- 1Department of Energy and Technology, Swedish University of Agricultural Sciences, Uppsala, Sweden
- 2Department of Sanitation, Water and Solid Waste for Development (SANDEC), Swiss Federal Institute of Aquatic Science and Technology (EAWAG), Dübendorf, Switzerland
- 3Civil Engineering Department and the Future Water Institute, University of Cape Town, Cape Town, South Africa
Fresh human urine, after it is alkalized to prevent the enzymatic hydrolysis of urea, can be dehydrated to reduce its volume and to produce a solid fertilizer. In this study, we investigated the suitability of MgO to alkalize and dehydrate urine. We selected MgO due to its low solubility (<2 g·L−1) and relatively high saturation pH (9.9 ± 0.2) in urine. Using a laboratory-scale setup, we dehydrated urine added to pure MgO and MgO mixed with co-substrates (biochar, wheat bran, or calcium hydroxide) at a temperature of 50°C. We found that, dehydrating urine added to a mixture of MgO (25% w/w), biochar, and wheat bran resulted in a mass reduction of >90% and N recovery of 80%, and yielded products with high concentrations of macronutrients (7.8% N, 0.7% P and 3.9% K). By modeling the chemical speciation in urine, we also showed that ammonia stripping rather than urea hydrolysis limited the N recovery, since the urine used in our study was partially hydrolyzed. To maximize the recovery of N during alkaline urine dehydration using MgO, we recommend treating fresh/un-hydrolysed urine a temperature <40°C, tailoring the drying substrate to capture
Introduction
In the decentralized sanitation sector, there is a growing body of research focusing on urine-diversion based systems that treat and recycle human urine as crop fertilizer (Larsen et al., 2013; Martin et al., 2020). Several promising technologies at various scales are being developed across the world for the on-site treatment of urine (Harder et al., 2019). One among these technologies is alkaline dehydration (Simha et al., 2020a; Simha et al., 2020b; Simha et al., 2020c), where urine is dried to produce a solid fertilizer with 10–30 times higher concentrations of plant nutrients than what is originally present in freshly excreted urine. The treatment involves alkalizing urine (pH ≥ 10) to inhibit the enzyme-catalyzed hydrolytic degradation of urea (Krajewska, 2009). In freshly excreted urine, approximately 85% of the N is present as urea (Kirchmann and Pettersson, 1994), whereas in hydrolyzed urine, N is present as ammonia and ammonium instead (Chipako and Randall, 2020). Urease (urea amidohydrolase, EC 3.5.1.5), a nickel-containing metalloenzyme catalyses the hydrolysis of urea (Blakeley et al., 1982; Blakeley and Zerner, 1984). Urease-producing bacteria are widespread in the environment (Mobley and Hausinger, 1989), and are commonly found in urine-diverting sanitation systems (Udert et al., 2003). Therefore, the motivation to alkalize urine is to inhibit enzymatic ureolysis, as this allows evaporating water from urine and recovering N as urea, a widely used crop fertilizer (Dampney et al., 2003).
Several compounds (e.g., thiols, acetohydroxamic acid) can competitively inhibit urease activity (Krajewska, 2009). In previous studies, we have demonstrated that urease present in source-separated urine can be inhibited simply by increasing the pH of urine; according to Geinzer (2017), a pH above 10 is necessary for inactivation. To alkalize urine, wood ash (Dutta and Vinneras, 2016; Senecal and Vinneras, 2017; Senecal et al., 2018; Simha et al., 2018), Ca(OH)2 (Dutta and Vinneras, 2016; Randall et al., 2016; Simha et al., 2020a; Simha et al., 2020b), KOH (Simha et al., 2018) and anion-exchange resin (Simha et al., 2018) can be used as alkalizing agents. While all the aforementioned can increase the pH of fresh urine, not all are equally effective as alkaline substrates for dehydrating urine. Here, effectiveness refers to the volume of urine that can be treated by a unit mass of a substrate under a given set of drying conditions. When urine that is added to an alkaline substrate is dried convectively, its pH gradually decreases, primarily due to the absorption of CO2 which produces
In this regard, there can be many advantages to using sparingly soluble alkaline earth metal compounds [e.g., Ca (OH)2 or Mg (OH)2] as substrates for alkaline urine dehydration. First, because of their low solubility, urine can be dosed passively, meaning the compounds would dissolve as needed depending on the volume of urine added (Flanagan and Randall, 2018). Secondly, urine can be easily saturated with these compounds to attain saturation pH values that do not significantly differ for different urine compositions (Randall et al., 2016). Thirdly, the pH does not vary when urine is dosed in excess of that required for achieving saturation (Randall et al., 2016; Simha et al., 2020b); this is in contrast to alkalizing urine with alkali metal hydroxides (e.g., KOH), where the volume of urine determines dosage and subsequently, the pH.
Past studies have demonstrated that Ca (OH)2 is an effective substrate for alkalising (Randall et al., 2016; Flanagan and Randall, 2018) and dehydrating (Dutta and Vinneras, 2016; Simha et al., 2020b) urine. In this study, we wanted to investigate whether magnesium oxide (MgO) could be used instead for treating urine by alkaline dehydration. The motivation for investigating this was two-fold. On hydration, MgO produces Mg (OH)2 according to the following reaction (Rocha et al., 2004):
When sufficient MgO is added to urine, it creates a saturated solution and increases the urine pH to 9.9 ± 0.2 (Simha et al., in preparation). The increase in pH could be sufficient for inhibiting enzymatic urea hydrolysis but has the co-benefit of minimizing the potential loss of urea to chemical hydrolysis, which is strongly pH dependent (Blakeley et al., 1982). The solubility of MgO in fresh urine at 25°C is <1.5 g·L−1 (Simha et al., in preparation), much lower than the solubility of Ca (OH)2, which according to Randall et al. (2016) varies between 3.5 and 5 g·L−1, since it depends on the urine composition.
MgO has been used previously by others for treating urine, especially for recovering P by precipitating struvite from hydrolyzed urine (Ganrot et al., 2007; Wilsenach et al., 2007). However, in this treatment chain, because of poor capture of N and K, only about 20% of the monetary value of urine as fertilizer can be recovered (Etter et al., 2011). To the best of our knowledge, MgO has not been used to treat urine by alkaline dehydration. Hence, the overall objective of this study was to investigate the alkaline dehydration of urine when added to MgO. The aim was to treat approximately 20 L urine d−1m−2 using pure MgO or MgO mixed with the following co-substrates—biochar, wheat bran, or Ca (OH)2. The co-substrates biochar and bran were added to break up peptide films that usually form over the surface of urine and limit the evaporative removal of water (Tettenborn et al., 2007). Specifically, we investigated the 1) recovery of N; 2) changes in the physical and chemical properties of the drying substrate; and, 3) the elemental composition of the end-products. In addition, by modeling the chemical speciation, we provided insights into the solubility and saturation pH of MgO added to human urine.
Methodology
Materials
Urine
Around 20 volunteers (male and female, aged 25–65 years) anonymously donated urine in 500 ml sterile high-density polyethylene bottles at different times of the day. The filled bottles were collected at the end of the working day (<12 h) and refrigerated at 3°C (±1°C) for <14 days. When it was needed, urine was removed from the fridge, mixed in a 5 L Florence flask, heated using a water bath to 37 ± 1°C (to simulate its temperature at excretion), and used in the drying experiments.
Alkaline substrate
Laboratory grade MgO (Acros Organics, Belgium) was used in powder form (d99 < 150 µm). Food grade wheat bran (Kungsörnen, Sweden), technical grade Ca (OH)2 (Nordkalk Corporation, Sweden) and non-activated biochar (Vindelkol AB, Sweden; particle Ø < 1 mm) were used without any pre-treatment.
Five different alkaline substrates were evaluated through an exploratory approach (Table 1). In all substrates, the content of MgO was in excess to that required for saturating approximately 1 L of fresh urine. The co-substrates were pure biochar and a mixture of biochar and wheat bran. Both the co-substrates were tested with and without addition of Ca (OH)2. This was done to assess the effect of high pH (>12) at the start of the treatment on the recovery of N from urine.

TABLE 1. Mass composition (as % of total mass) of the five drying substrates used in this study. The total mass of each substrate was 30 g.
Experiment
The study was performed in a modified benchtop incubator that was used in Simha et al. (2020b). Briefly, the setup consisted of four air pumps (Rena 301, Rena Aquatic Supply, United States) which introduced air at the rate of 5 L·min−1 while eight computer fans (Spire Corp, Netherlands) redistributed the air inside the incubator cavity. The incubator had two stainless steel grates over which square polystyrene Petri dishes (Sarstedt, Germany; dimensions 100 mm × 100 mm × 20 mm) were placed. The temperature of the incubator was set to have an average drying temperature of 50°C. There were two reasons for selecting this drying temperature. First, we wanted to minimize the possibility of losing urea-N to chemical hydrolysis, which increases rapidly above 50°C (Blakeley et al., 1982). Secondly, we wanted to have high dehydration rates, and a previous experiment demonstrated that up to 20 L of urine could be dried per day per square meter at this temperature (Simha et al., 2020b).
At the start of the experiment, the Petri dishes contained 30 g triplicates of the five alkaline substrates. To each Petri dish, pre-heated urine (37 ± 2°C) was added and dried at 50°C for a fixed duration of time. Then, the Petri dishes were withdrawn from the incubator, marking the end of what we called a drying cycle. In the first drying cycle, 90 ml of urine was added to prevent air suspension of the substrate; in the rest of the cycles, 30 ml of urine was added. Based on results obtained in Simha et al. (2020b), the drying time was fixed at 7 min·ml−1 urine. However, the incubator was operated for an additional hour if it was observed that urine pooled over surface of the substrate; there were five such prolonged drying cycles. In total, there were 35 drying cycles and the total drying time was 5.6 days, with each Petri dish treating 1.11 L of urine. We performed the drying treatment in cycles in order to represent real-life implementation, where the surface area available for drying urine inside bathrooms is usually small and drying would have to be performed after every urination event.
Chemical and Physical Analyses
At the end of every working day, 10 ml aliquots of the urine used in the experiments was sampled and refrigerated at −20°C. At the end of the experiment, the urine was thawed, pooled and analyzed for pH, electrical conductivity (EC), Total Solids (TS), Volatile Solids (VS), total N, total P and NH4-N. The pH was measured using a combination electrode coupled to a PHM210 meter (Radiometer Analytical, France). EC was measured using a handheld multimeter (Cond 340i, WTW, Germany) and a TetraCon 325 probe (WTW, Germany). Urine samples were dried at 110°C for 14 h for determining TS and then combusted at 550°C for 6 h for determining VS. The TS content of urine was adjusted to account for the loss of urea due to heat degradation. The urea concentration was calculated by estimating urea-N to be 70% of the total N in urine, since the NH4-N concentration was 1.7 g·L−1.
Spectroquant® test kits (Merck KGaA, Germany) were used according to manufacturer instructions to measure NH4-N (test kit 100683; measurement range 5–150 mg·L−1), total N (test kit 109713; measurement range 0.1–25 mg·L−1), and total P (test kit 114848; measurement range 0.05–5.0 mg·L−1). For this analysis, urine was diluted with deionized water and then digested using either Spectroquant® Crack-Set 10 (for total P) or Crack-Set 20 (for total N). Colorimetric readings were taken with a photometer (NOVA 60 A Spectroquant®, Merck KGaA, Germany).
The alkaline substrate was characterized, before and after urine dehydration for pH, EC, TS, VS and elemental composition (N, P, K, C and S). The pH and EC were measured for 1:5 (w/v) substrate: urine suspensions at room temperature (21 ± 2°C) after a resting time of 1 h. The N and C content was determined using an elemental analyser (LECO TruMac® CN, United States) while the P, K, and S content was measured by optical emission spectrometry (Optima Avio 200, PerkinElmer, United States).
Calculations
To evaluate the effectiveness of the substrates to reduce the mass of urine, on wet basis (WB) we calculated the % mass reduction (mass.redWB; Eq. 1), mass concentration factor (mass.cfWB; Eq. 2) and the average dehydration rate (
A mass balance was carried out to calculate the experimental recovery of total N from urine. This was then compared against the potential recovery of urea-N when drying urine at the incubator’s average recorded temperature (48.1 ± 1.5°C). At this temperature, the half-life of urea was calculated to be 133 days using the equation described in Simha et al. (2020b). We assumed that N in urine is present only as urea and NH4+ and approximated the urea-N concentration in urine as 70% of the total N measured by the experiment (5.7 ± 0.1 g·L−1) since the NH4-N concentration was 1.7 g·L−1.
Statistical Analysis
The Shapiro-Wilk test was used to verify data normality and the Brown-Forsythe-Levene test was used to verify equal variance between the data for the different drying substrates. To identify significant differences between the substrates, at 95% confidence interval, analysis of variance (ANOVA) followed by Tukey’s multiple comparison test (Honest Significant Difference, HSD) was performed. Additionally, unpaired two-sample Student’s t-test was performed to compare the physicochemical properties of the substrates at the start and at the end of the treatment. All the statistical analyses were performed using RStudio version 1.2.5042 and R version 4.0.0 (RStudio Team, 2016). The R-packages car (Fox and Weisberg, 2018) and agricolae (de Mendiburu, 2020) were used for these analyses.
Modeling Chemical Speciation
The composition shown in Table 2 was used to calculate the chemical speciation at different conditions using OLI Stream Analyzer (OLI Systems Inc., 2020) and the Mixed Solvent Electrolyte (MSE) model option of the software. These simulations were run to understand the solubility of MgO in urine, the saturation pH of urine, as well as the effect of temperature on both parameters. We also modeled the urine with a reduced ammonia N concentration (400 mg·L−1) as against the composition measured in this study, 1,700 mg·L−1, to understand the effect ammonia N has on the solubility of MgO. The results were used to develop speciation curves for ammonia N in urine at different pH values, temperatures, and water content (as the treatment involves dehydration of urine). For this simulation, we used MgO/Ca (OH)2 to increase the pH of urine and acid (HCl) to decrease its pH so as to have a range from 6 to 13.
Results
Mass Balance
Treating urine by alkaline dehydration reduced its mass by >90% in all the substrates. Pure MgO was the most efficient in terms of reducing the mass of urine; the mass concentration factor reduced with addition of co-substrates (Table 3). The end-product obtained by drying urine in pure MgO weighed the least (14 times less) and was significantly different (p < 0.001) than the rest. The average dehydration rate varied between 19.1 ± 4.3 (for MgO-Bc) and 19.7 ± 4.5 kg·m−2d−1 (for pure MgO), although there was no significant difference (p < 0.05) between the substrates.

TABLE 3. The mass reduction (mass.redWB), mass concentration factor (mass.cfWB), dehydration rate (dry.rateWB), and recovery of N after treating urine with MgO-based drying substrates.
A mass balance was carried out to estimate the recovery of N from urine after the dehydration treatment (Supplementary Figure S1). The least N recovery (only 67%) was observed for urine treated using pure MgO. However, the recovery improved in the presence of co-substrates (Table 3). The recovery was highest when urine was dehydrated in MgO (25% w/w) mixed with equal parts biochar and wheat bran. Furthermore, the addition of Ca (OH)2 to the substrate resulted in lower N recovery although the difference was not always significant.
Treating urine by dehydration at 50°C and pH of approximately 10 resulted in >20% loss of N. Of the total N present in urine, approximately 70% was present in the form of urea-N (Methodology section). Following Warner (1942), under the treatment conditions, the potential loss of urea-N to chemical hydrolysis was only 1.2%. Therefore, the loss of N was due to other mechanisms.
End-Product Composition
On a TS basis, drying urine in pure MgO yielded end-products containing 6.5% N, 0.5% P, and 3.3% K. Adding co-substrates to MgO improved its NPK content (Table 4). The most concentrated NPK product was obtained when the substrate contained biochar as well as wheat bran; on average, it had 7.8% N, 0.7% P and 3.9% K. The presence of Ca (OH)2 did not significantly alter this composition (p < 0.05). The C content of urine dried in pure MgO was only 6.8% but was as high as 31% for MgO-Bc. The end-product C content depended on the amount of biochar or wheat bran initially added to the substrate. All the end-products also contained 0.6% S.
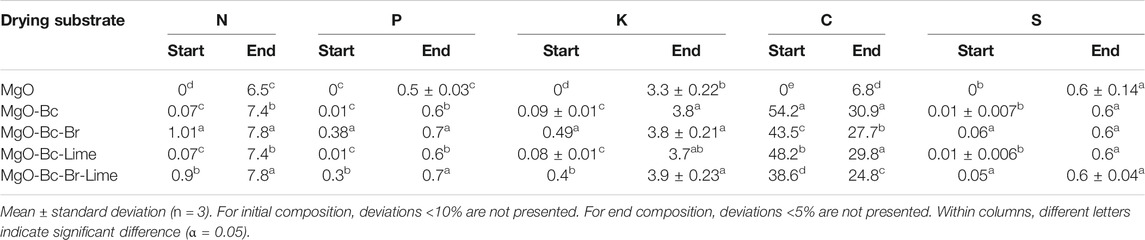
TABLE 4. The elemental composition (as % of TS) of the drying substrate at the start and at the end, after dehydrating urine for 5.6 days.
Physicochemical Properties
At the start of the treatment, at room temperature (21 ± 2°C), all the substrates were alkaline (pH ≥ 9.8). The substrates with only MgO as the alkalizing agent had a pH of about 10, reflecting the pH of urine saturated with MgO/Mg (OH)2 (Simha et al., in preparation). Likewise, the initial pH of the substrates containing Ca (OH)2 was >12.5, which is the saturation pH of urine alkalized with >5 g Ca (OH)2 L−1 (Randall et al., 2016).
Following treatment, the pH of all the substrates significantly decreased, especially in the substrates that initially contained Ca (OH)2. Yet, there was no significant difference (p > 0.05) between the different substrates since their average pH was around 10 (Table 5). All the substrates apart from pure MgO had similar EC, approximately 25 mS·cm−1 when measured as 1:5 (substrate: urine) suspensions. Compared to the initial EC, the end-products had higher EC because of accumulation of urine salts [Simha et al. (2020a) for more information on the salt content of dried urine fertilizer]. The EC of the urine added to the treatment was 11.7 ± 1.2 mS·cm−1.
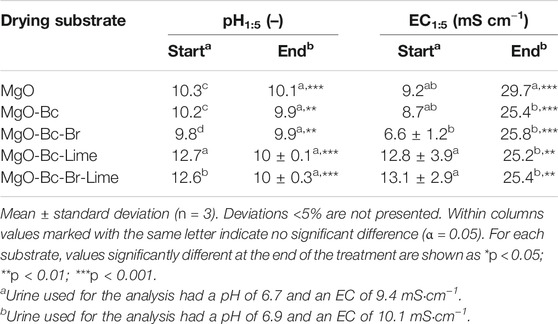
TABLE 5. pH and EC of the substrates at the start and at the end of the dehydration treatment measured in 1:5 (w/v) substrate: urine suspensions at room temperature (21 ± 2°C).
Discussion
Factors Affecting the Recovery of Nitrogen
The initial ammonia N concentration of the urine used in the experiment was 30% of its total N concentration, and considerably higher than what is expected in fresh urine (<5%). This indicated that urea was partially hydrolyzed during the storage of urine and before it was used in the drying treatment. When the partially hydrolyzed urine was treated by alkaline dehydration, between 20 and 33% of the N was lost, depending on the type of the alkaline substrate. Drying urine at 50°C using pure MgO recovered 67% of the N. At the same drying temperature, Simha et al. (2020b) reported a N recovery of 78% when drying fresh human urine with initial ammonia concentration of 590 mg·L−1 using pure Ca (OH)2. The N recovery of MgO improved with the addition of co-substrates; the recovery was maximum when MgO was mixed with both biochar and wheat bran (Table 2). Substituting MgO with Ca (OH)2 resulted in a higher saturation pH but lowered the N recovery, although the differences were insignificant (p < 0.05).
The potential loss of urea-N (70% of the total N) due to chemical hydrolysis was calculated to be just 1.2%. The average pH of the substrates collected at the end of the experiment was 10 ± 0.2 (measured at 21 ± 2°C), indicating they were still strongly alkaline and thus, should have inhibited enzymatic ureolysis. However, both the solubility and the pH of the alkalizing agents used in this study were influenced by temperature (Figures 1A,B). At 50°C, the saturation pH of urine added to MgO was only 8.8 (Figure 1B). It is unclear how the reduced pH in combination with the high EC of the substrates affected the urease enzyme activity. The high ammonia concentration in the urine used in this study (Table 2) indicated the presence of urease enzymes. Yet, the end-products recovered >70% N suggesting that the enzyme activity was inhibited to some extent. Further studies are required to fully understand the enzymatic activity in such treatment conditions.
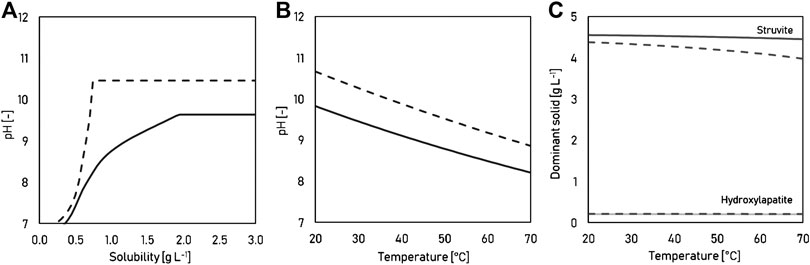
FIGURE 1. Plots show the simulated (A) pH of urine vs. the solubility of MgO at 25°C; (B) pH of urine saturated with MgO vs. temperature; and (C) dominant solids (struvite and hydroxylapatite) formed in saturated urine at different temperatures. Solid line represents the urine composition of this study (Table 1), while dashed line represents the same but with
The most plausible explanation for the high N loss is ammonia stripping (Huang and Shang, 2006; Başakçilardan-Kabakci et al., 2007). In urine, ammonia nitrogen exists as both free ammonia/dissolved gas (NH3) and ammonium
Yet, our findings indicate that the drying substrates can significantly influence the rate of ammonia gas transfer. First, the material properties of the substrate can affect the molecular diffusion of ammonia. For instance, we often observed urine pooling over the surface of pure MgO because of its low solubility. However, the pooling of urine was either seldom or not observed when MgO was mixed with biochar and/or bran; both co-substrates have high water absorption capacities Supplementary Figure S2. It is possible that the presence of co-substrates limited the rate of molecular diffusion of NH3(aq), and therefore its transfer to the gas phase. Secondly, the drying substrate can prevent N loss by precipitating
The average ratio of Mg:NH4:PO4 in urine was 1:0.5:2.8 whereas, in theory, a ratio of 1:1:1 is needed to form struvite (Ronteltap et al., 2007). In freshly excreted urine, Mg2+ is present in low concentration (Jönsson et al., 1997), which limits the self-precipitation of P as struvite. All the drying substrates used in this study contained MgO, which on hydration provided Mg2+; the Mg:PO4 ratio varied between 2.2:1 (at 50°C) and 2.5:1 (at 20°C). Hence, the N capture as struvite was limited by
Study Implications and Limitations
In this study, we reduced >90% of the mass of human urine by dehydrating it in alkaline substrates containing different amounts of MgO. Overall, we were able to treat 37 L urine kg−1 substrate. This suggests that <15 kg of drying substrate is needed to treat the urine excreted by an average person in a year [550 kg according to Vinnerås et al. (2006)] and to yield 40–54 kg end-product cap−1y−1. Therefore, drying urine has the potential to improve the logistics surrounding urine recycling since the alternative is to store, transport and apply large volumes of liquid urine as fertilizer (Johansson et al., 2001). The dried urine products can find use as crop fertilizer as they contain 7.8% N, 0.4% P, and 3.9% K. It may be possible to improve the fertilizer value of the products by drying more urine within the same substrate, since the pH of the substrate was still ≥10.
Our primary focus in this study was to investigate the use of MgO to alkalize and dehydrate human urine. We found that <2 g MgO L−1 is required to saturate urine and increase its pH to about 10, which is sufficient alkalinity to inactivate urease enzymes, according to Geinzer (2017). We also observed that the alkalinity of all the substrates was preserved despite dehydrating urine added to them. While our study did not explore the maximum urine volume that can be treated before the substrate pH decreased to <10, we found that at least 150 L of urine can be treated using 1 kg MgO. This translates to an MgO requirement of only 3.6 kg cap−1y−1 and an annual cost of US$ 1.1 cap−1y−1. The world’s Mg reserves are virtually unlimited and the bulk price of MgO is very low (US$ 0.3 kg−1) (Bray and Ghalayin, 2020). We therefore find MgO to be a very promising substrate for performing alkaline urine dehydration.
There were two limitations in our study. First, we did not expect the urine we used in our experiments to be partially hydrolyzed during the collection. The technology, alkaline urine dehydration, is designed to treat freshly excreted urine. Secondly, on modeling the chemical speciation, we found out that MgO displayed inverse solubility, i.e., its solubility in urine decreased with temperature (Figure 1A). Therefore, at 20°C, adding the urine we used in our study to MgO resulted in a saturation pH of 9.8, but increasing its temperature to 50°C during the dehydration treatment decreased the pH to 8.8. Even if the urine was not partially hydrolyzed, as was the case in our experiments, increasing its temperature to >40°C would decrease the saturation pH to <10. This would increase the risk for reactivation of urease, since alkalizing urine with MgO to a pH just above 10 only inactivates the enzyme reversibly. Maintaining a high pH is more important than the potential benefit of having a higher dehydration rate at high temperatures. Therefore, based on results we obtained from our experiment and from the chemical modeling, we tentatively recommend that urine should be dehydrated at temperature below 40°C if it is alkalized using MgO in order to limit the loss of urea to enzymatic hydrolysis.
Due to the presence of active urease enzymes, the urine used in this study had high initial ammonia N concentration, about 30% of the total N. However, under the dehydration treatment conditions (pH 10 and 50°C), >80% of the ammonia was in the form of NH3(aq) which could not be recovered. Thus, at least 24% of the N loss was attributed to ammonia stripping. Chemical speciation modeling showed that only 4% of the N could be recovered as precipitated struvite, suggesting that the N loss as NH3(g) was much higher. For instance, in pure MgO, the N loss was 33%, of which only 1.2% of the N was lost due to chemical hydrolysis of urea. Therefore, to maximise the N recovery during alkaline dehydration, it is essential to collect and alkalize fresh urine to pH > 10 in short time periods. For practical implementation of the technology, this means that dehydration has to take place next to the toilet as this reduces the likelihood of urea hydrolyzing because of the enzymatic activity of microbial biofilms, which are invariably present in the pipe network. Nonetheless, two options exist if urine has to be transported by pipe. First, urine can be pre-treated to prevent urea hydrolysis, e.g., by ion exchange (Simha et al., 2018) or by adding alkalising agents on-site (Chipako and Randall, 2020). Second, the drying substrate can be tailored to capture
Conclusion
This study explored the use of MgO for treating human urine. We found MgO to be an effective alkalizing agent, as <2 g·L−1 was required to saturate urine and increase its pH from <7 to around 10. However, we also demonstrated that MgO displayed inverse solubility in urine, and that at temperatures >40 °C, its saturation pH decreased <10, which increased the risk for reactivation of urease. We also demonstrated that MgO could be used for treating urine by alkaline dehydration. At a drying temperature of 50°C, we recovered up to 80% of the N from urine by using an alkaline substrate that comprised of MgO mixed with biochar and wheat bran. The products obtained by dehydrating urine had high concentrations of macronutrients (7.8% N, 0.7% P, and 3.9% K) and can find use as crop fertilizers. Our study also demonstrated that in addition to urea hydrolysis, N from urine could be lost during alkaline dehydration due to ammonia stripping, especially if urine is partially hydrolyzed, either during storage or during dehydration and thus, has high ammonia N concentration. Therefore, based on experimental observations and preliminary modeling results, we recommended treatment conditions that can minimise the loss of N as NH3.
Data Availability Statement
The raw data supporting the conclusions of this article will be made available by the authors, without undue reservation.
Author Contributions
PS, BV, and CF conceptualized the study and designed the experiments. CF and PS performed the experiments, the physicochemical analyses, and the formal data analysis. DGR modeled the chemical speciation of urine using OLI. BV supervised the study, provided resources and acquired funding. PS wrote the first draft of the manuscript. All authors contributed to manuscript revision, read and approved the submitted version.
Funding
Research presented in this study was financed by grants from the Swedish Research Council, “Productive on-site sanitation system: new value chain for urine based fertilizer” (Grant Number 2015-03072) and “UDT 2.0—Urine Dehydration Technology for Sanitation 2.0” (Grant Number 2018-05023). DG Randall was supported through the FLAIR Fellowship by the Royal Society and the African Academy of Sciences.
Conflict of Interest
The authors declare that the research was conducted in the absence of any commercial or financial relationships that could be construed as a potential conflict of interest.
Acknowledgments
Sven Smårs helped build the experimental setup. Colleagues at SLU are thanked for donating urine.
Supplementary Material
The Supplementary Material for this article can be found online at: https://www.frontiersin.org/articles/10.3389/fenvs.2020.619901/full#supplementary-material.
References
Başakçilardan-Kabakci, S., İpekoğlu, A. N., and Talinli, I. (2007). Recovery of ammonia from human urine by stripping and absorption. Environ. Eng. Sci. 24 (5), 615–624. doi:10.1089/ees.2006.0412
Blakeley, R. L., Treston, A., Andrews, R. K., and Zerner, B. (1982). Nickel (II)-promoted ethanolysis and hydrolysis of N-(2-Pyridylmethyl) urea. A model for urease. J. Am. Chem. Soc. 104 (2), 612–614. doi:10.1021/ja00366a040
Blakeley, R. L., and Zerner, B. (1984). Jack bean urease: the first nickel enzyme. J. Mol. Catal. 23 (2–3), 263–292. doi:10.1016/0304-5102(84)80014-0
Bray, E. L., and Ghalayin, Z. T. (2020). 2017 minerals yearbook magnesium compounds. VA, Virginia: U.S. Department of the Interior and U.S. Geological Survey.
Chipako, T. L., and Randall, D. G. (2020). Urine treatment technologies and the importance of pH. J. Environ. Chem. Eng. 8 (1), 103622. doi:10.1016/j.jece.2019.103622
Dampney, P. M. R., Basford, W., Goodlass, G., Miller, P., and Richards, I. (2003). Report for Defra Project NT2601. Production and use of nitrogen fertilizers (Accessed June, 2003).
de Mendiburu, F. (2020). Statistical procedures for agricultural research. 2nd Edn. NJ, New Jersey: Wiley.
Dutta, S., and Vinnerås, B. (2016). Fertilizer from dried human urine added to ash and lime—a potential product from eco-sanitation system. Water Sci. Technol. 74 (6), 1436–1445. doi:10.2166/wst.2016.324 |
Etter, B., Tilley, E., Khadka, R., and Udert, K. M. (2011). Low-cost struvite production using source-separated urine in Nepal. Water Res. 45 (2), 852–862. doi:10.1016/j.watres.2010.10.007 |
Flanagan, C. P., and Randall, D. G. (2018). Development of a novel nutrient recovery urinal for on-site fertilizer production. J. Environ. Chem. Eng. 6 (5), 6344–6350. doi:10.1016/j.jece.2018.09.060
Fox, J., and Weisberg, S. (2018). An R companion to applied regression. Los Angeles, London: Sage Publications.
Ganrot, Z., Dave, G., and Nilsson, E. (2007). Recovery of N and P from human urine by freezing, struvite precipitation and adsorption to zeolite and active carbon. Bioresour. Technol. 98 (16), 3112–3121. doi:10.1016/j.biortech.2006.10.038 |
Geinzer, M. (2017). Inactivation of the urease enzyme by heat and alkaline pH treatment. Master’s thesis. Uppsala, Sweden: Swedish University of Agricultural Sciences.
Harder, R., Wielemaker, R., Larsen, T. A., Zeeman, G., and Öberg, G. (2019). Recycling nutrients contained in human excreta to agriculture: pathways, processes, and products. Crit. Rev. Environ. Sci. Technol. 49 (8), 695–743. doi:10.1080/10643389.2018.1558889
Huang, J.-C., and Shang, C. (2006). Advanced physicochemical treatment processes-air stripping. London, United Kingdom: IWA.
Johansson, M., Jönsson, H., Höglund, C., Richert Stintzing, A., and Rodhe, L. (2001). Urine separation–closing the nutrient cycle. Final report on the R&D project source-separated human urine–a future source of fertilizer for agriculture in the Stockholm region. Stockholm, Sweden: Stockholm Water Company.
Jönsson, H., Stenström, T. A., Svensson, J., and Sundin, A. (1997). Source separated urine-nutrient and heavy metal content, water saving and faecal contamination. Water Sci. Technol. 35 (9), 145–152.
Kirchmann, H., and Pettersson, S. (1994). Human urine-chemical composition and fertilizer use efficiency. Fert. Res. 40 (2), 149–154. doi:10.1007/BF00750100
Krajewska, B. (2009). Ureases I. Functional, catalytic and kinetic properties: a review. J. Mol. Catal. B Enzym. 59 (1–3), 9–21. doi:10.1016/j.molcatb.2009.01.003
Larsen, T. A., Udert, K. M., and Lienert, J. (2013). Source separation and decentralization for wastewater management. London, United Kingdom: IWA Publishing.
Martin, T. M. P., Esculier, F., Levavasseur, F., and Houot, S. (2020). Human urine-based fertilizers: a review. Crit. Rev. Environ. Sci. Technol. 1–47. doi:10.1080/10643389.2020.1838214
Mobley, H. L., and Hausinger, R. P. (1989). Microbial ureases: significance, regulation, and molecular characterization. Microbiol. Rev. 53 (1), 85–108. |
Randall, D. G., Krähenbühl, M., Köpping, I., Larsen, T. A., and Udert, K. M. (2016). A novel approach for stabilizing fresh urine by calcium hydroxide addition. Water Res. 95, 361–369. doi:10.1016/j.watres.2016.03.007 |
Rocha, S. D. F., Mansur, M. B., and Ciminelli, V. S. T. (2004). Kinetics and mechanistic analysis of caustic magnesia hydration. J. Appl. Chem. Biotechnol. 79 (8), 816–821. doi:10.1002/jctb.1038
Ronteltap, M., Maurer, M., and Gujer, W. (2007). Struvite precipitation thermodynamics in source-separated urine. Water Res. 41 (5), 977–984. doi:10.1016/j.watres.2006.11.046 |
Senecal, J., Nordin, A., Simha, P., and Vinnerås, B. (2018). Hygiene aspect of treating human urine by alkaline dehydration. Water Res. 144, 474–481. doi:10.1016/j.watres.2018.07.030 |
Senecal, J., and Vinnerås, B. (2017). Urea stabilisation and concentration for urine-diverting dry toilets: urine dehydration in ash. Sci. Total Environ. 586, 650–657. doi:10.1016/j.scitotenv.2017.02.038 |
Simha, P., Senecal, J., Nordin, A., Lalander, C., and Vinnerås, B. (2018). Alkaline dehydration of anion-exchanged human urine: volume reduction, nutrient recovery and process optimisation. Water Res. 142, 325–336. doi:10.1016/j.watres.2018.06.001 |
Simha, P., Deb, C. K., Randall, D. G., and Vinnerås, B. (in preparation). Solubility curves for MgO and Mg(OH)2 in fresh urine. Bethesda, Maryland: NCBI.
Simha, P., Karlsson, C., Viskari, E.-L., Malila, R., and Vinnerås, B. (2020a). Field testing a pilot-scale system for alkaline dehydration of source-separated human urine: a case study in Finland. Front. Environ. Sci. 8, 570637. doi:10.3389/fenvs.2020.570637
Simha, P., Lalander, C., Nordin, A., and Vinnerås, B. (2020b). Alkaline dehydration of source-separated fresh human urine: preliminary insights into using different dehydration temperature and substrate. Sci. Total Environ. 733, 139313. doi:10.1016/j.scitotenv.2020.139313 |
Simha, P., Senecal, J., Gustavsson, D. J. I., and Vinnerås, B. (2020c). “Resource recovery from wastewater: a new approach with alkaline dehydration of urine at source,” in Current developments in biotechnology and bioengineering: sustainable bioresources for emerging bioeconomy, Editors A. Pandey, R. Kataki, D. Pant, and S. Khanal (Netherlands: Elsevier Publications).
Tettenborn, F., Behrendt, J., and Otterpohl, R. (2007). Final Report. Resource recovery and removal of pharmaceutical residues-treatment of separate collected urine (Accessed March, 2017).
Udert, K. M., Larsen, T. A., Biebow, M., and Gujer, W. (2003). Urea hydrolysis and precipitation dynamics in a urine-collecting system. Water Res. 37 (11), 2571–2582. doi:10.1016/s0043-1354(03)00065-4 |
Vinnerås, B., Palmquist, H., Balmér, P., and Jönsson, H. (2006). The characteristics of household wastewater and biodegradable solid waste—a proposal for new Swedish design values. Urban Water J. 3 (1), 3–11. doi:10.1080/15730620600578629
Warner, R. C. (1942). The kinetics of the hydrolysis of urea and of arginine. J. Biol. Chem. 142 (2), 705–723.
Keywords: ammonia, fertilizer, nitrogen recycling, urine source separation, wastewater, urine dehydration, sanitation, MgO
Citation: Simha P, Friedrich C, Randall DG and Vinnerås B (2021) Alkaline Dehydration of Human Urine Collected in Source-Separated Sanitation Systems Using Magnesium Oxide. Front. Environ. Sci. 8:619901. doi: 10.3389/fenvs.2020.619901
Received: 21 October 2020; Accepted: 14 December 2020;
Published: 20 January 2021.
Edited by:
Efthalia Chatzisymeon, University of Edinburgh, United KingdomReviewed by:
Kangning Xu, Beijing Forestry University, ChinaElizabeth Tilley, University of Malawi, Malawi
Copyright © 2021 Simha, Friedrich, Randall and Vinnerås. This is an open-access article distributed under the terms of the Creative Commons Attribution License (CC BY). The use, distribution or reproduction in other forums is permitted, provided the original author(s) and the copyright owner(s) are credited and that the original publication in this journal is cited, in accordance with accepted academic practice. No use, distribution or reproduction is permitted which does not comply with these terms.
*Correspondence: Prithvi Simha, UHJpdGh2aS5TaW1oYUBzbHUuc2U=, cHJpdGh2aS5zaW1oYUBtZXNwb20uZXU=