- 1NORCE Norwegian Research Centre, Environment, Bergen, Norway
- 2Norwegian Veterinary Institute, Bergen, Norway
Microplastic pollution is omnipresent in biota around the globe, and concerns are rising that humans are exposed to microplastics (MP) through food. Investigations of MP in wild animals relevant for human consumption and the effects in exposed birds and mammals is warranted. We investigated the concentrations of MP in organs and tissues of fish, seabirds, terrestrial and marine mammals from a plastic polluted area near Bergen, Norway. A standardized autopsy included evaluation of condition, bacteriological and histopathological analyzes. Tissues were analyzed for MP (>10 µm) by pyrolysis Gas Chromatography Mass Spectrometry (py-GCMS) and inspected by polarized light microscopy. We analyzed samples of stomach and intestinal wall, liver and muscle/fillet from three flounders, three cod, three seabirds, three otters and one seal, kidneys from seabirds, otters and the seal, and gills from the fishes. No large plastic items were observed in the gastrointestinal tracts. Eight of 13 animals had MP in one or several tissues. MP was found in intestine (5), stomach (4), liver (3), muscle (3). No MP was found in the seal, and only in the stomach wall of one otter. In seabirds, MP was found in the intestine, stomach and liver, but not muscle. The highest concentration was 3.4 µg/g wet weight in cod liver. Three of the nine investigated polymers were found above the Limit of Quantification (LOQ): Polyvinylchloride>polystyrene>>polyethylene terephthalate. MP was quantified in one of four replicates of cod muscle and one of two replicates of cod liver. No MP was observed by microscopy. The results show levels under or close to the current LOQ. Replicates indicate uneven MP distribution in tissues and resulted in higher prevalence of MP for cod. No adverse effects could be related to MP. The sample size was small, and conclusions cannot be drawn regarding effects or risks. The animals were by-catch, and mostly in good condition when caught. Procedural blanks and air-controls showed very low MP, and support that the MP come from environmental sources. Further studies are needed to determine levels of microplastic in edible tissues and the current wildlife exposure through the food web.
LSID: Harbor seal (Phoca vitulina), LSID: urn:lsid:zoobank.org:act:064A76C7-49C4-4231-842D-67C82DACD58E; Eurasian otter (Lutra lutra), LSID: urn:lsid:zoobank.org:pub:E1B2A866-06A0-4687-A18D-482D27424816; Red breasted merganser (Mergus serrator), LSID: urn:lsid:zoobank.org:act:7723FFD6-5FBF-4134-AE15-4B7728D78DD4; Common guillemot, common murre or thin billed murre (Uria aalge), LSID: unknown; Cod (Gadus morhua), LSID: urn:lsid:zoobank.org:act:389BE401-2718-4CF2-BBAE-2E13A97A5E7B; Flounder (Limanda limanda), LSID: urn:lsid:zoobank.org:act:A9350141-7463-4878-85E4-4629F310F317.
Introduction
The increasing amounts of plastic pollution in the ocean is a global concern, yet the distribution, environmental fate and detrimental effects of microplastic (MP) (<1000 µm, (Hartmann et al. 2019) in the ocean is still poorly understood. Evidence from the past years have documented the omnipresence of microplastic pollution in deep oceans, in Antarctica and the Arctic, on land, and in air and atmospheric fallout (Dris et al. 2016; Bergmann et al. 2017; Courtene-Jones et al. 2017; Munari et al. 2017; Bergmann et al. 2019; Wright et al. 2020). Recent expert group opinions state that current knowledge is insufficient to evaluate the risks from human or ecosystem exposure to MP (Skåre et al., 2019). It has also been noted that global ecosystem effects are hard to document at this stage, but that the lack of documentation should not be taken as evidence of no risk (SAPEA 2019). A recent, systematic literature review and resulting Species Sensitivity Distribution concluded that the lowest Hazard concentration or Predicted No Effect Concentration was within the range that can be considered environmentally relevant in some regions (Skåre et al., 2019), thus effects of MP may already be detrimental to populations of vulnerable species in some highly polluted regions. Moreover, this indicates that effects will become increasingly evident if general levels continue to rise. Prospective studies and models report that levels high enough to cause detrimental effects of sedimented and beached plastics are expected before the end of the century, and that such levels have already been reported in different ecosystem compartments (Everaert et al. 2018). MP fragments in the ocean likely end up on the ocean floor (Barnes et al. 2009; Woodall et al. 2014) while macroplastic items either sink or are deposited along the shorelines, depending on their density and buoyancy (Jambeck et al. 2015; Lebreton et al. 2017; Lebreton and Andrady 2019). Along shorelines, macroplastics degrade and generate MP by a number of processes such as UV-radiation, temperature changes, microbial degradation, abrasion and leaching of plasticizers (Andrady et al. 2003; Andrady 2011; Andrady 2017; Urbanek et al. 2018). The MP generated from degradation on land may potentially re-enter the coastal waters by wind and wave-erosion and increase microplastic exposure of marine ecosystems in the water column and on the sea floor along the coastline.
The Norwegian coastline is over 100 000 km long, where the shoreline with uninhabited islands and inlets accumulates floating debris brought by the Norwegian Coastal Current and the predominant winds from the south-west (Bastesen et al. 2020). Models have shown how the Norwegian coastline is a trap for floating debris (Onink et al. 2019). Mammals, seabirds and fish that live in these coastal habitats are in this way exposed to a high volume of deposited and/or floating plastic debris despite the scarce human population. The coastline is also the site for coastal fisheries and hosts a high number of fish farms that use, lose and discard plastic equipment. Although the concentrations and distribution of MP along the Norwegian coastline are not yet fully mapped and understood, recent publications document the occurrence, levels and variation of MP in sediments and waters in urban areas (Gomiero et al., 2019b; Haave et al., 2019) and even on the Norwegian continental shelf (Jensen and Cramer, 2017). Ingestion and uptake of plastic fragments by a range of marine species have also been documented over the past decade (Browne et al., 2008; Andrady, 2011; Bravo Rebolledo et al., 2013; Lusher et al., 2013; Van Cauwenberghe and Janssen, 2014; Watts et al., 2014; Van Cauwenberghe et al., 2015; Brate et al., 2016; Lusher et al., 2016), and the implications are that humans are exposed through consumption of seafood (Andrady, 2011; Lusher et al., 2017). Although uptake and transfer of micro and nanoplastics through the food web have been proven experimentally (Mattsson et al., 2015; Mattsson et al., 2017) the environmental relevance of such transfer for ecosystem and human health at current concentrations is still poorly understood (Smith et al., 2018; Ribeiro et al., 2019). A knowledge base on the levels of MP contamination in edible tissues and the associated health risks in exposed animals and humans is needed in order to perform relevant Environmental Risk Assessments (ERA), now and with predicted future increases in plastic pollution and release.
By investigating wild animals living in a plastic polluted area we aimed to elucidate whether MP are present at quantifiable levels in tissues of exposed wildlife at current environmental levels. This will also add to knowledge about MP potentially reaching humans through dietary intake of wild caught species. This is a necessary step to evaluate relevant exposure scenarios and provide long- term risk assessment for human consumers. The aim of this study was 1) to investigate the occurrence and levels of MP in tissues of wild animals from a plastic polluted area, 2) to perform a qualitative assessment of health, investigating a standardized battery of health parameters 3) Assess the relation between microplastic analyses and observed health parameters
Materials and Methods
We investigated the concentrations of MP in tissues of fish, seabirds, terrestrial and marine mammals from a highly plastic polluted area on the west-coast of Norway, at the Sotra archipelago outside the city of Bergen (Figure 1). Preliminary studies done by NORCE in a regional project (RFFV #277264) found that the volumes of accumulated plastic debris at Sotra was approximately eight metric tons per kilometer (in 2018). The estimated global average has been estimated to a little over a metric ton per kilometer coastline (Smith and Markic, 2013; Ryan et al., 2014).
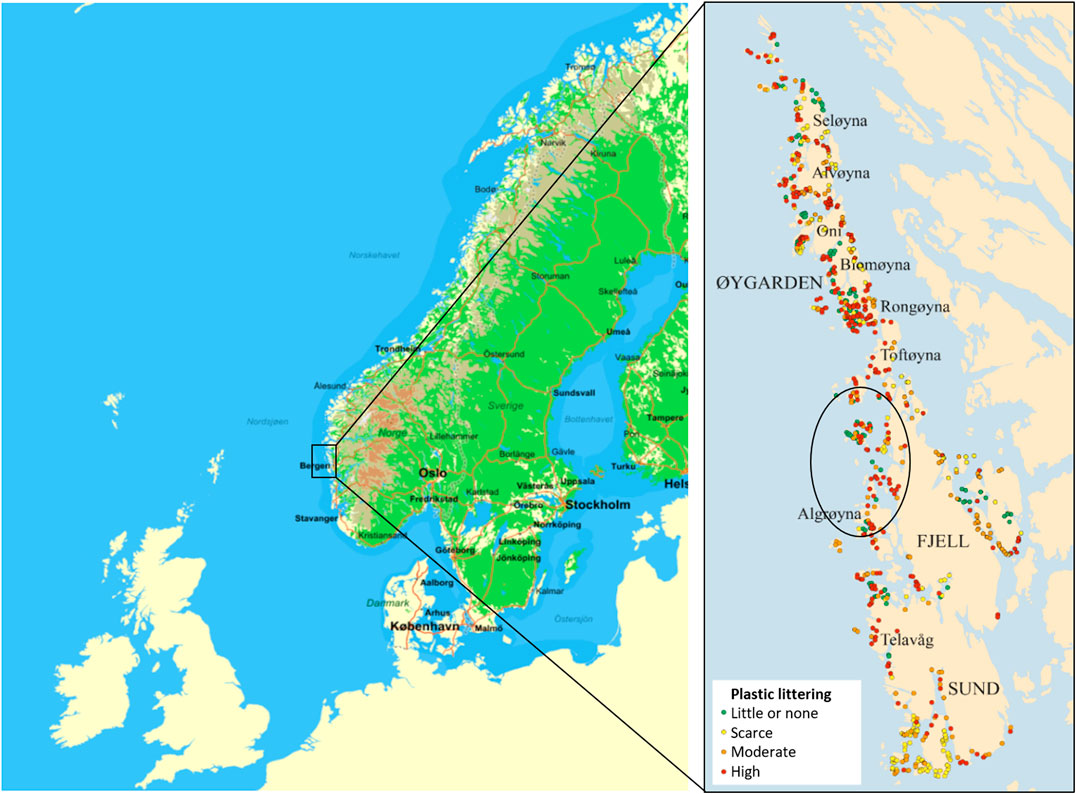
FIGURE 1. Map of Norway with the Sotra Achipelago to the West, and the plastic exposed area (circled) where the samples were caught. Map of plastic littered bays from Bastesen et al. (2020).
Investigated Wildlife
Fresh or frozen birds and mammals were donated to the study by local fishermen (Table 1). The samples were caught from December 25. 2017 to March 15. 2019. The animals were mainly taken as by-catch in fishing nets and crab-boxes. The animals obtained for investigation of both microplastic and health parameters were three otters (Lutra lutra), two sawbill ducks (red breasted merganser) (Mergus serrator) and one common guillemot (common murre; Uria aalge) that were delivered to the Norwegian Veterinary Institute (NVI) in Bergen. One Harbor seal (Phoca vitulina) was autopsied outdoors. Three cod (Gadus morhua) and three flounders (Limanda limanda) were caught specifically for the project by collaborating volunteers from the Norwegian Hunter and Anglers Association and delivered fresh to NVI. Another three otters were sampled for health parameters, but not included for chemical MP investigation. Five of the otters and one bird were frozen at −20°C for up to ten months before sampling, as not enough fresh animals were obtained during the duration of the project.
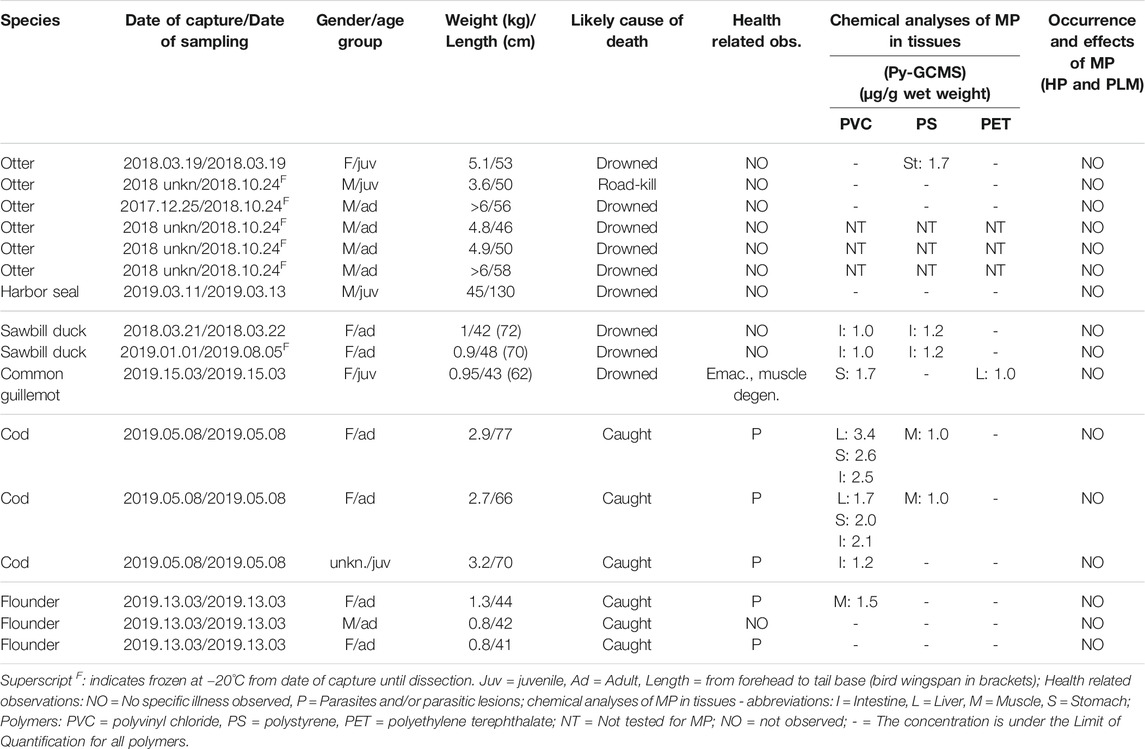
TABLE 1. Details of the sampled animals, results of the autopsies, general health, histopathological observations and chemical analyses of MP in tissues from wildlife from Sotra 2018–2019.
Gross and Histopathological Examination and Bacteriology
All animals were subject to autopsy according to guidelines at the NVI (http://kvalitet/eknet/docs/pub/dok01304.htm). In addition to a general gross pathological investigation of the animal, the gastrointestinal tract was examined for presence of large pieces of plastic materials. Tissue samples for histopathological examination from most organs including tissues with visible lesions were fixed in 10% neutral buffered formalin, embedded in paraffin and routinely processed into sections of 3–4 µm thickness (Supplementary Table S1). Tissues were stained with haematoxylin and eosin (H&E) following standard procedures and examined by light microscopy. Autopsy and histopathological examination were performed by trained pathologists.
Bacteriological examination for pathogens was performed according to procedures at the NVI. For all animals, samples from an internal organ (kidney or spleen) were sampled. From otters and birds, samples for bacteriology were also collected from lungs and intestines.
Polarization Microscopy for Microplastics in Tissues
All H&E stained histopathological sections (Supplementary Table S1) were examined by polarized light microscopy (PLM) in order to assess occurrence and localisation of possible plastic particles in the tissues. A Leica DM 2500 LED microscope with analyser and polarizer filter was used. This method may enlarge structures by 1000 x and allows the detection of very small birefringent particles including the size limit for the chemical analysis used in this study of 10 µm.
Tissue and Organ Sampling for Chemical Analysis
During autopsy, the stomach and intestinal contents were examined for visible macro plastics and blockage of the intestines. A chemical analysis aimed to detect presence of MP within tissues of internal organs was performed. Samples were taken for chemical analyses of the liver, stomach wall and intestines, as well as a piece of a large muscle from all the animals (Table 1). In mammals an internal muscle of the back (M. iliopsoas) was taken, in birds a cut from the thickest part of the large pectoral muscle (M. pectoralis major) and in fish a piece of the central dorsal fillet behind the dorsal fin was taken. The kidneys were also sampled in birds and mammals, the spleen in otters, and the gills of fish. From the harbor seal we also analyzed a piece of the lung. The stomachs were opened, and the intestines were emptied and inspected for large plastics that might influence the plastic uptake, health and wellbeing of the animals. The intestines were rinsed through with 50–100 ml saline using a small glass funnel. Samples were weighed, packed in aluminum foil and frozen at −20˚C until analysis.
Chemical Analysis of Microplastics in Tissues
The surfaces of gills and intestines were rinsed again before further analyses, to minimize the potential to have particles attached to the surface that might be considered to be within the tissues. A sample size of 6–30 g of tissue was used for chemical analyzes of MP, limited by availability of tissue and the efficiency of enzymatic degradation. Due to relevance as human food source, four replicate samples of muscle and two replicates of liver were analyzed from the two first cods. Budget constraints allowed only single replicates in the remaining samples.
Extraction of particles was based on previous published methods (Gomiero et al., 2019a). To extract microplastic particles (MP > 10 µm), samples were placed in microplastic free (burned at 500°C), 125 ml volume D4 borosilicate sintered glass filtration funnels (crucibles, with porosity 4, ROBU, VWR Cat.no 511-1322) and placed in a 600 ml glass beaker for support during treatment. The beaker was covered with aluminium foil to prevent airborne contamination. Samples were first incubated overnight (50°C) in 70 ml 5% SDS solution, then placed on a vacuum filtration assembly and the SDS gently vacuumed off. The residual material was rinsed with 30 ml Milli-Q water, 70 ml of protease (1:5) in glycine buffer was added, the sample sonicated and incubated for 36 h at 50°C. Digestates were then vacuumed off and residual material rinsed again with 30 ml of Milli-Q, incubated for 48 h at 30°C with 50 ml of lipase (Sigma, Germany) in PBS (1:5) at pH 7.4 , vacuumed off and rinsed with Milli-Q. A final strong oxidative digestion was performed using 50 ml hydrogen peroxide (H2O2, 30%, VWR International, Germany) at 50°C for 12 h. After removal of the H2O2, the residual material in the crucibles was quantitatively transferred into a in a separation funnel with zinc chloride solution (final density 1.70–1.75 g/cm3) by gently scratching the sintered glass filter surface. The mixture was stirred for 30 min before being left to settle for 72 h in pre cleaned separator funnels. The supernatant containing the floating plastic particles was collected by filtration on a glass fiber filter GF/A (Gomiero et al., 2019b) before packing into a pyrolytic tin cup. 10 ml of tetramethylammonium hydroxide (TMAH, 25% in water) was added to the tin cups pre-loaded with samples and allowed to dry at 40˚C prior to py-GCMS. Py-GCMS measurements were performed by a Shimadzu Optima 2010C GCMS controlled by GCMS solution V 4.45, equipped with a Rxi-5ms column (RESTEC, Bellefonte, PA) and coupled with Frontiers lab's Multi-Shot Pyrolizer EGA/PY-3030D with auto-shot sampler (BioNordika, Norway). Pyrolysis is performed at 590˚C, according to (Fischer and Scholz-Bottcher, 2017; Gomiero et al., 2019b) Eight of the most commonly used plastic polymers (polyethylene - PE, polypropylene - PP, polystyrene - PS, polyvinyl chloride - PVC, polyamide - PA, polymethyl methacrylate - PMMA, Polycarbonate - PC and polyethylene terephthalate-PET) of purity >99% were used to set up the calibration and quantification curves. In order to identify single polymers unambiguously in complex environmental samples, specific indicator compounds were chosen by pyrolyzing polymer standards. The obtained pyrograms were compared with a customized database and cross-checked with literature data following recommendations and selecting criteria from Fischer and Scholz-Bottcher (2017) and Gomiero et al. (2019b). To obtain calibration curves for quantification, standards between 10 and 360 mg of polymer were weighed directly into the pyrolysis tin cups using a XPE205 DeltaRange Mettler Toledo balance coupled with an Anti-Static Electricity Discharger tool (Sartorius, Germany). Individual polymers are identified by means of preselected combination of retention time and mass markers and quantified by integrating the chromatograms of their associated indicator ions. The limit of quantification (LOQ) was calculated according to Hermabessiere et al., (2018).
Contamination Control and Quality Assurance
To prevent contamination during and after sampling, the autopsies were performed on a steel bench using steel scalpels, scissors and plyers, placing the tissues on steel trays or aluminum foil, avoiding plastic materials as far as possible. Sterile containers and cups for formaldehyde fixation were of plastics, but these were not used for tissues for chemical analyses of microplastics. Surfaces in the dissection lab were wiped with tissue paper and water before start, and tissues were placed on pre-rinsed steel trays and clean tin foil for weighing and packaging. Nitrile gloves and semi-synthetic lab-coats were worn, and the lab did not have specialized air filtration, thus the surface of the samples were considered potentially contaminated after sampling. Before further chemical analysis in the dedicated MP lab at NORCE Stavanger, samples were rinsed with Milli-Q, and the surface layer of tissue was removed to avoid potentially contaminated surface layers of tissues. Removal of external layers was however not possible for delicate structures such as gills and intestines or stomachs. All liquid reagents for the chemical analyses, including Milli-Q water, were prefiltered over glass fiber filter (GF/A, 1.2 µm, Whatman). All glassware and equipment for the chemical analyses were heated to 550˚C in a muffle oven before use, wet traps for air-contamination and blank procedure controls for reagents were applied (Supplementary Table S2).
The LOQ for target polymers was as low as 1 µg/g wet weight (ww) for all the investigated polymers except PMMA that had a LOQ of 5 µg/g ww.
Data Treatment
SPSS v25 for Windows (IBM Statistics, United States) was used for statistical investigations and to create graphs.
Results
Animal Health and Condition–Pathological and Bacteriological Examination
Four of the animals were juveniles around the age of maturation, while the majority were adults. Most of the animals were in normal condition with food in their stomach. The birds and mammals were found dead, caught as bycatch in fishing nets and crab-boxes. The pathological investigation supported death by drowning for the birds and five of six otters (Table 1). One otter was found by the road, probably hit by a car. No bacteriological pathogens were isolated from the investigated animals. Details of size/weight, health status, likely cause of death, concentrations of microplastics and health observations can be seen in Table 1.
Mammals: One otter had multiple trauma with crushings and bleedings indicating that it was hit by a car. In five of six otters and the seal, findings were consistent with drowning, such as fluid, froth and congestion of the respiratory tract. No specific illnesses were detected. The young seal was in good condition but had nematodes in the lungs and stomach.
Birds: Gross pathology revealed circulatory disturbances of the respiratory tract indicated drowning. The sawbill ducks were in normal or mildly reduced condition. No specific diseases were observed. The guillemot had muscle degeneration of unknown cause and was emaciated.
Fish: Two cods and all three flounders were matured or in maturing. One matured cod was emaciated. In the cods and in one of the flounders we made observations common to adult fish, such as parasitic nodules in mesenterium and organs, some of them containing nematodes identified as Anisakis sp. One cod had a few small areas of chronic skin inflammation and one had a parasitic inflammation in an eye. Inflammation of gill and epicardium of unknown cause was seen in one flounder.
Ingestion of Non-food Items
No macroplastic items were observed in the stomachs or intestines of any mammal, fish or bird. Organic debris other than prey items, such as small rocks and sticks were observed in birds and fish stomachs.
Histological Examination for Microplastics and Tissue Effects
No particles or structures consistent with plastics were observed by either PLM or histopathology. In tissues positive for MP by the chemical analysis, no tissue reaction like thickening of intestinal epithelium, inflammation or necrosis that could be associated with MP were observed.
Polarization Microscopy
Polarization microscopy of the histological sections did not reveal particles or structures consistent with plastics.
Chemical Analysis for Microplastics
Analyses for microplastic particles of (theoretically) >10 µm (MP) were performed on tissues from five organs of each of the 13 animals, when organ sizes permitted both microplastic and histological analyses. Results are given in Table 1.
Microplastics in Animal Species
MP were not observed above LOQ in any tissue sample from the seal. MP was quantified in the stomach tissue sample of one of three otters (Table 1), while the other tissues from otters were negative. In seabirds, MP of the PVC and PS of the same concentrations were found in the intestines of the two sawbill ducks, while the guillemot had quantifiable levels of MP in the stomach (PVC) and liver (PET), but no MP in the intestinal tissue. The cods had the highest frequency and tissue concentrations of MP of all the animals (Figure 2), but cod was also the only species where replicate samples were analyzed. It is noteworthy that MP was only observed in muscle tissue from fish, not in birds or mammals, although this may have been influenced by the higher number of replicate analyses of cod tissues. Two of three cods had MP in all three tissues; intestines, liver and muscle. Among the flounders, MP was found in only one muscle sample, and in no other tissues (Table 1).
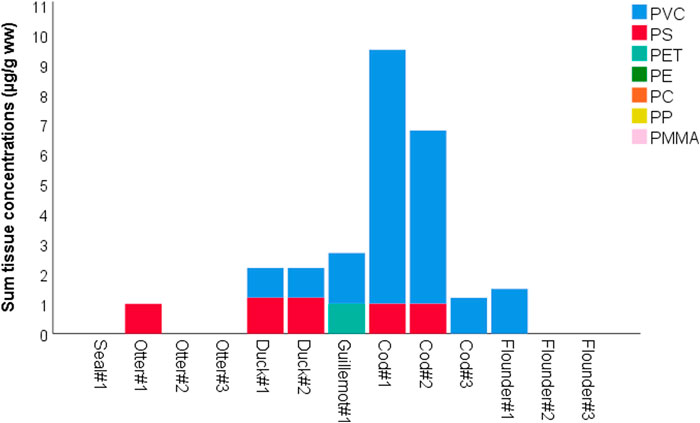
FIGURE 2. Sum of tissue concentrations (µg/g ww) of detected microplastic polymer types in coastal animals from Sotra in 2018–2019.
Microplastics in Different Tissues
Eight of the 13 investigated animals had quantifiable levels of MP in at least one of the tissue samples. Seven of the eight animals had MP in the stomach wall or intestines, while four different individuals had detectable levels of MP in muscles and/or liver (Table 1). In falling order, MP was most frequently found in tissue samples from intestines (5), stomachs (4), livers (3) and muscles (3). The highest single MP concentration found in any tissue was 3.4 µg/g wet weight (ww) found in cod liver (Table 1). No MP were found in samples of gill, lung, spleen or kidney after one replicate analysis of each tissue sample.
Polymer Types
Three of the nine investigated polymers were found in tissues: PVC was the most common polymer and was detected in seven animals in samples of intestine (5), stomach (3), liver (2) and muscle (1). PS was found in five animals, in intestines (2), muscle (2) and stomach (1). PET was found in one sample from the liver of a seabird, but not in any other samples or animals. All the polymers PE, PC, PP, PMMA, PA-66 were below the Limit of Quantification (LOQ) in all samples.
Blank Controls
Two of the 40 blank analyses from the extraction procedure room showed a measurable concentration of two the eight synthetic polymers (PE and PS) in the air contamination control in two separate weeks (Supplementary Table S2). The forty blank analyses of prefiltered reagents and the 40 blanks from the analysis room air controls showed no polymer contamination above the LOD (for liquid LOD, see Supplementary Table S3).
Discussion
General Observations and Tissue Distribution
This study focused on presence of microplastic in tissues of naturally exposed wild animals. Analyses of microplastics in stomach and intestinal contents and within ingested prey items were not within the scope of this work. The intestines are per definition external to the body, and MP in the intestinal lumen thus demonstrates ingestion, not uptake. Efficient egestion has been shown in several species (Frydkjaer et al., 2017; Ory et al., 2018; Woods et al., 2018), and the content of MP in stomach and intestines at any point in time is not necessarily representative for the long-term exposure, but shows the momentary status.
Microplastics Uptake and Transport
The findings of MP in internal organs above the background contamination suggests that translocation of MP to tissues of wildlife vertebrate species exposed in their natural habitats occurs. Uptake of MP after experimental exposure has previously been observed (Avio et al., 2015; Deng et al., 2017), but this is one of very few studies that have demonstrated MP uptake in vertebrates under natural exposure. Previous publications have shown MP in liver of anchovies (Collard et al., 2017), but to our knowledge this is the first study showing uptake of MP in tissues of wild birds. Previous studies indicate that the mode of uptake and translocation is through intestinal wall and transport of particles may occur via the portal system and chyle to the liver and other organs (Volkheimer, 1975; Volkheimer, 1993; Volkheimer, 2001) (Volkheimer, 1975). Transport was believed to happen also between cells, as peristaltic movement increased the uptake.
Uptake by the specialized epithelial M-cells of the gut associated lymphoid tissues like the Peyer’s patches has previously been discussed as a means of entry of MP in mammals for subsequent dissemination by lymph, blood and macrophages (Cannon and Swanson, 1992; Florence, 1997). Enterocytes with M-cell-like characteristics with capacity to absorb intact macromolecules are also reported in teleost fish (Fuglem et al., 2010). However, uptake by regular enterocytes is also emphasized (Carr et al., 2012), as well as entry between the enterocytes for larger particles and a spread from the digestive tract through the portal vein (Volkheimer, 1975; Volkheimer, 1993; Volkheimer, 2001). Hematogenous dissemination through the portal system would explain the presence of MP in liver, as the primary target for the blood from the digestive tract. Further hematogenous dissemination throughout the body is also likely, as MP was also detected in muscle of fish. Whether the secondary vascular system of fish might also play a role for such transport is, however, not known (Rummer et al., 2014).
Target Tissues
The highest single observed concentration of any polymer was found in a liver sample of cod (Table 1; Figure 3). The single observation is not evidence of liver as a target organ for plastic accumulation, however, it may seem that the liver is an important part of the process of uptake and/or excretion, as a high prevalence of MP in livers was also observed by Collard et al., (2017) studying European anchovies (Engraulis encrasicolus, L.). Another study of MP in wild and farmed salmon showed that MP was found in liver and muscle in equally high concentrations, and that muscle tissue may be just as suitable for monitoring as liver (Gomiero et al., 2020a). Another possible route of uptake and transfer to internal organs of fish could be via the gills, but no MP was found in the gill samples in our study. In recent studies we have observed PE particles in gill samples of farmed and wild salmon (Gomiero et al., 2020b).
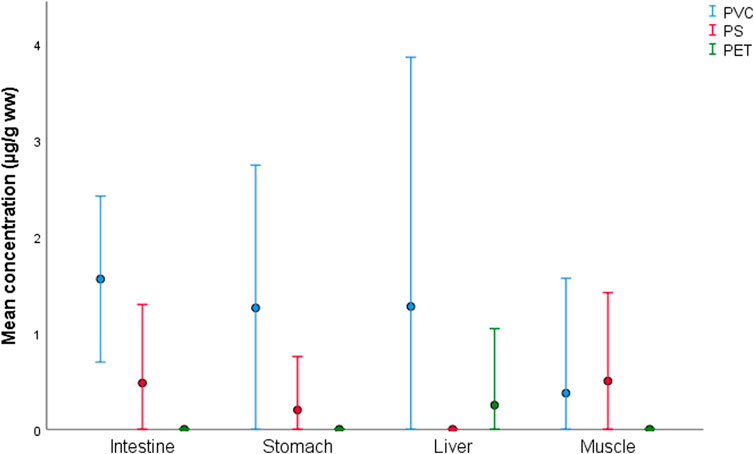
FIGURE 3. Mean and 95% confidence intervals of concentrations (µg/g ww) of microplastic polymers quantified in tissues of coastal animals from Sotra in 2018–2019.
Microplastic Localization Within Tissues
The observation of MP in samples of stomach and intestines has been observed by a number of previous studies (reviewed in Lusher et al., 2017). Although intestines were rinsed with saline during sampling, and all samples were rinsed again before enzymatic degradation, MP may still have been attached to the surface of the mucosal layer or trapped between folds and invaginations. For the liver and muscle, the findings indicate the presence of MP inside these organs and cannot be explained by potential surface contamination, as the surface layers were removed prior to enzymatic digestion. It is therefore not unlikely that the MP may also have been present within the tissue layers of the intestines. The study cannot dismiss the possibility that the observed MP is localized within blood or lymph vessels in the investigated tissues. The absence of MP in blanks and a range of other investigated tissues, such as spleen, kidney and lungs suggest that the method is able to discriminate between absence and presence of MP. Absence could also be explained by low concentrations (under the LOQ) or patchy distribution where a small sample of <30 g may have missed occasional MP in the tissues.
It was not within the scope of this study to investigate the localization of plastic particles within the tissue, or the mode of uptake and transport of MP through the tissues. Our results document, however, that transfer of MP over external barriers into tissues and between organs may occur for both fishes and birds under natural conditions, and that closer investigation of uptake and translocation are warranted.
Food Web-Transfer, Bioaccumulation and Biomagnification
Most of the investigated animals were adults, and there were a number of negative findings per species (Table 1). With only three specimen per species, the data is not sufficient to comment on potential bioaccumulation (uptake and retention of MP leading to increasing levels of MP from the environment to biota) or biomagnification (increase of MP with trophic level). The animals are mainly predators in the same ecosystem but are not directly linked themselves as prey/predators.
In the stomach of the sawbill ducks we observed only small fish (2–5 cm), although they can also take larger fishes. The bottom-dwelling flatfish eat polychaetes and benthic invertebrates. The cod is a generalist and an opportunist that takes a range of prey from the benthic sediments such as polychaetes, crabs and other crustaceans, but also take fish on the bottom and in the water column. Cod from western Norway have also been caught with large plastic items in their stomach. Documented in the media is a large plastic bottle (www.dagbladet.no/nyheter/jeg-fikk-vondt-av-a-oppdage-dette/69687244), and even a sex-toy (www.nrk.no/mr/torsk-hadde-dildo-i-magen-1.11663195). The cod, as a generalist is more likely to try ingesting anything that looks edible, including plastic. Such generalist feeding habits have previously been correlated with higher plastic ingestion (Silva et al. 2018). The coastal cod in this study is a resident type of the Atlantic cod, and individuals therefore live their entire life-cycle in the same region, and do not go on oceanic foraging trips, unlike the migrating stocks of Atlantic cod (“skrei”) that spend most of their life in the open Atlantic waters. With its opportunistic foraging and small home-range, the coastal cod may be a good candidate as a fish species for coastal monitoring of microplastics.
The seal and otters also move between the surface and deep waters to breath and hunt for a range of fish, mussels and crabs. Otters sleep and nest on land in inlets and sheltered areas where the shoreline is often heavily polluted with accumulated macro and mega-plastics. Otter dens have been observed under remnants of boat wrecks, and their trails are commonly seen between the masses of stranded debris that are abundant in this unpopulated area. It is obvious that otters, seals and birds are likely to have many daily encounters with floating and beached plastic debris in this region. It is therefore interesting to observe the low levels or absence of detectable microplastics in the mammals, both old and young, living surrounded by large and smaller plastic debris. It may be speculated that the mammals are able to discriminate plastic from prey and do not ingest as much plastic items as the heavily polluted habitat should imply. Otters sometimes eat only parts of their prey, and possibly avoid the GI tract, but smaller fish can be eaten whole. Eating the prey whole, which the seal does, would expose the mammals to MP in the GI-tract of the prey items, and therefore, potential intestinal uptake and transport into mammalian tissues as well. Although we did not observe MP in liver and muscle of the mammals, we cannot exclude that such uptake also occurs in mammals.
The distribution patterns and polymer types detected in the tissues were similar in individuals of the same species, such as the cods and sawbill ducks. Such coherent findings of similar polymers and concentrations in the same species, indicate that the observation represents a pattern of exposure and uptake and may suggest that similar sources of MP and similar feeding behavior and exposure routes is reflected in the tissue levels. Similarly, it has previously been reported that foraging behavior and different habitat use during different life stages influences the levels of MP fibers in the GI tract of three species of snooks (Centropomidae spp, (Silva et al. 2018; Ferreira et al. 2019) However, a higher number of specimen, and a higher number of positive findings are needed to elucidate the distribution patterns and range of MP within and between species to correlate to foraging behavior.
Good contamination controls with no observed PVC in the blank controls support that the findings represent actual presence of MP in tissues. We can however not say anything about the residence time of the MP in the tissues, and whether the MP is in the process of being excreted, or by which mechanisms uptake and excretion occurs. The results we observe of MP in tissues may well represent a snapshot of the situation, and may reflect MP in blood vessels running through the tissues. The observation is in itself not evidence of bioaccumulation or biomagnification. To elucidate bioaccumulation or biomagnification it is necessary to perform a study with larger sample volumes per tissue and/or several parallels per sample in order to determine the concentration of MP in muscle and tissues of individuals related to age, size and trophic level. The current study identifies the presence of MP in tissues and indicates strongly that such investigations are warranted. It is also necessary to perform controlled studies to elucidate the mechanisms of uptake, translocation, excretion, and the localization of MP within tissues, to ultimately understand the excretion and half-life of MP.
Observed Polymers in Samples of Wild Animals
The most prevalent polymer found in tissues was PVC, which is a versatile and much used polymer in anything from building materials, pipes and constructions to electronics and hygienic materials in health care. The polymer has a density of 1.38 kg/L, thus it is heavier than Atlantic Seawater (1.02–1.03 kg/L). PS was the other detected polymer type, which is used for clear plastics (packaging, cutlery) or colored and used for a range of utensils (toys, household items) as well as electronics and car parts. PS has a density of 1.03–1.06 kg/L. However, expanded Polystyrene (EPS) can frequently be found on the beaches of this area, with a density of only 10–30% that of water. EPS is much used as insulating fish-boxes and for buoyancy devices at sea. Exposure to PS in the form of EPS is therefore likely. PET was observed in only one sample. PET is often used for drinking bottles. PET is also in the polyester family, often used for textiles, and the most common synthetic textile produced. Data are lacking on the prevalence and distribution of plastic polymers in sediments and water along most of the Norwegian coastline, and only a few quantitative analyses of sediments have been performed in Western Norway (Gomiero et al., 2019b; Haave et al., 2019). PVC, PS and PET were observed in most of the sediment samples of these studies but were not dominant. However, polymer composition in sediments shows great variations (Haave et al., 2019), and one cannot generalize to occurrence of polymers based on two studies from different regions. The general polymer contamination of sediments in the study area has not yet been investigated. Mapping the occurrence and levels of microplastics along the coastline is necessary in order to understand potential wildlife exposure to MP.
Tissue Effect
The histopathological investigations did not reveal any tissue reaction that could be related to the presence of MP. Only low levels of MP, close to the Limit of Quantification were observed in this study, in contrast to high doses used in experimental studies that report histopathological reactions in zebrafish and mice respectively (Lu et al., 2016; Deng et al., 2017). However, histopathological studies that claim to observe effects have been criticized for poor quality (Baumann et al., 2016), or histopathology has revealed no tissue effect (Karami et al., 2017). This study is too small to conclude on the impact of MP on animal health, but the confirmation of MP in tissues of animals exposed under natural conditions is a strong indication that tissue uptake, residence and health effects of MP should be carefully followed in a long-term perspective.
It is considered plausible that MP uptake into tissues may have long term effects due to plastic-associated chemicals. For this study it is unlikely that we will be able to correlate such effects with the observable and momentary presence of MP in the tissue, in part due to low levels of MP and a too low number of samples to see correlations. Also, if the MP is not accumulating but is excreted after a short residence time in the tissue, attempting to demonstrate long-term effects based on a snapshot of momentary presence of MP is not advisable. Moreover, the mode of action for MP and the level of biological organization at which effects will appear (biochemical, cellular, tissue, organ, individual) are not yet properly elucidated. More research into the modes of action is needed to understand the long-term effects of MP in wildlife.
Method Limitations
Cost and Time for Extraction and Preparation
Analysis of MP by py-GCMS in biological samples demands complete removal of proteins and fats while maintaining the integrity of the plastic polymers, as remnants of organic material, proteins and fats will hamper the chemical characterization. Currently recommended methods (Loder et al., 2017; Gomiero et al., 2019b; Gomiero et al., 2020b) use gentle enzymatic digestion and oxidizing agents, which is time consuming and costly. This leads to small volumes of tissue used (≤30 g), resulting in high LOQs. Larger samples or a higher number of replicates would mean a better representation of the organ sampled, but require more time and reagents, increasing costs. The current enzymatic digestion and preparation thus makes the analyses time consuming and expensive and the high costs are an obstacle to perform large studies with a sufficiently high number of samples or replicates for a better resolution of the data. The low sensitivity and low number of samples with quantified microplastic concentrations in this study hampers statistical analyses.
Are Samples Representative?
In this study we performed replicate analysis of the cod muscle and livers, to investigate how representative a small sample is. Our results showed that concentrations of MP were above LOQ in one of four replicates of cod muscle and one of two parallel liver samples, performed in two cod. This means that small sample volumes and limitations of cost and time to single replicates in all the other samples may have led to false negatives.
Sensitivity
The LOQ for py-GCMS corresponds to the plastic mass of one spherical particle of about 10 µm in size. The 10 µm filters used theoretically limit the size to >10 µm, but smaller particles can also be trapped on the filters. In support of the suspected uneven MP distribution in tissues, no MP in any sample was observed by PLM. The finding of concentrations close to LOQ, at the same time as no observations were made of MP by PLM, may also indicate that MP are present as very small particles that may evade detection by PLM. The indications of a patchy distribution of MP also implies that a thin section of tissue is likely to miss the MPs when present at low concentrations, and that a higher number of samples would be needed to observe MP embedded in the tissues. High exposure concentrations have been used to investigate modes of uptake and excretion, and to determine target organs for MP (Volkheimer et al., 1968; Deng et al., 2017), and so far current environmental levels of microplastics are far from these high-exposure conditions.
Reliability, Quality Control
Neither PVC nor PET were observed in the control samples, whereas PS was observed in one control sample throughout the lab work (Supplementary Table S2). Evidence of polymers in tissues that were not present in the lab or in contamination controls, indicate that MP observed in tissues from internal organs is not contamination from the lab environment but represent actual uptake of PVC into wildlife.
Potential Human Exposure Through Food
This study is not sufficient to draw conclusions on human exposure through ingestion of wild caught fish or birds. To conclude on human exposure through ingestion of food, a larger sample size would be needed. The current study documents the presence of MP, but results indicate that positive findings are correlated with a higher number of replicate analyses, or a higher sample volume. Due to the indication of patchy distribution of MP in organs, it may, moreover, be inaccurate to extrapolate from the concentrations in a tissue sample to the entire organ. Thus, the concentrations cannot be considered representative of the average and the few replicates from cod fillet or cod liver are insufficient to draw conclusions on human exposure through consumption of these tissues. Presence in the alimentary tract is moreover not an indication of harm caused to the animal, but is an evidence of oral intake, indicating likely uptake of MP over the intestine.
Conclusion
This novel study demonstrates that low levels of MP in tissues of wild animals can be detected and quantified by current methods. This is to our knowledge the first study to demonstrate MP in tissues of birds exposed to relevant environmental concentrations of plastics and microplastics in their natural habitat.
Presence of MP does not document bioaccumulation or biomagnification but may represent recently absorbed particles in blood vessels, absorbed into tissues or in the process of being excreted. Observations of higher levels in the liver may suggest that the liver is involved in uptake or excretion of MP.
The concentrations in tissues are currently close to, and sometimes below the Limit of Quantification, and it is likely that there are several false negatives due to a limited number of replicates. The study, however, does not demonstrate that analytical methods are sufficiently sensitive to detect current levels of MP in wildlife, and that it is now possible to start investigating baseline levels of MP in environmentally exposed animals.
The contamination control shows low levels of contamination and no evidence of the most frequently observed polymer in tissues, PVC. The number of false positives is expected to be low, and we believe the methods are more likely to underestimate than over-estimate the concentrations.
Although the levels are currently low, the presence indicates uptake into biota and a potential transfer throughout the food web, including humans, through ingestion of muscle tissue or liver from wild fish and birds. The study does not permit conclusions at this stage on human exposure through wild caught food.
No detrimental effects of current levels of MP are observed. However, the current study, with a low number of positive findings of MP in tissues, is too small to conclude on detrimental or non-detrimental effects of MP.
Method development is needed to map MP distribution and potential accumulation in tissues in a cost-efficient manner. The sensitivity of current methods for MP quantification is not comparable to the analytical sensitivity for other environmental contaminants. It is expected that the sensitivity of the analyses will improve in the years to come, and that lower concentrations can be detected with small sample volumes. This will give highly desired data for potential human exposure through food. It is of the highest interest to elucidate the mechanisms of uptake, transport and excretion following realistic exposure levels in wildlife, and the following potential for human exposure through food. Data from a higher number of species, individuals and investigated areas is needed to provide relevant Environmental Risk Assessments for coastal regions.
This study documents MP throughout different tissues from several wild species exposed through their natural habitat. In combination with the evidence of patchiness of MP within tissues and likely false negatives, this warrants more studies with a higher number of species, individuals and replicates from several regions, highly plastic polluted or clean, to increase the understanding of current microplastic pollution in the coastal ecosystem and the potential harm to the ecosystem health and human exposure through edible tissues.
Data Availability Statement
The raw data supporting the conclusions of this article will be made available by the authors, without undue reservation.
Ethics Statement
Ethical review and approval was not required because the studies did not involve tests on live animals but analyzed tissues of dead animals accidentally caught as bycatch in fishing nets and delivered to us by local fishermen. The fish caught for analyses were caught by recreational fishermen and were lawful harvest of sustainable fish-stocks.
Author Contributions
MH, AG, AO, HN, and JS contributed conception and design of the study. MH had the main responsibility for application and project organization. MH, JS, AO, and HN performed the autopsies and sampling. AG developed the method and performed the chemical analyses for microplastics, JS and AO performed histopathology and pathogen analysis. MH organized the data, wrote the first draft of the manuscript. AO, JS, and AG wrote sections of the manuscript. All authors contributed to manuscript revision, read and approved the submitted version.
Funding
Funded by Plastreturs Miljøprosjekt. In-kind funding by The Norwegian Veterinary Institute, Norwegian Association of Hunters and Anglers and Norwegian Research Centre (NORCE).
Conflict of Interest
The authors declare that the research was conducted in the absence of any commercial or financial relationships that could be construed as a potential conflict of interest.
Acknowledgments
Thanks to Kenneth Bruvik and Rune Gaasø for essential contributions to the initialization and realization of the project, for delivery of bycatch of birds and mammals, and thanks to pupils from TAM for catching fish for the project. Thanks to involved personnel: Kjell-Birger Øysæd at NORCE PlastLab for assistance in the py-GCMS analyses, Eivind Bastesen for creating maps, and the technical staff at NVI and students at the University of Bergen for laboratory assistance during autopsies.
Supplementary Material
The Supplementary Material for this article can be found online at: https://www.frontiersin.org/articles/10.3389/fenvs.2021.575058/full#supplementary-material.
References
Andrady, A. L., Hamid, H. S., and Torikai, A. (2003). Effects of climate change and UV-B on materials. Photochem. Photobiol. Sci. 2 (1), 68–72. doi:10.1039/b211085g
Andrady, A. L. (2011). Microplastics in the marine environment. Mar. Pollut. Bull. 62 (8), 1596–1605. doi:10.1016/j.marpolbul.2011.05.030
Andrady, A. L. (2017). The plastic in microplastics: a review. Mar. Pollut. Bull. 119 (1), 12–22. doi:10.1016/j.marpolbul.2017.01.082
Avio, C. G., Gorbi, S., and Regoli, F. (2015). Experimental development of a new protocol for extraction and characterization of microplastics in fish tissues: first observations in commercial species from Adriatic Sea. Mar. Environ. Res. 111, 18–26. doi:10.1016/j.marenvres.2015.06.014
Barnes, D. K., Galgani, F., Thompson, R. C., and Barlaz, M. (2009). Accumulation and fragmentation of plastic debris in global environments. Philos. Trans. R. Soc. Lond. B, Biol. Sci. 364 (1526), 1985–1998. doi:10.1098/rstb.2008.0205
Bastesen, E., Haave, M. H. A., and Neby, S. (2020). Utvikling av kartleggingsmetoder og estimat for oppryddingskostnader for makroplast i strandsonen. NORCE report 4-2020, 9.
Baumann, L., Schmidt-Posthaus, H., Segner, H., and Wolf, J. C. (2016). Comment on “uptake and accumulation of polystyrene microplastics in zebrafish (Danio rerio) and toxic effects in liver”. Environ. Sci. Technol. 50 (22), 12521–12522. doi:10.1021/acs.est.6b04193
Bergmann, M., Mützel, S., Primpke, S., Tekman, M. B., Trachsel, J., and Gerdts, G. (2019). White and wonderful? Microplastics prevail in snow from the alps to the arctic. Sci. Adv. 5 (8), eaax1157. doi:10.1126/sciadv.aax1157
Bergmann, M., Wirzberger, V., Krumpen, T., Lorenz, C., Primpke, S., Tekman, M. B., et al. (2017). High quantities of microplastic in arctic deep-sea sediments from the HAUSGARTEN observatory. Environ. Sci. Technol. 51 (19), 11000–11010. doi:10.1021/acs.est.7b03331
Bråte, I. L. N., Eidsvoll, D. P., Steindal, C. C., and Thomas, K. V. (2016). Plastic ingestion by Atlantic cod (Gadus morhua) from the Norwegian coast. Mar. Pollut. Bull. 112 (1–2), 105–110. doi:10.1016/j.marpolbul.2016.08.034
Bravo Rebolledo, E. L., Van Franeker, J. A., Jansen, O. E., and Brasseur, S. M. (2013). Plastic ingestion by harbour seals (Phoca vitulina) in The Netherlands. Mar. Pollut. Bull. 67 (1–2), 200–202. doi:10.1016/j.marpolbul.2012.11.035
Browne, M. A., Dissanayake, A., Galloway, T. S., Lowe, D. M., and Thompson, R. C. (2008). Ingested microscopic plastic translocates to the circulatory system of the mussel, Mytilus edulis (L). Environ. Sci. Technol. 42 (13), 5026–5031. doi:10.1021/es800249a
Cannon, G. J., and Swanson, J. A. (1992). The macrophage capacity for phagocytosis. J. Cell Sci. 101 (Pt 4), 907–913.
Carr, K. E., Smyth, S. H., McCullough, M. T., Morris, J. F., and Moyes, S. M. (2012). Morphological aspects of interactions between microparticles and mammalian cells: intestinal uptake and onward movement. Prog. Histochem. Cytochem. 46 (4), 185–252. doi:10.1016/j.proghi.2011.11.001
Collard, F., Gilbert, B., Compère, P., Eppe, G., Das, K., Jauniaux, T., et al. (2017). Microplastics in livers of European anchovies (Engraulis encrasicolus, L). Environ. Pollut. 229, 1000–1005. doi:10.1016/j.envpol.2017.07.089
Courtene-Jones, W., Quinn, B., Gary, S. F., Mogg, A. O. M., and Narayanaswamy, B. E. (2017). Microplastic pollution identified in deep-sea water and ingested by benthic invertebrates in the Rockall Trough, North Atlantic Ocean. Environ. Pollut. 231 (Pt 1), 271–280. doi:10.1016/j.envpol.2017.08.026
Deng, Y., Zhang, Y., Lemos, B., and Ren, H. (2017). Tissue accumulation of microplastics in mice and biomarker responses suggest widespread health risks of exposure. Sci. Rep. 7, 46687. doi:10.1038/srep46687
Dris, R., Gasperi, J., Saad, M., Mirande, C., and Tassin, B. (2016). Synthetic fibers in atmospheric fallout: a source of microplastics in the environment? Mar. Pollut. Bull. 104 (1–2), 290–293. doi:10.1016/j.marpolbul.2016.01.006
Everaert, G., Van Cauwenberghe, L., De Rijcke, M., Koelmans, A. A., Mees, J., Vandegehuchte, M., et al. (2018). Risk assessment of microplastics in the ocean: modelling approach and first conclusions. Environ. Pollut. 242 (Pt B), 1930–1938. doi:10.1016/j.envpol.2018.07.069
Ferreira, G. V. B., Barletta, M., Lima, A. R. A., Morley, S. A., and Costa, M. F. (2019). Dynamics of marine debris ingestion by profitable fishes along the estuarine ecocline. Sci. Rep. 9 (1), 13514. doi:10.1038/s41598-019-49992-3
Fischer, M., and Scholz-Böttcher, B. M. (2017). Simultaneous trace identification and quantification of common types of microplastics in environmental samples by pyrolysis-gas chromatography-mass Spectrometry. Environ. Sci. Technol. 51 (9), 5052–5060. doi:10.1021/acs.est.6b06362
Florence, A. T. (1997). The oral absorption of micro- and nanoparticulates: neither exceptional nor unusual. Pharm. Res. 14 (3), 259–266. doi:10.1023/a:1012029517394
Frydkjaer, C. K., Iversen, N., and Roslev, P. (2017). Ingestion and egestion of microplastics by the cladoceran Daphnia magna: effects of regular and irregular shaped plastic and sorbed phenanthrene. Bull. Environ. Contam. Toxicol. 99 (6), 655–661. doi:10.1007/s00128-017-2186-3
Fuglem, B., Jirillo, E., Bjerkås, I., Kiyono, H., Nochi, T., Yuki, Y., et al. (2010). Antigen-sampling cells in the salmonid intestinal epithelium. Dev. Comp. Immunol. 34 (7), 768–774. doi:10.1016/j.dci.2010.02.007
Gomiero, A., Strafella, P., Øysæd, K. B., and Fabi, G. (2019a). First occurrence and composition assessment of microplastics in native mussels collected from coastal and offshore areas of the northern and central Adriatic Sea. Environ. Sci. Pollut. Res. Int. 26 (24), 24407–24416. doi:10.1007/s11356-019-05693-y
Gomiero, A., Øysæd, K. B., Agustsson, T., van Hoytema, N., van Thiel, T., and Grati, F. (2019b). First record of characterization, concentration and distribution of microplastics in coastal sediments of an urban fjord in south west Norway using a thermal degradation method. Chemosphere 227, 705–714. doi:10.1016/j.chemosphere.2019.04.096
Gomiero, A., Haave, M., Bjorøy, Ø., Herzke, D., Kögel, T., Nikiforov, V., et al. (2020a). Quantification of microplastic in fillet and organs of farmed and wild salmonids-a comparison of methods for detection and quantification (SALMODETECT). NORCE report 8-2020, 43.
Gomiero, A., Marte, H., Tanja, K., Ørjan, B., Mona, G., Trygve, B. L., et al. (2020b). Tracking of Plastic emissions from aquaculture industry (TrackPlast). NORCE report 4-2020 NORCE, 71.
Haave, M., Lorenz, C., Primpke, S., and Gerdts, G. (2019). Different stories told by small and large microplastics in sediment - first report of microplastic concentrations in an urban recipient in Norway. Mar. Pollut. Bull. 141, 501–513. doi:10.1016/j.marpolbul.2019.02.015
Hartmann, N. B., Hüffer, T., Thompson, R. C., Hassellöv, M., Verschoor, A., Daugaard, A. E., et al. (2019). Are we speaking the same language? Recommendations for a definition and categorization framework for plastic debris. Environ. Sci. Technol. 53 (3), 1039–1047. doi:10.1021/acs.est.8b05297
Hermabessiere, L., Himber, C., Boricaud, B., Kazour, M., Amara, R., Cassone, A. L., et al. (2018). Optimization, performance, and application of a pyrolysis-GC/MS method for the identification of microplastics. Anal Bioanal. Chem. 410 (25), 6663–6676. doi:10.1007/s00216-018-1279-0
Jambeck, J. R., Geyer, R., Wilcox, C., Siegler, T. R., Perryman, M., Andrady, A., et al. (2015). Marine pollution. Plastic waste inputs from land into the ocean. Science 347 (6223), 768–771. doi:10.1126/science.1260352
Jensen, H. K. B., and Cramer, J. (2017). MAREANOs pilotprosjekt på mikroplast- resultater og forslag til videre arbeid. NGU 2017.043.
Karami, A., Groman, D. B., Wilson, S. P., Ismail, P., and Neela, V. K. (2017). Biomarker responses in zebrafish (Danio rerio) larvae exposed to pristine low-density polyethylene fragments. Environ. Pollut. 223, 466–475. doi:10.1016/j.envpol.2017.01.047
Lebreton, L., and Andrady, A. (2019). Future scenarios of global plastic waste generation and disposal. Palgrave Commun. 5 (1), 6. doi:10.1057/s41599-018-0212-7
Lebreton, L. C. M., van der Zwet, J., Damsteeg, J. W., Slat, B., Andrady, A., and Reisser, J. (2017). River plastic emissions to the world's oceans. Nat. Commun. 8, 15611. doi:10.1038/ncomms15611
Löder, M. G. J., Imhof, H. K., Ladehoff, M., Löschel, L. A., Lorenz, C., Mintenig, S., et al. (2017). Enzymatic purification of microplastics in environmental samples. Environ. Sci. Technol. 51 (24), 14283–14292. doi:10.1021/acs.est.7b03055
Lu, Y., Zhang, Y., Deng, Y., Jiang, W., Zhao, Y., Geng, J., et al. (2016). Uptake and accumulation of polystyrene microplastics in Zebrafish (Danio rerio) and toxic effects in liver. Environ. Sci. Technol. 50 (7), 4054–4060. doi:10.1021/acs.est.6b00183
Lusher, A., Hollman, P., and Mendoza-Hill, J. (2017). Microplastics in fisheries and aquaculture- Status of knowledge on their occurrence and implications for aquatic organisms and food safety. FAO Fisheries and Aquaculture, Technical Paper 615(147).
Lusher, A. L., McHugh, M., and Thompson, R. C. (2013). Occurrence of microplastics in the gastrointestinal tract of pelagic and demersal fish from the English Channel. Mar. Pollut. Bull. 67 (1–2), 94–99. doi:10.1016/j.marpolbul.2012.11.028
Lusher, A. L., O'Donnell, C., Officer, R., and O'Connor, I. (2016). Microplastic interactions with North Atlantic mesopelagic fish. Ices J. Mar. Sci. 73 (4), 1214–1225. doi:10.1093/icesjms/fsv241
Mattsson, K., Ekvall, M. T., Hansson, L. A., Linse, S., Malmendal, A., and Cedervall, T. (2015). Altered behavior, physiology, and metabolism in fish exposed to polystyrene nanoparticles. Environ. Sci. Technol. 49 (1), 553–561. doi:10.1021/es5053655
Mattsson, K., Johnson, E. V., Malmendal, A., Linse, S., Hansson, L. A., and Cedervall, T. (2017). Brain damage and behavioural disorders in fish induced by plastic nanoparticles delivered through the food chain. Sci. Rep. 7 (1), 11452. doi:10.1038/s41598-017-10813-0
Munari, C., Infantini, V., Scoponi, M., Rastelli, E., Corinaldesi, C., and Mistri, M. (2017). Microplastics in the sediments of terra nova bay (ross sea, Antarctica). Mar. Pollut. Bull. 122 (1–2), 161–165. doi:10.1016/j.marpolbul.2017.06.039
Onink, V., Wichmann, D., Delandmeter, P., and van Sebille, E. (2019). The role of ekman currents, geostrophy, and Stokes drift in the accumulation of floating microplastic. J. Geophys. Res. Oceans 124 (3), 1474–1490. doi:10.1029/2018JC014547
Ory, N. C., Gallardo, C., Lenz, M., and Thiel, M. (2018). Capture, swallowing, and egestion of microplastics by a planktivorous juvenile fish. Environ. Pollut. 240, 566–573. doi:10.1016/j.envpol.2018.04.093
Ribeiro, F., O'Brien, J. W., Galloway, T., and Thomas, K. V. (2019). Accumulation and fate of nano- and micro-plastics and associated contaminants in organisms. Trac Trends Anal. Chem. 111, 139–147. doi:10.1016/j.trac.2018.12.010
Rummer, J. L., Wang, S., Steffensen, J. F., and Randall, D. J. (2014). Function and control of the fish secondary vascular system, a contrast to mammalian lymphatic systems. J. Exp. Biol. 217 (Pt 5), 751–757. doi:10.1242/jeb.086348
Ryan, P. G., Lamprecht, A., Swanepoel, D., and Moloney, C. L. (2014). The effect of fine-scale sampling frequency on estimates of beach litter accumulation. Mar. Pollut. Bull. 88 (1–2), 249–254. doi:10.1016/j.marpolbul.2014.08.036
SAPEA (2019). A scientific perspective on microplastics in nature and society. SAPEA Evid. Rev. Rep. 4, 173. doi:10.26356/microplastics
Silva, J. D. B., Barletta, M., Lima, A. R. A., and Ferreira, G. V. B. (2018). Use of resources and microplastic contamination throughout the life cycle of grunts (Haemulidae) in a tropical estuary. Environ. Pollut. 242: 1010–1021. doi:10.1016/j.envpol.2018.07.038
Skåre, J. U., Alexander, J., Haave, M., Jakubowicz, I., Knutsen, H. K., Lusher, A., et al. (2019). Microplastics; occurrence, levels and implications for environment and human health related to food. Scientific opinion of the Scientific Steering Committee of the Norwegian Scientific Committee for Food and Environment. Oslo, Norway: Norwegian Scientific Committee for Food and Environment (VKM), 16.
Smith, M., Love, D. C., Rochman, C. M., and Neff, R. A. (2018). Microplastics in seafood and the implications for human health. Curr. Environ. Health Rep. 5 (3), 375–386. doi:10.1007/s40572-018-0206-z
Smith, S. D. A., and Markic, A. (2013). Estimates of marine debris accumulation on beaches are strongly affected by the temporal scale of sampling. PLoS One 8 (12), e83694. doi:10.1371/journal.pone.0083694
Urbanek, A. K., Rymowicz, W., and Mirończuk, A. M. (2018). Degradation of plastics and plastic-degrading bacteria in cold marine habitats. Appl. Microbiol. Biotechnol. 102 (18), 7669–7678. doi:10.1007/s00253-018-9195-y
Van Cauwenberghe, L., Claessens, M., Vandegehuchte, M. B., and Janssen, C. R. (2015). Microplastics are taken up by mussels (Mytilus edulis) and lugworms (Arenicola marina) living in natural habitats. Environ. Pollut. 199, 10–17. doi:10.1016/j.envpol.2015.01.008
Van Cauwenberghe, L., and Janssen, C. R. (2014). Microplastics in bivalves cultured for human consumption. Environ. Pollut. 193, 65–70. doi:10.1016/j.envpol.2014.06.010
Volkheimer, G., Schulz, F. H., Lehmann, H., Aurich, I., Hübner, R., Hübner, M., et al. (1968). Primary portal transport of persorbed starch granules from the intestinal wall. Med. Exp. Int. J. Exp. Med. 18 (2), 103–108. doi:10.1159/000137143
Volkheimer, G. (1975). Hematogenous dissemination of ingested polyvinyl chloride particles. Ann. N. Y Acad. Sci. 246, 164–171. doi:10.1111/j.1749-6632.1975.tb51092.x
Volkheimer, G. (2001). The phenomenon of persorption: persorption, dissemination, and elimination of microparticles. in Old Herborn University Seminar Monograph. Herborn: Herborn Litterae, 14, 7–18.
Watts, A. J., Lewis, C., Goodhead, R. M., Beckett, S. J., Moger, J., Tyler, C. R., et al. (2014). Uptake and retention of microplastics by the shore crab Carcinus maenas. Environ. Sci. Technol. 48 (15), 8823–8830. doi:10.1021/es501090e
Woodall, L. C., Sanchez-Vidal, A., Canals, M., Paterson, G. L., Coppock, R., Sleight, V., et al. (2014). The deep sea is a major sink for microplastic debris. R. Soc. Open Sci. 1 (4), 140317. doi:10.1098/rsos.140317
Woods, M. N., Stack, M. E., Fields, D. M., Shaw, S. D., and Matrai, P. A. (2018). Microplastic fiber uptake, ingestion, and egestion rates in the blue mussel (Mytilus edulis). Mar. Pollut. Bull. 137, 638–645. doi:10.1016/j.marpolbul.2018.10.061
Keywords: microplastics, tissue, uptake, wildlife, food web, polarization microscopy, histopathology
Citation: Haave M, Gomiero A, Schönheit J, Nilsen H and Olsen AB (2021) Documentation of Microplastics in Tissues of Wild Coastal Animals. Front. Environ. Sci. 9:575058. doi: 10.3389/fenvs.2021.575058
Received: 22 June 2020; Accepted: 25 January 2021;
Published: 15 March 2021.
Edited by:
Andrew Turner, University of Plymouth, United KingdomReviewed by:
Sutapa Ghosal, California Department of Public Health, United StatesMario Barletta, Independent researcher, Recife, Brazil
Copyright © 2021 Haave, Gomiero, Schönheit, Nilsen and Olsen. This is an open-access article distributed under the terms of the Creative Commons Attribution License (CC BY). The use, distribution or reproduction in other forums is permitted, provided the original author(s) and the copyright owner(s) are credited and that the original publication in this journal is cited, in accordance with accepted academic practice. No use, distribution or reproduction is permitted which does not comply with these terms.
*Correspondence: Marte Haave, bWFydGUuaGFhdmVAbm9yY2VyZXNlYXJjaC5ubw==