- Faculty of Biosciences and Aquaculture, Nord University, Bodø, Norway
The ubiquitous presence of microplastics and their marine ecotoxicity are major public concerns. Microplastics are ingested accidentally by the marine fauna or are taken up indirectly through the food chain. These particles can accumulate in cells and tissues and affect the normal biological functions of organisms, including their defense mechanisms. There is limited information available about the response of immune cells to microplastics; the degree of uptake by the cells, the response of different organs or the impact of environmental concentrations of microplastic are matters that remain unclear. Moreover, very little is known about the toxicity of different polymer types. This study aimed to shed light on the physical impact of small microplastics (1–5 μm) on cells from Atlantic salmon. Immune cells from intestine, blood, and head kidney were exposed to green fluorescent polyethylene microplastic (PE-MP), yellow fluorescent polystyrene microplastic (PS-MP) and both. High (50 mg/L), medium (5 mg/L), and low (0.05 mg/L) concentrations were tested for 1, 24, 48, and 72 h to study cell mortality and microplastic uptake. Quantitative data of microplastic uptake by fish immune cells were obtained for the first time by imaging flow cytometry. Salmon immune cells showed a relatively low ability to phagocytose microplastics. Less than 6% of the cells ingested the particles after 48 h of exposure to high concentrations. Cells also phagocytosed microplastics at low concentrations although at low rates (<0.1%). PE-MPs was phagocytosed by higher percentage of cells compared to PS-MPs and the former bioaccumulated in time while the latter decreased over time. However, each cell generally phagocytosed more PS-MPs particles than PE-MPs. Cells from different tissues showed different responses to the microplastic polymers. In conclusion, this study shows that immune cells of Atlantic salmon can phagocytose microplastics, and the impact is dependent on the microplastic type. PE-MPs, the most abundant polymer in the oceans and a widely used plastic in salmon aquaculture, was more easily taken up than PS-MPs. Furthermore, the study demonstrates how imaging flow cytometry can be applied in microplastics research.
Introduction
The increasing presence of microplastics (MPs) in the marine environment is of global concern. MPs are defined as plastic particles smaller than 5 mm (Arthur et al., 2009), although it has been recently proposed to redefine the limits of MPs to 1 to <1,000 μm to fit the SI nomenclature and for a better consensus among scientists (Hartmann et al., 2019). MPs in the marine environment mainly come from the degradation and fragmentation of different plastic items (GESAMP, 2015), resulting in rough and irregular shaped particles (Choi et al., 2018). Plastic debris mainly consist of fossil fuel-derived synthetic polymers, which can be classified into thermoplastic and thermoset (UNEP, 2016). Thermoplastic polymers are the most abundant plastic types found in the marine environment; a study conducted in 2014 found that almost half of the MPs collected in the North Atlantic ocean was polyethylene (PE) and polypropylene (PP) (Enders et al., 2015). Similarly, a study carried out in 2015 found that PE accounted for 73% of the small MPs (20–999 μm) collected in the North Atlantic Subtropical Gyre, followed by PP, PVC, and PS (Ter Halle et al., 2017). Other synthetic polymers such as polyethylene terephthalate (PET) and polyamides (PA) are also widely detected in the marine environment (Suaria et al., 2016; Erni-Cassola et al., 2019).
MPs are ingested by a wide range of marine organisms (Lusher et al., 2017b; Alimba and Faggio, 2019). Particles can accumulate in the digestive tract of animals and affect, among other parameters, their behavior, growth and survival (Von Moos et al., 2012; Choi et al., 2018; Foley et al., 2018; Assas et al., 2020). For example, a 7-days exposure of zebrafish (Danio rerio) to 5 μm PS-MPs resulted in their accumulation in the gills, liver, and gut of the fish, leading to increased oxidative stress; underlining the potential toxic effect of the MP (Lu et al., 2016). A few studies have documented that fine MPs can adhere to tissues [for example fish skin] and translocate across living cells into the circulatory system, dispersing the particles to other organs (Wang et al., 2020) and impacting the health of the cells and the immune system of the organisms (Wright and Kelly, 2017). Small MPs (<3 μm) have been found in both the digestive tract and muscles of painted comber (Serranus scriba) fish collected in the coast of Tunisia (Zitouni et al., 2020). MPs can affect several immune parameters such as the phagocytic activity, respiratory burst and the expression of immune-related genes in fishes (Espinosa et al., 2017; Choi et al., 2018). Therefore, it is important to study the impact of MPs on fish immune cells. An important aspect to consider when studying the toxicity of microplastics is the polymer type. The type of polymers ingested by an organism depends on its ecological niche. Pelagic fish tend to ingest low-density polymers since they normally float in seawater, while demersal fish preferably ingest polymers with higher densities than seawater as such particles sink and are mainly present in deep waters (Bråte et al., 2017). A few studies have reported that different MP polymers induce different toxicities in fish. A study on fathead minnow (Pimephales promelas) showed that both PS and PC nanoparticle aggregates (41 nm−1,710 μm) induced degranulation of primary granules of neutrophils, but only PC nanoparticles affected the oxidative burst of the cells (Greven et al., 2016). PVC-MP and PE-MP (40–150 μm) induced different responses in gilthead seabream (Sparus aurata) and European sea bass (Dicentrarchus labrax); while PVC-MPs decreased the phagocytic capacity of leucocytes, high concentrations (100 mg/ml) of PE-MPs significantly increased oxidative stress (Espinosa et al., 2018). In zebrafish, 10 days of exposure to 10 mg/L of PP-MP decreased the survival rate of the fish by 27%, while exposure to the same concentration of PA-MP, PE-MP, PVC-MP, and PS-MP (70 μm) did not affect survival (Lei et al., 2018). Recently, a study on Japanese medaka (Oryzias latipes) showed that environmental concentrations of a mixture of virgin PE-MP and PP-MP (~400 μm in size) affected development and behavior of the larvae. After 30 days of exposure to the plastic mixture, fish had a higher head/body length ratio than the control group, and their swimming speed was also affected (Pannetier et al., 2020). These findings highlight the need to study polymer-specific impacts on fish immunity.
Even though publications related to MP accumulation in fish and invertebrates have increased considerably since 2010 (Lusher et al., 2017b), research into how MPs affect living organisms is still in its infancy, and many questions remain unanswered. For instance, the effects of various types of polymers, the degree of uptake of MP by immune cells, the response of different tissues and organs to MP, and the response of the organisms to environmentally relevant concentrations of MPs, have to be delineated through further research.
In this work, we studied the impact of two MPs, PE and PS, on Atlantic salmon (Salmo salar L.), the commercially most important farmed marine fish species (FAO, 2018). A recent study revealed that PE accounted for ~40% of the MPs ingested by five fish species from the North and Baltic Sea (Rummel et al., 2016). Moreover, marine litter in Arctic, North Atlantic, North Sea, Skagerrak, and Baltic Sea is dominated by PS plastic (Strand et al., 2015). In addition, the weathering of plastic equipment used in aquaculture, such as cage floats or feed pipes that are mainly made of PE, is littering the marine environment (Lusher et al., 2017a). Hence, it is important to understand how Atlantic salmon, a farmed species that is produced mainly in open sea cages, is affected by PE and PS. We examined the physical impact of MPs in terms of uptake by cells. For this purpose, immune cells from three tissues, distal intestine (DI), blood, and head kidney (HK), were exposed to two types of polymers, PE-MP and PS-MP; either to each polymer singly or to a combination of the two, at three concentrations (50, 5, and 0.05 mg/L) for 1, 24, 48, and 72 h. The DI is the main entry point of MPs in the body, and blood cells can act as vehicles to carry MPs to other organs (Ding et al., 2018). The HK is one of the main organs where the hematopoiesis occurs and it is considered as a lymphoid organ, where immune cells are produced (Zapata et al., 2006; Whyte, 2007). After the exposure of leucocytes to the MPs, the mortality of the salmon immune cells as well as their phagocytic ability and capacity to ingest MP were determined by employing imaging flow cytometry. The present study sheds light on (1) the quantitative uptake of MPs by fish immune cells, (2) the response of immune cells exposed to two different polymers, (3) the effect of relatively low MP concentrations on immune cells, and (4) the organ-specific response of immune cells to MP exposure.
Materials and Methods
Microplastics and Reagents for Cell Studies
PS (2.1 ± 0.12 μm) and PE (1–5 μm) microspheres, were acquired from Magsphere Inc. (California, USA) and Cospheric (California, USA), respectively. PS-MPs had a more uniform sphericity than PE-MPs. The agglomeration rate of PS particles was 2.04 ± 2.12%, and of PE particles, 3.22 ± 1.32% (Supplementary File 1). In order to distinguish the two polymers, we obtained PS-MPs and PE-MPs that emit yellow and green fluorescence, respectively. For the exposure studies with each polymer, stock solutions (2.5 g/L, 250 mg/L, and 2.5 mg/L) were prepared prior to the experiments.
Leibovitz's L-15 Medium (L-15) (Sigma, Oslo, Norway), employed for cell isolation, was adjusted to a pH of 7.3 and osmolarity of 380 mOsm by adding a solution consisting of 5% (v/v) 0.41 M NaCl, 0.33 M NaHCO3, and 0.66 (w/v) D-glucose, and supplemented with 100 μg/mL gentamicin sulfate (Sigma), 2 mM L-glutamine (Sigma), 15 mM HEPES (Sigma), and 50 U/mL penicillin, 50 μg/mL streptomycin (Sigma). An enriched L-15 medium supplemented with 2% fetal bovine serum and 10 U/mL heparin (L-15+) was used to isolate and culture the cells from DI, blood and HK tissues. Percoll solutions (Sigma) adjusted to 380 mOsm by adding NaCl were also prepared for the cell isolation process. DTT (0.145 mg/mL dithiothreitol + 0.37 mg/mL EDTA in Ca2+ & Mg2+ free HBSS, Sigma), DNAse I (0.05 mg/mL; Sigma) and digestive (0.37 mg collagenase IV/mL washing medium, Thermo Fisher Scientific, Oslo, Norway) solutions were employed to extract cells from the DI.
Leucocyte Harvesting From Atlantic Salmon
Atlantic salmon smolts (60 g) were procured from Sundsfjord Smolt, Nygårdsjøen, Norway and transferred to the Research Station of Nord University, Bodø, Norway. The fish were maintained in a seawater flow-through system (7–8°C) and fed on commercial feeds (Skretting AS, Stavanger, Norway) for several months. Fish in the size range 500–800 g were then employed for the study. The selected fish were starved for 24 h and euthanized with an overdose of MS-222 (Tricaine methane sulphonate; Argent Chemical Laboratories, Redmond, USA; 160 mg/L) prior to cell extraction.
Immune cells from DI, blood and HK tissues were extracted as described by Attaya et al. (2018), Haugland et al. (2012), and Park et al. (2020), respectively, with some minor modifications. Briefly, 2 mL of blood from each individual was withdrawn with a heparinized syringe and mixed in 6 mL of L-15+. HK was dissected and placed in 4 mL of L-15+, and the cells were harvested by passing the tissue through a 40 μm cell strainer and washing twice with L-15+ by centrifugation (500 × g, 5 min, 4°C). The monocytes/macrophages from both the blood and the HK cell suspensions were then separated using Percoll 34/51% (by centrifugation; 500 × g, 30 min, 4°C) and then washed twice with L-15 by centrifugation (500 × g, 5 min, 4°C). The resulting cell layer was carefully collected and transferred to a petri dish for a 2-day incubation at 12°C to allow the macrophage-like cells to adhere. Thereafter, the supernatant was removed and the adherent cells on the petri dish were detached with ice-cold PBS supplemented with 5 mM EDTA. The collected cells were centrifuged (500 × g, 5 min, 4°C) and employed for the exposure experiment. As for the DI tissue, it was dissected out, washed with 1x PBS, and then incubated in 6 mL of DTT solution for 20 min at RT in order to remove as much mucus as possible. The tissue was washed with L-15+ supplemented with DNAse I to avoid cell clumping and remove excess DTT. The tissue fragment was then transferred into 6 mL of the digestive solution and placed on a shaking incubator (200 rpm) for 60 min at RT. The tissue and supernatant were then harvested by passing through a 100 μm cell strainer and the resulting cell suspension was washed twice with L-15+ by centrifugation (500 × g, 5 min, 4°C) and layered on a Percoll solution of 25/75%. After centrifugation (500 × g, 30 min, 4°C), the leucocyte-rich Percoll intermediate layer was collected, washed twice with L-15 by centrifugation (500 × g, 5 min, 4°C) and later used in the exposure experiment. For the exposure study, cells from 36 individuals were employed to prepare the 6 replicates of the experiment, each of which consisted of a random pool of cells from six fish.
Exposure Experiment
To understand the polymer-specific response of salmon immune cells, they were exposed to either PE-MP or PS-MP or a mixture of both (PE+PS-MP). The treatment employing a combination of PE-MP and PS-MP was selected to ascertain the synergetic or antagonistic effect of the two polymers. Three different concentrations of the polymer types were tested: high (50 mg/L), medium (5 mg/L), and low (0.05 mg/L) concentration. The low concentration was selected based on the highest concentration of MP reported for the North Pacific Gyre (California Current Ecosystem LTER and Goldstein, 2017). However, this concentration does not reflect the environmental concentration of the microplastic size used in this experiment since mesh size used for the sampling was 333 μm. The medium and high concentrations were higher than the concentrations found in the environment, but still lower than what is being used in several MP exposure experiments. A negative control (C), where cells were not exposed to MP, was also set up to ascertain the viability of the cells. DI (0.2 × 106 cells/100 μL), blood (0.5 × 106 cells/100 μL), and HK (0.5 × 106 cells/100 μL) cells were incubated in L-15+ along with the MPs at the different concentrations mentioned above, and the tubes with the cells were maintained at 12°C on a rotator (Rotator PTR-60, Grant-bio, Cambridge, IK) for 1, 24, 48, or 72 h. Six replicates per treatment were employed for the study.
After the exposure, the cells were analyzed by ImageStream®X Mk II Imaging Flow Cytometer (Luminex Corporation, Austin, TX, USA) as described by Park et al. (2020). Briefly, cells were washed by centrifugation (500 × g, 5 min, 4°C), resuspended in 50 μL of 1x PBS, and 1 μL of propidium iodide (PI, 1 mg/mL, Sigma) was added prior to loading the sample in the imaging flow cytometer to assess the mortality. A total of 30,000 images per sample were acquired with the 488 nm argon-ion laser set up at 0.15 mW to detect MP particles and dead cells. Cell mortality, phagocytic ability and phagocytic capacity were analyzed using IDEAS 6.1.822.0 software (Luminex) (Park et al., 2020). Cell mortality was determined as the percentage of cells with red fluorescence (stained by propidium iodide) among the total cells. The phagocytosis assay, consisting of phagocytic ability and phagocytic capacity, was used to study the uptake and bioaccumulation of MPs in the immune cells. The phagocytic ability was defined as the percentage of cells with MP particles inside (i.e., cells with green fluorescence, yellow fluorescence, or both). The phagocytic capacity was defined as the number of MP particles ingested by each phagocytic cell, which were detected by the function “spot count” of the IDEAS software. The phagocytic capacity was calculated and normalized using the following equation:
Where Ci is the number of phagocytic cells with i phagocytosed particles and n the total number of phagocytic cells. Cells with surface binding particles were counted as phagocytic cells and included in the phagocytic ability and capacity data analysis. Surface binding is considered an early stage of the phagocytosis since it triggers the physical exploration of the particle by the extension of pseudopods by the cells, the intracellular signaling and eventually the internalization of the particle (Underhill and Goodridge, 2012).
Statistical Analysis
Statistical analyses were done with the software RStudio 1.1.463. Poisson regression models were fitted to the data on cell mortality, phagocytic ability and phagocytic capacity. This approach helped us to understand the relationship between the response variable and the predictor variables. Data from each tissue were analyzed separately and statistical differences were considered significant at p < 0.05.
For the cell mortality (n = 6), two factor regressions were carried out, considering Time (1, 24, 48, and 72 h) and Treatment (C, PE-0.05, PE-5, PE-50, PS-0.05, PS-5, PS-50, PE+PS-0.05, PE+PS-5, and PE+PS-50) as the predictor variables. The factors Polymer type and Concentration were grouped into Treatment to avoid incorrect comparisons. For both the phagocytic ability (n = 6) and phagocytic capacity (n = 6), three factor regressions were carried out, considering Time (1, 24, and 48 h; 72 h was excluded), Polymer type (PE-MP, PS-MP, and PE+PS-MP) and Concentration (50, 5, and 0.05 mg/L) as the predictor variables. For the DI data, the Concentration 0.05 mg/L was excluded from both phagocytic ability and phagocytic capacity analyses due to the fewer number of samples with phagocytic cells.
For all three response variables and for each tissue, several Poisson regression models were fitted to detect the differences of interest. For this purpose, one specific level of each factor was fixed as first line in each model, with which the comparisons were made.
Results
Cell Mortality
Cell mortality was assessed to examine the response of leucocytes to MPs, in terms of time, polymer type and MP concentration. MP type or concentration was not found to have a significant effect on cell mortality. On the other hand, time affected the cell mortality significantly, based on the statistical analyses described below (Figure 1; Supplementary File 2, Supplementary Tables 1–3).
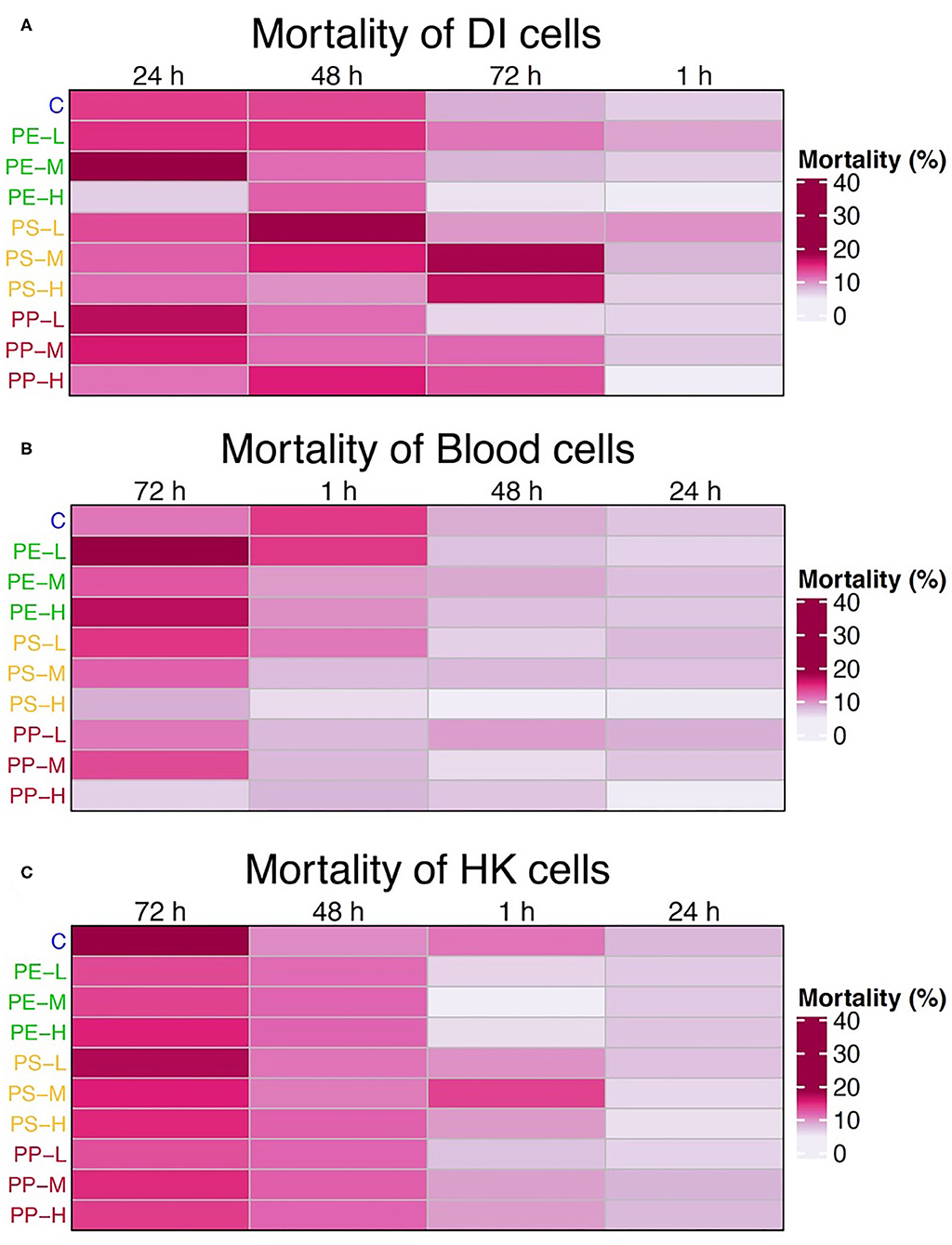
Figure 1. Heatmaps showing the average mortality of (A) intestinal, (B) blood, and (C) HK cells at 1, 24, 48, and 72 h for each treatment group. Time is arranged based on the decreasing level of mortality, from left to right. Cell treatments on the left of the map are color coded based on the polymer types: blue = control, green = PE, yellow = PS, and red (PP) = PE + PS; and ordered by concentration (L = 0.05 mg/L, M = 5 mg/L, and H = 50 mg/L). The mortality is expressed as the percentage of dead cells (cells with red fluorescence) from the total number of cells.
The mortality of DI cells increased significantly at 24 and 48 h compared to 1 h (p < 0.05) in 90% of the cases, while only 40% increased at 72 h compared to 1 h (p < 0.01). On the other hand, mortality was higher at 72 h for blood and HK cells. In the case of blood, mortality at 72 h was significantly higher in almost 50% of the cases (p < 0.05), and 24 h was the timepoint with the lowest mortality. For the HK cells, the mortality increased significantly at 72 h compared to 1 and 24 h (p < 0.05) in 95% of the cases, and at 48 h the mortality was significantly higher than 1 h (p < 0.05) in 40% of the cases. Yet the mortality at this timepoint was still lower than at 72 h.
Considering the general trend in mortality, the 72 h timepoint was not considered for the subsequent studies.
Microplastic Uptake
Immune cells from all the three tissues (DI, blood, and HK) of Atlantic salmon phagocytosed PE-MPs and PS-MPs of 1–5 μm in size (Figures 2–5). Phagocytosis was documented at different concentrations including the lower MP concentration (0.05 mg/L) (Figure 3). Single cells from blood and HK, but not from DI, had the capacity to phagocytose both PE-MPs and PS-MPs simultaneously (Figure 5).
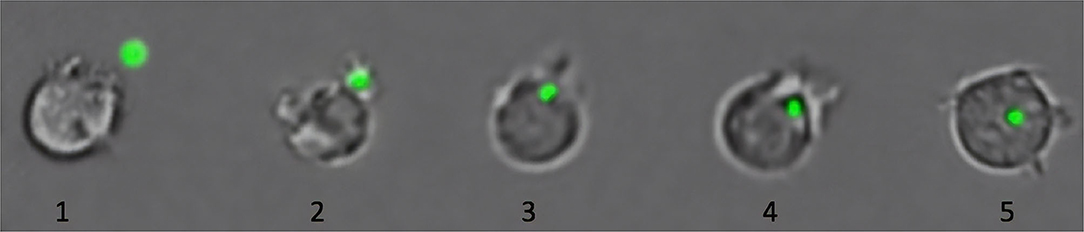
Figure 2. Phagocytosis of microplastics (MPs) by immune cells of Atlantic salmon. As shown in this figure, cells ingest MP (green fluorescent particle) by performing the first steps of phagocytosis: detection and recognition of the MP (1), attachment of the cell to the MP by formation of pseudopods (2), and internalization of the MP (3–5) (Esteban et al., 2015). The last steps of the phagocytosis, involved in the digestion of the particle, are not present due to the lack of specific pathways to degrade plastic (Gewert et al., 2015). The collated images are from representative pictures of five different cells captured at different phagocytic stages. Cells are not to scale.
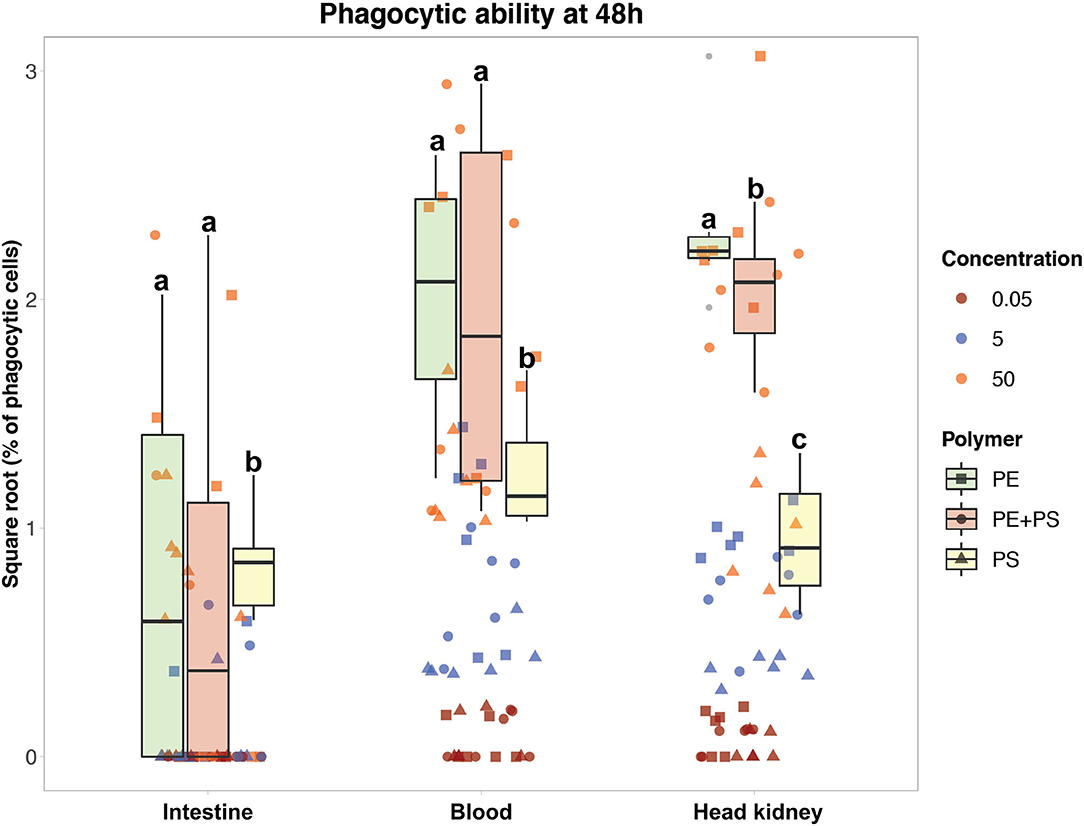
Figure 3. Phagocytic ability of Atlantic salmon immune cells after 48 h of exposure to MP. The color codes for the three MP concentrations are: maroon (0.05 mg/L), blue (5 mg/L), and orange (50 mg/L). The boxplots represent the phagocytic ability of the cells exposed to the high MP concentration (50 mg/L) of PE (green), PE + PS (red), and PS (yellow) at 48 h. Different letters above the box plots represent statistical differences in the ability of the cells from the same tissue to phagocytose different polymers.
Phagocytic Ability to PE-MP and PS-MP
The ability of immune cells from all three tissues to phagocytose different MP polymer types is shown in Figures 3, 4. The cells had higher phagocytic ability toward PE-MP than PS-MP, and in some cases the values for the former MP were six times higher than those observed for the latter polymer type. The phagocytic ability was found to increase with the MP concentration. In Figure 3, the phagocytic ability of the three concentrations at 48 h is shown, the timepoint with most distinct differential responses. Cells from all the three tissues presented higher MP uptake when the concentration increased independently of the polymer type; there were statistical differences for comparisons based on concentrations at all timepoints and polymer types (p < 0.001) (Supplementary File 2, Supplementary Tables 4–6).
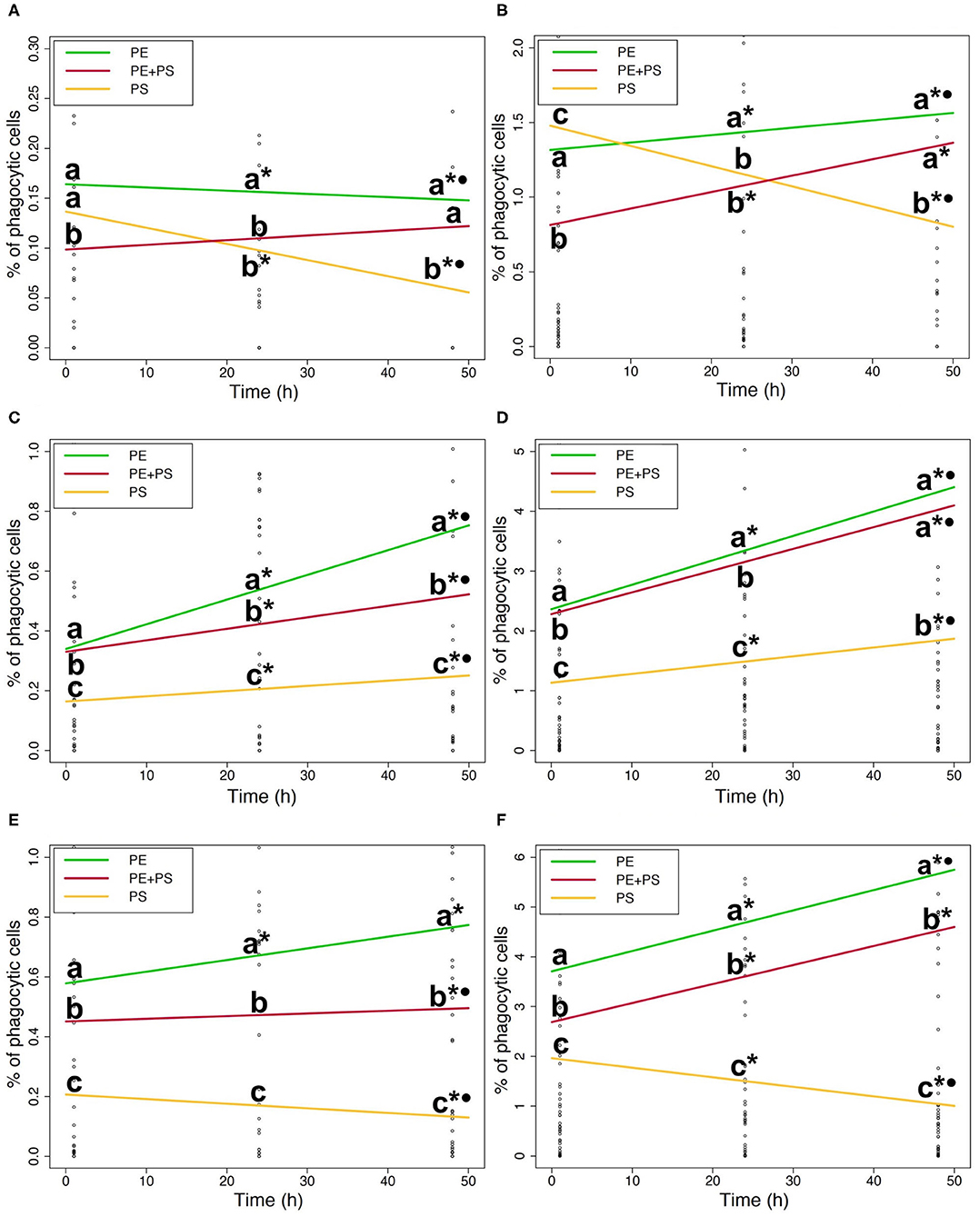
Figure 4. (A). Phagocytic ability of DI cells to microplastic (5 mg/L). (B). Phagocytic ability of DI cells to microplastic (50 mg/L). (C). Phagocytic ability of blood cells to microplastic (5 mg/L). (D). Phagocytic ability of blood cells to microplastic (50 mg/L). (E) Phagocytic ability of HK cells to microplastic (5 mg/L). (F) Phagocytic ability of HK cells to microplastic (50 mg/L). Phagocytic ability of DI (A,B), blood (C,D), and HK (E,F) cells exposed to the medium (left) and the high (right) MP concentrations. The lines show the general MP accumulation trends in time. The color codes for the MPs are: PE (green), PS (yellow), and PE + PS (red). Different letters above the lines represent statistical differences between polymer types at each time point. Asterisk and dot represent statistical differences between time points of a polymer type; *shows that there are differences compared to 1 h and •shows that there are differences compared to 24 h.
The ability of DI immune cells to phagocytose MP varied with the polymer type (Figures 4A,B). The phagocytic ability of cells exposed to high and medium concentrations of PS-MP decreased significantly with time (p < 0.05; * and • in Figures 4A,B). In contrast, at high concentrations of PE-MPs and PE+PS-MPs the phagocytic ability increased at 24 and 48 h compared to 1 h (p < 0.001; * in Figure 4B). PE-MPs were more phagocytosed than PS-MPs by DI cells exposed to high and medium concentrations at both 24 and 48 h (p < 0.001; a and b in Figures 4A,B). Similarly, cells exposed to high and medium concentrations of PE+PS-MPs had higher phagocytic ability than those exposed to PS-MPs at 48 h (p < 0.001; a and b in Figures 4A,B).
The phagocytic ability of blood cells exposed to high and medium concentrations of PE-MPs and PE+PS-MPs was significantly higher than to PS-MPs at all three timepoints (p < 0.001; a, b, and c in Figures 4C,D). However, differences in the ability to phagocytose PE-MPs and PE+PS-MPs varied depending on the concentration. Cells exposed to the medium concentration of PE-MPs presented a higher phagocytic ability than the mixture at all times (p < 0.05; a and b in Figure 4C). Nevertheless, at 48 h, the phagocytic ability of cells exposed to high concentrations of PE-MPs and PE+PS-MPs were similar (a at 48 h in Figure 4D). Overall, the ability of blood immune cells to phagocytose all the three polymer types increased at 24 and 48 h compared to 1 h (p < 0.05; * in Figures 4C,D). However, cells exposed to high and medium concentrations of PS-MPs had less phagocytic cells at 48 h compared to 24 h (p < 0.001).
The differences in the phagocytosis of different polymer types by the HK immune cells were distinct as shown in Figures 4E,F. The phagocytic ability of the cells exposed to high and medium concentrations of PE-MPs was significantly higher than those of PE+PS-MPs and PS-MPs at all times (p < 0.01; a, b, and c in Figures 4E,F). Moreover, low concentrations of PE-MPs induced a significantly higher phagocytic ability than PS-MPs (p < 0.05). Cells exposed to high and medium concentrations of PE+PS-MPs showed a higher phagocytic ability than those of cells exposed to PS-MPs (p < 0.001; b and c in Figures 4E,F). Different trends in time depending on the polymers were evident in HK cells (Figures 4E,F). The phagocytic ability to PE-MPs increased significantly with time after exposures to high and medium concentrations (p < 0.01; * and • in Figures 4E,F). By contrast, the phagocytic ability to medium and high concentrations of PS-MPs decreased with time (p < 0.05; * and • in Figures 4E,F). However, the phagocytic ability of HK cells exposed to high concentrations of PE+PS-MPs increased at 24 and 48 h (p < 0.001; * in Figure 4F) while that of the cells exposed to medium concentrations had lower phagocytic ability after 48 h of exposure (p < 0.001; *• in Figure 4E).
Overall, blood and HK cells had higher abilities to phagocytose MP (Figure 3). HK cells exposed to 50 mg/L of PE-MPs were found to have the highest phagocytic ability, observed at 48 h, with an average value of 5.5% (SD 2.0). The highest ability to phagocytose PS-MPs was observed at 24 h in blood cells exposed to 50 mg/L (1.9%, SD 1.0), and the highest ability to phagocytose PE+PS-MPs was found at 48 h in blood cells exposed to the high concentration (4.3%, SD 3.3). DI cells had the lowest phagocytic ability among the three tissues; those exposed to 50 mg/L of PE-MP had the highest phagocytic ability (2.0%, SD 1.6), at 24 h. Cells from all three tissues had a low degree of phagocytosis at 0.05 mg/L, the lower concentration of MP; few cells were able to phagocytose MP (0.08% was the highest phagocytic ability).
Phagocytic Capacity to PE-MP and PS-MP
The phagocytic capacity (i.e., the number of particles ingested by an immune cell) of Atlantic salmon to MP differed from tissue to tissue (Supplementary File 2, Supplementary Tables 7–9). DI immune cells had high phagocytic capacity to PE-MPs and PE+PS-MPs as compared to PS-MPs after 48 h of exposure to the highest MP concentration (p < 0.05). However, unlike the phagocytic ability, the phagocytic capacity of DI cells was not affected by the MP concentration.
Blood immune cells had higher phagocytic capacity to engulf PE-MPs at 24 h, while it was at 48 h for the other MPs (p < 0.05). However, statistical differences in the phagocytic capacity of blood cells to take up the different polymers were not evident, although PS-MPs were, in average, more ingested than the other MP types at both medium and high concentrations.
HK immune cells had the highest capacity to phagocytose MPs. While more than 7 PS-MP particles were observed inside these cells at 1 and 24 h of exposure, a maximum of five particles were seen in cells exposed to PE-MP for 24 h (Figure 5). Yet, the capacity of the cells to ingest the different MP polymer types did not vary significantly. The phagocytic capacity of HK cells increased with the MP concentration. Cells exposed to high concentrations of PE-MPs and PE+PS-MPs had higher phagocytic capacity than cells exposed to low concentrations at all timepoints (p < 0.05), as well as cells exposed to the medium concentration at 48 h (p < 0.05). However, HK cells exposed to 50 mg/L of PS-MPs only showed high phagocytic activity as compared to 0.05 and 5 mg/L at 1 and 24 h (p < 0.01).
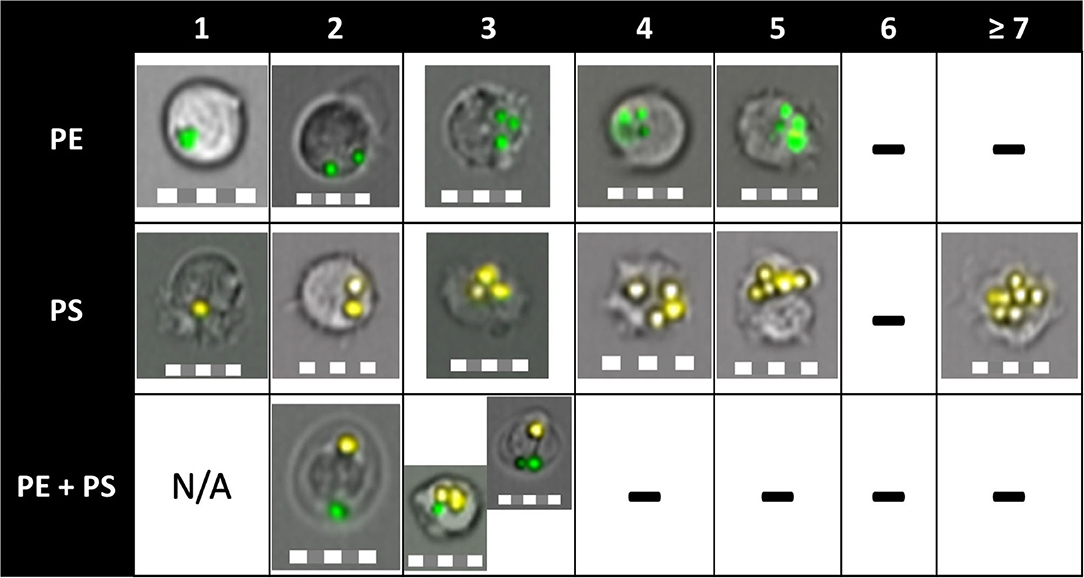
Figure 5. Phagocytic capacity of Atlantic salmon immune cells to ingest PE-MP, PS-MP, or both. The column names indicate the number of particles inside a cell. “-” indicate the absence of cells that engulfed that number of particles. Cells were able to ingest up to five PE particles and up to eight PS particles, which translated to a higher phagocytic capacity of the cells toward PS-MP. HK and blood cells were able to phagocytose two different polymer types at the same time (shown on 3rd row, engulfed PE-MP and PS-MP), a fact proven for the first time in this study. Pictures were obtained by imaging flow cytometry from all tissues, timepoints, and MP concentrations. Scale = 10 μm.
Discussion
The adverse effects of microplastics (MPs) on the immune system of animals have been intensively studied in recent years. Nevertheless, the physical response of cells to these small particles is poorly understood. This study aimed to provide new insights into the impact of MPs on the fish immune system at the cellular level. For this purpose, Atlantic salmon immune cells from DI, blood and HK were exposed to high, medium and low concentrations of PE-MPs, PS-MPs, and PE+PS-MPs for 1, 24, 48, and 72 h. Here we report for the first-time quantitative data on the accumulation of different MP polymer types in fish leucocytes, analyzed by imaging flow cytometry. The present study shows that Atlantic salmon immune cells can phagocytose MPs, although to a relatively low degree. Less than 6% of the cells exposed to high MP concentrations phagocytosed them, and a maximum of 0.08% of cells was found to phagocytose the MPs at low concentrations. The concentration had a clear effect on the response of immune cells exposed to MP, as observed in other organisms, including humans (Kögel et al., 2019). Increased release of MPs into the environment can therefore have more serious consequences than previously thought.
From the results of the present study, it is clear that the phagocytic ability and capacity of the cells from the different tissues of salmon are influenced by the type of polymer to which it is exposed. Overall, salmon immune cells had a higher ability to phagocytose PE-MPs compared to PS-MPs. PE-MPs bioaccumulated in the cells, while the number of cells with PS-MPs decreased over time. This indicates that the cells are able to discriminate the MP types, as discussed below. The decrease in the ability of the cells to phagocytose PS-MPs may be attributed to the process of exocytosis. Macrophages have been shown to exocytose nanoparticles of different nature (Oh and Park, 2014). In human cells, a time dependent exocytosis was observed, and ~66% of the endocytosed nanoparticles were exocytosed after 48 h of exposure (Asharani et al., 2009). However, exocytosis of particles, compared to endocytosis, is poorly understood and, to our knowledge, no studies are found that report exocytosis of MPs on fish. Nevertheless, a few recent studies have reported the excretion of PS-MPs by living organisms. Accumulated PS nanoparticles decreased after a few days of exposure of zebrafish embryos and larvae to the particles (Pitt et al., 2018). The authors suggested that 72 hpf larvae were able to excrete the PS through the gastrointestinal tract. Japanese medaka exposed to 10 μm PS-MPs was reported to egest 0.5–2% of the ingested microplastic per day (Zhu et al., 2020). Nevertheless, Japanese medaka exposed for 3 weeks to 2 μm PS-MPs still had significant amounts of particles in their intestine (Assas et al., 2020). A study carried out with Pacific oyster (Crassostrea gigas) found that PS-MPs did not accumulate in the gut and that the organism could egest these particles through the feces (Sussarellu et al., 2016). The shape of the microbeads was suggested to be the main factor determining the egestion of those particles by the oyster, and could, in turn, explain the higher phagocytic ability of the cells to PE-MPs than PS-MPs observed in this study. A study carried out on rat macrophages found that shape is the main factor regulating the phagocytosis, rather than the particle size. Spherical particles were phagocytosed less successfully than other shapes because the angle formed between the particle and the cell in the initial contact (Ω) was bigger (Champion and Mitragotri, 2006). The PS-MPs used in the present study had a uniform shape, with more than 80% of the particles showing a perfect sphericity, while the PE-MP particles were more irregularly shaped, with only 25% of the particles being spherical (Supplementary File 1). Hence, the higher ability of the cells to phagocytose PE-MPs could potentially be due to their lower Ω compared to PS-MPs. This would also explain the similar phagocytic response observed between cells exposed to PE+PS-MPs and PE-MPs. Cells exposed to PE+PS-MPs phagocytosed PE-MPs faster and more successfully than PS-MPs, relatively masking the effect of the latter particles on the cells. This hypothesis became evident after looking into the cells exposed to PE+PS-MPs at 1 h and comparing the number of phagocytic cells with internalized PE-MP with the ones with internalized PS-MPs (Supplementary Figure). The roughness and shape are hence important factors to consider when assessing the toxicity of MPs, as it has also been reported in in vivo studies (Choi et al., 2018). This is especially relevant since <1% of the MPs found in marine waters are spherical (Isobe, 2016). The higher phagocytic ability of the cells to PE-MPs could also be due to the less uniform size of these particles (1–5 μm) compared to the PS-MPs (2.1 μm). However, analyses of the images of the cells with internalized PE-MPs did not reveal any evidence of cells ingesting smaller particles (1 μm) compared to larger particles (5 μm). Similarly, mice macrophages exposed to 1.2 and 5.2 μm PS-MPs did not show differences in the phagocytic ability to these particles (Tomazic-Jezic et al., 2001). Nevertheless, the authors found differences when they assessed the ability of bigger particles (12.5 μm). Another important factor to consider when analyzing the results of the phagocytic ability is the number of particles/L. The concentration of microplastic added to each sample was calculated in mg/L, but since the density of PE and PS is different, cells incubated with PE-MP were not exposed to the same number of particles as cells exposed to PS-MP. An estimation of the number of particles/L was done to assess whether the higher phagocytic ability of the cells toward PE-MP could be due to the higher number of particles/L. Our calculation revealed that cells exposed to 50, 5, and 0.05 mg/L of PE-MP were exposed to 4.8 × 109, 4.8 × 108, and 4.8 × 106 particles/L respectively, while cells exposed to PS-MP were exposed to more particles/L in comparison (1.75 × 1010, 1.75 × 109, and 1.75 × 107, respectively). These results underline that cells had a higher response to PE-MPs than to PS-MPs.
As for the phagocytic capacity, blood and HK cells generally ingested more PS-MP particles than PE-MPs. The higher phagocytic capacity of cells toward PS-MPs could be related to the polarity of the polymer, as previously suggested for PS and PC micro- and nanoparticles (Greven et al., 2016). PS tends to become positively charged when it is in contact with water, while PE is consider a non-polar polymer and its charges remain stable (Albrecht et al., 2009). The positively charged PS have higher ability to interact with the cells, stimulating its uptake by cells. Positively charged particles show higher interaction with the cells because of electrostatic attraction, given the negative charge of the cell plasma membrane. Moreover, the binding of PS could be associated to hydrogen bond formation between the charged particles and the hydrophilic region of the phospholipids from the plasma membrane, as suggested for cellulose (Bhattacharya et al., 2010; Ma et al., 2017). Thus, the higher phagocytic capacity of the cells toward the PS-MPs could partly be explained by the polarity of the polymer.
Immune cells from the three tissues responded differently to MPs. Blood and HK cells showed a higher ability to phagocytose MPs compared to DI cells (Figure 3). DI immune cells consisted of different leucocytes, and not all of them were phagocytic. On the other hand, the harvested adherent cells from blood and HK were mainly monocytes/macrophages, the main phagocytic cells composing the leucocytes along with the neutrophils (Delves and Roitt, 2000). This abundance in macrophages would explain the main difference in phagocytic ability observed between tissues. However, in Atlantic salmon, the percentage of monocytes/macrophages found in the leucocytes is only 10% lower than the percentage of monocytes/macrophages found in the adherent cells isolated with the protocol used in the present study (Park et al., 2020). Hence, differences in the phagocytic ability of cells from different tissues could also be explained by the principle of organ-specific innate immunity. This principle states that the activation of innate immunity differs between different organs, which have specialized mechanisms to achieve a more efficient response (Raz, 2007). The lower ability of fish DI cells to phagocytose MPs could be a beneficial adaptation to prevent or reduce the uptake of particles from the food. Several fish species ingest and accumulate MPs in the digestive tract (Bråte et al., 2017) as well as in several other tissues, such as the gills, liver and even in the brain (Ding et al., 2018). It has been suggested that MPs translocate from the gut into the circulatory system in fish, which in turn will transport them to other tissues and organs (Ding et al., 2018; Wang et al., 2020). The present study provides new understanding of the MP accumulation potential in Atlantic salmon immune cells and sheds light on potential entry routes for MP particles.
Cell mortality increased at 72 h in all the groups, including the control group. Hence inferences about the cytotoxicity of the MPs based on the mortality of the control group cannot be drawn. Nevertheless, Figure 2 suggests that MP did not induce any clear polymer- or concentration-dependent signs of cytotoxicity in the studied salmon immune cells. A study on gilthead seabream and European sea bass leucocytes reported no decrease in viability of cells exposed to high concentrations (1, 10, and 100 g/L) of PE-MP and PVC-MP for 1 and 24 h. However, immune parameters such as respiratory burst and phagocytic capacity of the cells were affected (Espinosa et al., 2018). A sublethal effect of MP on fish immune cells was also observed in fathead minnow, where exposure of neutrophils to PS or PC micro- and nanoparticles induced cell degranulation as well as oxidative burst activity (Greven et al., 2016). In the present study, we used fluorescent-dyed MPs and therefore did not measure any additional parameters other than cell mortality. Earlier studies have shown that some fluorochromes can leach and accumulate in tissues (Catarino et al., 2019), and influence the superoxide production as well as other immune parameters directly (De Clerck et al., 1994). Our focus was to understand the physical impact of salmon immune cells to different types of MP polymers, by assessing the phagocytic ability and capacity of the cells.
In the present study, we found that MPs can impact the immune cells of Atlantic salmon even at relatively low concentrations and that different polymers can induce distinct responses on the cells. Exposure of farmed salmon to MP can have economic implications for this prime aquaculture industry (FAO, 2018), especially if the MP particles act as vectors for contaminants (Browne et al., 2013). Moreover, plastic is widely used in aquaculture and MP released from sources such as feeding pipes into the surrounding waters may potentially be ingested by the farmed fish (Lusher et al., 2017a; Gomiero et al., 2020). We found that PE-MP accumulates more easily than PS-MP in the immune cells of the Atlantic salmon. PE is the most abundant MP polymer in the marine environment (Enders et al., 2015; Ter Halle et al., 2017; Erni-Cassola et al., 2019; Pannetier et al., 2020) and, therefore, the most often ingested polymer by fishes (Rummel et al., 2016; Bråte et al., 2017). Hence, the impact of PE-MPs on wild populations of salmonid species cannot be ignored.
Conclusion
The present study used an imaging flow cytometry approach to understand how different MP polymers are taken up by fish cells. This technique enabled us to prove for the first time that a single cell can phagocytose two different microplastic polymer types simultaneously. In conclusion, Atlantic salmon immune cells isolated from different tissues phagocytosed microplastic polymers, even at relatively low concentrations. Polyethylene microparticles were more easily ingested than polystyrene ones. With increasing amounts of plastic debris in the marine environment, the study highlights how their uptake into the tissues can lead to damaging effects on aquatic life. The methodology adopted in the present study opens new possibilities for observing the impact of micro and nanoplastic on several species, including humans.
Data Availability Statement
The raw data supporting the conclusions of this article will be made available by the authors, without undue reservation.
Ethics Statement
Ethical review and approval was not required for the animal study because, in accordance the Norwegian regulation of the Research Animal Act (FOR-2015-06-18-761). The Approval of trials regulation §6 states that approval requirement does not apply to experiments involving only the killing of animals to use organs or tissues from them. Live fish was handled by personnel with FELASA-C course, based on the policies by the Federation of European Laboratory Animal Science Association.
Author Contributions
IA-G, PO, and VK: conceptualization and design of the study. IA-G and YP: methodology, protocols, and experiment set up. IA-G: analysis of data and writing. IA-G, YP, VK, and PO: review and editing. All authors contributed to the article and approved the submitted version.
Conflict of Interest
The authors declare that the research was conducted in the absence of any commercial or financial relationships that could be construed as a potential conflict of interest.
Acknowledgments
Bisa Saraswathy from Nord University and Nicolas Dupont from UiO (University of Oslo) are acknowledged for the suggestions and help provided for the statistical analysis of the data. The support from Virginia Abellán Zapata on the mathematic formulation is also appreciated.
Supplementary Material
The Supplementary Material for this article can be found online at: https://www.frontiersin.org/articles/10.3389/fenvs.2020.560206/full#supplementary-material
References
Albrecht, V., Janke, A., Németh, E., Spange, S., Schubert, G., and Simon, F. (2009). Some aspects of the polymers' electrostatic charging effects. J. Electrostat. 67, 7–11. doi: 10.1016/j.elstat.2008.10.002
Alimba, C. G., and Faggio, C. (2019). Microplastics in the marine environment: current trends in environmental pollution and mechanisms of toxicological profile. Environ. Toxicol. Pharmacol. 68, 61–74. doi: 10.1016/j.etap.2019.03.001
Arthur, C., Baker, J. E., and Bamford, H. A. (2009). Proceedings of the International Research Workshop on the Occurrence, Effects, and Fate of Microplastic Marine Debris, September 9–11, 2008. Tacoma, WA: University of Washington Tacoma.
Asharani, P., Hande, M. P., and Valiyaveettil, S. (2009). Anti-proliferative activity of silver nanoparticles. BMC Cell Biol. 10:65. doi: 10.1186/1471-2121-10-65
Assas, M., Qiu, X., Chen, K., Ogawa, H., Xu, H., Shimasaki, Y., et al. (2020). Bioaccumulation and reproductive effects of fluorescent microplastics in medaka fish. Marine Pollut. Bullet. 158, 111446. doi: 10.1016/j.marpolbul.2020.111446
Attaya, A., Wang, T., Zou, J., Herath, T., Adams, A., Secombes, C. J., et al. (2018). Gene expression analysis of isolated salmonid GALT leucocytes in response to PAMPs and recombinant cytokines. Fish Shellfish Immunol. 80, 426–436. doi: 10.1016/j.fsi.2018.06.022
Bhattacharya, P., Lin, S., Turner, J. P., and Ke, P. C. (2010). Physical adsorption of charged plastic nanoparticles affects algal photosynthesis. J. Phys. Chem. C 114, 16556–16561. doi: 10.1021/jp1054759
Bråte, I. L. N., Huwer, B., Thomas, K. V., Eidsvoll, D. P., Halsband, C., Almroth, B. C., et al. (2017). Micro-and Macro-Plastics in Marine Species From Nordic Waters. Copenhagen: Nordic Council of Ministers. doi: 10.6027/TN2017-549
Browne, M. A., Niven, S. J., Galloway, T. S., Rowland, S. J., and Thompson, R. C. (2013). Microplastic moves pollutants and additives to worms, reducing functions linked to health and biodiversity. Curr. Biol. 23, 2388–2392. doi: 10.1016/j.cub.2013.10.012
California Current Ecosystem LTER and Goldstein, M. (2017). Numerical (No m-3) concentrations (mg m-3) of subsurface microplastic debris collected by bongo net aboard the Scripps Environmental Accumulation of Plastic Expedition (SEAPLEX) cruise, August 2009. ver 2. Environ. Data Initiative. doi: 10.6073/pasta/67b4659a0fc883d40f12849090e1c011
Catarino, A. I., Frutos, A., and Henry, T. B. (2019). Use of fluorescent-labelled nanoplastics (NPs) to demonstrate NP absorption is inconclusive without adequate controls. Sci. Total Environ. 670, 915–920. doi: 10.1016/j.scitotenv.2019.03.194
Champion, J. A., and Mitragotri, S. (2006). Role of target geometry in phagocytosis. Proc. Natl. Acad. Sci. U. S. A. 103, 4930–4934. doi: 10.1073/pnas.0600997103
Choi, J. S., Jung, Y.-J., Hong, N.-H., Hong, S. H., and Park, J.-W. (2018). Toxicological effects of irregularly shaped and spherical microplastics in a marine teleost, the sheepshead minnow (Cyprinodon variegatus). Marine Pollut. Bullet. 129, 231–240. doi: 10.1016/j.marpolbul.2018.02.039
De Clerck, L. S., Bridts, C. H., Mertens, A. M., Moens, M. M., and Stevens, W. J. (1994). Use of fluorescent dyes in the determination of adherence of human leucocytes to endothelial cells and the effect of fluorochromes on cellular function. J. Immunol. Method. 172, 115–124. doi: 10.1016/0022-1759(94)90384-0
Delves, P. J., and Roitt, I. M. (2000). The immune system. N. Engl. J. Med. 343, 37–49. doi: 10.1056/NEJM200007063430107
Ding, J., Zhang, S., Razanajatovo, R. M., Zou, H., and Zhu, W. (2018). Accumulation, tissue distribution, and biochemical effects of polystyrene microplastics in the freshwater fish red tilapia (Oreochromis niloticus). Environ. Pollut. 238, 1–9. doi: 10.1016/j.envpol.2018.03.001
Enders, K., Lenz, R., Stedmon, C. A., and Nielsen, T. G. (2015). Abundance, size and polymer composition of marine microplastics ≥10μm in the Atlantic Ocean and their modelled vertical distribution. Marine Pollut. Bullet. 100, 70–81. doi: 10.1016/j.marpolbul.2015.09.027
Erni-Cassola, G., Zadjelovic, V., Gibson, M. I., and Christie-Oleza, J. A. (2019). Distribution of plastic polymer types in the marine environment: a meta-analysis. J. Hazard. Mater. 369, 691–698. doi: 10.1016/j.jhazmat.2019.02.067
Espinosa, C., Beltrán, J. M. G., Esteban, M. A., and Cuesta, A. (2018). In vitro effects of virgin microplastics on fish head-kidney leucocyte activities. Environ. Pollut. 235, 30–38. doi: 10.1016/j.envpol.2017.12.054
Espinosa, C., Cuesta, A., and Esteban, M. Á. (2017). Effects of dietary polyvinylchloride microparticles on general health, immune status and expression of several genes related to stress in gilthead seabream (Sparus aurata L.). Fish Shellfish Immunol. 68, 251–259. doi: 10.1016/j.fsi.2017.07.006
Esteban, M. Á., Cuesta, A., Chaves-Pozo, E., and Meseguer, J. (2015). Phagocytosis in teleosts. Implications of the new cells involved. Biology 4, 907–922. doi: 10.3390/biology4040907
FAO (2018). The State of World Fisheries and Aquaculture 2018-Meeting the sustainable development goals. Rome: FAO.
Foley, C. J., Feiner, Z. S., Malinich, T. D., and Höök, T. O. (2018). A meta-analysis of the effects of exposure to microplastics on fish and aquatic invertebrates. Sci. Total Environ. 631, 550–559. doi: 10.1016/j.scitotenv.2018.03.046
GESAMP (2015). “Sources, fate and effects of microplastics in the marine environment: a global assessment,” in IMO/FAO/UNESCO-IOC/UNIDO/WMO/IAEA/UN/UNEP/UNDP Joint Group of Experts on the Scientific Aspects of Marine Environmental Protection, ed P. J. Kershaw. Rep. Stud. GESAMP No. 90, 96. Available online at: https://ec.europa.eu/environment/marine/good-environmental-status/descriptor-10/pdf/GESAMP_microplastics%20full%20study.pdf
Gewert, B., Plassmann, M. M., and Macleod, M. (2015). Pathways for degradation of plastic polymers floating in the marine environment. Environ. Sci. Process. Impacts 17, 1513–1521. doi: 10.1039/C5EM00207A
Gomiero, A., Haave, M., Kögel, T., Bjorøy, Ø., Gjessing, M., Lea, T. B., et al. (2020). TRACKing of PLASTtic Emissions From Aquaculture Industry. Bergen: TrackPlast.
Greven, A. C., Merk, T., Karagöz, F., Mohr, K., Klapper, M., Jovanović, B., et al. (2016). Polycarbonate and polystyrene nanoplastic particles act as stressors to the innate immune system of fathead minnow (Pimephales promelas). Environ. Toxicol. Chem. 35, 3093–3100. doi: 10.1002/etc.3501
Hartmann, N. B., HüFfer, T., Thompson, R. C., Hassellö, V. M., Verschoor, A., et al. (2019). Are we speaking the same language? recommendations for a definition and categorization framework for plastic debris. Environ. Sci. Technol. 53, 1039–1047. doi: 10.1021/acs.est.8b05297
Haugland, G. T., Jordal, A.-E. O., and Wergeland, H. I. (2012). Characterization of small, mononuclear blood cells from salmon having high phagocytic capacity and ability to differentiate into dendritic like cells. PLoS ONE 7:e49260. doi: 10.1371/journal.pone.0049260
Isobe, A. (2016). Percentage of microbeads in pelagic microplastics within Japanese coastal waters. Marine Pollut. Bullet. 110, 432–437. doi: 10.1016/j.marpolbul.2016.06.030
Kögel, T., Bjorøy, Ø., Toto, B., Bienfait, A. M., and Sanden, M. (2019). Micro-and nanoplastic toxicity on aquatic life: determining factors. Sci. Total Environ. 709:136050. doi: 10.1016/j.scitotenv.2019.136050
Lei, L., Wu, S., Lu, S., Liu, M., Song, Y., Fu, Z., et al. (2018). Microplastic particles cause intestinal damage and other adverse effects in zebrafish Danio rerio and nematode Caenorhabditis elegans. Sci. Total Environ. 619, 1–8. doi: 10.1016/j.scitotenv.2017.11.103
Lu, Y., Zhang, Y., Deng, Y., Jiang, W., Zhao, Y., Geng, J., et al. (2016). Uptake and accumulation of polystyrene microplastics in zebrafish (Danio rerio) and toxic effects in liver. Environ. Sci. Technol. 50, 4054–4060. doi: 10.1021/acs.est.6b00183
Lusher, A., Hollman, P., and Mendoza-Hill, J. (2017a). Microplastics in Fisheries and Aquaculture: Status of Knowledge on Their Occurrence and Implications for Aquatic Organisms and Food Safety. Rome: FAO Fisheries and Aquaculture Technical Paper.
Lusher, A., Welden, N., Sobral, P., and Cole, M. (2017b). Sampling, isolating and identifying microplastics ingested by fish and invertebrates. Analyt. Method. 9, 1346–1360. doi: 10.1039/C6AY02415G
Ma, Y., Poole, K., Goyette, J., and Gaus, K. (2017). Introducing membrane charge and membrane potential to T cell signaling. Front. Immunol. 8:1513. doi: 10.3389/fimmu.2017.01513
Oh, N., and Park, J.-H. (2014). Endocytosis and exocytosis of nanoparticles in mammalian cells. Int. J. Nanomed. 9:51. doi: 10.2147/IJN.S26592
Pannetier, P., Morin, B., Le Bihanic, F., Dubreil, L., Clérandeau, C., Chouvellon, F., et al. (2020). Environmental samples of microplastics induce significant toxic effects in fish larvae. Environ. Int. 134:105047. doi: 10.1016/j.envint.2019.105047
Park, Y., Abihssira-García, I. S., Thalmann, S., Wiegertjes, G., Barreda, D., Olsvik, P. A., et al. (2020). Imaging flow cytometry protocols for examining phagocytosis of microplastics and bioparticles by immune cells of aquatic animals. Front. Immunol. 11:203. doi: 10.3389/fimmu.2020.00203
Pitt, J. A., Kozal, J. S., Jayasundara, N., Massarsky, A., Trevisan, R., Geitner, N., et al. (2018). Uptake, tissue distribution, and toxicity of polystyrene nanoparticles in developing zebrafish (Danio rerio). Aquatic Toxicol. 194, 185–194. doi: 10.1016/j.aquatox.2017.11.017
Raz, E. (2007). Organ-specific regulation of innate immunity. Nat. Immunol. 8:3. doi: 10.1038/ni0107-3
Rummel, C. D., Löder, M. G. J., Fricke, N. F., Lang, T., Griebeler, E.-M., Janke, M., et al. (2016). Plastic ingestion by pelagic and demersal fish from the North Sea and Baltic Sea. Marine Pollut. Bullet. 102, 134–141. doi: 10.1016/j.marpolbul.2015.11.043
Strand, J., Tairova, Z., Danielsen, J., Hansen, J. W., Magnusson, K., Naustvoll, L.-J., et al. (2015). Marine Litter in Nordic Waters. Copenhagen: Nordisk Ministerråd. doi: 10.6027/TN2015-521
Suaria, G., Avio, C. G., Mineo, A., Lattin, G. L., Magaldi, M. G., Belmonte, G., et al. (2016). The Mediterranean Plastic Soup: synthetic polymers in Mediterranean surface waters. Sci. Rep. 6:37551. doi: 10.1038/srep37551
Sussarellu, R., Suquet, M., Thomas, Y., Lambert, C., Fabioux, C., Pernet, M. E. J., et al. (2016). Oyster reproduction is affected by exposure to polystyrene microplastics. Proc. Natl. Acad. Sci. U. S. A. 113, 2430–2435. doi: 10.1073/pnas.1519019113
Ter Halle, A., Jeanneau, L., Martignac, M., Jardé, E., Pedrono, B., Brach, L., et al. (2017). Nanoplastic in the North Atlantic subtropical gyre. Environ. Sci. Technol. 51, 13689–13697. doi: 10.1021/acs.est.7b03667
Tomazic-Jezic, V. J., Merritt, K., and Umbreit, T. H. (2001). Significance of the type and the size of biomaterial particles on phagocytosis and tissue distribution. J. Biomed. Mater. Res. 55, 523–529. doi: 10.1002/1097-4636(20010615)55:4<523::AID-JBM1045>3.0.CO;2-G
Underhill, D. M., and Goodridge, H. S. (2012). Information processing during phagocytosis. Nat. Rev. Immunol. 12, 492–502. doi: 10.1038/nri3244
UNEP (2016). UNEP Frontiers 2016 Report: Emerging Issues of Environmental Concern. Nairobi: United Nations Environment Programme.
Von Moos, N., Burkhardt-Holm, P., and KöHler, A. (2012). Uptake and effects of microplastics on cells and tissue of the blue mussel Mytilus edulis L. after an experimental exposure. Environ. Sci. Technol. 46, 11327–11335. doi: 10.1021/es302332w
Wang, W., Ge, J., and Yu, X. (2020). Bioavailability and toxicity of microplastics to fish species: a review. Ecotoxicol. Environ. Safety 189:109913. doi: 10.1016/j.ecoenv.2019.109913
Whyte, S. K. (2007). The innate immune response of finfish–a review of current knowledge. Fish Shellfish Immunol. 23, 1127–1151. doi: 10.1016/j.fsi.2007.06.005
Wright, S. L., and Kelly, F. J. (2017). Plastic and human health: a micro issue? Environ. Sci. Technol. 51, 6634–6647. doi: 10.1021/acs.est.7b00423
Zapata, A., Diez, B., Cejalvo, T., Gutierrez-De Frias, C., and Cortes, A. (2006). Ontogeny of the immune system of fish. Fish Shellfish Immunol. 20, 126–136. doi: 10.1016/j.fsi.2004.09.005
Zhu, M., Chernick, M., Rittschof, D., and Hinton, D. E. (2020). Chronic dietary exposure to polystyrene microplastics in maturing Japanese medaka (Oryzias latipes). Aquatic Toxicol. 220:105396. doi: 10.1016/j.aquatox.2019.105396
Zitouni, N., Bousserrhine, N., Belbekhouche, S., Missawi, O., Alphonse, V., Boughatass, I., et al. (2020). First report on the presence of small microplastics (≤ 3 μm) in tissue of the commercial fish Serranus scriba (Linnaeus. 1758) from Tunisian coasts and associated cellular alterations. Environ. Pollut. 236:114576. doi: 10.1016/j.envpol.2020.114576
Keywords: microplastic (MP), ecotoxicology, phagocytosis, imaging flow cytometry (IFC), Atlantic salmon (Salmo salar L.), polystyrene (PS), polyethylene (PE), immune cells
Citation: Abihssira-García IS, Park Y, Kiron V and Olsvik PA (2020) Fluorescent Microplastic Uptake by Immune Cells of Atlantic Salmon (Salmo salar L.). Front. Environ. Sci. 8:560206. doi: 10.3389/fenvs.2020.560206
Received: 08 May 2020; Accepted: 27 October 2020;
Published: 20 November 2020.
Edited by:
Cristina Panti, University of Siena, ItalyReviewed by:
Zhiquan Liu, East China Normal University, ChinaMatteo Baini, University of Siena, Italy
Copyright © 2020 Abihssira-García, Park, Kiron and Olsvik. This is an open-access article distributed under the terms of the Creative Commons Attribution License (CC BY). The use, distribution or reproduction in other forums is permitted, provided the original author(s) and the copyright owner(s) are credited and that the original publication in this journal is cited, in accordance with accepted academic practice. No use, distribution or reproduction is permitted which does not comply with these terms.
*Correspondence: Isabel S. Abihssira-García, aXNhYmVsLnMuYWJpaHNzaXJhLWdhcmNpYUBub3JkLm5v