In or Out of Equilibrium? How Microbial Activity Controls the Oxygen Isotopic Composition of Phosphate in Forest Organic Horizons With Low and High Phosphorus Availability
- 1Institute of Agricultural Science, ETH Zürich, Zurich, Switzerland
- 2Eco&Sols, Montpellier SupAgro, Univ Montpellier, CIRAD, INRAE, IRD, Montpellier, France
- 3Department of Biosystems Science and Engineering, ETH Zürich, Basel, Switzerland
- 4Department of Soil Sciences, Research Institute of Organic Agriculture FiBL, Frick, Switzerland
While there are estimates of the abiotic processes contribution to soil phosphorus (P) availability, less is known about the contribution of biological processes. Two main enzymatic processes involved in soil P cycling are known to alter the oxygen isotopic composition of phosphate (δ18O-P), each in a different way, through the cleavage of the P–O bond: the intracellular P turnover and the organic P hydrolysis. The former induces isotopic equilibration between phosphate and water and is considered the major process affecting soil available P via microbial P release. The latter induces depleted δ18O-P in the phosphate released from the mineralization of organic P. We studied P dynamics in organic horizons of two contrasting soils (low- and high-P availability) from temperate beech forests. We labeled the soil with 18O-enriched water and followed changes in the δ18O-P of different soil P pools in the presence or absence of added leaf litter during 3 months of incubation. δ18O-P values of almost all P pools progressively increased indicating oxygen incorporation from the enriched soil water into phosphate via the above-mentioned enzymatic processes. δ18O-P of available P increased more in the P-rich soil than in the P-poor soil and approached the isotopic equilibrium between phosphate and water, revealing the impact of microbial P release into the available P pool. However, in the P-poor soil, the available P brought the isotopic signature induced by phosphatase enzymes, indicating that it was mostly originated from the hydrolysis of organic P. Therefore, under P-limiting conditions, the isotopic effect of organic P hydrolysis can outweigh the isotopic equilibrium effect. Finally, two independent isotopic approaches with 33P and δ18O-P provided very similar estimates of P exchanged between the available P and other inorganic soil pools. This suggests that δ18O-P can be successfully used to trace P fluxes, provided that the underlying processes do not break the P–O bonds of the phosphate molecule.
Introduction
In forest ecosystems, plants and their mycorrhizae take up most of their phosphorus (P) as dissolved orthophosphate (PO43–, hereafter phosphate) from the soil solution, which represents often a small proportion of the total P in soil. The soil solution is continuously replenished by abiotic processes, such as the release of P from sorbed phases, by the dissolution of P containing minerals, and by biological processes, namely mineralization of organic P (Po). Microbes mineralize Po from plant litter and soil organic matter via enzymatic hydrolysis. The newly released P is taken up by microbes (immobilization), sorbed to the soil solid phases or replenishes the soil solution. Upon cell death, microbial P ultimately enters the non-living Po and inorganic P pools, thus constituting a potentially available P pool.
While there are estimates of the contribution of abiotic inorganic P pools to P availability (Helfenstein et al., 2020), less is known about the contribution of biological processes. This contribution varies widely, depending on factors such as land-use and inorganic P availability (Becquer et al., 2014; Bünemann, 2015; Pistocchi et al., 2018). Integrating this knowledge into soil P cycling models is crucial to predict the effects of changing environmental conditions, such as the ones induced by climate change, or of global P resources scarcity on net primary production.
Assessing soil P dynamics is challenging, because exchanges between P pools often occur without net changes in pool size. The use of radioactive tracers 33P and 32P is to date the sole option to quantify gross P fluxes, such as gross P immobilization and mineralization (Bünemann, 2015 and references therein), the transfer from the soil to the plant (Frossard et al., 2011 and references therein) and the fate of P added with plant residues or fertilizers (Fardeau et al., 1995; Daroub et al., 2000; Pistocchi et al., 2018). Studies on various forest soils have highlighted the impact of Po mineralization on P fluxes notably when inorganic P availability is low (Achat et al., 2009; Mooshammer et al., 2012; Spohn et al., 2013; Bünemann et al., 2016; Pistocchi et al., 2018).
Although radioisotopes have several advantages (e.g., negligible P mass addition, direct P tracing, simple to analyze), they also have several drawbacks related to safety issues, short half-lives, and low sensitivity to biological P fluxes when the baseline of isotopic dilution, i.e., the flux due to abiotic processes, is high (Pistocchi et al., 2018; Siegenthaler et al., 2020).
In the last two decades, many studies have shown that biological processes involved in soil P cycling alter the isotopic composition of oxygen in phosphate (δ18O-P) through the cleavage of the P–O bond and the incorporation of O atoms from water (Tamburini et al., 2012 and references therein). Whereas in the absence of biological activity, the P–O bond is stable and exchanges of oxygen (O) between phosphate and water are negligible. Two main enzyme-mediated processes have an impact on δ18O-P. Firstly, intracellular P turnover controlled by the enzyme inorganic pyrophosphatase leads to the complete exchange of the four O atoms in phosphate with O from water. This reaction produces a temperature-dependent isotopic equilibrium between phosphate and water (Longinelli and Nuti, 1973; Chang and Blake, 2015).
Secondly, the intra- and extracellular phosphatase enzymes incorporate one or two O atoms from water into phosphate released by the breakdown of Po compounds (P mono- and diesters). One O atom is incorporated during the hydrolysis of a phosphomonoester and two O atoms are incorporated during the hydrolysis of a phosphodiester. These transfers are associated with an enzyme-dependent fractionation. Most phosphatase enzymes (e.g., alkaline and acid phosphatase and phytase) have a negative fractionation factor, meaning they release phosphate with a depleted δ18O-P (Liang and Blake, 2006b; von Sperber et al., 2014, 2015).
The δ18O-P of available P in the soil can be affected by these processes through various mechanisms. Phosphate ions released by microorganisms to the soil solution potentially contribute a δ18O-P close to isotopic equilibrium, as influenced by the intracellular inorganic pyrophosphatase (Zohar et al., 2010; Poll et al., 2006). Negative offsets from isotopic equilibrium may be caused by the hydrolysis of Po compounds by extracellular or intracellular phosphatase enzymes (Helfenstein et al., 2018). Positive offsets might result from the uptake of phosphate by organisms, as they preferentially take up lighter phosphate isotopologues, thus increasing the δ18O-P of the extracellular phosphate pool (Blake et al., 2005; Lis et al., 2019).
An approach used to detect the main biological processes affecting soil P cycling consists of labeling the soil solution with 18O-enriched water (Bauke et al., 2017; Siebers et al., 2018; Siegenthaler et al., 2020). Enzyme-mediated processes inducing the P–O bond cleavage are therefore detected by tracing the incorporation of O from water into specific phosphate pools.
Tracing P fluxes with this approach is possible provided that the process generating the P flux does not induce a cleavage of the P–O bond, i.e., phosphate is transferred as intact molecule. Abiotic processes, such as sorption/desorption and precipitation/dissolution meet this condition. Additionally, phosphate ions are sorbed or precipitate with negligible isotopologues discrimination (Liang and Blake, 2006b; Jaisi et al., 2010). Through these abiotic processes, the δ18O-P of phosphate acquired via biological processes can be transferred to other inorganic P pools present in the solid phase. This was observed in a study on Andosols along a rainfall gradient, where P bound to calcium was found to carry an equilibrium isotopic signature after losing the δ18O-P of the original parent material (Helfenstein et al., 2018).
Within the German Priority Program SPP1685 “Ecosystem Nutrition: Forest Strategies for Limited Phosphorus Resources” we studied P dynamics and the biological contribution to P availability in organic horizons (Oe) of two contrasting (low- and high-P availability) and well-characterized soils from temperate forests. We chose organic horizons, as they are essential for the recycling of nutrients in forests, supplying up to 99% of plant P demand where inorganic P availability is low (Brandtberg et al., 2004; Jonard et al., 2009; Hauenstein et al., 2018).
We labeled the soil with 18O-enriched water and measured the δ18O-P from different soil P pools in the presence or absence of added leaf litter at several time points during 3 months of incubation. In parallel, we conducted an identical incubation with 33P to quantify P fluxes, as reported in Pistocchi et al. (2018). The results of the present study will be discussed also in relation to this parallel study and the analysis of microbial community composition of Mészáros et al. (2020).
Our hypothesis was that under low P availability, the prevalence of Po mineralization on P fluxes would cause a negative offset in the δ18O-P of the available P due to the negative fractionation of phosphatase enzymes. Under high P availability conditions, we expected the δ18O-P of available P pool to be affected by either (i) abiotic exchanges with mineral phases: in this case, we would observe no incorporation of oxygen from water into phosphate; or (ii) microbial P turnover: in this case, the δ18O-P of the available P would approach isotopic equilibrium. Additionally, we measured the δ18O-P in alkaline and acid-extractable P to trace abiotic exchanges of these pools with the available P. Finally, we made the hypothesis that O incorporation from labeled water into P pools is proportional to microbial activity as suggested by Melby et al. (2013).
Materials and Methods
Sites, Soil Sampling and Preparation
The soil organic horizons come from two European Beech forest sites in Germany (Fagus sylvatica L., 100–120 years old). The site Bad Brückenau (BBR) is located at about 800 m above sea level (asl) in Northern Bavaria (50°21′7.26″N, 9°55′44.53″E). The site Lüss (LUE) is located at 100 m asl in Lower Saxony (52°50′21.77″N, 10°16′2.37″E). The soils are classified as Dystric Skeletic Cambisol (Hyperhumic, Loamic; IUSS Working Group WRB, 2006) and developed on basalt bedrock, and as a Hyperdystric Folic Cambisol (Arenic, Loamic, Nechic, Protospodic), developed on Pleistocene sand, respectively. The texture of the mineral topsoil in BBR is silty clay loam, while in LUE is loamy sand. A detailed description of the two sites is reported in Lang et al. (2017).
At each site, we collected samples from the leaf litter deposited during the previous autumn and from the organic horizons, in April, 2015 at LUE and May, 2015 at BBR. Litter was collected from the soil surface, and then five to six subsamples were taken from the Oe horizon at each site and bulked into a composite sample (hereafter referred to as BBR and LUE soil, respectively).
The soil was sieved while moist to < 5 mm. The litter was dried at 35°C, manually crushed and sieved twice to collect the fraction between 20 and 5 mm. Soil and litter samples were stored at 4°C for a period of 2 weeks (BBR) to 1 month (LUE) before the experiment.
The gravimetric water content of field moist soil was determined by drying for 20 h at 105°C. The water holding capacity (WHC) of the field moist soils was determined by saturating the soils with water and then allowing gravitational water to drain by putting them in a sand bath for 4 h.
Experimental Design and Incubation Experiment
The soils were split in two and two incubations were undertaken. The first was the labeling experiment with 18O enriched water. The second was a soil respiration experiment to measure CO2 produced as an indicator of microbial activity under the same condition of the labeling experiment. The two incubations lasted 93 and 97 days, respectively.
In both cases, the experiment design had two factors: the soil (BBR and LUE) and the litter treatment, which included the absence [non-amended treatment (NL)] or presence [leaf-litter amended treatment (L)] of leaf litter amendment. All treatments were replicated three times for the labeling experiment and four times for the respiration experiment.
Before splitting the soil, a 3-week pre-incubation at approximately 40% of the maximum WHC was undertaken, during which we monitored the respiration to obtain constant soil respiration rates (Oehl et al., 2001).
For the labeling experiment, soils were weighed in polyethylene zip lock bags (equivalent of 108 g dry soil each) and randomly assigned to the treatments. The labeling solution was prepared with 98% 18O-enriched water (Sercon Limited, Crewe, United Kingdom, the final δ18O in the labeling water was = 34.30‰). Three ml of the labeling solution was added to each of the three replicate bags, spread on the top of the soil by pipetting and then mixed for 1 min by hand through agitating the bag. This process was then repeated to add a total of nine and 12 ml of labeling solution per bag, for BBR and LUE soils, respectively. The added volumes increased the BBR and LUE soils water content to approximately 50% of their WHC. Finally, litter was added to half of the bags, in the ratio of 10 mg per g of dry soil, equivalent to 4.6 mg C g–1 soil, which corresponds approximately to natural leaf litter inputs at the two sites (Lang et al., 2017). The bags were left slightly open and arranged in a completely randomized design, in plastic trays which were covered and incubated in the dark at 19°C. To reduce evaporation, which affects the δ18O of soil water, the air moisture was kept approximately constant by placing a beaker with water in each tray.
The labeling experiment was combined with sequential extractions. Concentrations of P were measured in resin-extractable P (inorganic available P, hereafter referred to as Pres) and hexanol-labile P pools (Phex) at days 0, 4, 11, 29, and 93 after labeling. Additionally, at days 0, 4, and 93 we performed a modified Hedley sequential extraction (Tiessen and Moir, 1993; Tamburini et al., 2018) to follow the fate of 18O into other P pools (see section “Soil Phosphorus Pools Concentrations”).
For the soil respiration experiment, a set of subsamples (10 g dry weight equivalent) including all soil × litter treatment combinations was prepared on day 0 adding ultrapure H2O instead of the labeling solution. Each sample was placed in an air-tight jar (1 L volume) with an alkaline trap made of 20 ml 0.2 M NaOH solution, including four blanks without soil. The jars were then incubated together with the labeled samples. The CO2 emitted by the soil was measured at weekly intervals by back titration of the trapping solution (Alef, 1995).
Analytical Methods
Soil General Characteristics and Phosphatase Activity
For a detailed description of soil general characteristics and of the corresponding analytical methods refer to Pistocchi et al. (2018).
Potential acid and alkaline phosphatase activities were determined on soil/water suspensions by microplate fluorescence assay (Marx et al., 2001; Poll et al., 2006). A 4-methylumbelliferon (MUF) substrate was added to six replicates of soil/water suspensions under either acidic (pH 6.1) or alkaline (pH 11) conditions. Fluorescence was read on a fluorescence plate reader (Biotek FLx800, Fisher Scientific GmbH, Schwerte, Germany). The analysis was done on 1 g dry weight equivalent samples taken at days 0, 4, 11, 29, and 93 and subsequently frozen until analyzed.
Soil Phosphorus Pools Concentrations
The Hedley sequential extraction scheme was upscaled and adapted for δ18O-P analysis (Tamburini et al., 2018). The initial step of the sequential extraction follows the method proposed by Kouno et al. (1995) and modified by Bünemann et al. (2004). First, two moist soil subsamples were treated in parallel: one subsample was extracted with anion exchange resins (BDH #55164, 12 cm × 4 cm, Pres), the other was fumigated with liquid hexanol and extracted with anion exchange resins (Phex). In the upscaled version, the amount of soil, resins and hexanol were adapted to obtain sufficient amount of P for isotope analyses, e.g., up to 100 g equivalent dry soil for LUE, keeping the solid to liquid ratio 1:15 (Tamburini et al., 2018). We did not include a P spiked subsample for quantifying the P recovery according to the method, as this was done in the parallel experiment with 33P tracing (Pistocchi et al., 2018). The Pres is a proxy of the available P, while the difference between Phex and Pres provides an estimate of the microbial P (Pmic).
The soil residue of the subsample extracted with hexanol was used for the subsequent steps of the sequential extraction. First, it was extracted with NaOH/EDTA, targeting the inorganic and organic P bound to Fe and Al oxides (hereafter PiNaOH and PoNaOH). After removing the resins, NaOH and EDTA disodium salt were added to the soil suspensions in solid form to reach the required concentration (0.25 M NaOH and 0.05 M EDTA). After 16 h shaking, the samples were centrifuged (5300 g for 15 min), filtered through Millipore nylon filters (0.8 μm), and the filtrates were collected for PiNaOH and PoNaOH determination and purification for isotopes analyses.
Subsequently, the soil residue was extracted with 1 M HCl, targeting sparingly soluble P bound to Ca (PiHCl), but in acidic soil likely including P bound to Fe and Al oxyhydroxides not entirely extracted during the previous step (Werner et al., 2017). A volume of 1 M HCl was added to the soil residue in the proportion of 10–1 and the extracts collected after shaking overnight and filtering using glass fiber filters (0.8 μm, Millipore).
The concentrations of Pres, Phex, PiNaOH, and PiHCl were determined colorimetrically (UV-1800, Shimadzu, Canby, United States) with the malachite green method (Ohno and Zibilske, 1991). The organic fraction, PoNaOH, was determined by difference with the total P concentration in the NaOH–EDTA extract, measured after liquid digestion with concentrated nitric acid and potassium persulfate.
Oxygen Isotopic Values
Oxygen isotopic values in soil water and phosphate
Soil water was quantitatively extracted by cryogenic vacuum extraction (Orlowski et al., 2013) for each combination of soil per treatment at days 0, 4, 11, 29, and 93 after labeling. Oxygen isotopic composition of the soil water (δ18Ow) was measured by equilibration with CO2 using a gas bench (Thermo Scientific GasBench II) connected to an isotope ratio mass spectrometer (Thermo Scientific Delta V plus) at the facilities of the Department of Earth Science of the ETH Zürich (Seth et al., 2006). Calibration was made with international standards SMOW (Standard Mean Ocean Water), SLAP (Standard Light Antarctic Precipitation), and GISP (Greenland Ice Sheet Precipitation), distributed by the International Atomic Energy Agency (IAEA, Vienna, Austria). Results are reported in the standard delta notation (δ18O) as per mill deviation to the Vienna Standard Mean Ocean Water (VSMOW). Reproducibility of the measurements based on repeated measurements of internal standards was better than 0.03‰.
The Pres, Phex, and PiHCl extracts were purified following the protocol of Tamburini et al. (2010) and modified by Pistocchi et al. (2014). In NaOH–EDTA extracts, both PoNaOH and PiNaOH are present and a separation prior the purification is needed. According to Tamburini et al. (2018), the NaOH–EDTA pool was divided into high-molecular weight (mostly organic) and low-molecular weight (mostly inorganic) compounds using size exclusion gel chromatography with a Sephadex G25 Medium, presenting a 5 KDa cut-off (ÄKTAprime plus, GE Healthcare Bio-Sciences AB, Uppsala, Sweden). The inorganic pool was purified following the standard protocol, after a precipitation of magnesium ammonium phosphate, which targets inorganic P. The organic pool was hydrolysed by UV radiation, with one split containing 18O-enriched water to check for possible incorporation of O into the newly formed inorganic phosphate.
The P concentration in NaOH–EDTA extracts from LUE was not high enough for the separation–purification procedure and therefore only the δ18O-P values of the initial PoNaOH (δ18O-PoNaOH) are presented.
Purified phosphate in the form of Ag3PO4 was weighted in silver capsules in two or three analytical replicates each consisting in 300–600 μg of Ag3PO4 and a small amount of glassy carbon powder. Samples were analyzed on a thermal conversion elemental analyzer (vario PYRO cube, Elementar Analysensysteme GmbH, Langenselbold, Germany), coupled to an IsoPrime 100 isotopic ratio mass spectrometer (IRMS) at the Laboratory of the Plant Nutrition Group (ETH Zürich). In each run, repeated measurements of internal Ag3PO4 standard (Acros Organics, Geel, Belgium, δ18O = 14.2‰), two benzoic acid international standards (IAEA 601: δ18O = 23.1‰, IAEA 602 δ18O = 71.3‰), and in-house made standards were included for instrumental drift correction and calibration. The δ18O composition is expressed in the delta notation with respect to VSMOW (Vienna Standard Mean Ocean Water). The δ18O-P of a specific P pool is referred to as: δ18O-Ppool identification. Analytical uncertainties, as determined from the replicate analysis of the standards, were of 0.4‰.
Oxygen isotopic values in leaf litter
Samples of the LUE and BBR leaf litter used in the incubation were extracted and purified according to the protocol of Tamburini et al. (2018). The protocol targets two pools extracted sequentially: the metabolic Pi with trichloroacetic acid (TCA) and the organic P with NaOH–EDTA. In detail, plant material weighed in duplicate (6 g each) was first crushed with the help of liquid N2 and then extracted with either 18O-labeled or unlabeled 0.3 M TCA. The supernatants were separated after homogenization with a Polytron (Kinematica AG, Lucerne, Switzerland) and shaking (0.5 h) via filtration with glass fiber filters (APFF04700 Merck Millipore). The residue was further extracted with 0.25 M NaOH – 0.05 M Na2(EDTA)2⋅H2O, following the protocol described in Noack et al. (2014).
The TCA and NaOH–EDTA supernatants were measured for Pi (PiTCA, PiNaOH_litter) and Po (PoTCA, PoNaOH_litter) and purified for δ18O-P analysis, following the procedure described by Pfahler et al. (2013) and Tamburini et al. (2018), respectively.
Data Analyses
Expected δ18O-P at Isotopic Equilibrium and From Organic P Hydrolysis
The expected δ18O-P of phosphate in equilibrium with soil water (δ18O-Peq) was calculated for each time point rearranging the equation from Chang and Blake (2015) as follows:
where T is the temperature in K during the incubation and δ18Ow (‰) is the measured oxygen isotopic composition of soil water.
The expected δ18O-P of phosphate released from hydrolysis of phosphoesters (δ18O-PPase) via phosphatase enzymes was calculated according to this equation:
where x is the proportion of O atoms inherited from the phosphate group in the Po compound (0.75 for phosphomonoester and 0.5 for phosphodiester), δ18O-Po (‰) is the O isotopic composition of the phosphate group in the Po compound, δ18Ow (‰) is the measured O isotopic composition of soil water, and ε (‰) is the enzyme-specific fractionation factor. Here we estimated δ18O-Po as the measured isotopic composition of Po extracted by NaOH–EDTA (δ18O-PoNaOH) either from soil or leaf litter (see Table 1 and sections “Oxygen isotopic values in soil water and phosphate” and “Oxygen isotopic values in leaf litter”).
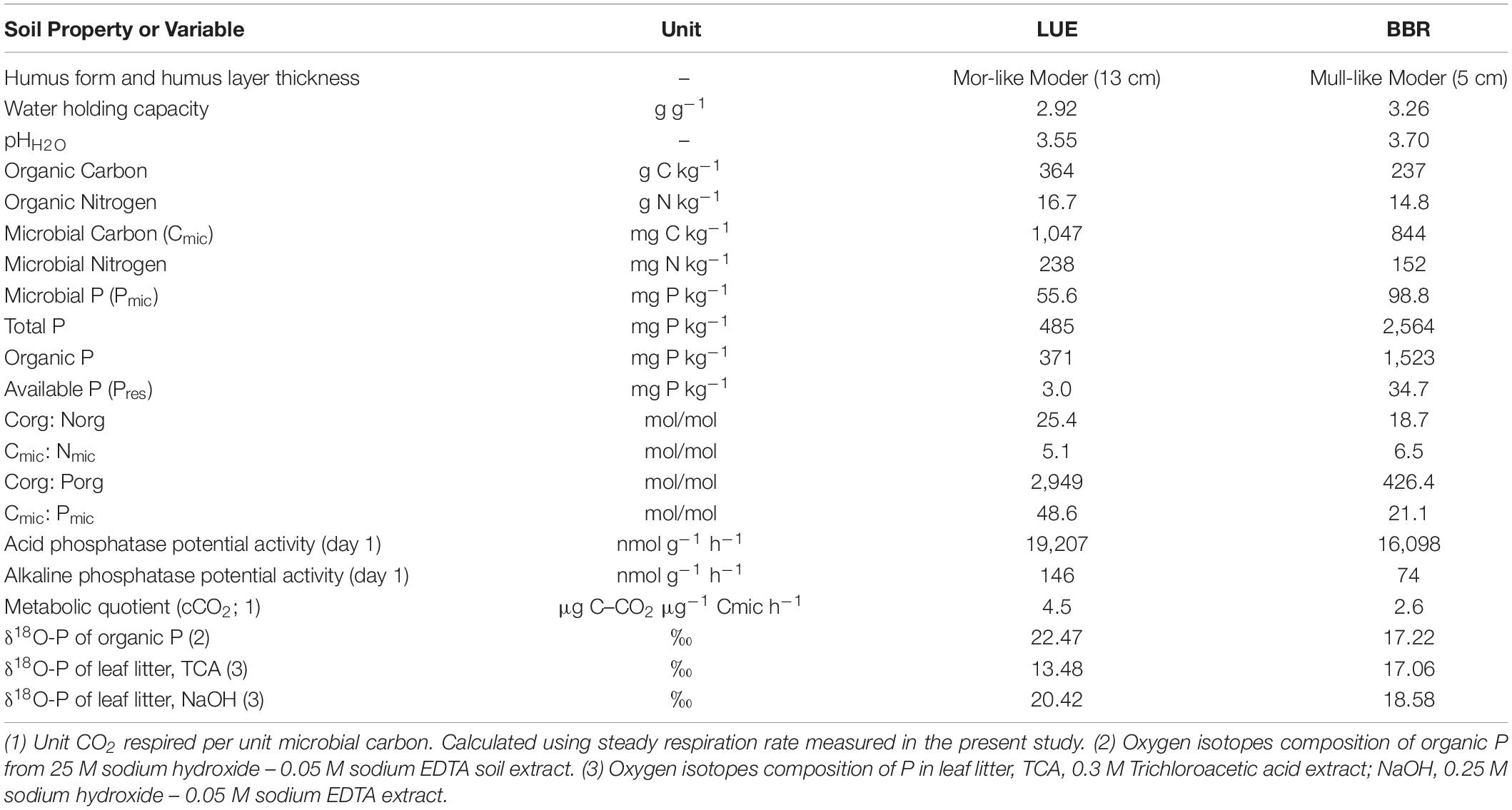
Table 1. General characteristics of the two studied Oe horizons at site Lüss (LUE) and Bad Brückenau (BBR).
Calculation of δ18O-P
The oxygen isotopic composition of phosphate in the Pmic pool (δ18O-Pmic), was calculated with a mass balance equation (Tamburini et al., 2012):
where δ18O-Phex (‰) and δ18O-Pres (‰) are the oxygen isotopic composition of phosphate in the hexanol and resin extracts, respectively, and Phex (mg P kg–1) and Pres (mg P kg–1) are the P concentrations in the corresponding extracts. When δ18O-Phex and δ18O-Pres were close, i.e., a difference of less than twice the standard deviation of analytical replicates (< 0.8‰), the δ18O-Phex was taken directly as δ18O-Pmic. We did not apply any conversion factor (Kp) to correct for incomplete recovery of Pmic due to possible ineffectiveness of the fumigant. Indeed, we cannot assume that all Pmic compartments, including the ones non-extractable with hexanol, have the same isotopic composition.
Some extractions were done in duplicate with 18O labeled and unlabeled reagents, i.e., TCA, UV digestion of the organic pool of NaOH–EDTA and HCl, to track the incorporation of labeled oxygen into the δ18O-P via inorganic hydrolysis (Tamburini et al., 2010). If incorporation was detected, the actual δ18O-P was calculated according to Pistocchi et al. (2017):
where δ18O-Pl (‰) and δ18O-Pnl (‰) are the oxygen isotopic composition of phosphate for the labeled and unlabeled samples, respectively, and accordingly δ18Owl (‰) and δ18Ownl (‰) the oxygen isotopic composition of labeled and unlabeled extraction solution. When hydrolysis was negligible, as in most cases, the subsamples were considered as duplicates.
Incorporation of O From Water Into Phosphate
Through the process of labeling with 18O-enriched water, it is possible to calculate how much O from soil water was incorporated into phosphate molecules of a specific P pool over time. Oxygen incorporation (%) was calculated as the slope of the straight line between two points in the δ18Ow-δ18O-P plot (Liang and Blake, 2006a):
Where δ18Owt and δ18Owt0 are the oxygen isotopic compositions of soil water and δ18O-Pt and δ18O-Pt0 are the oxygen isotopic compositions of phosphate in a given pool at time point t and at day 0, respectively.
Incorporations of 25 or 50% indicate that overall one out of four or two out of four O atoms, respectively, are incorporated from water into phosphate. 100% O incorporation indicates all four O of phosphate derived from water.
For δ18O-P values obtained with the Equations 3, 4, and 5 the mean and standard deviation per sample were obtained with a Monte Carlo error propagation simulation (Anderson, 1976). Calculations were repeated 10 million times by varying the δ18O signatures according to their mean and standard deviations. For the δ18Ows, we assumed a standard deviation of 0.03‰ corresponding to the analytical error.
Statistical Analyses
A two-way ANOVA (1st factor = litter application, 2nd factor = date) was applied to analyze the variables measured during the incubation for each soil separately except the respiration rates. These latter were analyzed with a mixed model, where the litter amendment was the fixed factor and the time of the measurement (weekly) a random factor with the replicate nested in it. Model simplification (one-way ANOVA) was done when possible or when data were missing, e.g., δ18O-PoNaOH in LUE soil. In some cases, there were not enough replicates for statistical tests. These results are discussed qualitatively. The Tukey test was used for post hoc comparison. The Student’s paired t-test was used when comparing single dates and cumulative values, after checking for homogeneity of variances. The Shapiro–Wilk test was used to assess normality of the data. All analyses were performed in R 3.1.1 (R version 3.1.1, R Core Team).
Results
Soil Respiration
The soil respiration declined during the first 3–4 weeks by approximately 10 and 25% for LUE and BBR soils, respectively. After the first month, the respiration remained approximately constant (Figure 1). The soil respiration of LUE soil was almost double that of the one measured for BBR soil. Similarly, the metabolic quotient cCO2, calculated with the average respiration rates over the last 8 weeks, was almost double in LUE soil (Table 1).
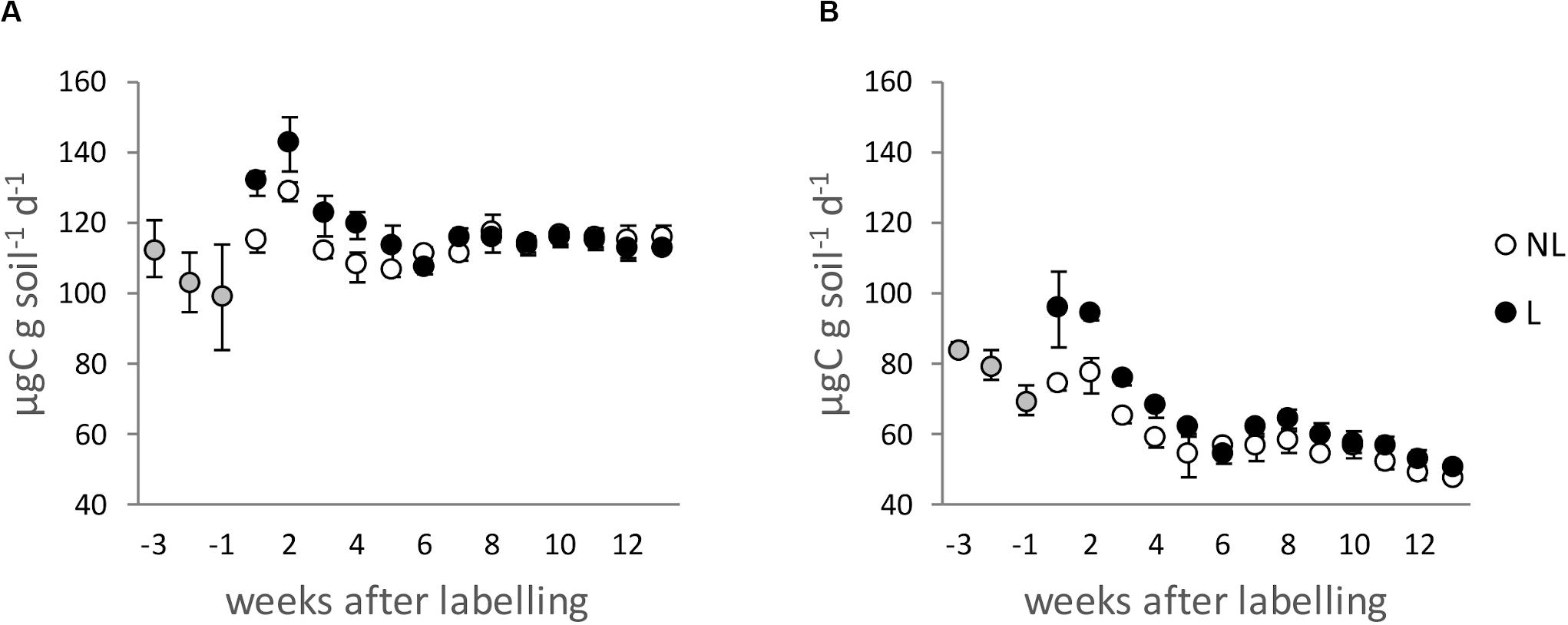
Figure 1. CO2 emitted from Lüss (LUE; A) and Bad Brückenau (BBR; B) soils during the incubation. Gray circles indicate respiration rates during the pre-incubation period, white circles the respiration rates in the non-amended treatment (NL) and black circles in the leaf-litter amended treatment (L). Error bars indicate replicates standard deviation.
During the first 4 weeks, differences between treatments were detectable, with leaf litter-amended soils showing higher respiration rates (Figure 1). This resulted in a cumulative additional carbon (C) release for the litter-amended soils of 3.4 and 11.1% for LUE and BBR soils, respectively, significant only for the latter (Supplementary Table 1 SI).
Soil General Characteristics and Potential Phosphatases Activity
The two soils were acidic (3.77 and 3.65 for BBR and LUE soil, respectively) but differed in almost all considered variables (Table 1). In particular, concentration of total, organic and available P was much higher in BBR than in LUE soil.
Potential acid phosphatase activity was similar for LUE and BBR soils, while potential alkaline phosphatase activity was double in LUE soil, although much lower than the acid phosphatase activity (Table 1 and Supplementary Figure 1SI). Therefore, for the calculation of the expected value from Po hydrolysis by phosphatases with Equation 2 we used the fractionation factor (ε) of – 10‰ attributed to acid phosphatases (von Sperber et al., 2014). No differences were found between litter treatments.
The Concentration and δ18O-P of Resin-Extractable and Microbial P Pools
In both LUE and BBR soils the concentration of Pres increased, while Pmic remained largely stable over the study time with small fluctuation in the low P LUE soil and decreased significantly in the high P BBR soil (Supplementary Table 2 SI). In the litter-amended BBR soil the Pres was slightly higher, with a mean effect size of + 2.1 mg kg–1. The litter addition increased the Pmic slightly in both soils, with a mean effect size of + 4.9 and + 4.4 mg kg–1 for LUE and BBR, respectively (Supplementary Table 2 SI).
The δ18O-Pres and δ18O-Pmic increased by several units during the incubation in both LUE and BBR soils (Figure 2). Therefore, incorporation of O from labeled soil water into phosphate occurred.
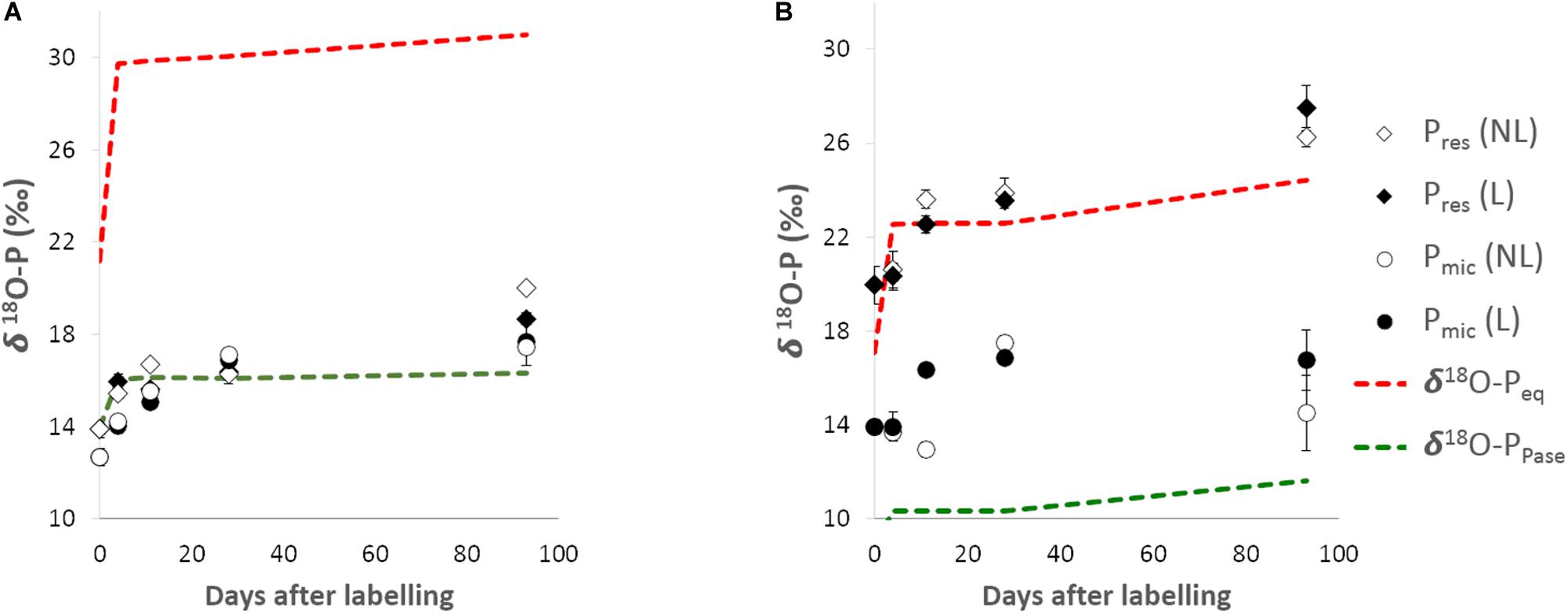
Figure 2. The δ18O-P of available P (Pres) and microbial P (Pmic) pools in Lüss (LUE; A) and Bad Brückenau (BBR; B) soils over the course of the incubation. The expected isotopic equilibrium between phosphate and water (Equation 1) is indicated by the red dotted line and the expected isotopic signature of phosphate from organic P (Po) hydrolysis of soil organic matter via acid phosphatase (Equation 2) is indicated by the green dotted line. Error bars indicate replicates standard deviation. NL, non-amended; L, leaf litter-amended.
For the low P LUE soil, both δ18O-Pres and δ18O-Pmic had a similar initial value (13.9 and 12.7‰, respectively) and both followed a slight increasing trend over the duration irrespective of the application of leaf litter. This change in value was similar to that predicted by the δ18O-PPase, i.e., expected from the hydrolysis of Po via acid phosphatases (Equation 2; Figure 2A).
For the high P BBR soil, however, the initial values of δ18O-Pres and δ18O-Pmic were different: 19.9‰ vs. 14.0‰, respectively. The δ18O-Pmic increased slightly over time and remained below the expected equilibrium between phosphate and water (δ18O-Peq in Equation 1), whereas the δ18O-Pres approached and exceeded the δ18O-Peq by day 93 (Figure 2B). The δ18O-Pmic values were in average lower for the non-amended treatment (p < 0.05).
For both soils, the picture does not change if we consider the δ18O-PPase values calculated using the Po in the litter, as they are similar to the ones from soil Po (see δ18O-PPase litter, Supplementary Table 2 SI).
The Concentration and δ18O-P of NaOH- and HCl-Extractable Pools
In the LUE soil incubation, concentrations of the inorganic pools PiNaOH and PiHCl remained approximately stable during the incubation. The organic pool, PoNaOH, increased slightly (+ 36.8 mg kg–1 at day 93). No differences were detected between the litter amended and non-amended treatments (Table 3).
In BBR, a slight increase (mean effect size of + 49.0 mg kg–1 at day 93) of the PiNaOH was registered over the incubation time. The PiHCl and PoNaOH remained stable over the incubation time. No differences due to the leaf litter addition were observed (Table 3).
The δ18O-PiHCl in the LUE soil incubation had a slight increase (+ 1‰). We could not analyze the δ18O-PiNaOH or δ18O-PoNaOH in LUE soil because of the insufficient amount of P extracted. In the BBR soil, we detected a significant increase of the δ18O-P of both inorganic and organic pools. The δ18O-PiNaOH increased by 4.4‰, as an average of the L and NL treatments. A similar increase was measured for the δ18O-PiHCl (Table 3). Despite those increases neither δ18O-PiNaOH nor δ18O-PiHCl reached the corresponding value of the available P (Pres) at day 93, meaning that they did not completely equilibrate with the Pres at the end of the incubation. Similarly, the δ18O-PoNaOH also increased slightly, but by only approximately 1‰ at the end of the incubation.
Differences due to the litter addition were found in the BBR soil for the δ18O-PoNaOH (+ 1‰ for the L treatment at day 4) and for the δ18O-PiHCl (−2.3‰ for the L treatment at day 93).
18O Incorporation Into P Pools
The addition of 18O-enriched water successfully increased O isotopic ratio of soil water (Supplementary Table 3 SI) and resulted in incorporation of 18O from water into major soil P pools. The trend, however, differed in the two soils. In LUE, both the Pres and Pmic incorporated O at similar rate: around one out of four O atoms per phosphate (25%) were exchanged in the first 20–30 days of incubation and up to two out of four (50%) were exchanged after 93 days (Figure 3A). In BBR instead, the 18O from water was incorporated faster, but it did not exceed 50% in the Pmic by day 93, while it had reached 90% in the Pres, suggesting an almost complete exchange of the four O of phosphate with water (Figure 3B). A decline of the O incorporation in Pmic in the BBR soil was observed between the second-last and the last sampling. This decline was more pronounced in the treatment without litter. A stronger relationship was found between O incorporation in Pres and cumulated respiration in BBR soil as compared to LUE (Figure 4A).
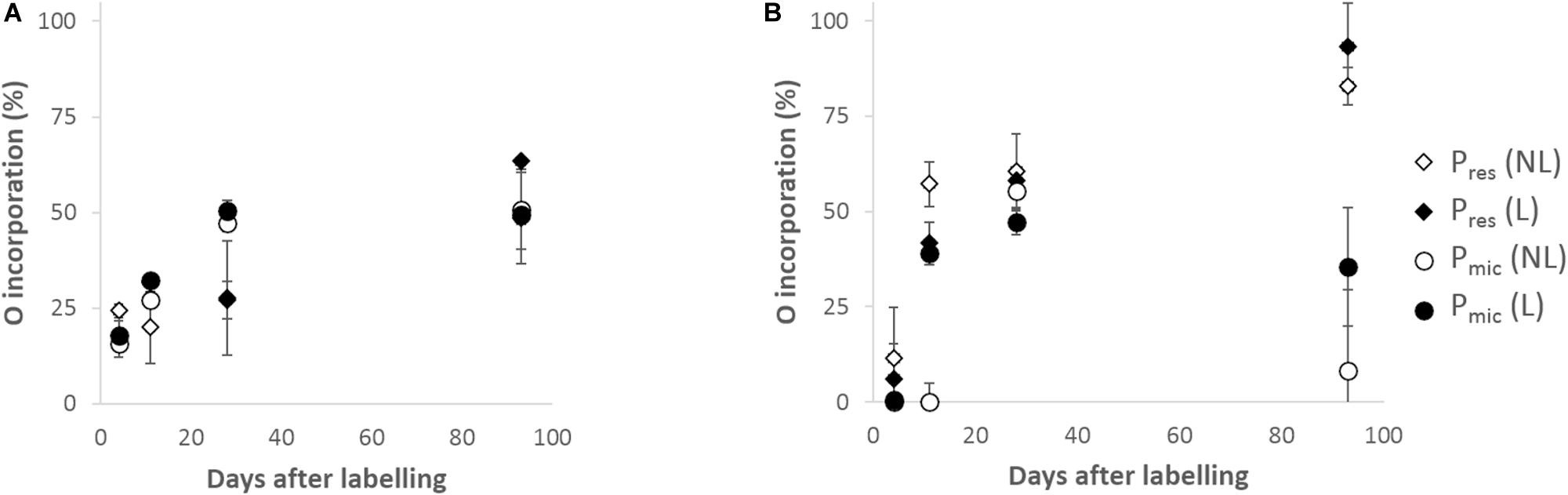
Figure 3. Oxygen incorporation from water into available P (Pres) and microbial P (Pmic) pools according to Equation 5 in Lüss (LUE; A) and Bad Brückenau (BBR; B) soils during the incubation. Error bars indicate standard deviation from Monte Carlo error propagation. NL, non-amended; L, leaf litter-amended.
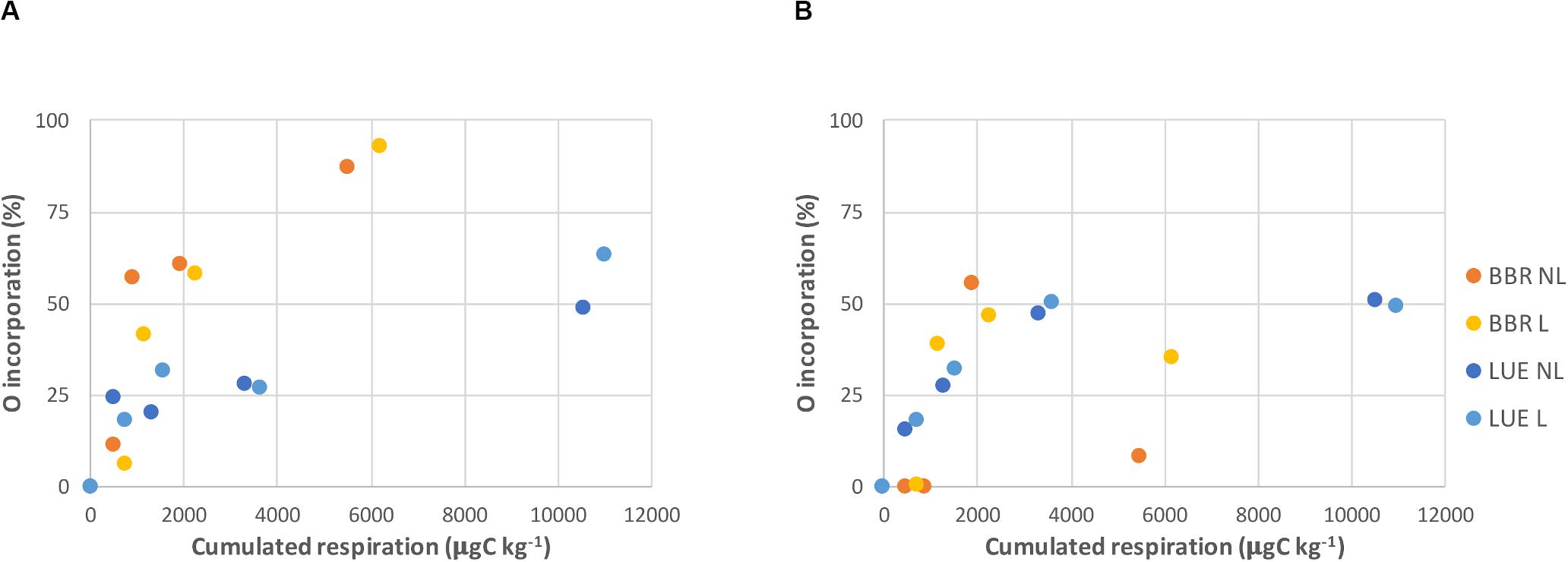
Figure 4. Oxygen incorporation from labeled water into the available (Pres; A) and microbial P (Pmic; B) in Bad Brückenau (BBR) and Lüss (LUE) soils against cumulated respiration. NL, non-amended; L, leaf litter-amended.
Discussion
The increase in δ18O-P value of almost all P pools indicates that biological processes were involved in P cycling in both soils, as the exchange of oxygen between soil phosphate and water involves enzymatic reactions (Tudge, 1960).
Biological Processes Affecting Available P in the Low P Availability LUE Soil
Under low P availability, δ18O-Pmic and δ18O-Pres were very similar and followed the same temporal trend (Figure 2A). There are three potential explanations for this pattern: (i) There was phosphate efflux from Pmic to Pres, so the Pres pool was strongly influenced by the Pmic pool as observed in other P-poor soils (Tamburini et al., 2012; Weiner et al., 2018); (ii) There was no phosphate efflux from Pmic to Pres, but the dominant enzymatic processes were the same in both pools, thus imprinting the same isotopic signature; finally (iii) There was no phosphate efflux from Pmic to Pres, and the two pools were impacted by different enzymatic processes which resulted in similar δ18O-P just by chance.
The parallel 33P dilution experiment showed that gross mineralization strongly contributed to the Pres pool. It also highlighted a fast and important P immobilization into the microbial biomass (around 95% of gross mineralization, see Pistocchi et al., 2018). The 33P experiment, however, gave no direct evidence of an efflux from Pmic to Pres. This may point either to the first or to the second explanation. Lis et al. (2019) observed in an in vitro experiment that cyanobacteria growing under P-depleted conditions took up P through phosphate specific transporters (Pst) without leaking it. Therefore, the second explanation, i.e., no or little phosphate efflux from Pmic to Pres and same dominant enzymatic processes, is more likely (see Figure 5A modified after Lis et al., 2019). However, compared to their study we did not observe isotopic equilibrium in the intracellular and extracellular P.
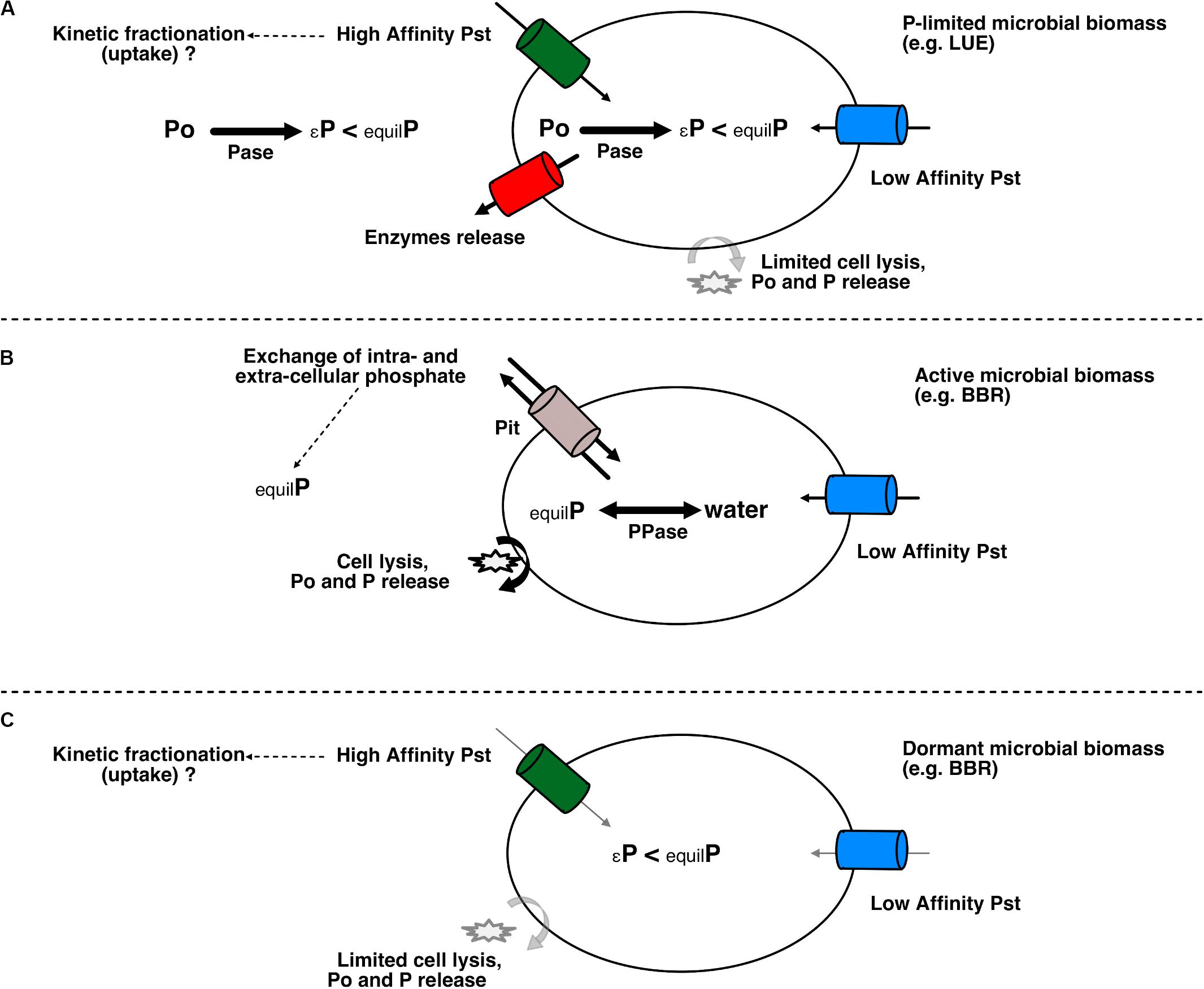
Figure 5. Schematic representation of microbial processes under active stressed or dormant status and their effect on δ18O-Pres and δ18O-Pmic adapted with permission from Lis et al. (2019). Copyright (2019) American Chemical Society. (A) Case of microbes under low P conditions [e.g., Lüss (LUE) soil]. Intracellular P is not at isotopic equilibrium, since inorganic pyrophosphatase (PPase) is not dominant and other enzymes, e.g., phosphomonoesterase, are controlling the δ18O-Pmic. Only influx of phosphate ions is observed through phosphate specific transporters (high and low affinity Pst) and cell lysis might be limited. Phosphatase enzymes are actively excreted by the cells to hydrolyse Po compounds. These enzymatic reactions imprint an isotopic kinetic effect on the δ18O-Pres. (B) Case of microbes not limited by any environmental factor [e.g., active microbes in Bad Brückenau (BBR) soils]. Oxygen in intracellular phosphate is equilibrated with oxygen in water via the action of PPase. Intracellular phosphate is leaked out to the soil environment by either the low affinity Pit transporter or cell lysis (see Lis et al., 2019). δ18O-Pres is controlled by the intracellular P and exhibits an equilibrium signature. (C) Case of microbes in a dormant state (limited by factors other than P availability, such as in BBR soils): uptake from the soil solution is limited and intracellular processes (e.g., phosphatases catalyzed reactions or other processes guaranteeing the minimal functioning of the cell; see Blagodatskaya and Kuzyakov (2013)] are imprinting an isotopic kinetic effect on δ18O-Pmic. This portion of the microbial biomass have little impact on the Pres. EquilP, phosphate with oxygen at equilibrium with oxygen in water; εP, phosphate released from hydrolysis of Po with a kinetic isotope imprint.
Of further consideration is that both δ18O-Pmic and δ18O-Pres were similar to the value expected for phosphomonoester hydrolysis over the first 30 days of incubation (one out of four O was exchanged with water, see Figure 2A). According to a two end-members mass balance including the isotopic equilibrium (δ18O-Peq, Equation 1) and the δ18O-PPase (Equation 2), this contribution represented almost 100% of the Pres over this period.
By the end of the incubation, the O incorporation into Pres had attained 50% (two out of four O atoms exchanged with water; Figure 3A), suggesting the contribution of other processes. There are two possible explanations: (i) that a single enzymatic process which incorporates two O atoms from water into phosphate was prevailing; or (ii) two or more processes contributed, resulting in 50% average O incorporation. The first explanation would be that the extracellular hydrolysis of phosphodiesters was occurring. However, Lang et al. (2017) found that the potential activity of phosphodiesterases over phosphomonoesterases was very low at this site. The contribution of multiple processes is, therefore, more likely. A two end-members balance including δ18O-Peq and δ18O-PPase as previously hypothesized, suggests that phosphate derived from monoester hydrolysis constitutes about 80% of the Pres at the end of the incubation. Interestingly, this value agrees with the results of parallel 33P incubation, where 94% of P exchanged with the soil solution came from gross mineralization (Pistocchi et al., 2018).
We conclude that under low P availability, both the Pres and the Pmic pools were dominated by phosphomonoesterase catalyzed reactions.
The attainment of the isotopic equilibrium was until now considered the main biological effect influencing the available P, corresponding to Pres as defined in this study, via the release of intracellular phosphate ions (Zohar et al., 2010; Tamburini et al., 2012; Stout et al., 2014; Weiner et al., 2018; Lis et al., 2019). Our results support the hypothesis that under P-limiting conditions, the isotopic effect of Po hydrolysis can outweigh the isotopic equilibrium effect driven by inorganic pyrophosphatase in both Pmic and Pres pools (Figure 5A).
Biological Processes Affecting Available P in the High P Availability BBR Soil
Under high P availability, the Pres pool carried a very distinct isotopic signature from the Pmic pool (Figure 2B). The δ18O-Pres was close to or higher than the δ18O-Peq (isotopic equilibrium between phosphate and water, Equation 1), while δ18O-Pmic was in between δ18O-Peq and the δ18O-PPase (Equation 2).
The contribution of P from leaf litter does not explain the δ18O-Pres values, as we did not find significant differences between litter treatments. The exchange with other soil P pools via sorption/desorption or precipitation/dissolution does not explain them either, as the PiNaOH and PiHCl all had lower δ18O-P than δ18O-Pres (see Table 2), although these P pools likely exchanged phosphate ions with the Pres pool (see section “Exchanges Between Available P and Other Inorganic and Organic P Pools”).
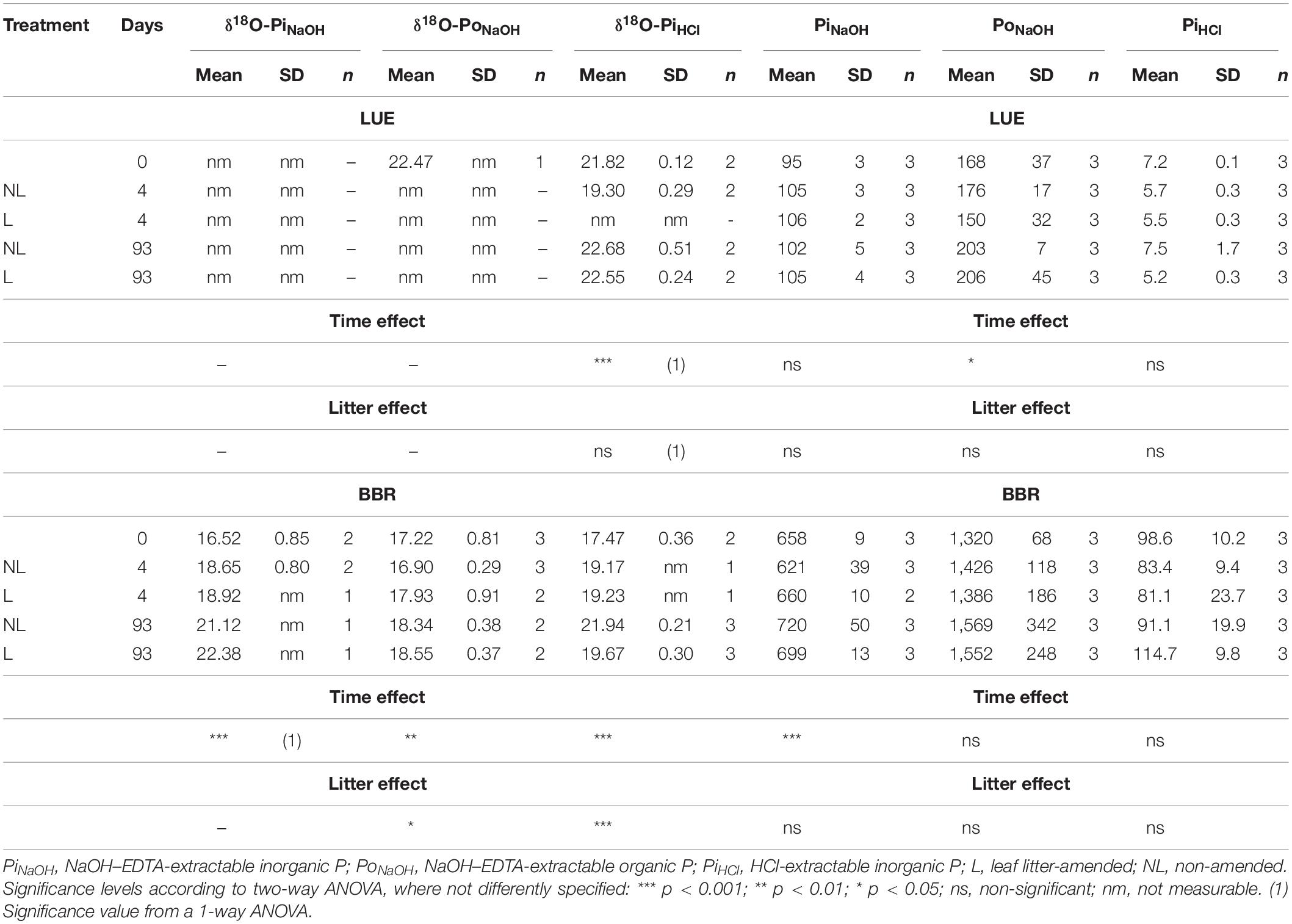
Table 2. Concentration and δ18O-P values of inorganic and organic P pools over the incubation period.
The incorporation of O atoms from labeled water was around 90% in the Pres pool by day 93, suggesting the prevalence of a process that leads to an exchange of all four O atoms. The only known enzyme which could cause a complete exchange of O atoms with water is the inorganic pyrophosphatase (Cohn, 1958), which is mostly intracellular (Poll et al., 2006). However, the Pmic pool in the BBR soil, showed consistently lower O incorporation when compared to the Pres. The presence of different microorganisms in either active or dormant states, all extracted with hexanol but carrying different isotopic signatures, could possibly explain the difference in δ18O-P between Pres and Pmic pools. However, only the active microbial community would carry a signature close to δ18O-Peq and contribute to the Pres via P uptake, intracellular turnover and release (Siegenthaler et al., 2020). Indeed, inorganic pyrophosphatase is involved in DNA synthesis, amongst other processes, and therefore is more active when cells are growing (Kottur and Nair, 2018). The microorganisms in a dormant state would interact poorly with the Pres pool and incorporate less O from water (see section “δ18O-P and Microbial Activity” for more discussion).
The O incorporation of close to 90% (Figure 2B) implies that almost all the phosphate in the final Pres pool (i.e., 45.8 and 49.8 mg kg–1, for the NL and L treatment, respectively) underwent intracellular P turnover catalyzed by inorganic pyrophosphatases over the investigated period. Part of this efflux from Pmic corresponded to a net loss, more pronounced in the NL treatment, which in turn translated in a net increase of Pres (Supplementary Table 2 SI) and a transfer to other Pi or Po pools (see section “Exchanges Between Available P and Other Inorganic and Organic P Pools”).
At the end of the incubation, the δ18O-Pres was higher than the expected equilibrium by + 3.0‰. Recent studies on temperate forest soils reported enriched isotopic ratios compared to expected equilibrium after a period of incubation (Gross and Angert, 2017; Weiner et al., 2018). They attributed this positive offset to the steady-state between the efflux of intracellular P at isotopic equilibrium and the P uptake by microbes inducing isotopic enrichment of the extracellular phosphate ions (Blake et al., 2005; Lis et al., 2019). Although this effect could explain our finding, this explanation appears unlikely. Firstly, in our system Pres increased during the incubation period, which does not fit into a Rayleigh fractionation model, which describes the kinetic fractionation induced by P uptake (Blake et al., 2005). Secondly, the mentioned studies observed the effect of P uptake by cells mostly under low environmental P conditions, which is not the case in the BBR soil.
It can also not be ruled out that a possible contribution from an unknown extracellular process occurred, leading to the exchange of four O atoms between phosphate and water, and/or the partial contribution of mineralization of Po compounds with heavy δ18O-P.
δ18O-P and Microbial Activity
Saaby Johansen et al. (1991) and Melby et al. (2013) showed a positive relationship between δ18O-P de-labeling and respiration in incubation experiments with addition of 18O-labeled phosphate. Siegenthaler et al. (2020) suggested that a high incorporation of O from labeled water into the Pmic pool reflects a high microbial activity.
In this study, we observed a higher respiration rate, therefore a higher microbial activity, in the low P LUE soil when compared to the high P BBR soil. Similarly, the microbial respiration per unit microbial biomass (metabolic quotient, cCO2) of the LUE soil was twice the value recorded for the BBR soil (Table 1). In contrast, the O incorporation into the Pres per unit CO2 respired was lower in LUE soil (Figure 4A).
The dominance of phosphomonoesterase catalyzed reactions in the Pres pool of the LUE soil (see section “Biological Processes Affecting Available P in the Low P Availability LUE Soil”) explains the lower O incorporation. This together with the higher cCO2 might indicate differences in the microbial communities composition and/or a stronger investment of the LUE microbial community into Po mining via the production of extracellular phosphatase enzymes (Manzoni et al., 2010; Spohn and Chodak, 2015). The community composition analyses of Mészáros et al. (2020) and the δ18O-P data of the present study suggest that both factors possibly contribute simultaneously.
The O incorporation into the Pmic pool was similar in the two soils during the first month of incubation, then lower in the BBR soil, which showed a strong decrease in the non-amended treatment (Figures 3B, 4B).
We assumed earlier (section “Biological Processes Affecting Available P in the High P Availability BBR Soil”) that in the BBR soil only part of the microbial community was actively turning over P and imprinting an isotopic equilibrium signature on it. The below-equilibrium δ18O-Pmic and corresponding O incorporation resulted, therefore, from the relative contribution of δ18O-Pmic at the isotopic equilibrium and δ18O-Pmic below isotopic equilibrium. Lower δ18O-Pmic would either occur because of the dominance of intracellular phosphomonoesterase reactions or because of little exchange of O between intracellular phosphate and labeled water (Chen et al., 2019). Since the δ18O-Pmic was lower than isotopic equilibrium already before the labeling, the first explanation seems more likely. The two typologies outlined in Figures 5B,C would, therefore, contribute to the δ18O-Pmic in the BBR soil.
The decrease in the O incorporation in the δ18O-Pmic of the non-amended BBR soil by the end of the incubation was concomitant with a strong decrease in Pmic concentration (Supplementary Table 2 SI). These effects could be determined by a decrease in the activity of microbial cells or a shift in the microbial community composition in response to less favorable environmental conditions (Fanin et al., 2013; Mooshammer et al., 2014). The respiration rates were very similar between treatments over the last 2 months of incubation (Figure 1). However, the analysis of microbial community composition showed a shift in both the fungal and bacterial communities. Additionally, the bacterial community changed differently in the amended and non-amended treatments over the last incubation period (Mészáros et al., 2020). The second explanation seems, therefore, more likely.
In LUE the incorporation of O from labeled water into Pmic was driven by phosphomonoesterase catalyzed reactions as for Pres (see section “Biological Processes Affecting Available P in the Low P Availability LUE Soil”). Pfahler et al. (2013) reported δ18O-P of soybean leaves decreased under P-limiting conditions and suggested this was the result of increased recycling of Po within plant tissue. Therefore, P-limiting conditions might induce a tighter intracellular Po recycling and translate in below-equilibrium δ18O-Pmic values (Figure 5A). Interestingly, we found below-equilibrium δ18O-Pmic also in non-incubated samples taken at the site LUE at two different dates (unpublished results), which suggests this effect is not an artifact of the incubation.
In summary, despite similar below-equilibrium δ18O-Pmic in LUE and BBR soils, data such as δ18O-Pres, respiration rates and microbial communities composition, suggest a tighter P cycling in LUE. Interestingly, such differences were not reflected in different potential acid phosphatases activities (Table 1 and Supplementary Figure 1 in SI). Our data also suggest that the relationship between microbial activity and O incorporation into phosphate is not simply proportional as we initially hypothesized but likely modulated by P availability.
Exchanges Between Available P and Other Inorganic and Organic P Pools
Due to the low extractable P concentration in LUE, we can only discuss results from the BBR soil.
In the parallel 33P experiment the Pres and the PiNaOH were shown to exchange rapidly in the BBR soil, with the tracer found in the PiNaOH pool after 4 days. This suggests a fast exchange process, such as sorption/desorption. However, 3-months of incubation were not sufficient to attain a complete exchange between these two pools (Pistocchi et al., 2018).
As these exchanges are abiotic, i.e., without P–O bond cleavage, it is possible to trace P fluxes also with the δ18O-P, provided that the two exchanging pools have different initial isotopic signatures.
The increase of the δ18O-PiNaOH suggests that phosphate ions were transferred from an enriched P pool, presumably Pres. As was found with the 33P, the PiNaOH and the Pres did not attain similar isotopic compositions by the end of the incubation. On the contrary, their isotopic signature diverged, due to a large increase of the δ18O-Pres (see Table 3 and Supplementary Table 2 SI). It would appear that over the time scale of this incubation study, the biological processes that led to the isotopic enrichment of the Pres had a stronger effect than the ions exchange with the PiNaOH pool.
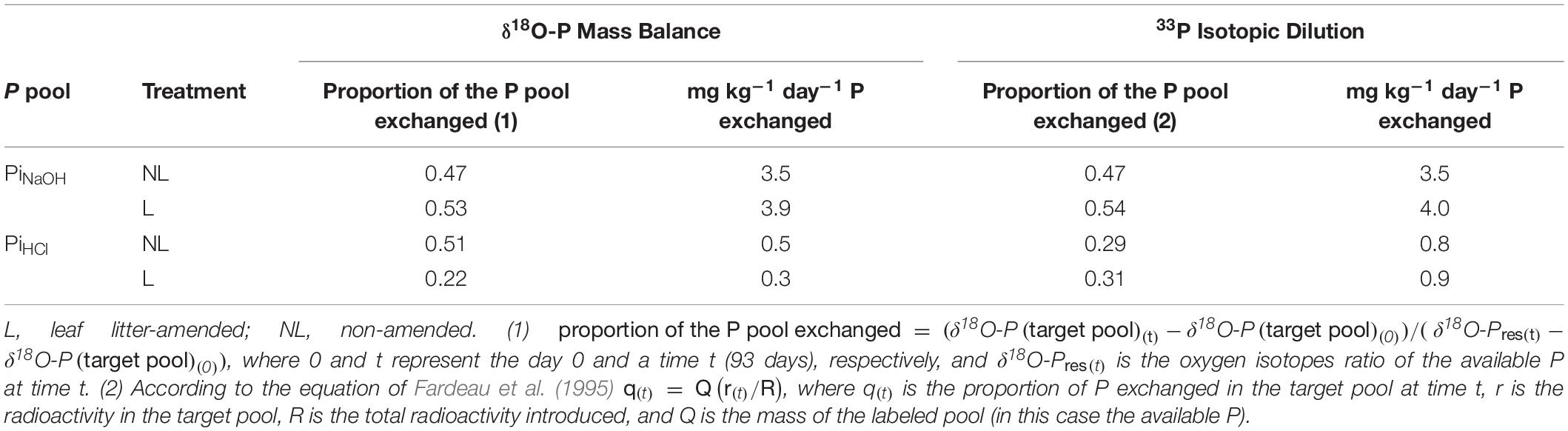
Table 3. Estimation of P exchange fluxes in the Bad Brückenau (BBR) soil between the available and other inorganic P pools over 93 days according to a mass balance (δ18O-P data from this study) or to an isotopic dilution approach (33P data from Pistocchi et al., 2018).
A mass balance, assuming constant P concentrations over the incubation, yields an exchange flux between Pres and PiNaOH of approximately 3.5–3.9 mg kg–1 day–1, which is similar to estimates provided by the 33P tracing experiment (Table 3).
The δ18O-PiHCl also increased over time, suggesting an exchange with an isotopically enriched P pool. Similarly, we assume that the PiHCl exchanged phosphate ions with the Pres pool via abiotic reactions, allowing us to trace P fluxes via the δ18O-P. Through a mass balance calculation, we estimated 0.3–0.5 mg kg–1 day–1 of P was exchanged on average between these two P pools (Table 3).
The addition of leaf litter resulted in a slightly lower δ18O-PiHCl at the end of the incubation (Table 2). To explain this, we have to assume that an abiotic transfer of depleted phosphate from the litter to the PiHCl has occurred. Although the inorganic P in the litter had a slightly depleted δ18O-P (17.06‰ for the TCA extract, see Table 1), the amount of inorganic P contained within the litter was small compared to the PiHCl pool. Furthermore, no other differences in other inorganic P pools of the leaf litter-amended soils were observed. Therefore, we cannot exclude the lower δ18O-PHCl was not simply a processing error.
In summary, the Pres exchanged mostly with the PiNaOH pool, which is in agreement with previous studies on BBR (Lang et al., 2017; Pistocchi et al., 2018) or on low-pH and Fe/Al oxides-rich soils (Buehler et al., 2002; Helfenstein et al., 2020).
The δ18O-PoNaOH increased slightly in the BBR soil (+ 1.1–1.3‰ in the NL and L treatment, respectively). The effect of leaf litter on the δ18O-PoNaOH was minor, as a difference between the treatments was only observed at day 4, and this subsequently leveled out. The increase in microbial activity observed in the first few weeks after the addition of the litter could have determined a faster turnover of Po derived from microbes. If the microbial Po was isotopically enriched as a consequence of the incorporation of O from labeled water, it would enrich the δ18O-PoNaOH once released from cells. Unfortunately, the δ18O-P of microbial Po is unknown, which prevents the calculation of its contribution to the PoNaOH pool. Measuring the isotopic signature of microbial Po or single microbial metabolites constitutes a major research gap that needs to be investigated in order to better clarify Po dynamics (Tamburini et al., 2018).
Conclusion
Until now the isotopic equilibrium between phosphate and water was believed to be the main biological effect on microbial and available P. Here, we show that a below-equilibrium signature can be still an indicator of control of microbial P on the available P via Po hydrolysis reactions. This effect is possibly induced by P limiting conditions for microbes.
Labeling with 18O-water allowed the identification of the major biological process contributing to available P where radioisotope tracers fail because of low sensitivity, i.e., high baseline of isotopic dilution, as in the case of P-rich soils. Two independent isotopic approaches (33P and δ18O-P) provided very similar estimates of P exchanged between the Pres and PiNaOH pools. This suggests that δ18O-P can be successfully used to trace P fluxes, provided that the underlying processes do not break the P – O bonds of the phosphate molecule.
Data Availability Statement
The dataset on fungal and bacterial community composition discussed in this study can be found in the Portail Data INRAE V1 repository (https://doi.org/10.15454/XOFCHY).
Author Contributions
CP wrote the manuscript with the inputs from all the co-authors. CP and ÉM conducted the experiments and performed the analyses. EF, FT, EB, and CP contributed to the experiment design. All authors contributed to the article and approved the submitted version.
Funding
This work was funded by the Swiss National Science Foundation (SNF project 200021E-149130). The ETH Library funded the publication fee.
Conflict of Interest
The authors declare that the research was conducted in the absence of any commercial or financial relationships that could be construed as a potential conflict of interest.
Acknowledgments
We want to acknowledge Friedericke Lang and Jaane Krueger for the project coordination, their support in sampling organization, and the many information they provided. We are also very grateful to the two reviewers for providing valuable and detailed comments to the manuscript. We also want to thank Laurie Schönholzer for her support in the laboratory analysis.
Supplementary Material
The Supplementary Material for this article can be found online at: https://www.frontiersin.org/articles/10.3389/fenvs.2020.564778/full#supplementary-material
References
Achat, D. L., Bakker, M. R., Augusto, L., Saur, E., Dousseron, L., Morel, C., et al. (2009). Evaluation of the phosphorus status of P-deficient podzols in temperate pine stands: combining isotopic dilution and extraction methods. Biogeochemistry 92, 183–200. doi: 10.1007/s10533-008-9283-7
Alef, K. (1995). “Soil respiration,” in Methods in Soil Microbiology and Biochemistry, eds K. Alef and P. Nannipieri (San Diego, CA: Alef K. and Nannipieri P).
Anderson, G. M. (1976). Error propagation by the Monte Carlo method in geochemical calculations. Geochim. Cosmochim. Acta 40, 1533–1538. doi: 10.1016/0016-7037(76)90092-2
Bauke, S. L., Sperber, C., von Siebers, N., Tamburini, F., and Amelung, W. (2017). Biopore effects on phosphorus biogeochemistry in subsoils. Soil Biol. Biochem. 111, 157–165. doi: 10.1016/j.soilbio.2017.04.012
Becquer, A., Trap, J., Irshad, U., Ali, M. A., and Claude, P. (2014). From soil to plant, the journey of P through trophic relationships and ectomycorrhizal association. Front. Plant Sci. 5:548. doi: 10.3389/fpls.2014.00548
Blagodatskaya, E., and Kuzyakov, Y. (2013). Active microorganisms in soil: critical review of estimation criteria and approaches. Soil Biol. Biochem. 67, 192–211. doi: 10.1016/j.soilbio.2013.08.024
Blake, R. E., O’Neil, J. R., and Surkov, A. V. (2005). Biogeochemical cycling of phosphorus: insights from oxygen isotope effects of phosphoenzymes. Am. J. Sci. 305, 596–620. doi: 10.2475/ajs.305.6-8.596
Brandtberg, P.-O., Bengtsson, J., and Lundkvist, H. (2004). Distributions of the capacity to take up nutrients by Betula spp. and Picea abies in mixed stands. For. Ecol. Manag. 198, 193–208. doi: 10.1016/j.foreco.2004.04.012
Buehler, S., Oberson, A., Rao, I. M., Friesen, D. K., and Frossard, E. (2002). Sequential phosphorus Extraction oof a 33P-labeled Oxisol under contrasting agricultural systems. Soil Sci. Soc. Am. J. 66, 868–877. doi: 10.2136/sssaj2002.0868
Bünemann, E. K. (2015). Assessment of gross and net mineralization rates of soil organic phosphorus – A review. Soil Biol. Biochem. 89, 82–98. doi: 10.1016/j.soilbio.2015.06.026
Bünemann, E. K., Augstburger, S., and Frossard, E. (2016). Dominance of either physicochemical or biological phosphorus cycling processes in temperate forest soils of contrasting phosphate availability. Soil Biol. Biochem. 101, 85–95. doi: 10.1016/j.soilbio.2016.07.005
Bünemann, E. K., Steinebrunner, F., Smithson, P. C., Frossard, E., and Oberson, A. (2004). Phosphorus dynamics in a highly weathered soil as revealed by isotopic labeling techniques. Soil Sci. Soc. Am. J. 68, 1645–1655. doi: 10.2136/sssaj2004.1645
Chang, S. J., and Blake, R. E. (2015). Precise calibration of equilibrium oxygen isotope fractionations between dissolved phosphate and water from 3 to 37°C. Geochim. Cosmochim. Acta 150, 314–329. doi: 10.1016/j.gca.2014.10.030
Chen, J., Seven, J., Zilla, T., Dippold, M. A., Blagodatskaya, E., Kuzyakov, Y., et al. (2019). Microbial C:N:P stoichiometry and turnover depend on nutrients availability in soil: a 14C, 15N and 33P triple labelling study. Soil Biol. Biochem. 131, 206–216. doi: 10.1016/j.soilbio.2019.01.017
Cohn, M. (1958). Phosphate-water exchange reaction catalyzed by inorganic pyrophosphatase of yeast. J. Biol. Chem. 230, 369–380.
Daroub, S. H., Pierce, F. J., and Ellis, B. G. (2000). Phosphorus fractions and fate of phosphorus-33 in soils under plowing and no-tillage. Soil Sci. Soc. Am. J. 64, 170–176. doi: 10.2136/sssaj2000.641170x
Fanin, N., Fromin, N., Buatois, B., and Hättenschwiler, S. (2013). An experimental test of the hypothesis of non-homeostatic consumer stoichiometry in a plant litter–microbe system. Ecol. Lett. 16, 764–772. doi: 10.1111/ele.12108
Fardeau, J. C., Guiraud, G., and Marol, C. (1995). The role of isotopic techniques on the evaluation of the agronomic effectiveness of P fertilizers. Nutr. Cycling Agroecosyst. 45, 101–109. doi: 10.1007/bf00790659
Frossard, E., Achat, D. L., Bernasconi, S. M., Bünemann, E. K., Fardeau, J.-C., Jansa, J., et al. (2011∗). “The use of tracers to investigate phosphate cycling in soil–plant systems,” in Phosphorus in Action. (Berlin: Bunemann E. K).
Gross, A., and Angert, A. (2015). What processes control the oxygen isotopes of soil bio-available phosphate? Geochim. Cosmochim. Acta 159, 100–111.
Gross, A., and Angert, A. (2017). Use of 13 C- and phosphate 18 O-labeled substrate for studying phosphorus and carbon cycling in soils: a proof of concept: 13 C- and P- 18 O dual-labeled substrate to trace soil C and P cycling. Rapid Commun. Mass Spectrom. 31, 969–977. doi: 10.1002/rcm.7863
Hauenstein, S., Neidhardt, H., Lang, F., Krüger, J., Hofmann, D., Pütz, T., et al. (2018). Organic layers favor phosphorus storage and uptake by young beech trees (Fagus sylvatica L.) at nutrient poor ecosystems. Plant Soil 432, 289–301. doi: 10.1007/s11104-018-3804-5
Helfenstein, J., Pistocchi, C., Oberson, A., Tamburini, F., Goll, D. S., Frossard, E., et al. (2020). Estimates of mean residence times of phosphorus in commonly considered inorganic soil phosphorus pools. Biogeosciences 17, 441–454. doi: 10.5194/bg-17-441-2020
Helfenstein, J., Tamburini, F., von Sperber, C., Massey, M. S., Pistocchi, C., Chadwick, O. A., et al. (2018). Combining spectroscopic and isotopic techniques gives a dynamic view of phosphorus cycling in soil. Nat. Commun. 9:3226.
IUSS Working Group WRB (2006). World Reference Base for Soil Resources 2006 – A Framework for International Classification, Correlation and Communication. Rome: Food and Agriculture Organization of the United Nations.
Jaisi, D. P., Blake, R. E., and Kukkadapu, R. K. (2010). Fractionation of oxygen isotopes in phosphate during its interactions with iron oxides. Geochim. Cosmochim. Acta 74, 1309–1319. doi: 10.1016/j.gca.2009.11.010
Jonard, M., Augusto, L., Morel, C., Achat, D. L., and Saur, E. (2009). Forest floor contribution to phosphorus nutrition: experimental data. Ann. For. Sci. 66:510. doi: 10.1051/forest/2009039
Kottur, J., and Nair, D. T. (2018). Pyrophosphate hydrolysis is an intrinsic and critical step of the DNA synthesis reaction. Nucleic Acids Res. 46, 5875–5885. doi: 10.1093/nar/gky402
Kouno, K., Tuchiya, Y., and Ando, T. (1995). Measurement of soil microbial biomass phosphorus by an anion exchange membrane method. Soil Biol. Biochem. 27, 1353–1357. doi: 10.1016/0038-0717(95)00057-l
Lang, F., Krüger, J., Amelung, W., Willbold, S., Frossard, E., Bünemann, E. K., et al. (2017). Soil phosphorus supply controls P nutrition strategies of beech forest ecosystems in Central Europe. Biogeochemistry 136, 5–29. doi: 10.1007/s10533-017-0375-0
Liang, Y., and Blake, R. E. (2006a). Oxygen isotope composition of phosphate in organic compounds: isotope effects of extraction methods. Organic Geochem. 37, 1263–1277. doi: 10.1016/j.orggeochem.2006.03.009
Liang, Y., and Blake, R. E. (2006b). Oxygen isotope signature of Pi regeneration from organic compounds by phosphomonoesterases and photooxidation. Geochim Cosmochim. Acta 70, 3957–3969. doi: 10.1016/j.gca.2006.04.036
Lis, H., Weiner, T., Pitt, F. D., Keren, N., and Angert, A. (2019). Phosphate Uptake by Cyanobacteria Is Associated with Kinetic Fractionation of Phosphate Oxygen Isotopes. ACS Earth Space Chem. 3, 233–239. doi: 10.1021/acsearthspacechem.8b00099
Longinelli, A., and Nuti, S. (1973). Revised phosphate-water isotopic temperature scale. Earth Planet. Sci. Lett. 19, 373–376. doi: 10.1016/0012-821x(73)90088-5
Manzoni, S., Trofymow, J. A., Jackson, R. B., and Porporato, A. (2010). Stoichiometric controls on carbon, nitrogen, and phosphorus dynamics in decomposing litter. Ecol. Monogr. 80, 89–106. doi: 10.1890/09-0179.1
Marx, M.-C., Wood, M., and Jarvis, S. C. (2001). A microplate fluorimetric assay for the study of enzyme diversity in soils. Soil Biol. Biochem. 33, 1633–1640. doi: 10.1016/s0038-0717(01)00079-7
Melby, E. S., Soldat, D. J., and Barak, P. (2013). Biological decay of 18O-labeled phosphate in soils. Soil Biol. Biochem. 63, 124–128. doi: 10.1016/j.soilbio.2013.03.020
Mészáros, É, Pistocchi, C., Frossard, E., Buenemann, E. K., and Tamburini, F. (2020∗). Changes of fungal and bacterial community composition of forest organic horizons with low and high phosphorus availability, Portail Data INRAE, V1. doi: 10.15454/XOFCHY
Mooshammer, M., Wanek, W., Schnecker, J., Wild, B., Leitner, S., Hofhansl, F., et al. (2012). Stoichiometric controls of nitrogen and phosphorus cycling in decomposing beech leaf litter. Ecology 93, 770–782.
Mooshammer, M., Wanek, W., Zechmeister-Boltenstern, S., and Richter, A. (2014). Stoichiometric imbalances between terrestrial decomposer communities and their resources: mechanisms and implications of microbial adaptations to their resources. Front. Microbiol. 5:22. doi: 10.3389/fmicb.2014.00022
Noack, S. R., Smernik, R. J., McBeath, T. M., Armstrong, R. D., and McLaughlin, M. J. (2014). Assessing crop residue phosphorus speciation using chemical fractionation and solution 31P nuclear magnetic resonance spectroscopy. Talanta 126, 122–129. doi: 10.1016/j.talanta.2014.03.049
Oehl, F., Oberson, A., Sinaj, S., and Frossard, E. (2001). Organic phosphorus mineralization studies using isotopic dilution techniques. Soil Sci. Soc. Am. J. 65, 780–787. doi: 10.2136/sssaj2001.653780x
Ohno, T., and Zibilske, L. (1991). Determination of low concentrations of phosphorus in soil extracts using malachite green. Soil Sci. Soc. Am. J. 55, 892–895. doi: 10.2136/sssaj1991.03615995005500030046x
Orlowski, N., Frede, H. G., Brüggemann, N., and Breuer, L. (2013). Validation and application of a cryogenic vacuum extraction system for soil and plant water extraction for isotope analysis. J Sensors Sensor Syst. 2, 179–193. doi: 10.5194/jsss-2-179-2013
Pfahler, V., Dürr-Auster, T., Tamburini, F., Bernasconi, S. M., and Frossard, E. (2013). 18 O enrichment in phosphorus pools extracted from soybean leaves. New Phytol. 197, 186–193. doi: 10.1111/j.1469-8137.2012.04379.x
Pistocchi, C., Mészáros, É, Tamburini, F., Frossard, E., and Bünemann, E. K. (2018). Biological processes dominate phosphorus dynamics under low phosphorus availability in organic horizons of temperate forest soils. Soil Biol. Biochem. 126, 64–75.
Pistocchi, C., Tamburini, F., Gruau, G., Ferhi, A., Trevisan, D., Dorioz, J. M., et al. (2017). Tracing the sources and cycling of phosphorus in river sediments using oxygen isotopes: methodological adaptations and first results from a case study in France. Water Res. 111, 346–356.
Pistocchi, C., Tamburini, F., Savoye, L., Sebilo, M., Baneschi, L., Lacroix, D., et al. (2014∗). Développement d’une méthode d’extraction et purification des phosphates à partir de matrices sédimentaires pour l’analyse isotopique de leur oxygène. Le cahier des techniques de l’INRA 81, 1–23.
Poll, C., Ingwersen, J., Stemmer, M., Gerzabek, H., and Kandeler, E. (2006). Mechanisms of solute transport affect small-scale abundance and function of soil microorganisms in the detritusphere. Eur. J. Soil Sci. 57, 583–595. doi: 10.1111/j.1365-2389.2006.00835.x
Saaby Johansen, H., Middelboe, V., and Larsen, S. (1991). Delabelling of 18O Enriched Phosphate Added to Soil as a Function of Biological Activity in the Soil. Vienna: IAEA.
Seth, B., Schneider, C., and Storck, F. (2006). Improved reliability of oxygen isotopic analysis of water using the Finnigan GasBench II periphery of a continuous flow isotope ratio mass spectrometer by backflushing of the sampling line. Rapid Commun. Mass Spectrom. 20, 1049–1051. doi: 10.1111/j.1365-2389.2006.00835.x
Siebers, N., Bauke, S. L., Tamburini, F., and Amelung, W. (2018). Short-term impacts of forest clear-cut on P accessibility in soil microaggregates: an oxygen isotope study. Geoderma 315, 59–64. doi: 10.1016/j.geoderma.2017.11.024
Siegenthaler, M. B., Tamburini, F., Frossard, E., Chadwick, O. A., Vitousek, P., Chiara, P., et al. (2020). A dual isotopic (32P and 18O) incubation study to disentangle mechanisms controlling phosphorus cycling in soils from a climatic gradient (Kohala, Hawaii). Soil Biol. Biochem. 149:107920. doi: 10.1016/j.soilbio.2020.107920
Spohn, M., and Chodak, M. (2015). Microbial respiration per unit biomass increases with carbon-to-nutrient ratios in forest soils. Soil Biol. Biochem. 81, 128–133. doi: 10.1016/j.soilbio.2014.11.008
Spohn, M., Ermak, A., and Kuzyakov, Y. (2013). Microbial gross organic phosphorus mineralization can be stimulated by root exudates – A 33P isotopic dilution study. Soil Biol. Biochem. 65, 254–263. doi: 10.1016/j.soilbio.2013.05.028
Stout, L. M., Joshi, S. R., Kana, T. M., and Jaisi, D. P. (2014). Microbial activities and phosphorus cycling: an application of oxygen isotope ratios in phosphate. Geochim. Cosmochim. Acta 138, 101–116. doi: 10.1016/j.gca.2014.04.020
Tamburini, F., Bernasconi, S. M., Angert, A., and Frossard, E. (2010). A method for the analysis of the δ18O of inorganic phosphate extracted from soils with HCl. Eur. J. Soil Sci. 61, 1025–1032. doi: 10.1111/j.1365-2389.2010.01290.x
Tamburini, F., Pfahler, V., Bünemann, E. K., Guelland, K., Bernascon, S. M., Frossard, E., et al. (2012). Oxygen isotopes unravel the role of microorganisms in phosphate cycling in soils. Environ. Sci. Technol. 46, 5956–5962. doi: 10.1021/es300311h
Tamburini, F., Pistocchi, C., Helfenstein, J., and Frossard, E. (2018). A method to analyse the isotopic composition of oxygen associated with organic phosphorus in soil and plant material. Eur. J. Soil Sci. 69, 816–826. doi: 10.1111/ejss.12693
Tiessen, H., and Moir, J. O. (1993). “Characterization of Available P by Sequential Extraction,” in Soil Sampling and Methods of Analysis, eds M. R. Carter and E. G. Gregorich (Ann Arbor, MI: Carter M.R).
Tudge, A. P. (1960). A method of analysis of oxygen isotopes in orthophosphate—its use in the measurement of paleotemperatures. Geochim. Cosmochim. Acta 18, 81–93. doi: 10.1016/0016-7037(60)90019-3
von Sperber, C., Kries, H., Tamburini, F., Bernasconi, S. M., and Frossard, E. (2014). The effect of phosphomonoesterases on the oxygen isotope composition of phosphate. Geochim. Cosmochim. Acta 125, 519–527. doi: 10.1016/j.gca.2013.10.010
von Sperber, C., Tamburini, F., Brunner, B., Bernasconi, S. M., and Frossard, E. (2015). The oxygen isotope composition of phosphate released from phytic acid by the activity of wheat and Aspergillus niger phytase. Biogeosciences 12, 4175–4184. doi: 10.5194/bg-12-4175-2015
Weiner, T., Gross, A., Moreno, G., Migliavacca, M., Schrumpf, M., Reichstein, M., et al. (2018). Following the turnover of soil bioavailable phosphate in mediterranean savanna by oxygen stable isotopes. J. Geophys. Res. 123, 1850–1862. doi: 10.1029/2017JG004086
Werner, F., Mueller, C. W., Thieme, J., Gianoncelli, A., Rivard, C., Höschen, C., et al. (2017). Micro-scale heterogeneity of soil phosphorus depends on soil substrate and depth. Sci. Rep. 7:3203. doi: 10.1038/s41598-017-03537-8
Keywords: soil, isotopic labeling, phosphatase, microbial phosphorus, mineralization
Citation: Pistocchi C, Mészáros É, Frossard E, Bünemann EK and Tamburini F (2020) In or Out of Equilibrium? How Microbial Activity Controls the Oxygen Isotopic Composition of Phosphate in Forest Organic Horizons With Low and High Phosphorus Availability. Front. Environ. Sci. 8:564778. doi: 10.3389/fenvs.2020.564778
Received: 22 May 2020; Accepted: 24 August 2020;
Published: 29 September 2020.
Edited by:
Roland Bol, Helmholtz Association of German Research Centres (HZ), GermanyReviewed by:
Alon Angert, Hebrew University of Jerusalem, IsraelSteve Granger, Rothamsted Research, United Kingdom
Copyright © 2020 Pistocchi, Mészáros, Frossard, Bünemann and Tamburini. This is an open-access article distributed under the terms of the Creative Commons Attribution License (CC BY). The use, distribution or reproduction in other forums is permitted, provided the original author(s) and the copyright owner(s) are credited and that the original publication in this journal is cited, in accordance with accepted academic practice. No use, distribution or reproduction is permitted which does not comply with these terms.
*Correspondence: Federica Tamburini, federica.tamburini@usys.ethz.ch