Spatio-Temporal Variability of Peat CH4 and N2O Fluxes and Their Contribution to Peat GHG Budgets in Indonesian Forests and Oil Palm Plantations
- 1Department of Environmental Sciences, University of Virginia, Charlottesville, VA, United States
- 2Center for International Forestry Research, Bogor, Indonesia
- 3International Center for Tropical Agriculture, Cali, Colombia
- 4Yayasan Konservasi Alam Nusantara, Jakarta, Indonesia
Land-use change in tropical peatlands substantially impacts peat emissions of methane (CH4) and nitrous oxide (N2O) in addition to emissions of carbon dioxide (CO2). However, assessments of full peat greenhouse gas (GHG) budgets are scarce and CH4 and N2O contributions remain highly uncertain. The objective of our research was to assess changes in peat GHG flux and budget associated with peat swamp forest disturbance and conversion to oil palm plantation and to evaluate drivers of variation in trace gas fluxes. Over a period of one and a half year, we monitored monthly CH4 and N2O fluxes together with environmental variables in three undrained peat swamp forests and three oil palm plantations on peat in Central Kalimantan. The forests included two primary forests and one 30-year-old secondary forest. We calculated the peat GHG budget in both ecosystems using soil respiration and litterfall rates measured concurrently with CH4 and N2O fluxes, site-specific soil respiration partitioning ratios, and literature-based values of root inputs and dissolved organic carbon export. Peat CH4 fluxes (kg CH4 ha−1 year−1) were insignificant in oil palm (0.3 ± 0.4) while emissions in forest were high (14.0 ± 2.8), and larger in wet than in dry months. N2O emissions (kg N2O ha−1 year−1) were highly variable spatially and temporally and similar across land-uses (5.0 ± 3.9 and 5.2 ± 3.7 in oil palm and forest). Temporal variation of CH4 was controlled by water table level and soil water-filled pore space in forest and oil palm, respectively. Monthly fluctuations of N2O were linked to water table level in forest. The peat GHG budget (Mg CO2 equivalent ha−1 year−1) in oil palm (31.7 ± 8.6) was nearly eight times the budget in forest (4.0 ± 4.8) owing mainly to decreased peat C inputs and increased peat C outputs. The GHG budget was also ten times higher in the secondary forest (10.2 ± 4.5) than in the primary forests (0.9 ± 3.9) on the account of a larger peat C budget and N2O emission rate. In oil palm 96% of emissions were released as CO2 whereas in forest CH4 and N2O together contributed 65% to the budget. Our study highlights the disastrous atmospheric impact associated with forest degradation and conversion to oil palm in tropical peatlands and stresses the need to investigate GHG fluxes in disturbed undrained lands.
Introduction
Peat swamp forests of Southeast Asia have experienced extensive deforestation and conversion over past decades. In Peninsular Malaysia, Sumatra and Borneo, only 29% of peatlands (or 4.6 Mha) remained covered by forests in 2015 while lands managed by smallholders or industrial groups amounted to 50% of peatlands (7.8 Mha) (Miettinen et al., 2016). Conversion of peat swamp forest, in particular to oil palm, is a large and growing source of greenhouse gas (GHG) emissions to the atmosphere (Koh et al., 2011; Miettinen et al., 2017; Austin et al., 2018). Efforts to characterize the GHG impact of this conversion have largely focused on alterations of soil CO2 emissions and phytomass C stocks (e.g., Hergoualc’h and Verchot, 2011; Miettinen et al., 2017). Changes in emissions of CH4 and N2O from peat can also be significant (Hergoualc’h and Verchot, 2012; Oktarita et al., 2017; Cooper et al., 2020) but have been far less well characterized (Skiba et al., 2020), despite N2O and CH4 being potent GHGs, with global warming potentials, respectively 268 and 86 times that of CO2 over a 20-year time horizon (Myhre et al., 2013). Reliable estimates of N2O emissions from degraded peatlands are lacking (Tian et al., 2020). Flux studies on peatlands in paired forest-oil palm plantation replicated sites are very scarce, with only one study to our knowledge (Cooper al., 2020). Some of the studies (regardless of sites replication and forest-oil palm pairing) were conducted over very limited periods [e.g., 3 months in Cooper al. (2020)], and many do not stratify the sampling according to microspatial differences affecting the fluxes (e.g., Melling et al., 2007). None measured simultaneously all peat C fluxes for assessing peat net CO2 and GHG exchanges. Full peat GHG budgets that include net CO2, CH4, and N2O fluxes and concurrent measurements of controlling factors in these ecosystems are thus critically lacking and needed to quantify the impact of forest-to-oil palm conversion on peat GHG emissions and to increase understanding of underlying mechanisms.
Undrained peat soils in the tropics are typically a net source of CH4, while drained peat soils can act as either a source or a small sink (Hergoualc’h and Verchot, 2012). CH4 is produced by methanogens through anaerobic digestion of organic matter either by CO2 reduction or by acetate fermentation (Le Mer and Roger, 2001). Methanotrophs consume CH4 produced in the soil as well as atmospheric CH4 for use as a C and energy source (King et al., 1990; Whalen et al., 1990; Jones and Nedwell, 1993). CH4 production and consumption occur simultaneously in the soil profile. General controls on wetland CH4 emissions include hydrology, temperature, and vegetation (Wright et al., 2013; Turetsky et al., 2014). The activity of methanotrophs is mainly limited by oxygen availability (Le Mer and Roger 2001) therefore drainage promotes methanotrophy while decreasing methanogenesis (Inubushi et al., 2003). The influence of hydrological factors affecting the fluxes result in strong spatio-temporal variability. In Indonesian peatlands under pristine conditions, soil CH4 fluxes have been reported to be highest during wet months when the water table level is close to the peat surface (Jauhiainen et al., 2005) but to decrease with flooding above a water table level threshold because of gas diffusion restriction (Ishikura et al., 2019). CH4 fluxes are also influenced by soil microtopography and were reported to be higher from waterlogged low-lying hollows than drier elevated hummocks in pristine peat forests of Indonesia and Peru (Jauhiainen et al., 2005; Ishikura et al., 2019; Hergoualc’h et al., 2020). Methanogenesis is thought to be more temperature-sensitive than methanotrophy (Turetsky et al., 2014) though in the tropics where temperature fluctuation is small, this factor is considered a less important control on CH4 fluxes than other variables (Wright et al., 2013). Methanogenesis is often fueled by recent plant photosynthate (Bridgham et al., 2012). Therefore, CH4 fluxes are altered following land use conversion as the result of vegetation changes and associated substrate supply modifications in addition to drainage. Fires that expose deeper peat layers also alter the substrate supply for methanogenesis (Jauhiainen et al., 2016).
Both undrained and drained peat soils in the tropics function as a source of N2O (Drösler et al., 2014; Parn et al., 2018). N2O is generated mainly by soil microbes through nitrification and denitrification. Nitrification is the aerobic oxidation of NH4+ or NH3 to NO2− and NO3− with N2O formed as a biproduct (Hynes and Knowles, 1984). Denitrifying bacteria reduce NO3− under oxygen (O2) limited conditions ultimately producing N2 but also N2O, with a N2O:N2 ratio depending on O2 availability. Coupling of nitrification and denitrification can occur in soils where oxic and anoxic microenvironments neighbor each other (Arah, 1997). Nitrifier denitrication, distinct from coupled nitrification—denitrification, can also be an important source of N2O (Wrage et al., 2001; Hergoualc’h et al., 2007). N2O production in soils depends upon substrate supply from mineralized organic matter, applied N fertilizer, or deposited atmospheric N as well as biotic factors such as plant-microbe interactions and abiotic factors including, e.g., soil moisture and temperature (Skiba and Smith, 2000; Butterbach-Bahl et al., 2013). Soil N2O emissions often correlate well with the soil water-filled pore space (WFPS), with emission rates in the tropics peaking around a WFPS of 60% and remaining high at 80% WFPS (Van Lent et al., 2015). Nitrous oxide fluxes from peat soils are governed by factors that limit the microbial processes of nitrification and denitrification, especially variations in water table level and soil inorganic N (Martikainen et al., 1993; Melling et al., 2007; Oktarita et al., 2017). Soil-atmosphere exchange of N2O may be highly spatially variable because of inter-site as well as micro-scale and seasonal variations (Takakai et al., 2006; Jauhiainen et al., 2012) in environmental conditions. Peat N2O fluxes in the tropics are characterized by the presence of temporal and spatial emission hotspots under natural and drained conditions (Takakai et al., 2006; Jauhiainen et al., 2012; Oktarita et al., 2017; Hergoualc’h et al., 2020) owing to the fine scales over which controlling factors vary. Peat N2O fluxes in Indonesian peatlands have been found to be higher during the rainy season than during the dry season (Takakai et al., 2006; Jauhiainen et al., 2012) but seasonal variation in emission is inconsistent across land uses within a peatland area and between peatland areas of Southeast Asia. In oil palm plantation, the response of N2O emissions to fertilization has been reported to be exponential but restricted to the small area of N application and transient (Sakata et al., 2015; Oktarita et al., 2017). As a result, the impact of fertilized-induced emissions has been observed to be minimal, with annual emissions stemming essentially from peat decomposition (Oktarita et al., 2017).
Peat GHG budgets are estimated from the contribution of individual gases (CH4, N2O, CO2) based on their respective global warming potential. Annual peat CO2 budgets include on- and off-site CO2 emissions/removals from soil organic matter mineralization, sequestration processes and leaching (Drösler et al., 2014). They are calculated as the balance between C inputs from above and below ground litter, and outputs via heterotrophic respiration and dissolved organic C export. Total respiration consists of root (autotrophic) and microbial (heterotrophic) respiration, with only the latter contributing actively to soil C stock changes. Degradation and drainage enhance CO2 production from microbial decomposition (Hergoualc’h and Verchot 2014) and losses of dissolved carbon in water (Rixen et al., 2016). Forest replacement by oil palms decreases aboveground litter production but enhances inputs of fresh organic material from dead roots and root exudates (Hergoualc’h and Verchot, 2014; Harianti et al., 2017). Peat soils are small sinks or sources of CO2 in pristine swamp forests but strong sources in oil palm plantations, with loss rates varying greatly depending on pre-conversion land-use history and post-conversion plantation age and management practices (Hergoualc’h and Verchot, 2014; Hergoualc’h et al., 2017).
To improve current understanding on GHG dynamics associated with peat swamp forest degradation and conversion to oil palm plantation, we conducted a 19-month study in a peatland in Central Kalimantan, Indonesia. We measured monthly CH4 and N2O fluxes from soils along with environmental factors in three undrained forest plots (two primary forests and one secondary forest) and three oil palm plantation plots managed by smallholders. Our measurements covered wet to dry transitions in a year with normal precipitation (2014) and a strong El Niño year (2015). Intra-plot spatial variability was captured by sampling in hummocks and hollows in forest, and near and far from palms in plantations. Total soil respiration and litterfall rates measured concurrently with CH4 and N2O fluxes provided for peat CO2 budgets computation using site-specific soil respiration partitioning ratios and literature-based values for root litter inputs and dissolved organic C losses. Our study addressed five questions: 1) How do peat CH4 and N2O emissions differ between and within oil palm plantation and forest? 2) How do they vary on seasonal timescales? 3) How do environmental parameters (moisture, temperature, peat chemistry) control emission rates of these gases? 4) How do peat GHG budgets differ between oil palm plantation and forest and between forest types? And 5) how important is the contribution of CH4 and N2O fluxes to peat GHG budgets in these ecosystems?
Materials and Methods
Site Description
We conducted our research at permanent plots in a peatland on the southern coast of Indonesian Borneo in Central Kalimantan, approximately 10 km outside the city of Pangkalan Bun (S 02°49,410′, E 111°48.785′). The plots were established in 2012 by the Center for International Forestry Research at three locations in undrained forest inside Tanjung Puting National Park and at three locations in nearby drained smallholder oil palm plantations.
The regional climate is humid tropical. Total annual precipitation is high and average daily temperature remains fairly constant during the year. During 2004–2014 mean annual rainfall in Pangkalan Bun was 2,058 mm and August was, on average, the driest month (105 mm) (National Oceanic and Atmospheric Administration’s Climate Data Center). Mean annual temperature over this period was 26.6°C. Mean monthly temperature ranged from 26.1°C in July to 27.2°C in May.
The plots comprised a range of peat depths, land-use history and vegetation age (Table 1). The three forest plots were located at different distances from the edge of the main channel of the Sekonyer River. Peat depth varied in forest plots from less than 50 cm at the plot closest to the river to almost 3 m at the plot located farthest from the river. The plot closest to the river (FOR-1) was a 30-year-old secondary forest, managed as an agroforestry garden before Tanjung Puting National Park was established in 1982 and communities were moved across the Sekonyer river (Novita et al., 2020; according to interviews with community members). FOR-1 vegetation was dominated by pioneer species such as Macaranga motleyana (Euophorbiaceae), Buchanania sessifolia (Anacardiaceae), Baccaurea stipulate (Phyllanthaceae), and Litsea firma (Lauraceae) (Novita et al., 2020). The other two forest plots (FOR-2, FOR-3) were primary forests dominated by Vatica oblongifolia (Dipterocarpaceae) and Santiria apiculate (Burseraceae) (Novita et al., 2020). Our oil palm plots were nucleus estate smallholder plantations, an important part of the palm oil industry in Indonesia. Smallholders manage 40% of total oil palm area in Indonesia (DJP, 2015) and accounted for roughly a third of national production in 2011 (Obidzinski et al., 2012). We use the term “plantation” to signify differences in palm age. Oil palm plot locations were selected to represent a range of vegetation ages, as well as peat depth (Table 1) and management practices. Our oil palm plots included both young (palm age ≤ 3 years) and mature (palm age > 3 years) palms (Corley and Tinker, 2003). Smallholders started planting their lands with oil palm in the late 2000s following the establishment of a large oil palm plantation adjacent to their properties in the late 1990s. Oil palm was planted in 2007 (OP-2007) and 2009 (OP-2009) on land cleared in 2005. Oil palm was planted in 2011 (OP-2011) on land previously cleared in 1989 and managed for rice and vegetable production.

TABLE 1. Characteristics of the sampling plots in Central Kalimantan, Indonesia (after Swails et al., 2018).
Smallholders applied fertilizers approximately every 3 months. Annual rates of fertilizer application were 150-84-124 kg N-P-K ha−1 year−1 in the youngest plantation (OP-2011) decreasing to 120-67-100 kg N-P-K ha−1 year−1 in the oldest plantation (OP-2007). Fertilization rate in OP-2009 was not provided by the smallholder. Information on fertilization dates in the plantations is not available. Land use history and land management practices in smallholder oil palm plantation plots are further described by Swails et al. (2019).
Sampling Regime and Laboratory Analysis
Sampling design is described in Figure 1. We designed our sampling approach to capture temporal and spatial heterogeneity in environmental conditions and trace gas fluxes from soils. We put emphasis on characterizing monthly variation which is known to be much larger than diel variation (e.g., Günther et al., 2014). Also, previous work on the research site and elsewhere in Indonesian peatlands found insignificant diel variation in GHG fluxes (Comeau et al., 2016; Novita, 2016). We collected measurements once per month from January 2014 until June 2015 and once more in September 2015. Plots were sampled on consecutive days between the hours of 08:00 and 12:00. Monthly monitoring over 12–24 months is considered standard to capture the temporal variability of emissions (Inubushi et al., 2003; Furukawa et al., 2005; Jauhiainen et al., 2008; Hergoualc’h et al., 2020). Fluxes were not intensively monitored during post-fertilization periods in the oil palm plantations, thus measured N2O fluxes represent emissions from peat decomposition. Fertilizer-induced N2O emissions were found to be minor (9% of total N2O emissions) in plantations on peat fertilized at 100-200 kg N ha−1 year−1 (Oktarita et al., 2017).
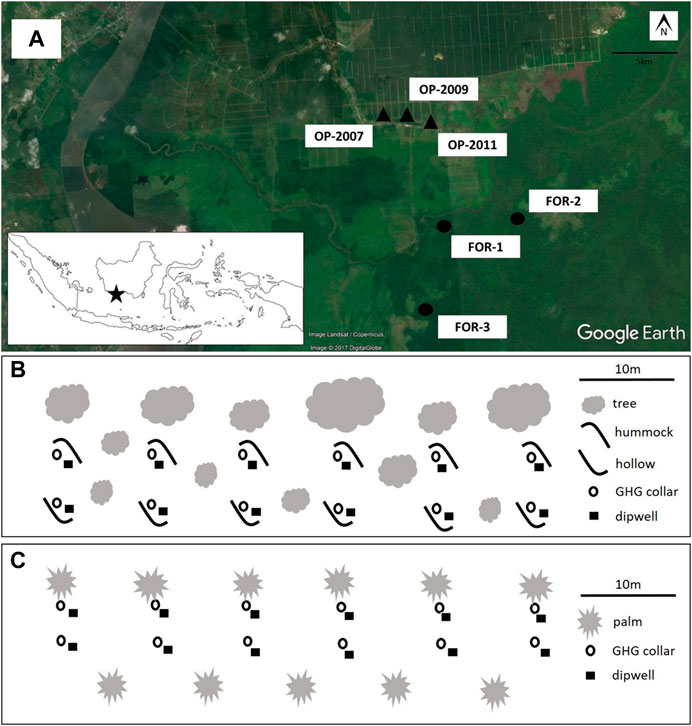
FIGURE 1. Research site and sampling design. Measurements were collected from a peatland on the island of Kalimantan (inset) in three plots in undrained forest (FOR-1, FOR-2, FOR-3) and three plots in nearby smallholder oil palm plantations (OP-2007, OP-2009, OP-2011) (A). In each plot, GHG collars (circles) and dipwells (squares) were installed at six subplot locations in oil palm (B) and forest (C). At each subplot, one collar and dipwell set was installed at the base of a palm and another at a distance of 3 m from the palm in oil palm plots. In forest plots, we installed one set on a hummock and one set in the adjacent hollow (after Swails et al., 2019).
Rates of CH4 and N2O fluxes were determined by the static chamber method (Hutchinson and Livingston, 1993; Verchot et al., 1999). Chambers were PVC collars (inner diameter 25 cm and height approximately 30 cm) permanently installed 16 months before the beginning of this study and equipped with opaque portable PVC hoods. A pair of collars was installed at six subplots for a total of 12 collars per plot (Figure 1). In oil palm plots, we installed at each subplot location one collar near the base of a palm under the palm canopy and one collar at mid-distance between two palms. In peat swamp forest, the microtopography was characterized by alternating raised mounds (hummocks) and depressions (hollows). In forest plots, at each subplot location we installed one collar on a hummock and one collar in the adjacent hollow. Samples were taken at 0, 10, 20, and 30 min after closing the chamber with the hood. We collected gas samples in 40 ml pre-evacuated glass vials using a syringe connected to the chamber hood outlet with silicone tubing. Vials were fitted with stopcocks sealed with polycarbonate caps to prevent gas leakage during transportation from the field.
Total soil respiration, soil temperature, air temperature, water table depth, and soil moisture (water-filled pore space) were monitored concurrently with trace gas fluxes, as described by Swails et al. (2019). Total soil respiration was measured by the dynamic closed chamber method (Pumpanen et al., 2009) with a portable infrared gas analyzer/EGM-4 (Environmental Gas Monitor) connected to a Soil Respiration Chamber (SRC-1) (PP System, Amesburry, United States) placed on a permanently installed PVC collar. We measured water table level in dipwells installed permanently next to each collar. The height difference between hummocks and hollows in forest was not measured. We report water table level relative to the soil surface on hummocks and hollows. Soil temperature to 5 cm was recorded using a soil temperature probe (Reotemp Digital TM99-A, United States). We used a pocket humidity/temperature pen (EXTECH 44550) to measure air temperature. Samples for water-filled pore space (WFPS) determination were collected bimonthly from the peat surface using stainless steel rings (height 5 cm and diameter 8 cm). We took six samples per plot, away from collars, three from hummock/near representative conditions and three from hollow/far representative conditions. Soil sampling locations for determination of WFPS were separated by approximately 20 m within plots. Samples were weighed in the field, transported in plastic bags to the laboratory, and oven-dried to constant mass at 60°C (Warren et al., 2012; Farmer et al., 2014). The gas samples were analyzed using a Shimadzu gas chromatograph (GC) equipped with an electron capture detector (ECD) for N2O, and a flame ionization detector (FID) for CH4.
Laboratory incubations were carried out to measure the rates of net mineralization and net nitrification. Soil samples were collected to a 5 cm depth in each plot in October 2013 and November 2013, outside of any fertilization event. The samples were collected adjacent to each chamber using a metal ring with a 277 cm3 internal volume. In October 2013 we took three samples from hummocks/area near palms and three samples from hollows/area mid-distance between palms, and in November 2013 we took six from each spatial position. The November 2013 soil samples were taken 10 m from each other, and the October 2013 soil samples were taken 20 m from each other. Samples were transported to the laboratory and refrigerated at 4 C until incubation. Coarse roots and litter were manually removed from the samples before incubation. We followed the procedure by Hart et al. (1994) to determine net mineralization and net nitrification rates. For each sample a 10 g soil subsample was extracted in 100 ml of 2M KCl to determine inorganic N concentrations. These extracts were shaken for an hour with a rotary shaker and allowed to settle for 24 h. A 20-ml aliquot of the supernatant was filtered (Whatmann filter paper no. 42) and frozen for later analysis. NH4+ content was analyzed on an auto analyzer (Bran LuebbeTM) using the colorimetric method with indophenol blue (Solorzano, 1969). Determination of NO3− was done on a U-2001 spectrophotometer (Hitachi) using the brucine procedure (EPA, 1971). A second subsample of 10 g was incubated in the dark at room temperature (25–28°C) for 7 days. After 7 days, the incubated sample was extracted according to the procedures described above. The net N mineralization rate was calculated as the change in inorganic N (NH4+ + NO3−) concentration; the net nitrification rate as the change in NO3− concentration over 7 days. Initial inorganic N stocks were calculated from the first extraction.
Soil samples for analysis of additional soil properties were collected from the peat surface (0–5 cm) in November 2013 using a metallic ring, air-dried for 72 h and sieved to <2 mm. Samples were taken in triplicate in areas intermediary between hummock and hollow in forests, or between near palm and far from palm positions in plantations. Soil sampling locations in each plot were separated by a distance of approximately 20 m. Exchangeable cations (Ca2+, Mg2+, K+, Na+), cation exchange capacity (CEC) and base saturation were determined by displacement from the soil colloids with ammonium acetate adjusted to pH 7 (Pansu et al., 2001). Samples for analysis of soil C and N content were collected in June of 2015 from the peat surface layer (0–5 cm). Analyses of total C and N content were conducted at the University of Virginia by dry combustion using a Thermo Scientific Flash 2000 CHNS/O analyzer after being oven dried at 60°C for 48 h to constant mass. More methodological details are available in the paper by Swails et al. (2019).
Calculations and Statistical Analysis
CH4 and N2O fluxes were calculated from the rate of change in concentration of the analyte in the chamber headspace, determined by linear regression based on the four gas samples (Verchot et al., 1999; Verchot et al., 2000). Whenever a sample had leaked (ambient CH4 and N2O concentration except at chamber closure) or the accumulation curve departed from linearity for both GHGs, indicating a chamber effect, the flux was calculated with three samples. Examples of cases where observations were discarded from the regression are provided in SI1. Where there was no trend in the accumulation curve, indicating low gas flux (for instance when uptakes and emissions occur simultaneously), no samples were excluded from the regression. In total, 7% of CH4 and N2O flux observations were excluded from analysis.
Trace gas fluxes and physical parameters were calculated per spatial position by land use (n = 18 in hummock/hollow and near/far conditions) for micro-spatial comparison. They were also calculated per plot by considering the representativeness of hummock/hollow and near/far conditions at subplot level. These results were used for plot comparison within a land use (n = 6 per plot) or for land use comparison (n = 18 per land use). Determination of the area ratio representative for each spatial position in oil palm and forest plots is described by Swails et al. (2019). The hummock to hollow area ratios in forest plots were 49:51 (FOR-1), 51:49 (FOR-2), and 57:43 (FOR-3). In oil palm plots, we used the ratio of area within a 2 m radius of palms (near) to the area outside of this radius (far). The 2 m-radius area around palms is where smallholders applied fertilizers and root density is usually highest (Khalid et al., 1999; Comeau et al., 2016). The near to far ratios in oil palm plots were 25:75 (OP-2011), 27:73 (OP-2009), and 37:63 (OP-2007).
Cumulative annual rates of CH4 and N2O emissions were estimated per plot using linear interpolation between measurements dates. We summed the average cumulative flux in the first 6 months of the year (January 2014–June 2014 and January 2015–June 2015) with cumulative flux in the last 6 months of 2014 (July–December). There was no monitoring in July and August 2015, so measurements collected during the September 2015 El Niño event were excluded from cumulative annual flux after Swails et al. (2019). We used Kruskal Wallis test to detect differences between average cumulative fluxes in the land-uses (n = 18) and among plots within a land-use (n = 6).
We used one-way repeated measures ANOVA with planned comparisons to detect differences in trace gas fluxes and physical parameters between spatial positions (hummock/hollow and near/far), among plots within a land use, and between land uses. Data were log transformed to meet normality assumptions. Comparisons were made over the entire study period and during dry and wet months. We treated months with total precipitation ≤100 mm as “dry” months, and months with total precipitation >100 mm as “wet” months after Aini et al. (2015) and Hirano et al. (2007). Relationships among trace gas fluxes and physical parameters were tested with univariate regression using monthly averages either per spatial position or per plot or land use. We additionally used univariate regression to test relationships among annual rates of trace gas fluxes and soil chemical properties using monthly average gas flux per plot. Soil mineral N content and net mineralization and nitrification rates per spatial position among plots within a land-use (n = 9), among plots within a land-use (n = 9), and between land-uses (n = 27) were compared using Kruskal Wallis test. Averages computed by pooling data from the two sampling dates were similar to averages computed separately for each experiment, therefore the data from the two sampling dates were pooled to increase statistical power of the dataset. Soil exchangeable cations, CEC, base saturation, and total C and N were compared for the two land-uses (n = 9) and among plots within a land-use (n = 3) using Kruskal Wallis test. Repeated measures ANOVA was computed using Statistical Analysis Software (SAS v 9.4). We used R (v 3.2.5) for all other analyses (Kruskal Wallis test and univariate regression). The criteria for significance was p < 0.05 for all statistical tests.
Peat CO2 and GHG Budgets
The peat CO2 budget was calculated as the sum of on- and off-site C emissions and removals (Hergoualc’h and Verchot, 2014) as:
Where Peat CO2 budget: Peat net C uptake or emission; SRh: Soil heterotrophic respiration; DOC: Dissolved organic carbon exported from the soil; Litterfall: above ground C inputs through litterfall; and Roots: below ground C inputs through root mortality and exudation. All components are in Mg C ha−1 year−1 and a positive budget indicates that the peat is a net source of CO2.
The peat CO2 budgets combined site-specific soil heterotrophic respiration and litterfall rates and default values for root inputs and dissolved organic losses from the literature (Hergoualc’h and Verchot, 2014). Heterotrophic respiration rates were estimated from total soil respiration using the partitioning ratios of 55.1 ± 2.8% and 71.8 ± 10.8% in forest and oil palm, respectively, determined previously at the sites considering micro-spatial conditions and, in oil palm plantations, plantation age (Hergoualc’h et al., 2017, SI2). Cumulative annual total soil respiration (SI2) was calculated as for CH4 and N2O fluxes (Swails et al., 2019).
Litterfall was collected monthly at the forest plots in one trap (0.3 m2) per subplot installed 100 cm above the soil. The litter was subsequently oven dried to constant mass at 60°C and weighed. Annual litterfall rate was computed by annualizing the average of monthly rates across plots and its dry mass was converted to carbon using a carbon fraction of 48% (Aalde et al., 2006). Litter input in oil palm plots was calculated from the average number of fronds removed from palms annually, the average dry mass of a frond and the average palm density across plots (162 palms ha−1). Annual frond removal was estimated by counting the number of pruning scars on six palms per plot and dividing by the age of the plantation. The dry mass of fronds was measured from three fronds per plot in June 2015. Fronds were cut, fresh-weighed, and leaflet and stalk subsamples were collected for moisture content determination by oven drying to constant mass at 60°C. Frond dry mass was converted to carbon by applying a carbon fraction of 41.8% (Lamade and Setiyo, 2012).
The peat GHG budget was computed by summing up the peat CO2 budget expressed in CO2 units and annual emissions of CH4 and N2O expressed in CO2 equivalent (CO2e) units. Non-CO2 gas fluxes were converted to CO2e considering the global warming potentials of 86 for CH4 and 268 for N2O over a 20-year time horizon and with climate-carbon feedbacks (Myhre et al., 2013). Though a 100-year time horizon is the convention for national GHG inventories, a 20-year time horizon is more appropriate for evaluating impacts of land-use conversion that typically occurs over 20–30 years in the tropics.
Results
CH4 and N2O Flux Rates
Over the 19 months of the study, mean CH4 flux rate was roughly fifteen times higher in forest plots (4.5 ± 0.7 mg CH4 m−2 d−1) than in oil palm plots (0.3 ± 0.7 mg CH4 m2 d−1, p < 0.0001, Figure 2), driven by a significant difference during wet months. In wet months CH4 flux in forest (5.4 ± 0.5 mg CH4 m−2 d−1) was 54 times higher than in oil palm (0.1 ± 0.5 mg CH4 m2 d−1, p < 0.0001) whereas no difference between the two ecosystems was observed in dry months (p = 0.3). Lower CH4 flux in dry months than in wet months was conspicuous only in forest (p = 0.002).
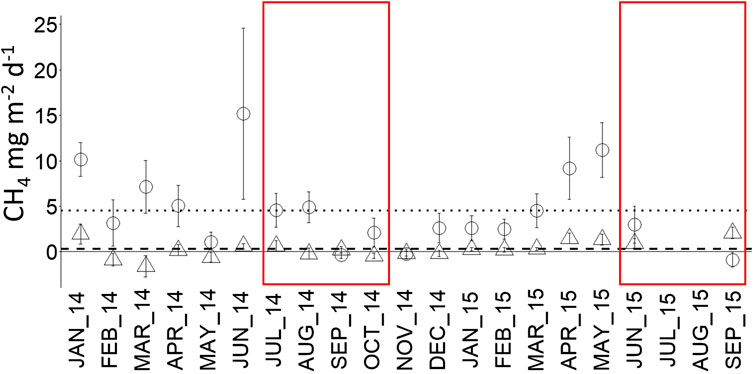
FIGURE 2. Monthly mean soil CH4 flux in forest (circle, dotted line) and oil palm (triangle, dashed line) from January 2014 to September 2015. Error bars represent standard error of the mean (n = 18). Dry months are indicated by red boxes. Dotted and dashed lines represent the 19 months averages.
Methane flux in forest ranged from 6.0 ± 1.8 mg CH4 m−2 d−1 in the plot nearest the river (FOR‐1, Figure 3A), to 5.1 ± 1.8 mg CH4 m−2 d−1 in plot (FOR-2, Figure 3B) to 2.9 ± 1.8 mg CH4 m−2 d−1 in the plot furthest from the river (FOR-3, Figure 3C). Notwithstanding, the flux was not significantly different among plots (p = 0.3) due to high intra-plot spatial variability. In oil palm, CH4 production ranged from 0.5 ± 0.3 mg CH4 m−2 d−1 in OP-2011 (Figure 3D) to 0.06 ± 0.25 mg CH4 m−2 d−1 in OP-2007 (Figure 3F) and was similar among plots (p = 0.1).
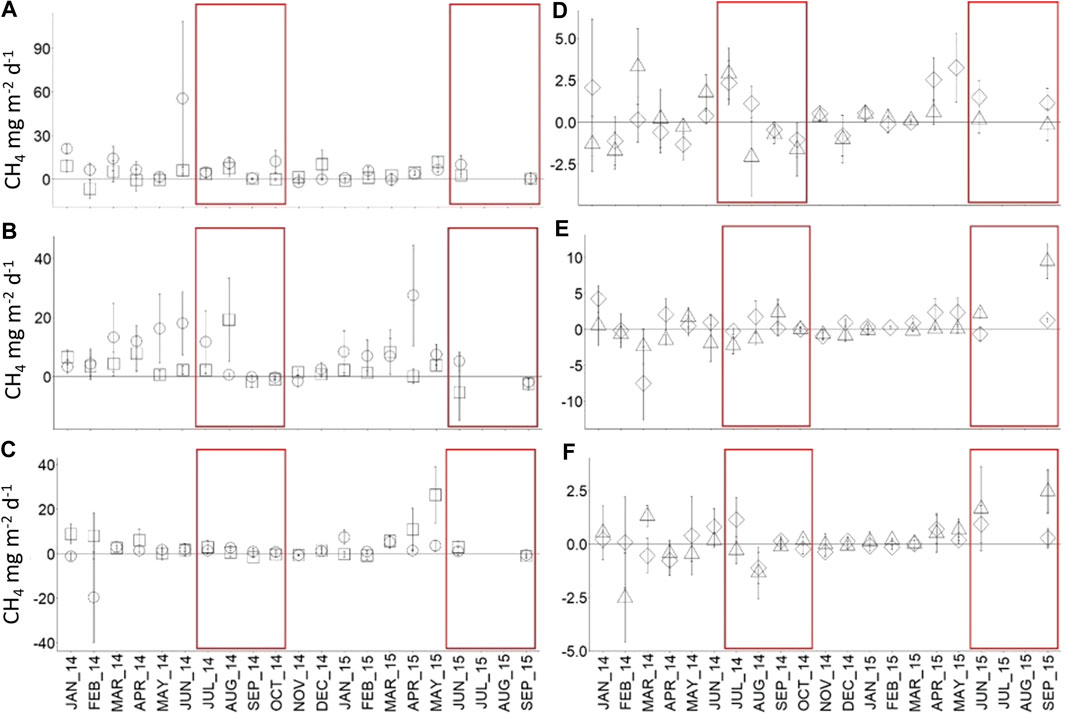
FIGURE 3. Monthly mean soil CH4 flux in hummock (square) and hollow (circle) in FOR-1 (A), FOR-2 (B), FOR-3 (C) and near (triangle) and far (diamond) positions from palm in OP-2011 (D), OP-2009 (E), and OP-2007 (F) from January 2014 to September 2015. Error bars represent standard error of the mean (n = 6). Dry months are indicated by red boxes. Note different scale between panels.
Hollows (5.5 ± 1.5 mg CH4 m−2 d−1) and hummocks (3.2 ± 1.6 mg CH4 m−2 d−1) exhibited similar CH4 flux (p = 0.8). Nonetheless during dry months, the flux was marginally higher in hollows than hummocks (p = 0.07) with a significant difference between spatial positions in FOR-1 (p = 0.02, Figure 3A) and FOR-3 (p = 0.02, Figure 3C). In oil palm, CH4 flux was similar near and far from palm, in wet months, dry months, and overall, at the plot and land-use level.
Mean N2O fluxes over the monitoring period were alike in oil palm (1.3 ± 0.3 mg N2O m−2 d−1) and forest (0.8 ± 0.3 mg N2O m−2 d−1) (p = 0.3), owing to high spatial and temporal variation in both ecosystems (Figure 4). The fluxes did not vary seasonally in oil palm (p = 0.9) whereas in forest they were marginally higher in wet months (3.3 ± 2.7 mg N2O m−2 d−1) than dry months (0.13 ± 0.44 mg N2O m−2 d−1) (p = 0.07).
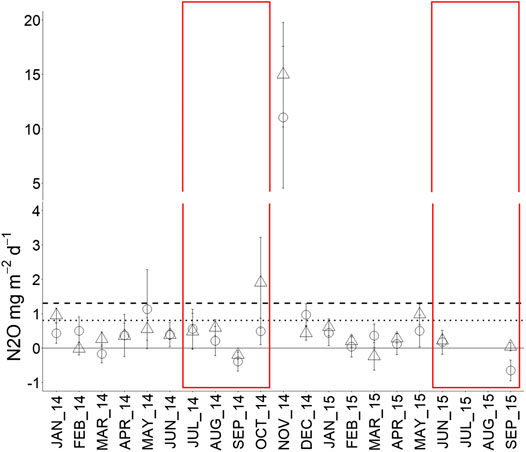
FIGURE 4. Monthly mean soil N2O flux in forest (circle, dotted line) and oil palm (triangle, dashed line) from January 2014 to September 2015 (n = 18). Error bars represent standard error of the mean. Dry months are indicated by red boxes. Dotted and dashed lines represent the 19 months averages.
In November 2014, the first month following the dry season, huge N2O emissions were recorded in FOR-1 and OP-2011 (Figures 5A,D). These were, respectively, 13 and 15 times higher than the ecosystem mean over the monitoring period. Consequently, the range of N2O flux was large in forest: From 1.8 ± 0.65 mg N2O m−2 d−1 in FOR-1 (Figure 5A) to 0.5 ± 0.69‒0.2 ± 0.67 mg N2O m−2 d−1 in FOR-2 and FOR-3 (Figures 5B,C). Similarly, oil palm exhibited a wide range of mean N2O flux: From 3.2 ± 0.4 mg N2O m−2 d−1 in OP-2011 (Figure 5D) to 0.3 ± 0.4–0.2 ± 0.4 mg N2O m−2 d−1 in OP-2009 and OP-2007 (Figures 5E,F). However, the mean flux rate was similar among plots in both forest (p = 0.8) and oil palm (p = 0.3).
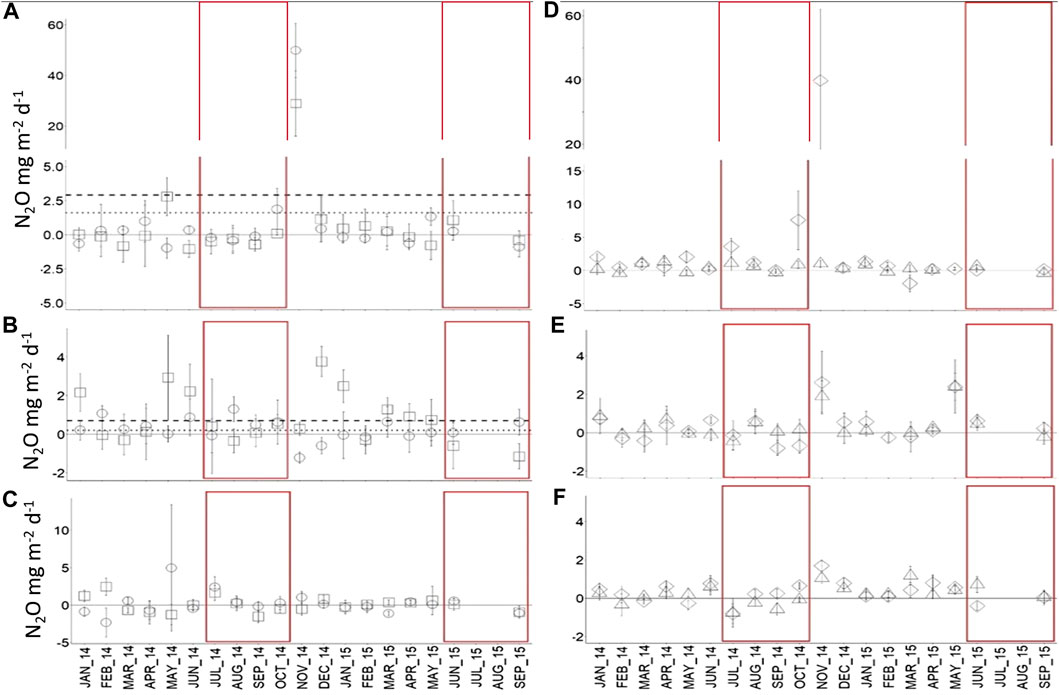
FIGURE 5. Monthly mean soil N2O flux in hummock (square, dotted line) and hollow (circle, dashed line) in FOR-1 (A), FOR-2 (B), FOR-3 (C) and near (triangle) and far (diamond) positions from palm in OP-2011 (D), OP-2009 (E), and OP-2007 (F) from January 2014 to September 2015. Error bars represent standard error of the mean (n = 6). Dry months are indicated by red boxes. Dotted and dashed lines representing the 19 months averages are displayed when significantly different between spatial positions. Note different scale between (A,D,C), and remaining panels.
N2O fluxes were not different in hollows (1.1 ± 0.4 mg N2O m−2 d−1) and hummocks (0.8 ± 0.4 mg N2O m−2 d−1) across plots (p = 0.6) but greater in hollows than hummocks in FOR-1 (Figure 5A, p = 0.04) and FOR-2 (Figure 5B, p = 0.004). In oil palm the soil at near and far from palm positions emitted N2O at a similar rate at the plot and land-use level.
Environmental Drivers of Trace Gas Fluxes
Inorganic N pools in forest and oil palm were dominated by NH4+ (Table 2). Soil NH4+ content was greater in forest plots than oil palm plots (p = 0.001) while the reverse was true for soil NO3− content (p < 0.0001). Net mineralization was higher in forest than oil palm (p = 0.008) but net nitrification was not different between the two land-uses. In forest plots soil NO3− content in hollows and net mineralization in hummocks as well as net mineralization at the plot scale was highest in FOR-3 (p = 0.0009, p = 0.0004, and p = 0.003, respectively). In oil palm plots net nitrification was higher far from palms than near palms in OP-2007 (p = 0.009). Soil NH4+ content was higher near palms than far from palms in OP-2011 (p = 0.02).
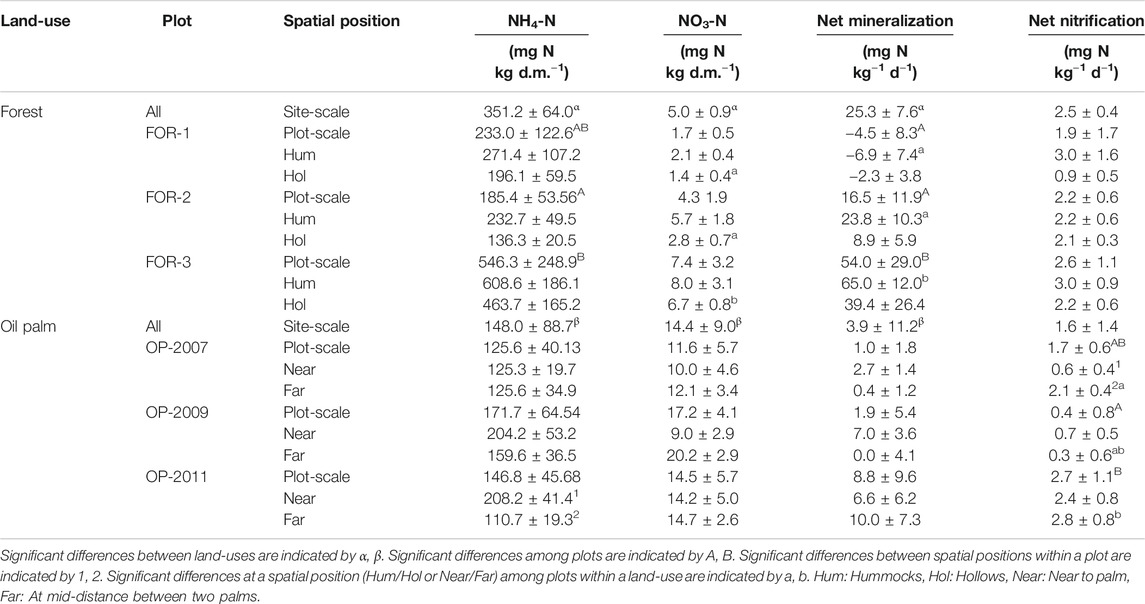
TABLE 2. Soil ammonium (NH4+) and nitrate (NO3−) contents and net mineralization and nitrification rates in forest and oil palm plots at site- and plot-scale and according to spatial position. Mean values and standard errors are presented.
Soil CEC, base saturation and C and N content were similar in both land uses (p = 0.3, 0.4, and 0.5), Table 3). In forests, there was a trend towards increasing CEC, base saturation and C content from FOR-1, which was closest to the river and was a secondary forest, to FOR-3, which was furthest from river and was a primary forest.
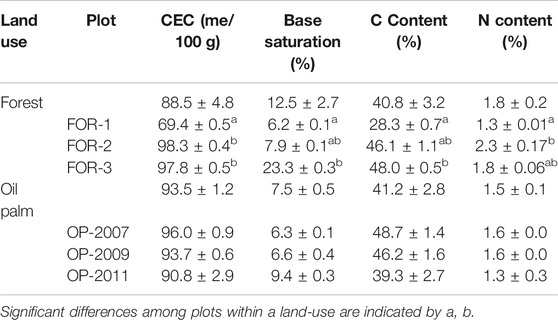
TABLE 3. Soil cation exchange capacity (CEC), base saturation, C and N content in forest and oil palm plots. Mean values and standard errors are presented.
Results on environmental parameters (water table level, WFPS, and litterfall rates in forest) are presented in SI3. Factors controlling temporal variability of soil non-CO2 fluxes were primarily hydrological. Fluxes of CH4 and N2O were related to water table level in forest, and CH4 fluxes to WFPS in oil palm. CH4 flux decreased as water table level dropped in forest plots (p = 0.02, Figure 6A). On the other hand, CH4 flux increased when soil WFPS increased in oil palm plots (p = 0.02, Figure 6B). Soil N2O flux increased as the water table rose close to the soil surface in forest plots (p = 0.02, Figure 6C) though the model relatively poorly explained the variation in N2O flux (R2 = 0.28). In both ecosystems, N2O flux varied widely when water table was near the soil surface, and N2O consumption occurred occasionally when water table level dropped far below the soil surface. Temporal fluctuations of CH4 and N2O fluxes were not related to soil or air temperature in either land use or to litterfall in forest.
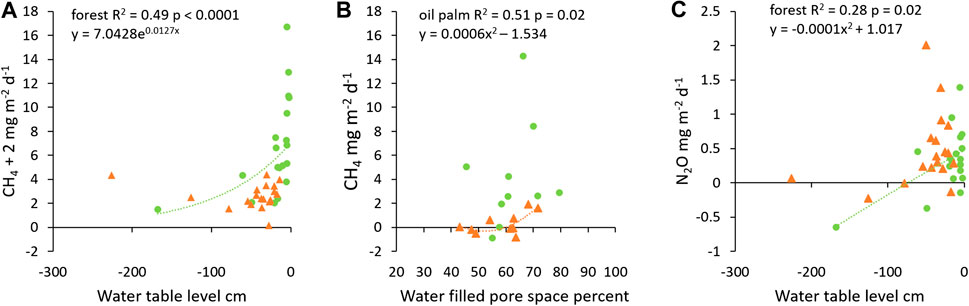
FIGURE 6. CH4 flux as a function of water table depth (A) and water-filled pore space (B) and N2O flux as a function of water table depth (C) in forest (green circle, green dotted line) and oil palm (orange triangle, orange dashed line). Each data point represents the monthly mean in a land use (n = 19 for water table depth and n = 10 for water filled pore space).
Cumulative Annual Fluxes of CH4 and N2O
Cumulative CH4 flux (kg CH4 ha−1 year−1) was two orders of magnitude higher in forest (14.0 ± 2.8) than oil palm (0.3 ± 0.4, Table 4, p < 0.0001). In forest plots there was a trend towards decreased CH4 flux with distance from river, but cumulative CH4 flux was not different among forest plots. Cumulative CH4 fluxes in oil palm plots were very close to each other and not different.
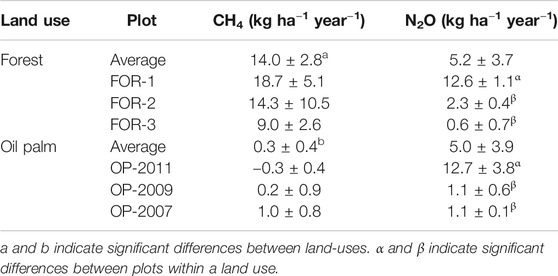
TABLE 4. Cumulative plot-scale soil CH4 and N2O flux rates ± standard error at the study sites. Mean values and standard errors are presented.
Cumulative N2O flux (kg N2O ha−1 year−1) was similar in the two land-uses (5.2 ± 3.7 in forest and 5.0 ± 3.9 in oil palm, Table 4). In forest, it was more than five times higher in secondary forest FOR-1 (12.6 ± 1.1) than in primary forests FOR-2 (2.3 ± 0.4) and FOR-3 (0.6 ± 0.7) (Table 4, p < 0.0001). In oil palm, N2O flux was more than 10 times higher in OP-2011 (12.7 ± 3.8) than in OP-2009 (1.1 ± 0.6) and OP-2007 (1.1 ± 0.1) (Table 4, p = 0.048). Among the six plots, annual N2O emissions increased with decreasing soil C content with highest emissions in the secondary forest and OP-2011 established on a land cleared 20 years before planting (Figure 7).
FOR-1 accounted for 81% of total cumulative N2O emissions in forest and OP-2011 accounted for 84% of total cumulative N2O emissions in oil palm. In addition, in OP-2011 three chambers located in the unfertilized zone (far from palm position) contributed 86% of emissions.
Net CO2 and GHG Emissions From Peat
Forest exhibited higher litterfall C inputs and lower C losses from heterotrophic respiration than oil palm (Table 5). During the monitoring period oil palm plantation was a strong net source of CO2 while forest was neither a source nor a sink. The difference in net CO2 emissions rate between ecosystems was as high as 28.9 ± 9.7 Mg CO2 ha−1 year−1. CH4 and N2O contributed importantly to the peat GHG budget in forest whereas in oil palm most emissions were released in the form of CO2. Forest to oil palm conversion implied large GHG emissions from the peat of about 28 ± 10 Mg CO2e ha−1 year−1.
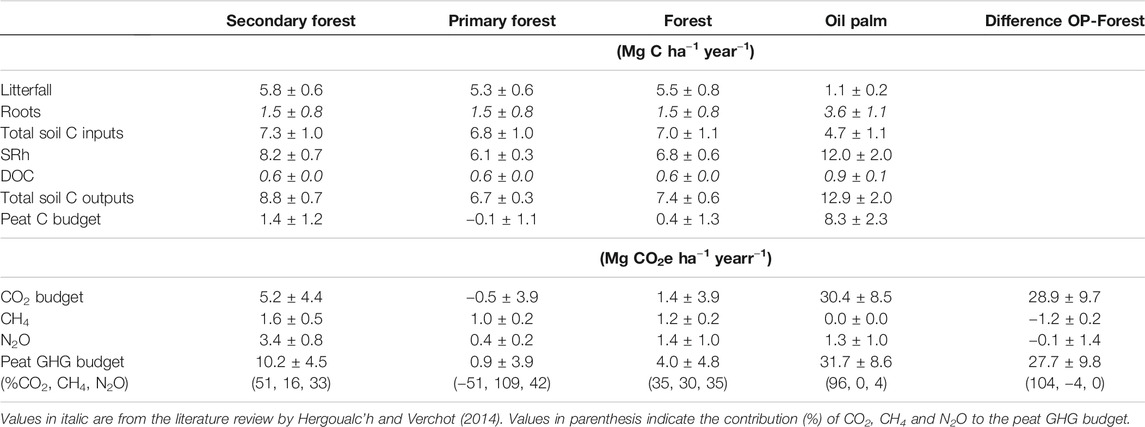
TABLE 5. Peat CO2 budget calculated as the difference of mean annual C outputs from heterotrophic soil respiration (SRh) and dissolved organic carbon (DOC) and mean annual C inputs from litterfall and root mortality in forest and oil palm (OP). Peat greenhouse gas (GHG) budget was computed as the sum of emissions of all GHG. Forest budgets are also disaggregated by forest type (secondary: FOR-1, primary: FOR-2 and FOR-3). Mean values ± standard error are expressed in Mg C or CO2-equivalent ha−1 year−1. Negative values indicate an emission reduction or removal.
Disaggregation of peat budgets by forest type shows that in the secondary forest the peat was a small net CO2 source as opposed to net CO2 neutrality in primary forests. As a result, and also owing to large soil N2O emissions, secondary forest was an important net GHG source. Regardless of their disturbance history, forests’ peat net GHG emissions were lower than in oil palm plantations.
Discussion
Spatio-Temporal Variability and Controls of CH4 Flux
Annual soil CH4 emission in forest plots (14.0 ± 2.8 kg CH4 ha−1 year−1) was comparable to fluxes measured in undrained peat swamp forest of Kalimantan in Indonesia (Inubushi et al., 2003; Jauhiainen et al., 2005) but under the 38 ± 13 kg CH4 ha−1 year−1 average for Southeast Asian peat swamp forests (Hergoualc’h and Verchot, 2014). It was also lower than CH4 emission in peat swamp forest from eddy covariance studies (e.g., Wong et al., 2018; Deshmukh et al., 2020) which account for peat surface flux as well as vegetation-mediated flux from tree stems and pneumatophores (Pangala et al., 2013; Van Lent et al., 2019).
In forest plots CH4 flux decreased in dry months when the water table fell further below ground level and oxygenation of peat surface layers promoted methanotrophy over methanogenesis, as observed in previous studies of Southeast Asian peatlands (Jauhiainen et al., 2005; Jauhiainen et al., 2008; Ishikura et al., 2019). In wet months waterlogging of surface layers created anoxic conditions conducive to anaerobic respiration and methanogenesis. Methane emissions increased in magnitude and became more variable as water table level approached the soil surface during wet months, indicating a threshold response to water table level, as observed by Jauhiainen et al. (2008).
Water table level alone only explained 49% of variation in CH4 flux in forest plots (Figure 6A). Soil WFPS may also have played a role in controlling CH4 flux when sites were not flooded, as suggested by higher soil moisture and CH4 flux in FOR-1 than in FOR-2 and FOR-3 (Supplementary Table S3). Moisture regime could also have controlled flux rates indirectly. For instance, visual inspection of the plots indicated higher density of pneumatophores near the river than further from it, potentially explaining the trend towards decreased CH4 emission with distance from river as resulting from lower plant-mediated transport. Methane production also often correlates with diverse indexes of soil carbon quality (Le Mer and Roger, 2001; Bridgham et al., 2012). The trend toward decreased emission rate with distance from river was associated with increased soil CEC, base saturation and C content (Table 3) which suggest changes in organic matter decomposition state (Andriesse, 1988). Also, there was a trend toward rising CH4 emission with rising total soil respiration (Supplementary Figure S5), with FOR-1 the only forest which was secondary and had suffered past burning events (Table 1), exhibiting highest emissions of CH4 and highest total soil respiration.
Though hollows and hummocks displayed a similar CH4 flux when all forest plots were considered together, the flux was greater in hollows than hummocks in FOR-1 and FOR-2 in dry months, when both microtopographies exhibited similar water table level (Swails et al., 2019). A higher CH4 flux in hollows than hummocks in pristine peat swamp forest usually coincides with a difference in moisture conditions between microtopographies (Ishikura et al., 2019; Hergoualc’h et al., 2020). As CH4 production is often fueled by recent plant photosynthate (Bridgham et al., 2012) difference in flux between the microtopographies at our sites may be linked to higher root exudates in hollows than hummocks when soil becomes unsaturated. In drained oil palm plots, CH4 emissions were negligible as typically found in drained tropical peatlands across various land uses (Hergoualc’h and Verchot, 2012). CH4 flux did not respond to changes in water levels, contrary to what was measured by Melling et al. (2005). However, as rainfall can briefly create waterlogged layers at the peat surface CH4 emissions increased with increasing WFPS (Figure 6B). There was no tendency toward CH4 uptake by soil during dry months or overall. Nevertheless, uptake occurred occasionally when the water table level was <50 cm below ground, indicating that water saturation at the soil surface was an important driver of CH4 emission and uptake. CH4 emissions did not vary according to spatial position (near and far from palms), though gravimetric soil moisture was higher far from palms than near palms (Swails et al., 2019).
Spatio-Temporal Variability and Controls of N2O Flux
Soil annual N2O emission in forest plots (5.2 ± 3.9 kg N2O ha−1 year−1) was within the range of fluxes from undrained peat swamp forests in Indonesia which vary from small net uptakes (−0.5 kg N2O ha−1 year−1, Inubushi et al., 2003) to high emissions (21.1 kg N2O ha−1 year−1, Hadi et al., 2005). N2O consumption is not uncommon across the tropics (Chapuis-Lardy et al., 2007) and soils in forest plots also appeared as an occasional sink. N2O consumption occurred primarily in hummocks, which are better aerated and can remain dry when lower lying hollows are wet (Swails et al., 2019). N2O uptake has been observed in Southeast Asian peatland (e.g., Takakai et al., 2006; Jauhiainen et al., 2012) and is usually promoted by low nitrate availability, high WFPS and more generally by conditions inhibiting N2O diffusion in the soil (Chapuis-Lardy et al., 2007). However, in soils with high soil C content, enhanced respiration can create anoxic microenvironments where denitrification takes place, even under well aerated conditions (Chapuis-Lardy et al., 2007), which may explain N2O uptake by hummocks in our forest plots. N2O emissions increased as water table rose closer to the soil surface in forest plots, in agreement with findings by Melling et al. (2007) but in disagreement with other studies, e.g., Hergoualc’h et al. (2020), Takakai et al. (2006), Jauhiainen et al. (2012) also observed a control of soil water status over N2O emissions in undrained forest, with greater flux in wet than dry months. Jauhiainen et al. (2012) attribute the increase in N2O flux to enhanced decomposition of litter inputs under optimum soil moisture conditions. As for CH4, the trend of decrease in cumulative N2O emissions with river distance was associated with a decrease in total soil respiration (Supplementary Figure S5) and changes in soil organic matter decomposition state (Table 3).
Annual emission in oil palm plots (5.0 ± 3.7 kg N2O ha−1 year−1) was above IPCC default value for oil palm plantations on peat (1.2 kg N2O ha−1 year−1, Drösler et al., 2014). Assessments of annual N2O emissions in oil palm plantations on peat (Melling et al., 2007; Sakata et al., 2015; Oktarita et al., 2017; Chaddy et al., 2019; Meijide et al., 2020) indicate much higher rates (32 ± 2 kg N2O ha−1 year−1, n = 8) than our result and IPCC default value. They also vary greatly according to site, management practices (tillage/no tillage or use of coated vs. conventional nitrogen fertilizers) (Sakata et al., 2015) and presence of hot spots (Oktarita et al., 2017). Emission rates in OP-2007 and OP-2009 were in the range of flux reported by Melling et al. (2007) while emissions in OP-2011 were closer to but lower than values reported by Sakata et al. (2015), Oktarita et al. (2017), Chaddy et al. (2019) and Meijide et al. (2020). Lower average emission at our site as compared to the literature may arise from low supply of labile carbon as indicated by the ratio of recalcitrant aromatic compounds to labile aliphatic compounds in soil organic matter (Swails et al., 2018) and also potentially from water table levels higher (Supplementary Table S3) than on average (65 cm, Hergoualc’h and Verchot, 2014). Water table level was poorly controlled by smallholders, particularly in OP-2011 (Table 1), which was flooded in March 2014 and May 2014. High water table can create anaerobic conditions, thereby decreasing N2O production directly by inhibiting nitrification and indirectly by decreasing the production of NO3− substrate for denitrification. Additionally, more anaerobic conditions promote the formation of N2 over N2O during denitrification. Emissions could also be slightly under-estimated as our experimental design did not use intensive sampling after fertilization to capture potential transient spikes in emissions from the area of nitrogen application.
OP-2011 included hot spots which contributed 86% of annual emissions. Hot spots have been observed in drained organic soils in the tropics (Oktarita et al., 2017), temperate (Lee et al., 2017) and Arctic zones (Repo et al., 2009; Marushchak et al., 2011). As in the study by Oktarita et al. (2017) hot spots were located far from palms (Figure 5D), where N uptake by roots may be lower (Nelson et al., 2006). Also “in vitro” net nitrification rate far from palms was higher in OP-2011 than elsewhere despite similar initial mineral N content across plots at this spatial position (Table 2) which suggests that hot spots are driven by microbial or fungal community composition, as proposed by Oktarita et al. (2017).
The strong relationship between annual N2O emissions and soil C content along the six plots (Figure 7) is agreement with findings by Ye et al. (2016) for rice fields on peat in the Sacramento-San Joaquin Delta. This relationship suggests that the highest emission in FOR-1 and OP-2011 may be explained by carbon limitations to microbial growth and linked to past land-use history in these plots. FOR-1 was a 30-year-old secondary forest, managed as an agroforestry garden previously. In OP-2011, forest clearance occurred in 1989 and was followed by successive crop rotations and associated fires while other plots were cleared more recently in 2005. The past history of cultivation in FOR-1 and OP-2011 may have depleted labile carbon supply in soils (Swails et al., 2018) and exposed deeper layers of peat mixed with mineral soil. When facing carbon limitations to growth, microbes break down dissolved organic nitrogen, using carbon to support energy, growth and maintenance requirements and secreting NH4+ as a by-product (Chapin et al., 2002). Increased availability of NH4+ enhances production of NO3− through nitrification under aerobic conditions (Robertson, 1989). Thus, nitrification may have been elevated during dry months in these plots. In FOR-1 as in OP-2011, we observed extremely high N2O emissions in November 2014. These pulses occurred at the beginning of the wet season, when precipitation increased after four dry months. The sudden change in soil moisture conditions combined with a large supply of NO3− could have promoted high rates of denitrification leading to large fluxes of N2O from soils in FOR-1 and OP-2011 at the beginning of the wet season, similar to observations in an oil palm plantation on tropical peatland by Chaddy et al. (2019).
The Global Warming Impact of Peat Forest Conversion to Oil Palm Plantation
Indonesian peatlands are known as an important and growing source of GHG emissions to the atmosphere but variations in estimates remain large. Our study indicates that forest to oil palm conversion resulted in a massive increase in peat net CO2 emission rate (29 Mg CO2e ha−1 year−1), a small decrease in CH4 emissions (1 Mg CO2e ha−1 year−1) and no alteration in N2O emissions (Table 5). Ensuing GHG loss over a 25-year rotation period typical of oil palm plantation amounts to 693 Mg CO2e ha−1. This result combined with C stock changes in above ground vegetation (excluding litter) measured at the sites by Novita et al. (2020) (189 and 28 Mg C ha−1 in forest and oil palm) leads to emissions of 1,283 Mg CO2e ha−1 over 25 years or 51 Mg CO2e ha−1 year−1. This assessment doesn’t account for massive emission from peat burning with each land-clearing fire releasing 541 Mg CO2e ha−1 (Drösler et al., 2014). It neither accounts for peat decomposition emissions that occurred during intermediary crop cultivation before conversion to oil palm. The larger share of CO2 than that of either CH4 or N2O to peat GHG emissions from land-use change is consistent with the literature on Southeast Asian peatlands (Hergoualc’h and Verchot, 2014). Drainage resulted in a drastic increase in CO2 emissions from accelerated soil organic matter decomposition, and vegetation cover change induced substantial reduction in aboveground litter inputs. The latter may be underestimated as branchfall was not monitored in forest.
While in forest, all gases contributed equally to the peat GHG budget, in oil palm CO2 was the dominant gas but several studies which found high N2O emissions stemming from peat decomposition highlighted the importance of this gas for GHG accounting (Sakata et al., 2015; Oktarita et al., 2017; Dommain et al., 2018). Forest conversion to croplands and shrublands substantially raises soil emissions of N2O according to the meta-analysis conducted by Hergoualc’h and Verchot (2014). This was not the case in our study because primary and secondary forests were merged and roughly 80% of the N2O flux from forest came from FOR-1. Discarding observations from FOR-1 resulted in a four times lower average cumulative N2O flux (0.4 Mg CO2e ha−1 year−1) and peat GHG budget (0.9 Mg CO2e ha−1 year−1) (Table 5) in primary forest than when all forest types were merged. Current data availability for GHG inventories only allows disaggregating peat GHG emission factors of forest by combined drainage and disturbance (primary/secondary) status, as trace gas measurements in undrained secondary forest have seldom been conducted. Our results indicate that undrained 30-year-old secondary forests still suffer from past land-use history and exhibit soil GHG emissions substantially different from that of primary forest. The lack of distinction between primary and secondary undrained peat forest has implications for national GHG accounting and research is critically needed to support development of more accurate emission factors.
Knowledge on all GHG is required for assessing either climate change impacts on the potential of peat forest to act as a C sink or as a GHG source (Wang et al., 2018), or climate impacts of land-use change in tropical peatlands. Additional assessments across the region are necessary to understand the cumulative impact of simultaneous changes in fluxes of CH4, N2O, and CO2 that occur with conversion and drainage of tropical peatlands.
Conclusion
Drainage and conversion of an Indonesian peat swamp forests to oil palm plantation implied a large net increase in peat GHG emissions, mainly released as CO2. Past land-use change also impacted peat net GHG emissions in a secondary peat swamp forest, decades following conversion. Spatio-temporal variability in peat CH4 and N2O flux in forests and oil palm plantations was related to hydrological drivers. Our study highlights the devastating impact of tropical peat swamp forest conversion on peat GHG emissions to the atmosphere, with and without drainage, and for many years following initial conversion.
Data Availability Statement
The datasets presented in this study can be found in online repositories. The names of the repository/repositories and accession number(s) can be found at: https://doi.org/10.17528/CIFOR/DATA.00201.
Author Contributions
ES designed and conducted the study, performed data analysis, and wrote the manuscript. KH, DL, and LV were integrally involved in the study design, data analysis and interpretation, and writing the manuscript. NN selected sites and conducted field and laboratory work in the same area, contributing data on soil mineral N content and rates of net mineralization and net nitrification to this manuscript.
Funding
This research was conducted under the Sustainable Wetlands Adaptation and Mitigation Program (SWAMP) and was generously supported by the governments of the United States of America (Grant No. MTO-069018), Norway (Grant Agreement No. QZA-12/0882) and Germany (Grant No. KI II 7—42206-6/75). It was undertaken as part of the CGIAR research program on Climate Change, Agriculture and Food Security (CCAFS).
Conflict of Interest
The authors declare that the research was conducted in the absence of any commercial or financial relationships that could be construed as a potential conflict of interest.
Acknowledgments
The authors are grateful to the staff of Tanjung Puting National Park for facilitating the study and providing lodging. We would also like to thank all assistants and villagers for their continuous help in the field, and also Desti Hertanti for her indispensable support to collect data, and Novi Wahyuni for her critical work in analyzing GHG concentrations.
Supplementary Material
The Supplementary Material for this article can be found online at: https://www.frontiersin.org/articles/10.3389/fenvs.2021.617828/full#supplementary-material.
References
Aalde, H., Gonzalez, P., Gytarski, M., Krug, T., Kurz, W. A., Lasco, R. D., et al. (2006). “Chapter 2: generic methodologies applicable to multiple land‐use categories,” in 2006 IPCC guidelines for national greenhouse gas inventories. Editors S. Eggleston, L. Buendia, K. Miwa, T. Ngara, and K. Tanabe (Hayama, Japan: IGES).
Aini, F. K., Hergoualc’h, K., Smith, J. U., and Verchot, L. (2015). Nitrous oxide emissions along a gradient of tropical forest disturbance on mineral soils in Sumatra. Agric. Ecosyst. Environ. 214, 107–117. doi:10.1016/j.agee.2015.08.022
Andriesse, J. P. (1988). Nature and management of tropical peat soils. FAO Soils Bull 59. Rome, Italy: Food and Agriculture Organization (FAO) of United Nations, 165.
Arah, J. R. M. (1997). Apportioning nitrous oxide fluxes between nitrification and denitrification using gas-phase mass spectrometry. Soil Biol. Biochem. 29, 1295–1299. doi:10.1016/s0038-0717(97)00027-8
Austin, K. G., Harris, N. L., Wijaya, A., Murdiyarso, D., Harvey, T., Stolle, F., et al. (2018). A review of land-based greenhouse gas flux estimates in Indonesia. Environ. Res. Lett. 13, 055003. doi:10.1088/1748-9326/aab531
Bridgham, S., Cadillo-Quiroz, H., Keller, J., and Zhuang, Q. (2012). Methane emissions from wetlands: biogeochemical, microbial, and modeling perspectives from local to global scales. Glob. Change Biol. 19, 1325–1346. doi:10.1111/gcb.12131
Butterbach-Bahl, K., Baggs, E. M., Dannenmann, M., Kiese, R., and Zechmeister-Boltenstern, S. (2013). Nitrous oxide emissions from soils: how well do we understand the processes and their controls?. Philos. Trans. R. Soc. B 368, 20130122. doi:10.1098/rstb.2013.0122 |
Chaddy, A., Melling, L., Ishikura, K., and Hatano, R. (2019). Soil N2O emissions under different N rates in an oil palm plantation on tropical peatland. Agriculture 9, 213. doi:10.3390/agriculture9100213
Chapin, F. S., Matson, P. A., and Mooney, H. A. (2002). Principles of terrestrial ecosystem ecology. New York, NY: Springer.
Chapuis-Lardy, L., Wrage, N., Metay, A., Chotte, J., and Bernoux, M. (2007). Soil, a sink for N2O? a review. Glob. Change Biol. 13, 1–17. doi:10.1111/j.1365-2486.2006.01280.x
Comeau, L., Hergoualc’h, K., Hartill, J., Smith, J., Verchot, L., Peak, D., et al. (2016). How do the heterotrophic and the total soil respiration of an oil palm plantation on peat respond to nitrogen fertilizer application? Geoderma 268, 41–51. doi:10.1016/j.geoderma.2016.01.016
Cooper, H. V., Evers, S., Aplin, P., Crout, N., Dahalan, M. P. B., and Sjogersten, S. (2020). Greenhouse gas emissions resulting from conversion of peat swamp forest to oil palm plantation. Nat. Commun. 11, 407. doi:10.1038/s41467-020-14298-w |
Corley, R. H. V., and Tinker, P. B. (2003). The oil palm. Oxford, United Kingdom: Blackwell Science.
Deshmukh, C. S., Julius, D., Evans, C. D., Susanto, A. P., Page, S. E., Gauci, V., et al. (2020). Impact of forest plantation on methane emissions from tropical peatland. Glob. Change Biol. 26, 2477–2495. doi:10.1111/gcb.15019
DJP (2015). Statistik perkebunan Indonesia; kelapa sawit 2014–2016. Jakarta, Indonesia: Direktorat Jenderal Perkebunan, 69.
Dommain, R., Frolking, S., Jeltsch-Thömmes, A., Joos, F., Couwenberg, J., and Glaser, P. (2018). A radiative forcing analysis of tropical peatlands before and after their conversion to agricultural plantations. Glob. Change Biol. 24, 5518–5533. doi:10.1111/gcb.14400 |
Drösler, M., Verchot, L. V., Freibauer, A., Pan, G., Evans, C. D., Bourbonniere, R. A., et al. (2014). “Chapter 2: drained inland organic soils,” in Supplement to the 2006 guidelines for national greenhouse gas inventories: wetlands. Editors T. Hiraishi, T. Krug, K. Tanabe, N. Srivastava, B. Jamsranjav, M. Fukudaet al. (Geneva, Switzerland: IPCC).
Farmer, J., Matthew, R., Smith, P., Langan, C., Hergoualc’h, K., Verchot, L., et al. (2014). Comparison of methods for quantifying soil carbon in tropical peats. Geoderma 214-215, 177–183. doi:10.1016/j.geoderma.2013.09.013
Furukawa, Y., Inubushi, K., Ali, M., Itang, A. M., and Tsuruta, H. (2005). Effect of changing groundwater levels caused by land-use changes on greenhouse gas fluxes from tropical peat lands. Nutr. Cycl. Agroecosyst. 71, 81–91. doi:10.1007/s10705-004-5286-5
Günther, A. B., Huth, V., Jurasinski, G., and Glatzel, S. (2014). Scale-dependent temporal variation in determining the methane balance of a temperate fen. Greenh. Gas Meas. Manag. 4, 41–48. doi:10.1080/20430779.2013.850395
Hadi, A., Inubushi, K., Furukawa, Y., Purnomo, E., Rasmadi, M., and Tsuruta, H. (2005). Greenhouse gas emissions from tropical peatlands of Kalimantan,Indonesia. Nutr. Cycl. Agroecosyst. 71, 73–80. doi:10.1007/s10705-004-0380-2
Harianti, M., Sutandi, A., Saraswati, R., Maswar, , and Sabiham, S. (2017). Organic acids exudates and enzyme activities in the rhizosphere based on distance from the trunk of oil palm in peatland. Malays. J. Soil Sci. 21, 73–88.
Hart, S. C., Stark, J. M., Davidson, E. A., and Firestone, M. K. (1994). “Nitrogen mineralization, immobilization and nitrification,” in Methods of soil analysis: part 2 microbial and biochemical properties. Editors R. W. Weaver, S. Angle, P. Bottomley, D. Bezdicek, S. Smith, A. Tabatabaiet al. (Madison, WI: Soil Science Society of America), 985–1018.
Hergoualc’h, K., Dezzeo, N., Verchot, L. V., Martius, C., Van Lent, J., Del Aguila‐Pasquel, J., et al. (2020). Spatial and temporal variability of soil N2O and CH4 fluxes along a degradation gradient in a palm swamp peat forest in the Peruvian Amazon. Glob. Change Biol. 26, 7198–7216. doi:10.1111/gcb.15354
Hergoualc’h, K., Hendry, D. T., Murdiyarso, D., and Verchot, L. V. (2017). Total and heterotrophic soil respiration in a swamp forest and oil palm plantations on peat in Central Kalimantan, Indonesia. Biogeochemistry 135, 203–220. doi:10.1007/s10533-017-0363-4
Hergoualc’h, K., Skiba, U., Harmand, J. M., and Oliver, R. (2007). Processes responsible for the nitrous oxide emission from a Costa Rican Andosol under a coffee agroforestry plantation. Biol. Fertil. Soils 43, 787–795. doi:10.1007/s00374-007-0168-z
Hergoualc’h, K., and Verchot, L. (2012). Changes in soil CH4 fluxes from the conversion of tropical peat swamp forests: a meta-analysis. J. Integr. Environ. Sci. 9, 93–101. doi:10.1080/1943815X.2012.679282
Hergoualc’h, K., and Verchot, L. (2014). Greenhouse gas emission factors for land use and land-use change in Southeast Asian peatlands. Mitig. Adapt. Strateg. Glob. Change 19, 789–807. doi:10.1007/s11027-013-9511-x
Hergoualc’h, K., and Verchot, L. (2011). Stocks and fluxes of carbon associated with land use change in Southeast Asian tropical peatlands: a review. Glob. Biogeochem. Cycles 25, GB2001. doi:10.1029/2009GB003718
Hirano, T., Segah, H., Harada, T., Limin, S., June, T., Hirata, R., et al. (2007). Carbon dioxide balance of a tropical peat swamp forest in Kalimantan, Indonesia. Glob. Change Biol. 13, 412–425. doi:10.1111/j.1365-2486.2006.01301.x
Hutchinson, G. L., and Livingston, G. P. (1993). “Use of chamber systems to measure trace gas fluxes,” in Symposium on agroecosystem effects on radiatively important trace gases and global climate change, at the meeting of the American society of agronomy, Denver, CO, October 27–November 1, 1991.
Hynes, R. K., and Knowles, R. (1984). Production of nitrous oxide by Nitrosomonas europaea: effects of acetylene, pH, and oxygen. Can. J. Microbiol. 30, 1397–1404. doi:10.1139/m84-222
Inubushi, K., Furukawa, Y., Hadi, A., Purnomo, E., and Tsuruta, H. (2003). Seasonal changes of CO2, CH4 and N2O fluxes in relation to land-use change in tropical peatlands located in coastal area of South Kalimantan. Chemosphere 52, 603–608. doi:10.1016/s0045-6535(03)00242-x |
Ishikura, K., Hirata, R., Hirano, T., Okimoto, Y., Wong, G. X., Melling, L., et al. (2019). Carbon dioxide and methane emissions from peat soil in an undrained tropical peat swamp forest. Ecosystems 22, 1852–1868. doi:10.1007/s10021-019-00376-8
Jauhiainen, J., Limin, S., Silvennoinen, H., and Vasander, H. (2008). Carbon dioxide and methane fluxes in drained tropical peat before and after hydrological restoration. Ecology 89, 3503–3514. doi:10.1890/07-2038.1 |
Jauhiainen, J., Silvennoinen, H., Hämäläinen, R., Kusin, K., Limin, S., Raison, R. J., et al. (2012). Nitrous oxide fluxes from tropical peat with different disturbance history and management. Biogeosciences 9, 1337–1350. doi:10.5194/bg-9-1337-2012
Jauhiainen, J., Silvennoinen, H., Könönen, M., Limin, S., and Vasander, H. (2016). Management driven changes in carbon mineralization dynamics of tropical peat. Biogeochemistry 129, 115–132. doi:10.1007/s10533-016-0222-8
Jauhiainen, J., Takahashi, H., Heikkinen, J. E. P., Martikainen, P. J., and Vasander, H. (2005). Carbon fluxes from a tropical peat swamp forest floor. Glob. Change Biol. 11, 1788–1797. doi:10.1111/j.1365-2486.2005.001031.x
Jones, H. A., and Nedwell, D. B. (1993). Methane emission and methane oxidation in land-fill cover soil. Microb. Ecol. 102, 185–195. doi:10.1111/j.1574-6968.1993.tb05809.x
Khalid, H., Zin, Z. Z., and Anderson, J. M. (1999). Quantification of oil palm biomass and nutrient value in a mature oil palm plantation: belowground biomass. J. Oil Palm Res. 11, 63–71.
King, G. M., Roslev, P., and Skovgaard, H. (1990). Distribution and rate of methane oxidation in sediments of the Florida Everglades. Appl. Environ. Microbiol. 56, 2902–2911. doi:10.1128/aem.56.9.2902-2911.1990 |
Koh, L. P., Miettinen, J., Liew, S. C., and Ghazoul, J. (2011). Remotely sensed evidence of tropical peatland conversion to oil palm. Proc. Natl. Acad. Sci. U. S. A. 108, 5127–5132. doi:10.1073/pnas.1018776108 |
Lamade, E., and Setiyo, I. E. (2012). “Variations of carbon content among oil palm organs in North Sumatra conditions: implications for carbon stock estimation at plantation scale,” in International conference on oil palm and environment (ICOPE 2012), Bali, Indonesia, February 22–24, 2012.
Le Mer, J., and Roger, P. (2001). Production, oxidation, emission and consumption of methane by soils: a review. Eur. J. Soil Biol. 37, 25–50. doi:10.1016/s1164-5563(01)01067-6
Lee, A., Winther, M., Priemé, A., Blunier, T., and Christensen, S. (2017). Hot spots of N2O emission move with the seasonally mobile oxic-anoxic interface in drained organic soils. Soil Biol. Biochem. 115, 178–186. doi:10.1016/j.soilbio.2017.08.025
Martikainen, P. J., Nykänen, H., Crill, P., and Silvola, J. (1993). Effect of a lowered water table on nitrous oxide fluxes from northern peatlands. Nature 366, 51–53. doi:10.1038/366051a0
Marushchak, M. E., Pitkämäki, A., Koponen, H., Biasi, C., Seppälä, M., and Martikainen, P. J. (2011). Hot spots for nitrous oxide emissions found in different types of permafrost peatlands. Glob. Change Biol. 17, 2601–2614. doi:10.1111/j.1365-2486.2011.02442.x
Meijide, A., de la Rua, C., Guillaume, T., Roll, A., Hassler, E., Stiegler, C., et al. (2020). Measured greenhouse gas budgets challenge emission savings from palm-oil biodiesel. Nat. Commun. 11, 1089. doi:10.1038/s41467-020-14852-6 |
Melling, L., Hatano, R., and Goh, K. J. (2005). Methane fluxes from three ecosystems in tropical peatland of Sarawak, Malaysia. Soil Biol. Biochem. 37, 1445–1453. doi:10.1016/j.soilbio.2005.01.001
Melling, L., Hatano, R., and Goh, K. J. (2007). Nitrous oxide emissions from three ecosystems in tropical peatland of Sarawak, Malaysia. Soil Sci. Plant Nutr. 53, 792–805. doi:10.1111/j.1747-0765.2007.00196.x
Miettinen, J., Hooijer, A., Verimmen, R., Liew, S. C., and Page, S. E. (2017). From carbon sink to carbon source: extensive peat oxidation in insular Southeast Asia since 1990. Environ. Res. Lett. 12, 024014. doi:10.1088/1748-9326/aa5b6f
Miettinen, J., Shi, C., and Liew, S. C. (2016). Land cover distribution in the peatlands of Peninsular Malaysia, Sumatra and Borneo in 2015 with changes since 1990. Glob. Ecol. Conserv. 6, 67–78. doi:10.1016/j.gecco.2016.02.004
Myhre, G., Shindell, D., Bréon, F. M., Collins, W., Fuglestvedt, J., Huang, J., et al. (2013). “Anthropogenic and natural radiative forcing,,” in Climate change 2013: the physical science basis. Contribution of working group I to the fifth assessment report of the intergovernmental panel on climate change. Editors T. F. Stocker, D. Qin, G. K. Plattner, M. Tignor, S. K. Allen, J. Boschunget al. (Cambridge, MA and New York, NY: Cambridge University Press).
Nelson, P. N., Banabas, M., Scotter, D. R., and Webb, M. J. (2006). Using soil water depletion to measure spatial distribution of root activity in oil palm (Elaeis guineensis Jacq.) plantations. Plant Soil 286, 109–121. doi:10.1007/s11104-006-9030-6
Novita, N. (2016). Carbon stocks and soil greenhouse gas emissions associated with forest conversion to oil palm plantations in Tanjung Puting tropical peatlands, Indonesia. PhD dissertation. Corvallis, OR: Oregon State University.
Novita, N., Kauffman, J. B., Hergoualc’h, K., Murdiyarso, D., Tryanto, D. H., and Jupesta, J. (2020). “Carbon stocks from peat swamp forest and oil palm plantation in central kalimantan, Indonesia,” in Climate change research, policy and actions in Indonesia. Editors R. Djalante, J. Jupesta, and E. Aldrian (Cham, Switzerland: Springer International Publishing).
Obidzinski, K., Andriani, R., Komarudin, H., and Andrianto, A. (2012). Environmental and social impacts of oil palm plantations and their implications for biofuel production in Indonesia. Ecol. Soc. 17, 25. doi:10.5751/es-04775-170125
Oktarita, S., Hergoualc’h, K., Anwar, S., and Verchot, L. V. (2017). Substantial N2O emissions from peat decomposition and N fertilization in an oil palm plantation exacerbated by hotspots. Environ. Res. Lett. 12, 104007. doi:10.1088/1748-9326/aa80f1
Pangala, S. R., Moore, S., Hornibrook, E. R., and Gauci, V. (2013). Trees are major conduits for methane gas egress from tropical forested wetlands. New Phytol. 197, 524–531. doi:10.1111/nph.12031 |
Pansu, M., Gautheyrou, J., and Loyer, J. Y. (2001). “Soil analysis,” in Sampling, instrumentation, quality control. (Lisse, The Netherlands:A.A. Balkema Publishers). |
Parn, J., Verhoeven, J. T. A., Butterbach-Bahl, K., Dise, N. B., Ullah, S., Aaa, A., et al. (2018). Nitrogen-rich organic soils under warm well-drained conditions are global nitrous oxide emission hotspots. Nat. Commun. 9, 1135. doi:10.1038/s41467-018-03540-1 |
Pumpanen, J., Longdoz, B., and Kutsch, W. L. (2009). “Field measurements of soil respiration: principles and constraints, potentials and limitations of different methods,” in Soil carbon dynamics—an integrated methodology. Editors A. Heinemeyer, W. L. Kutsch, and M. Bahn (Cambridge, MA: Cambridge University Press), 16–33.
Repo, M., Susiluoto, S., Lind, S., Jokinen, S., Elsakov, V., Biasi, C., et al. (2009). Large N2O emissions from cryoturbated peat soil in tundra. Nat. Geosci. 2, 189–192. doi:10.1038/ngeo434
Rixen, T., Baum, A., Wit, F., and Samiaji, J. (2016). Carbon leaching from tropical peat soils and consequences for carbon balances. Front. Earth Sci. 4. doi:10.3389/feart.2016.00074
Robertson, G. P. (1989). “Nitrification and denitrification in humid tropical ecosystems: potential controls on nitrogen retention,” in Mineral nutrients in tropical forest and savanna ecosystems. Editor J Proctor (Oxford: Blackwell Scientific), 55–69.
Sakata, R., Shimada, S., Arai, H., Yoshioka, N., Yoshioka, R., Aoki, H., et al. (2015). Effects of soil type and nitrogen fertilizer on nitrous oxide and carbon dioxide emissions in oil palm plantations. Soil Sci. Plant Nutr. 61, 48–60. doi:10.1080/00380768.2014.960355
Skiba, U., Hergoualc’h, K., Drewer, J., Meijide, A., and Knohl, A. (2020). Oil palm plantations are large sources of nitrous oxide, but where are the data to quantify the impact on global warming?. Curr. Opin. Environ. Sustain. 47, 81–88. doi:10.1016/j.cosust.2020.08.019
Skiba, U., and Smith, K. A. (2000). The control of nitrous oxide emissions from agricultural and natural soils. Chemosphere Glob. Change Sci. 2, 379–386. doi:10.1016/s1465-9972(00)00016-7
Solorzano, L. (1969). Determination of ammonia in natural water by the phenolhypochlorite method. Limnol. Oceanogr. 14, 799–801. doi:10.4319/lo.1969.14.5.0799
Swails, E., Hertanti, D., Hergoualc’h, K., Verchot, L., and Lawrence, D. (2019). The response of soil respiration to climatic drivers in undrained forest and drained oil palm plantations in an Indonesian peatland. Biogeochemistry 142, 37–51. doi:10.1007/s10533-018-0519-x
Swails, E., Jaye, D., Verchot, L., Hergoualc’h, K., Schirrmann, M., Borchard, N., et al. (2018). Will CO2 emissions from drained tropical peatlands decline over time? Links between soil organic matter quality, nutrients, and C mineralization rates. Ecosystems 21, 868–885. doi:10.1007/s10021-017-0190-4
Takakai, F., Morishita, T., Hashidoko, Y., Darung, U., Kuramochi, K., Dohong, S., et al. (2006). Effects of agricultural land-use change and forest fire on N2O emission from tropical peatlands, Central Kalimantan, Indonesia. Soil Sci. Plant Nutr. 52, 662–674. doi:10.1111/j.1747-0765.2006.00084.x
Tian, H., Xu, R., Canadell, J. G., Thompson, R. L., Winiwarter, W., Suntharalingam, P., et al. (2020). A comprehensive quantification of global nitrous oxide sources and sinks. Nature 586, 248–256. doi:10.1038/s41586-020-2780-0 |
Turetsky, M. R., Kotowska, A., Bubier, J., Dise, N. B., Crill, P., Hornibrook, E. R. C., et al. (2014). A synthesis of methane emissions from 71 northern, temperate, and subtropical wetlands. Glob. Change Biol. 20, 2183–2197. doi:10.1111/gcb.12580
Van Len, J., Hergoualc’h, K., and Verchot, L. V. (2015). Reviews and syntheses: soil N2O and NO emission from land use and land-use change in the tropics and subtropics: a meta-analysis. Biogeosciences 12, 7299–7313. doi:10.5194/bg-12-7299-2015
Van Lent, J., Hergoualc’h, K., Verchot, L., Oenema, O., and van Groenigen, J. W. (2019). Greenhouse gas emissions along a peat swamp forest degradation gradient in the Peruvian Amazon: soil moisture and palm roots effects. Mitig. Adapt. Strateg. Glob. Change 24, 625. doi:10.1007/s11027-018-9796-x
Verchot, L. V., Davidson, E. A., Cattânio, H., Ackerman, I. L., Erickson, H. E., and Keller, M. (1999). Land use change and biogeochemical controls of nitrogen oxide emissions from soils in eastern Amazonia. Glob. Biogeochem. Cycles 13, 31–46. doi:10.1029/1998gb900019
Verchot, L. V., Davidson, E. A., Cattânio, J. H., and Ackerman, I. L. (2000). Land-use change and biogeochemical controls of methane fluxes in soils of eastern Amazonia. Ecosystems 3, 41–56. doi:10.1007/s100210000009
Wang, S., Zhuang, Q., Lähteenoja, O., Draper, F., and Cadillo-Quiroz, H. (2018). Potential shift from a carbon sink to a source in Amazonian peatlands under a changing climate. Proc. Natl. Acad. Sci. U. S. A. 115, 12407–12412. doi:10.1073/pnas.1801317115 |
Warren, M. W., Kauffman, J. B., Murdiyarso, D., Anshari, G., Hergoualc'h, K., Kurnianto, S., et al. (2012). A cost-efficient method to assess carbon stocks in tropical peat soil. Biogeosciences 9, 4477–4485. doi:10.5194/bg-9-4477-2012
Whalen, S. C., Reeburgh, W. S., and Sandbeck, K. A. (1990). Rapid methane oxidation in a landfill cover soil. Appl. Environ. Microbiol. 56, 3405–3411. doi:10.1128/aem.56.11.3405-3411.1990 |
Wong, G. X., Hirata, R., Hirano, T., Kiew, F., Aeries, E. B., Musin, K. K., et al. (2018). Micrometeorological measurement of methane flux above a tropical peat swamp forest. Agric. For. Meteorol. 256–257, 353–361. doi:10.1016/j.agrformet.2018.03.025 |
Wrage, N., Velthof, G. L., van Beusichem, M. L., and Oenema, O. (2001). Role of nitrifier denitrification in the production of nitrous oxide. Soil Biol. Biochem. 33, 1723–1732. doi:10.1016/s0038-0717(01)00096-7
Wright, E. L., Black, C. R., Turner, B. L., and Sjögersten, S. (2013). Environmental controls of temporal and spatial variability in CO2 and CH4 fluxes in a neotropical peatland. Glob. Chang Biol. 19, 3775–3789. doi:10.1111/gcb.12330 |
Keywords: GHG emissions, peat swamp forest, oil palm, peatland, methane, nitrous oxide, Indonesia, tropical
Citation: Swails E, Hergoualc’h K, Verchot L, Novita N and Lawrence D (2021) Spatio-Temporal Variability of Peat CH4 and N2O Fluxes and Their Contribution to Peat GHG Budgets in Indonesian Forests and Oil Palm Plantations. Front. Environ. Sci. 9:617828. doi: 10.3389/fenvs.2021.617828
Received: 16 October 2020; Accepted: 04 February 2021;
Published: 18 March 2021.
Edited by:
Björn Klöve, University of Oulu, FinlandReviewed by:
Fumiaki Takakai, Akita Prefectural University, JapanNa Li, Chinese Academy of Science, China
Copyright © 2021 Swails, Hergoualc’h, Verchot, Novita and Lawrence. This is an open-access article distributed under the terms of the Creative Commons Attribution License (CC BY). The use, distribution or reproduction in other forums is permitted, provided the original author(s) and the copyright owner(s) are credited and that the original publication in this journal is cited, in accordance with accepted academic practice. No use, distribution or reproduction is permitted which does not comply with these terms.
*Correspondence: Erin Swails, ees8rg@virginia.edu