.50.2.0658
Wiesner, M., and Bottero, J.-Y. (2007). Environmental Nanotechnology - Applications and Impacts of Nanomaterials, 1st Edn. New York, NY: McGraw-Hill Education, doi: 10.1036/0071477500
Wiesner, M., Lowry, G., Alvarez, P., Dionysiou, D., and Biswas, P. (2006). Assessing the risks of manufactured nanomaterials. Environ. Sci. Technol. 40, 4336–4345. doi: 10.1021/es062726m
Keywords: nanomaterials, fate, particle stability, marine water, extracellular polymeric substances
Citation: Gondikas A, Gallego-Urrea J, Halbach M, Derrien N and Hassellöv M (2020) Nanomaterial Fate in Seawater: A Rapid Sink or Intermittent Stabilization? Front. Environ. Sci. 8:151. doi: 10.3389/fenvs.2020.00151
Received: 12 May 2020; Accepted: 07 August 2020;
Published: 29 September 2020.
Edited by:
Vera I. Slaveykova, Université de Genève, SwitzerlandReviewed by:
Enrica Alasonati, Laboratoire National De Metrologie Et D’Essais, FrancePeter H. Santschi, Texas A&M University, United States
Copyright © 2020 Gondikas, Gallego-Urrea, Halbach, Derrien and Hassellöv. This is an open-access article distributed under the terms of the Creative Commons Attribution License (CC BY). The use, distribution or reproduction in other forums is permitted, provided the original author(s) and the copyright owner(s) are credited and that the original publication in this journal is cited, in accordance with accepted academic practice. No use, distribution or reproduction is permitted which does not comply with these terms.
*Correspondence: Martin Hassellöv, bWFydGluLmhhc3NlbGxvdkBndS5zZQ==
†Present address: Andreas Gondikas, Department of Geology and Geoenvironment, National and Kapodistrian University of Athens, Athens, Greece; Maurits Halbach, Carl von Ossietzky University of Oldenburg, Oldenburg, Germany; Nicolas Derrien, Université de Bordeaux, Talence, France