- 1Soil Science and Soil Protection, Martin Luther University Halle-Wittenberg, Halle, Germany
- 2Chair of Soil Science, Technical University of Munich, Freising, Germany
- 3Institute of Soil Science, Leibniz Universität Hannover, Hannover, Germany
Water-extractable organic carbon (WEOC) is considered as the most important carbon (C) source for denitrifying organisms, but the contribution of individual organic matter (OM) fractions (i.e., particulate (POM) and mineral-associated (MOM)) to its release and, thus, to denitrification remains unresolved. Here we tested short-time effects of POM and MOM on potential denitrification and estimated the contribution of POM- and MOM-derived WEOC to denitrification and CO2 production of three agricultural topsoils. Suspensions of bulk soils with and without addition of soil-derived POM or MOM were incubated for 24 h under anoxic conditions. Acetylene inhibition was used to determine the potential denitrification and respective product ratio at constant nitrate supply. Normalized to added OC, effects of POM on CO2 production, total denitrification, and its product ratios were much stronger than those of MOM. While the addition of OM generally increased the (N2O + N2)-N/CO2-C ratio, the N2O/(N2O + N2) ratio changed differently depending on the soil. Gas emissions and the respective shares of initial WEOC were then used to estimate the contribution of POM and MOM-derived WEOC to total CO2, N2O, and N2O + N2 production. Water-extractable OC derived from POM accounted for 53–85% of total denitrification and WEOC released from MOM accounted for 15–47%. Total gas emissions from bulk soils were partly over- or underestimated, mainly due to nonproportional responses of denitrification to the addition of individual OM fractions. Our findings show that MOM plays a role in providing organic substrates during denitrification but is generally less dominant than POM. We conclude that the denitrification potential of soils is not predictable based on the C distribution over POM and MOM alone. Instead, the source strength of POM and MOM for WEOC plus the WEOC’s quality turned out as the most decisive determinants of potential denitrification.
Introduction
Agricultural soils vary in terms of the content and composition of organic matter (OM), which may affect their denitrification potential. Denitrification, the microbial reduction of nitrate (NO3−) via a series of enzymatic steps to NO2−, NO, N2O, and finally N2 (Philippot et al., 2007), results in net ecosystem losses of nitrogen (N) and release of the greenhouse gases N2O and CO2. Considering the role of N2O in atmospheric processes, understanding the factors controlling denitrification and its N2O/N2 product ratio is crucial for developing strategies to minimize emissions of greenhouse gases. In soil, denitrification mainly occurs in anoxic microhabitats (ˈhot spotsˈ) where electron acceptors, nitrogen oxides (e.g., NO2−, NO3−), and electron donors, organic carbon (OC), are sufficiently bioavailable (e.g., Groffman et al., 2009). Despite OM is known to serve as an electron donor, the role of OM quality in shaping the spatial and temporal patterns of denitrification is still rather unknown. Previous studies have shown that addition of fresh plant biomass or well-defined low-molecular-weight compounds affects denitrification rates, product ratios, and denitrifier populations (e.g., Beauchamp et al., 1989; Miller et al., 2008; Palmer et al., 2012). When comparing different C substances, soluble low-molecular-weight compounds are much more effective in promoting denitrification and related bacterial populations (e.g., organic acids, glycerol ≫ glucose, methanol) than insoluble polymers, such as cellulose and especially lignin (e.g., Valera and Alexander, 1961; deCatanzaro and Beauchamp, 1985; Rashid and Schaefer, 1988; Akunna et al., 1993). In addition, Henry et al. (2008) showed that artificial root exudates with higher proportion of sugars (80%) caused a much lower N2O/(N2O + N2) product ratio than a solution rich in organic acids and amino acids (40% sugars). Despite evidence of large differences in release and biodegradability of water-extractable OM (WEOM) (e.g., Don and Kalbitz, 2005; Kalbitz et al., 2005; Mastný et al., 2018), much less information is available on the effects of more complex soil OM fractions, such as particulate and mineral-associated OM (POM, MOM) (Lavallee et al., 2020).
Water-extractable OM is considered to be readily decomposable and most effective in promoting denitrification (e.g., Bremner and Shaw, 1958; Burford and Bremner, 1975), but its chemical composition can vary greatly. It may comprise low-molecular-weight C compounds, such as sugars, amino acids, and proteins, but also high-molecular-weight components, such as microbial-derived extracellular polymeric or humic substances (Kalbitz et al., 2003a; Marschner and Kalbitz, 2003). While WEOM leached from fresh plant residues and bacterial extracellular polymeric substances is rich in hydrophilic components, such as polysaccharides, WEOM derived from well-humified forest floor layers typically is enriched in phenolic compounds, such as lignin or lignocellulose, and, thus, is much less biodegradable (Kalbitz et al., 2003a, Kalbitz et al., 2003b; Marschner and Kalbitz, 2003). In previous studies, we have shown that water-extractable OC (WEOC) is a straightforward indicator of the denitrification potential of soils, well related to the chemical composition of the source materials, such as POM and fresh plant residues (Surey et al., 2020a; Surey et al., 2020b). A substantial role in denitrification of POM with adhering microorganisms has been also suggested by Parkin (1987) and Parry et al. (2000). Further, Gaillard et al. (2003) showed that soluble C from POM enters the adjacent soil (several mm) and fuels microbial processes. Consequently, POM-derived WEOC could be of crucial importance for the stimulation of denitrification processes. Nevertheless, MOM comprises up to 90% of total soil OC, particularly in subsoil horizons (Kögel-Knabner et al., 2008), but is usually much less bioavailable than POM (e.g., Swanston et al., 2002). Mineral-organic associations are basically formed by adsorption and precipitation reactions where dissolved OM reacts with mineral surfaces (e.g., Kaiser and Guggenberger, 2000; Kleber et al., 2015). The amount and composition of MOM in a given soil is strongly affected by the composition of dissolved OM as well as the presence and reactivity of pedogenic minerals (Kaiser and Guggenberger, 2000; Mikutta et al., 2009; Mikutta et al., 2010). Thus, MOM represents a continuum of mineral-bound C substances of varying availability and degradability (e.g., Mikutta et al., 2007). Especially in topsoils with high OC inputs and mineral C loadings, OC can repeatedly be displaced and desorbed from mineral surfaces (Leinemann et al., 2016; Liebmann et al., 2020). Consequently, MOM is a potential source of OC fueling denitrification, with its contribution probably depending on the amount and quality of WEOC.
In summary, it is currently unknown to what extent the POM and MOM fractions provide readily available OC, i.e., WEOC, for denitrification reactions. We expect that not only the potential denitrification but also its product ratios depend on the release of WEOC from POM and MOM. We hypothesize that WEOC derived from MOM contributes to potential denitrification but is less effective in driving denitrification than POM-derived WEOC. Moreover, we hypothesize that the contribution of POM and MOM to potential denitrification of bulk soils depends less on their mass proportions but can instead be estimated from the respective WEOC contents. To address our research questions, we isolated POM and MOM fractions from three agricultural soils and determined their WEOC contents. The bulk soils were subsequently amended with isolated POM and MOM fractions, and the potential denitrification and N2O/(N2O + N2) product ratios were measured over a period of 24 h.
Materials and Methods
Sampling Sites and Soil Characteristics
In summer 2016, we collected topsoil (0–20 cm) material at three different sites in Germany (Table 1). The first soil, a Haplic Luvisol with silty loam texture and pH (CaCl2) of 6.7 under arable management, was from a long-term trial at Höhere Landbauschule Rotthalmünster, approximately 150 km east of Munich (latitude N48°21′, longitude E13°11′, elevation 360 m a.s.l.; Yamashita et al., 2006). In the following, it is referred to as Rotthalmünster (Ro) soil. A clayey grassland soil (Fluvic Gleysol) with a pH (CaCl2) of 5.7, referred to as Giessen (Gi) soil, was collected at the Environmental Monitoring and Climate Impact Research Station Linden near Giessen (latitude N50°32′, longitude E8°41.3′, elevation 172 m a.s.l.; Jäger et al., 2003). The third soil was taken approximately 1.5 km south of Fuhrberg (latitude N52°32.9′, longitude E9°50.6′, elevation 43 m a.s.l.; Böttcher et al., 1999). The sandy arable soil with a pH (CaCl2) of 4.8 is classified as a Gleyic Podzol and referred to as Fuhrberg (Fu) soil. Soil samples were air-dried, and large plant particles and stones were removed by sieving to <2 mm. The clay fraction of the three soils had a comparable clay mineral composition, dominated by illite and kaolinite, with a substantial contribution of vermiculite in the Ro soil (Table 1; Supplementary Figure S1). Contents of pedogenic Fe oxides, expressed as dithionite-citrate-bicarbonate-extractable Fe (Fed), varied strongly among soils, following the order: Ro > Gi > Fu (Table 1).
Isolation of Organic Matter Fractions
Soils were fractionated according to density using sodium polytungstate solution adjusted to 1.6 g cm−3 as described in Surey et al. (2020a) to estimate the distribution of soil OC over POM and MOM fractions. The fraction <1.6 g cm−3 comprises free POM (i.e., not or only weakly associated with mineral particles; fPOM) and occluded POM present within water-stable aggregates (oPOM); the fraction >1.6 g cm−3 represents MOM. Since density fractionation might release soluble C from plant residues, we used electrostatic attraction (Kaiser et al., 2009) to recover unaltered POM material for the incubation experiments. Before separation, soil aggregates of the three study soils were gently crushed with mortar and pestle. Subsequently, POM was collected using a plastic stick electrostatically charged by rubbing; washing was avoided to leave the amounts of WEOC unaltered.
Chemical Characterization of Organic Matter Fractions
Prior to analyses and incubation experiments, POM materials were ground to <1 mm using an impact mill (Rekord A, Gebr. Jehmlich GmbH, Nossen, Germany). The MOM fractions were analyzed for total C and N (TN) using a Vario Max Cube; analyses of POM were carried out with a Vario EL cube (Elementar Analysensysteme GmbH, Langenselbold, Germany). Inorganic C was not detectable (Vario Max Cube, Elementar); therefore, total C was assumed to represent OC. Water-extractable OC and total N (WETN) were determined by suspending either 5 g of the MOM fractions or 500 mg of POM in 25 ml ultrapure water and shaking for 1 h. After centrifugation (only necessary for MOM) at 3,000 × g for 10 min (Cryofuge 8500i, Thermo Fisher Scientific, Waltham, MA, United States), the supernatants were passed through 0.45-µm membrane filters (Supor-450, Pall Cooperation, New York, NY, United States). All WEOM solutions were analyzed for dissolved OC (DOC) and TN using a multi N/C 3100 (Analytik Jena AG, Jena, Germany). Concentrations of Nmin (NO3− and NH4+) were determined using a Continuous-Flow Analyzer (ScanPlus, Skalar Analytical B.V., Breda, Netherlands). Water-extractable organic N (WEON) or ON was calculated by (WE)ON = (WE)TN ‒ Nmin. All reported (WE)OC and (WE)ON contents refer to dry mass determined at 105°C. The specific UV absorbance at 280 nm (an estimate of aromatic compounds) of the WEOM was determined using a photometer (SPECORD 210 PLUS, Analytik Jena AG) by normalizing the absorbance to the OC concentration.
Before analysis of POM and MOM fractions by solid-state 13C crosspolarization magic angle spinning NMR spectroscopy (13C-CPMAS NMR spectroscopy) with an Avance III 200 spectrometer (Bruker BioSpin GmbH, Karlsruhe, Germany), both fractions were ground with a vibratory disc mill (RS 100, Retsch GmbH, Haan, Germany). Samples were placed into a 7-mm zirconia rotor that was spun at 6.8 kHz at a ‘magic angle’ of 54.74°. Contact time was 1 ms and the recycle delay time was set to 0.4 s. The spectra were processed with 100 Hz line broadening, phase adjusted, and baseline corrected; no spinning side bands appeared in the spectra. Peaks were assigned to four integration areas: 10–45 ppm (alkyl C), 45–110 ppm (O/N-alkyl C), 110–160 ppm (aromatic C), and 160–220 ppm (carboxylic/carbonyl C); spectra are shown in Supplementary Figure S2.
Incubation, Gas Measurements, and Calculations
Soil samples were rewetted to 50% water holding capacity and aerobically preincubated for two weeks at 20°C in the dark to stimulate microbial growth before anoxic incubation. Subsequently, 10 g (dry mass) of soil were placed into 250 ml glass infusion bottles, either without (control/bulk soil) or with addition of soil-derived OM fractions (POM or MOM). The OM additions amounted to 2 g C kg−1 dry soil and increased the total OC of Ro, Gi, and Fu soil by 15, 7, and 8%, respectively. Then, 50 ml of a KNO3 solution (50 mg NO3−-N kg−1 dry soil) were added and well mixed with the soil materials to achieve homogeneous soil suspensions (soil/water ratio = 1/5, w/v) and to avoid nitrate limitation during incubation. Using soil suspensions instead of repacked soil minimized accessibility limitations, and, thus, allowed for comparing potential effects of OM fractions. The incubation bottles were sealed with a bromine-butyl-rubber stopper and crimped with an aluminum cap (32 mm; Chroma Globe GbR, Kreuznau, Germany). An O2-free atmosphere for anoxic incubations was obtained by evacuating (<250 mbar) and then flushing the bottles three times with Helium (He) gas (99.999%, Air Liquide, Düsseldorf, Germany); the final pressure was about 1,025 mbar. Soil suspensions were horizontally shaken during incubation at 20°C in the dark for 24 h, as this study focused on short-time effects in a typical ‘hot spot–hot moment situation’ (McClain et al., 2003). All incubations were carried out in triplicate.
As described in Surey et al. (2020b), gas samples (18 ml) were taken after 0, 2, 4, 6, 8, and 24 h, using a gastight syringe (25 ml, 25MDR-LL-GT; SGE Analytical Science Pty. Ltd., Ringwood, VIC, Australia), equipped with a push button valve (Luer Lock; SGE Analytical Science) and a 0.7-mm ID cannula (Sterican G26, 25 mm; B. Braun AG, Melsungen, Germany), and then transferred into preevacuated (90 mbar residual pressure, flushed with He) 12 ml Exetainer vials, which were sealed with a double septum cap (IVAVC329; IVA Analysentechnik e.k., Meerbusch, Germany). This resulted in an overpressure of >200 mbar in the Exetainer vials, which was necessary to avoid contamination with air during storage and for measuring gas concentrations. To avoid low pressure in the incubation bottles, 18 ml He (∼1 bar) were injected after gas sampling, resulting in constant absolute pressure of ∼1,025 mbar during incubation. The absolute pressure in the bottles was measured before and after gas sampling as well as after He injection, using a GMSD 2 BA-K31-L01 pressure sensor coupled with a Greisinger GMH 3151 reader (GSG Geologie-Service GmbH, Würzburg, Germany). Gas samples were analyzed for CO2 and N2O concentrations on a custom-tailored gas chromatography system by Chromtech (Bad Camberg, Germany), using an Agilent HP 7890B GC as basis (Surey et al., 2020a).
Cumulative emissions of gases represent the sum of produced amounts, i.e., the detected gas mass in the headspace at time point tx minus the mass at time point tx–1, considering the removed gas amount during each gas sampling. Gas masses in suspension were calculated by using the respective Henry’s law constant, volume of solution, headspace pressure, and gas concentration; pH was considered for estimating the different CO2 species. For setting up the acetylene (C2H2) inhibition technique (Yoshinari and Knowles, 1976), the entire incubation procedure described above was also carried out with injection of 30 ml of C2H2 (99.6%; Air Liquide) in exchange for 30 ml He, resulting in an initial C2H2 concentration of ∼10% (v/v). An C2H2 concentration of >5% (v/v) was expected to be maintained during the 24 h of incubation (Yeomans and Beauchamp, 1978; Terry and Duxbury, 1985). The amount of N2O in presence of C2H2 represented the total denitrification products (N2O + N2). Consequently, the ratio of N2O measured without and with C2H2 addition is an estimate of the molar N2O-N/(N2O + N2)-N product ratio. Cumulative amounts of N2O in presence of C2H2 and of CO2 in incubations without C2H2 were used to determine the molar (N2O + N2)-N/CO2-C ratio, which reveals the contribution of denitrification to total CO2 production. According to the stoichiometry of denitrification (Ottow, 2011), ratios between 0.8 and 1.0 indicate that the CO2 production is exclusively linked to denitrification reactions. In order to test for the microbial use efficiency of the WEOC in each POM and MOM fraction, we calculated the percentage increase/decrease (Δ%) in cumulative gas emissions per Δ% initial WEOC induced by the addition of OM fractions to bulk soil. This allows for comparison of all OM fractions across the different soils. Potential problems of the acetylene inhibition technique, i.e., underestimated N2 production due to acetylene inhibition of nitrification and incomplete inhibition of N2O reduction caused by either low NO3− concentrations or acetylene diffusion effects (Knowles, 1990; Almaraz et al., 2020), can be excluded for the incubation conditions used (anoxic, excess NO3−, soil suspension).
Estimation of Contributions of POM and MOM to Total Gas Production of Bulk Soils
Contributions of POM and MOM to total gas emission of bulk soils were calculated using the determined gas emissions from soils with added soil-derived OM fractions (POM and MOM). Based on the idea that gas production rates were mainly controlled by the composition of WEOC, we estimated the contribution of the OM fractions as follows. We assumed that POM-induced gas emission from amended soil (EPa) was equal to that induced by the POM originally present in the bulk soil (EPb), considering the respective POM/MOM ratio,
where
The same applies to the MOM-induced emission from the bulk soil (EMb), which can be calculated by
As in Eq. 2, EMa represents the emission of MOM-amended bulk soil; amounts of MOM-derived WEOC in the bulk soil and added MOM-derived WEOC are denoted by
Since air-drying of soil samples increases the release of WEOC (Kaiser et al., 2001), WEOC contents of bulk soils were determined for preincubated soils (soil/water ratio = 1/2, w/v; only stirred for 1 min; Table 2). Also, the WEOC contents of dried MOM fractions were extremely high (Table 3). Using these figures resulted in estimates of gas emissions exceeding those actually measured for bulk soils without OM addition by far (Eq. 4). Therefore, the contents of MOM-derived WEOC in bulk soil (Mb) were estimated by total WEOC of the bulk soil minus the measured POM-derived WEOC in the bulk soil (Pb). Here, Pb was, in turn, determined by multiplying the proportion of WEOC in POM-OC (for POM obtained by electrostatic attraction) with the respective total POM-OC content of the soil.
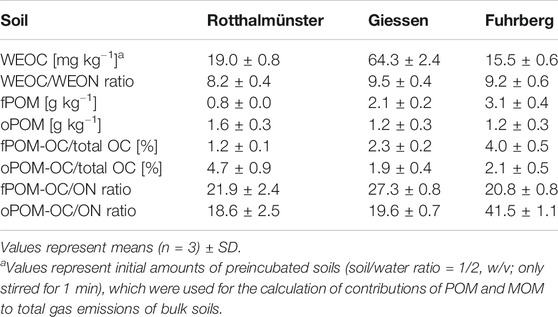
TABLE 2. Content of water-extractable organic carbon (WEOC) and the WEOC/WEON ratio as well as the content of free and occluded particulate organic matter (fPOM, oPOM), and their proportions in total OC and their OC/ON ratios for soils used for incubation experiments.
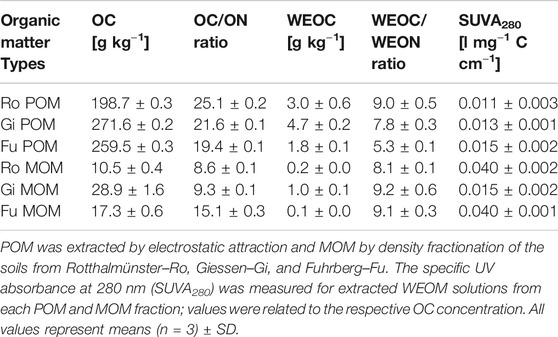
TABLE 3. Content of organic carbon (OC) and water-extractable OC (WEOC) as well as the OC/ON and WEOC/WEON ratios of particulate and mineral-associated organic matter (POM, MOM) fractions used for incubation experiments.
Statistical Evaluation
Basic statistical analyses were performed using Sigma Plot 11.0 (Systat Software Inc., Erkrath, Germany). One-way ANOVA with incubation treatment or time (after 2, 8, and 24 h) as independent variable followed by the Tukey HSD test was used for testing of differences in molar N2O/(N2O + N2) and (N2O + N2)-N/CO2-C ratios.
Results
Distribution and Chemical Composition of Soil Organic Matter Fractions
Despite the fact that the Ro soil contained the lowest amounts of total POM (fPOM + oPOM) and total OC, the content of WEOC was about 23% higher than that of the Fu soil (Tables 1, 2). While the Fu and Gi soils had more fPOM than oPOM, oPOM dominated in the Ro soil (Table 2). The Gi soil had the highest contents of total OC and WEOC but the lowest contribution of POM to total OC (4.2%). Soil WEOC contents were not related to total OC contents or proportions of POM fractions. The WEOC/WEON ratios of bulk soils differed little (8.2–9.5). Except for the higher OC/ON ratios of fPOM in the Gi soil (27), and especially of oPOM in the Fu soil (42), the OC/ON ratios of POM fractions were fairly similar (19–22). The OC/ON ratios of POM fractions showed no relations to the WEOC contents and WEOC/WEON ratios of bulk soils.
Likewise, WEOC contents of POM and MOM fractions used for incubation experiments were not linked to their OC/ON ratios and OC contents (Table 3). WEOC from the Fu POM and MOM fractions accounted for only 0.6–0.7% of their total OC contents, while it was 1.5–1.7 and 2.3–3.4% for POM and MOM of the other two soils, respectively. The WEOC/WEON ratio of Fu POM was remarkably lower (5.3) than that of the other two POM fractions (7.8–9.0), while WEOC/WEON ratios of MOM fractions were fairly similar (8.1–9.2). Also, the specific UV absorbance of WEOM from the Gi MOM fraction was 63% less than that from Ro and Fu MOM (Table 3). The POM and MOM fractions isolated from the Fu soil contained significantly more alkyl C than the other OM fractions, while proportions of O/N-alkyl C and aryl C were, in turn, smaller (Table 4). The distribution of C species across OM fractions, especially POM, from the Ro and Gi soil, differed a little. The more Fe oxide-rich Ro soil contained the highest proportion of carboxylic/carbonyl C in the MOM fraction; nevertheless, the OC contents of MOM fractions did not coincide with Fed contents (Tables 1, 3, and 4).
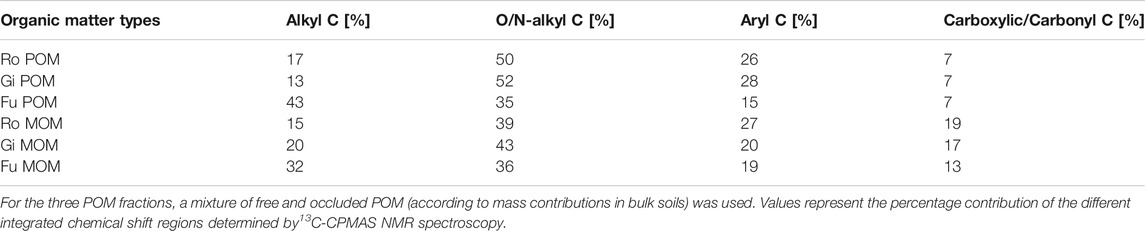
TABLE 4. Distribution of C species in particulate and mineral-associated organic matter (POM, MOM) fractions obtained by density fractionation from soils collected at Rotthalmünster–Ro, Giessen–Gi, and Fuhrberg–Fu.
Effect of Organic Matter Fractions on Potential Denitrification and CO2 Production
Addition of MOM resulted in increases in total denitrification (by about 55%) and cumulative CO2 production (by 25–54%) for all three soils (Figures 1A,C). Addition of POM to the Gi soil caused about 93% more N2O + N2 and 33% more CO2 emissions; for the Ro und Fu soils, the emissions of N2O + N2 increased by 350% and those of CO2 by 150%. Adding POM to the Ro soil caused larger N2O + N2 emissions after 24 h than to the more OC-rich Gi soil (14.7 ± 0.0 and 12.7 ± 0.3 mg N kg−1 dry soil, respectively). Cumulative CO2 emissions after 24 h were 22–28% or 37–63% of initial WEOC in soils with either MOM or POM addition, respectively.
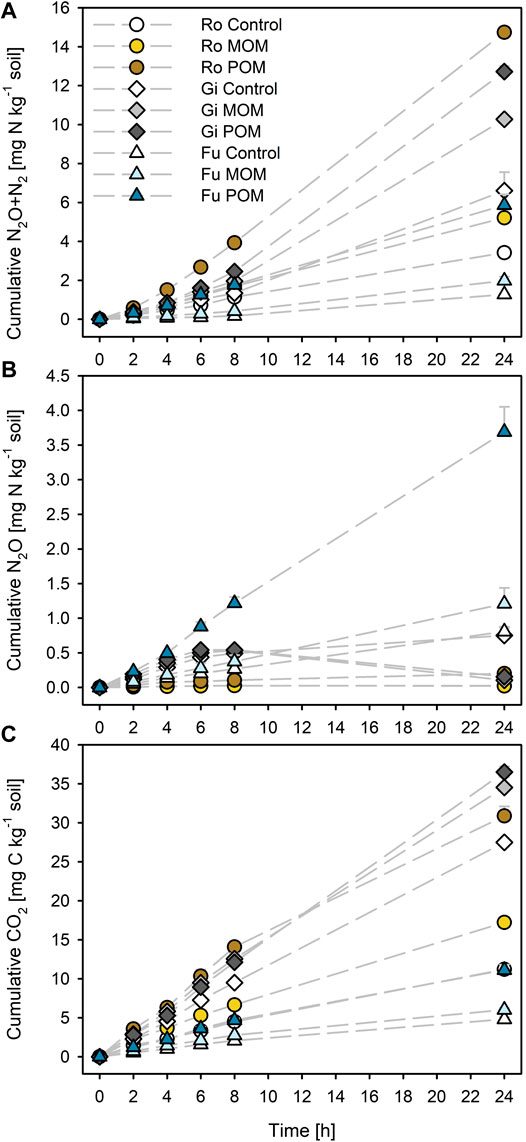
FIGURE 1. Cumulative (A) N2O + N2, (B) N2O, and (C) CO2 production during anoxic incubation at 20°C of the soils from Rotthalmünster–Ro, Giessen–Gi, and Fuhrberg–Fu without (control) and with addition of soil-derived particulate and mineral-associated organic matter (POM, MOM). Error bars represent SD of means (n = 3). All incubations received initial KNO3 additions of 50 mg NO3−-N kg−1 dry soil.
The cumulative N2O release from the Ro soil was generally low and decreased slightly after 8 h for the control and MOM addition but not for POM addition (Figure 1B). For the Gi soil, POM and MOM additions caused drastic decreases in cumulative N2O after 8 h as compared with the soil without amendment. By contrast, the cumulative N2O emission from the Fu soil increased linearly during the entire incubation time, irrespective of OM addition. The Fu soil amended with POM generally released the highest amounts of N2O.
The effect on cumulative N2O + N2 and CO2 emissions (Δ%) was generally highest for the Fu POM when normalized to respective percentage increase in initial WEOC caused by OM additions (Figures 2A,C). Addition of POM to the Ro soil caused the maximum effect on N2O emission after 24 h (about 5% N2O-N per 1% initial WEOC increase; Figure 2B). Addition of POM to the Gi soil caused higher effects on N2O-N per Δ% initial WEOC than all MOM fractions after 2 h but declined during incubation and was even negative after 24 h, similar as for the MOM addition. As with POM, the effect of MOM on cumulative N2O + N2 and CO2 emissions per Δ% initial WEOC was highest for the Fu soil; it was similar to that of Gi POM during the first 8 h (Figures 2A,C).
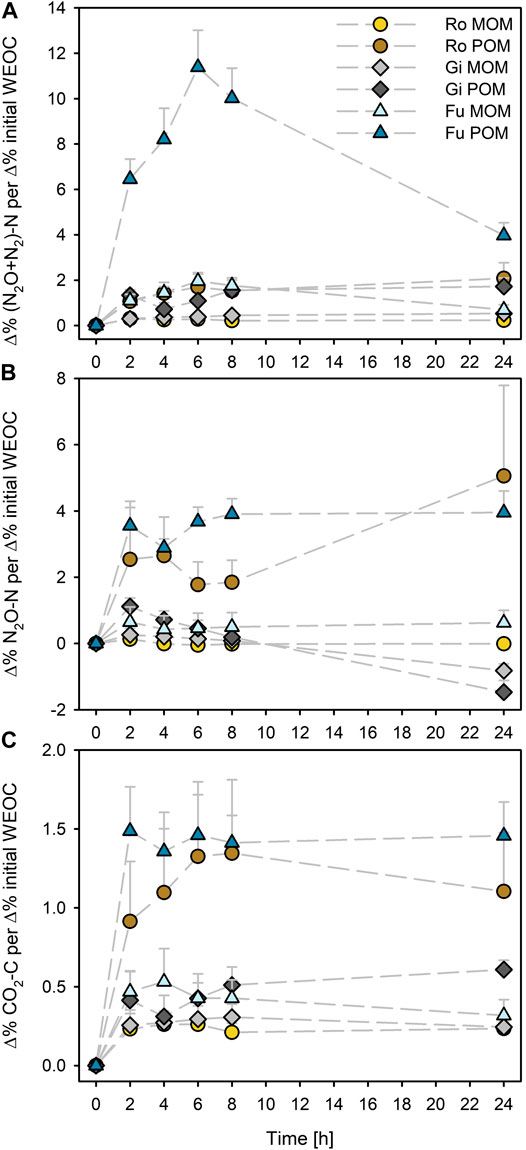
FIGURE 2. Percentage effect of additions of soil-derived particulate and mineral-associated organic matter (POM, MOM) on cumulative (A) N2O + N2, (B) N2O, and (C) CO2 production normalized to percentage of initial WEOC increases compared to the control (without OM addition) during anoxic incubation at 20°C of the soils from Rotthalmünster–Ro, Giessen–Gi, and Fuhrberg–Fu. Error bars represent SD of means (n = 3).
Effect of Organic Matter Fractions on Denitrification Product Ratios
Compared with the untreated control samples, additions of OM resulted in lower shares of N2O for the Gi and Fu soils but not for the Ro soil. Irrespective of OM addition, the release of N2O dominated in the Fu soil, resulting in the highest molar N2O/(N2O + N2) ratios (Table 5). In the Fu soil without OM addition, no N2 production occurred within the first 8 h, as indicated by the molar N2O/(N2O + N2) ratio of 1. Across soils and treatments, the molar N2O/(N2O + N2) ratios decreased and the molar (N2O + N2)-N/CO2-C ratio increased over incubation time (Table 5). Addition of POM caused high molar (N2O + N2)-N/CO2-C ratios, being highest for the Ro and Fu soils (0.41 and 0.45, respectively); the effects of MOM additions were negligible.
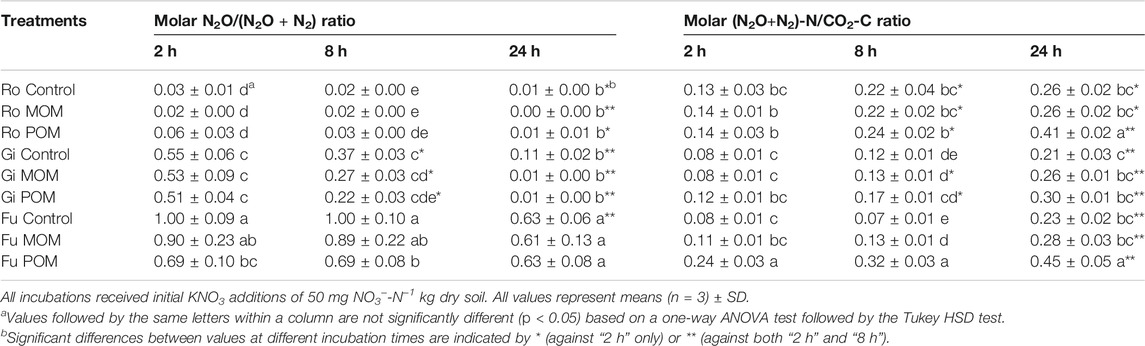
TABLE 5. Molar N2O/(N2O + N2) and (N2O + N2)-N/CO2-C ratios after 2, 8, and 24 h of anoxic incubation at 20°C of soils from Rotthalmünster–Ro, Giessen–Gi, and Fuhrberg–Fu without (control) and with addition of soil-derived particulate or mineral-associated organic matter (POM, MOM).
Contribution of POM and MOM to Total Gas Emissions From Bulk Soils
Based on our calculations, about 63, 32, and 69% of WEOC of the Ro, Gi, and Fu soil derived from POM, respectively, the remaining share was from the MOM. Accordingly, the calculated contribution of POM to total cumulative gas emissions from bulk soils varied widely (Figures 3A–I). The contribution of POM-derived WEOC to total N2O + N2, N2O, and CO2 emissions was dominant in the Ro (81, 91, and 79%, respectively) and Fu soil (85, 82, and 72%, respectively) but was almost equal to MOM-derived WEOC in the Gi soil (48–53%). The contribution of POM-derived WEOC to CO2 emissions was smaller than to the production of nitrogenous gases in all three soils. The relative contribution of OM fractions differed only slightly with incubation time, but percentage differences between calculated (sum of POM- and MOM-induced gas emissions) and measured total bulk soil emissions during incubation varied strongly for N2O for all three soils (−87 to +169%), and especially for N2O + N2 for the Fu soil (+142–460%). Except for the Fu soil, the calculated cumulative N2O + N2, N2O, and CO2 emissions within the first 8 h were well in line with those actually measured for the bulk soils (Figures 3A–I). Lowest percentage differences with little variations over the entire incubation period occurred for CO2 (−9 to +10%) for the Ro and Gi soils, and for N2O + N2 (+7–33%) for the Gi soil.
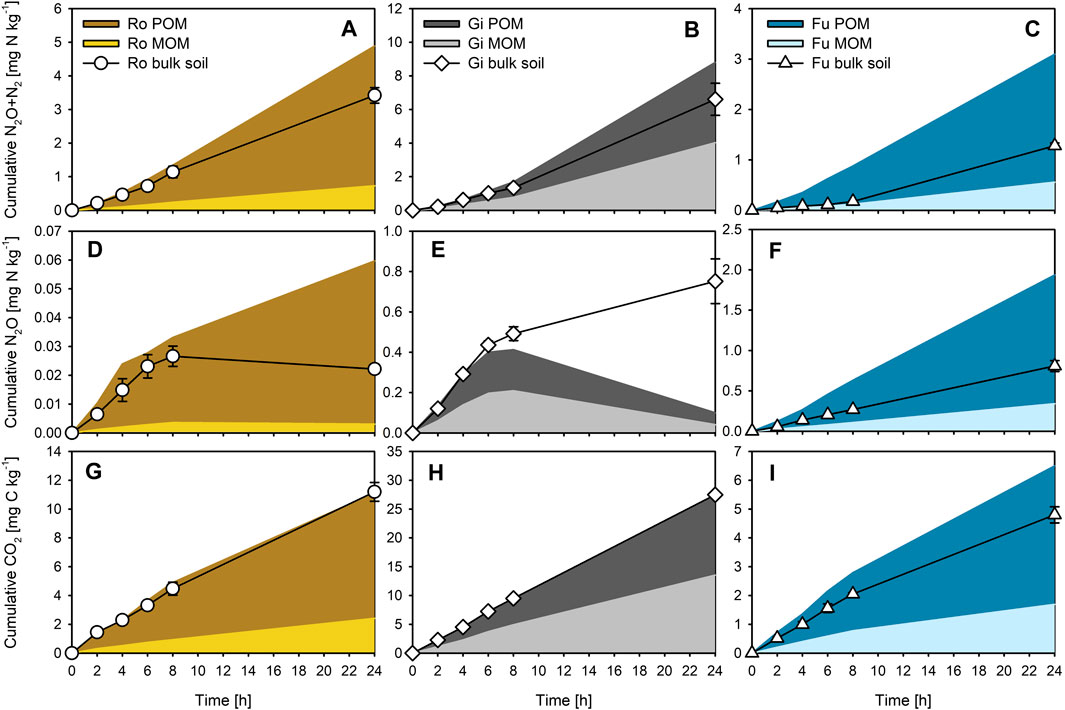
FIGURE 3. Estimated cumulative (A–C) N2O + N2, (D–F) N2O, and (G–I) CO2 production induced by WEOC derived from particulate and mineral-associated organic matter (POM, MOM), and measured cumulative production of the respective gases for the bulk soils, during anoxic incubation at 20°C of the soils from Rotthalmünster–Ro, Giessen–Gi, and Fuhrberg–Fu. Error bars represent SD of means (n = 3).
Discussion
Effect of Organic Matter Fractions on Potential Denitrification and CO2 Production
The incubation experiment revealed that short-time effects of POM on potential denitrification and CO2 production are much stronger than those of MOM when normalized to C equivalents (Figures 1A–C). Thus, POM clearly has a higher potential to fuel denitrification than MOM. This is well in line with previous studies showing that MOM comprises mainly less bioavailable C compounds (e.g., Kalbitz et al., 2005; Liebmann et al., 2020). Correspondingly, we found that only 22–28% of the initial WEOC in soils with MOM addition was emitted as CO2 within 24 h, while 37–63% of initial WEOC was mineralized in soils with POM addition. The results, thus, support the assumption that POM is a major driver of denitrification in soil (Parkin, 1987; Parry et al., 2000; Surey et al., 2020a). Nevertheless, all fractions contribute to CO2 and N gas emissions, largely according to their different composition and the related WEOC source strengths (Table 3).
The effect of POM addition on cumulative gas emissions (Δ%) was highest for the Fu soil when normalized to the induced increases in WEOC (Figures 2A–C). This could be due to high quality of WEOM, as indicated by the narrow WEOC/WEON ratio of 5.3 (Table 3). Such a narrow C/N ratio suggests the presence of a large proportion of microbial-derived OM. The C/N ratio of microbial biomass usually varies in the range of 6–9 (e.g., Cleveland and Liptzin, 2007). Due to the small WEOC contents of the Fu POM, rapid depletion of such readily available C compounds could be the reason for the declining effect of added Fu POM on cumulative N2O + N2 already after 6 h, when the maximum was reached (Figure 2B). Thus, as already shown in a previous study (Surey et al., 2020b), potential denitrification depends not only on the plant residues’ content of WEOM but also on its chemical composition (e.g., C/N ratio, aromaticity, shares of sugars, and organic acids). Consequently, when predicting denitrification potentials of different soils, the quantity of WEOC as well as its quality needs to be considered. Since the Ro POM was also more efficiently used than the other OM fractions (Figures 2B,C), we suggest that microorganisms in WEOC-poor soils are better adapted to POM-derived WEOM.
In summary, as hypothesized, the contribution of POM to potential denitrification is much higher than that of MOM (based on C equivalents). Also, the stronger percentage effects of added POM and its larger contents of readily mineralizable WEOC than of MOM underline the importance of POM-derived WEOM for the potential denitrification in soils.
Effect of Organic Matter Fractions on Denitrification Product Ratios
Previous studies have shown that not only the rate of denitrification but also the N2O/(N2O + N2) ratio is strongly affected by environmental conditions and soil properties (e.g., Bremner and Shaw, 1958; Knowles, 1982). Lower pH values tend to cause decreased denitrification rates but larger molar N2O/(N2O + N2) ratios (Čuhel et al., 2010). Therefore, differences in soil pH (Table 1) could explain that the molar N2O/(N2O + N2) ratio was highest for the Fu soil. Another important factor influencing the share of N2O is the ratio of bioavailable OC to NO3−-N, as demonstrated by Senbayram et al. (2012). The effects of POM and MOM additions on N2O production (Figures 1A, 2B) and molar N2O/(N2O + N2) ratio (Table 5) were well in line with this finding. Higher concentrations of WEOC at the beginning of the incubation of the OM-amended acidic Fu soil might have facilitated complete denitrification to N2, resulting in lower molar N2O/(N2O + N2) ratios than for the Fu soil without OM addition, despite having high initial NO3− concentrations. The addition of POM and MOM to the Gi soil resulted in the molar N2O/(N2O + N2) ratios declining to about zero by the end of incubation. Consequently, the OC-rich Gi bulk soil (high DOC/NO3−-N ratio) without OM addition released more N2O within 24 h than with additions of POM and MOM (Figure 1B). By contrast, the addition of OM fractions to the OC-poor Ro soil (low DOC/NO3−-N ratio) increased the share of N2O, especially at the beginning of incubation. Therefore, in situations where NO3− is not limited and O2 absent (‘hot spot/hot moment’), OM fractions releasing WEOC control the total denitrification as well as its molar N2O/(N2O + N2) ratio. In accordance with effects discussed in the previous section, additions of soil-derived POM resulted also in higher molar (N2O + N2)-N/CO2-C ratios than MOM additions, the ratio being maximum for the Fu soil with POM addition (Table 5). This supports the idea that the product ratios of denitrification respond to the chemical composition of WEOM (Surey et al., 2020b) and again indicates a higher quality and use efficiency of WEOM derived from plant residues than from MOM.
As hypothesized, not only the rate of denitrification but also the molar N2O/(N2O + N2) and (N2O + N2)-N/CO2-C product ratios are affected by the release of WEOM from MOM and especially from POM. Thus, WEOM in combination with the soil pH and available NO3−-N determines the relative contribution of the different gas products.
Estimation of Potential Denitrification Based on Functional Organic Matter Fractions
Apart from overestimations discussed below, our calculations suggest that the gas emissions from the OC-poor and silty Ro soil, as well as from the sandy Fu soil, were largely fueled by POM-derived WEOC (Figures 3A,C,D,F,G,I). This accords with findings of N2O + N2 emissions from silty soils (Haplic Chernozem; 1.6–2.3% total OC) of a long-term fertilization experiment being closely related to total WEOC (23–40 mg kg−1), which again related well to shares of POM (Surey et al., 2020a). This might also explain why denitrification ‘hot spots’ are often linked to POM (e.g., Parkin, 1987; Parry et al., 2000). Further, the lower contribution of POM-derived WEOC to CO2 emissions than to cumulative N gas emissions for all three soils (Figures 3A–I) reflects higher molar (N2O + N2)-N/CO2-C product ratios for POM than for MOM additions and emphasizes again the importance of plant residues as a C source for denitrifying organisms (e.g., Bremner and Shaw, 1958; Burford and Bremner, 1975; Surey et al., 2020b). However, the estimated contributions of Gi POM and MOM to total gas emissions were almost similar (Figures 3B,E,H), basically due to the relatively large WEOC contents of the MOM fraction (Table 3). In addition, Gi MOM-derived WEOM was characterized by a remarkably low (comparable to POM-derived WEOM) specific UV absorbance at 280 nm (Table 3), indicating smaller portions of aromatic C compounds (Chin et al., 1994), which are less easily decomposable than proteins and low-molecular-weight organic acids (Marschner and Kalbitz, 2003). Therefore, the activity of denitrifying organisms in the clayey grassland soil is likely less limited by readily decomposable OM but rather by the presence of NO3−. The much higher contributions of POM-derived WEOC to total gas emissions for the Ro than for the Gi soil, despite lower amounts of POM (Table 2), suggest that the contribution of OM fractions to total gas emissions of bulk soils depends less on their mass proportions but more on their WEOM content and quality. In addition, the Fu soil contained most POM (Table 2), but the total WEOC content was the lowest among all three soils. This might be due to its sandy texture and low aggregation level, facilitating strong leaching of POM (and MOM) during precipitation events, and, thus, promoting large losses of WEOC (Bremner and Shaw, 1958; Surey et al., 2020b). Also, the high ratios of alkyl C to O/N-alkyl C (Table 4) indicate advanced decomposition of the Fu POM and MOM (Baldock et al., 1997). Consequently, the total amount of WEOC and the related denitrification potential of different soils cannot be estimated from the plant residue content alone. We conclude, therefore, that the distribution of OM fractions is not a suitable direct predictor of the denitrification potential of soils.
Deviations between estimated (sum of POM- and MOM-induced gas amounts) and measured bulk soil emissions (Figures 3A–I) indicate that for part but not all soils the contribution of POM and MOM to potential denitrification can be well predicted based on respective WEOC contents. We suggest that the general overestimation of the sum of POM- and MOM-induced gas emissions from the Fu soil was mainly due to an inaccurate estimate of the contribution of WEOC from individual OM fractions. This generally remains a major experimental challenge, as not all OM fractions can be extracted from soil without alterations in physicochemical properties. Also a priming effect, i.e., accelerated decomposition of native soil OC due to OM additions, cannot be excluded, as it can vary widely among soils and with the chemical composition of added C substrates (Liu et al., 2020). However, the CO2 production of the Ro and Gi soils could be estimated remarkably well, which basically supports the validity of our approach. Deviations from measured N2O and N2O + N2 emissions can be mainly explained by changes in molar N2O/(N2O + N2) and (N2O + N2)-N/CO2-C product ratios caused by OM additions (Table 5; see Effect of Organic Matter Fractions on Denitrification Product Ratios). This indicates that also under field conditions the contribution of POM and MOM to the bulk soil WEOC can vary over time, thus influencing denitrification rates and product ratios.
Overall, our calculations imply that the contributions of POM and MOM to total gas emissions from arable and grassland soils can vary widely and depend less on the mass distribution of OM fractions but rather on the respective content and quality of their WEOC. Contrary to our second hypothesis, the contribution of POM and MOM to potential denitrification of bulk soils could be well estimated by use of respective WEOC contents for only two soils and not in general. Nevertheless, our results again imply that WEOC derived from POM is more relevant for promoting denitrification reactions but MOM-derived WEOC may significantly contribute in soils with relatively large shares of mineral-bound OC.
Conclusion
We showed that contributions of POM and MOM to denitrification can vary widely among soils, depending on physicochemical soil properties (texture, total OC content) and the chemical composition of OM fractions. Generally, POM is far more effective in fueling denitrification than MOM. This could partly explain why denitrification ‘hot spots’ are often associated with plant residues. Additional studies focusing on the contribution of OM fractions to soil denitrification in intact soils and under aerobic conditions could add to better understanding of the relevance of individual OM fractions for soil denitrification. The extraction methods to obtain soil OM fractions and to determine respective WEOC contents should be chosen carefully. Since WEOC contents and denitrification potentials of soils were not directly related to their contents of POM, our study emphasizes that the distribution of OM fractions is not suitable to predict the denitrification potential of soils in general. It appears recommendable to rely on direct determination of WEOC and its chemical composition. In addition, improved estimates of amounts and composition of WEOM produced during decomposition of plant residues might support better prediction of the denitrification potential of agricultural soils.
Data Availability Statement
The original contributions presented in the study are included in the article/Supplementary Material; further inquiries can be directed to the corresponding author.
Author Contributions
RS, KK, JB, and RM conceived the experiment. RS and CS conducted the experiments. RS, CS, and CM analyzed the data. RS prepared the first manuscript draft and all authors contributed to its refinement.
Funding
This study was funded by Deutsche Forschungsgemeinschaft within the research unit RU 2337: “Denitrification in Agricultural Soils: Integrated Control and Modeling at Various Scales (DASIM)” (Grants MI1377/8-1, BO1299/11-1). We acknowledge the financial support of the Open Access Publication Fund of the Martin Luther University Halle-Wittenberg.
Conflict of Interest
The authors declare that the research was conducted in the absence of any commercial or financial relationships that could be construed as a potential conflict of interest
Acknowledgments
We are grateful to Christine Krenkewitz, Gudrun Nemson-von Koch, and Alexandra Boritzki for laboratory assistance; Heidrun Beschow for the C/N analysis of the POM material; Anne Herwig and Leopold Sauheitl for gas measurements; and Isabel Prater for NMR spectroscopy of bulk soils.
Supplementary Material
The Supplementary Material for this article can be found online at: https://www.frontiersin.org/articles/10.3389/fenvs.2021.640534/full#supplementary-material.
References
Akunna, J. C., Bizeau, C., and Moletta, R. (1993). Nitrate and nitrite reductions with anaerobic sludge using various carbon sources: glucose, glycerol, acetic acid, lactic acid and methanol. Water Res. 27, 1303–1312. doi:10.1016/0043-1354(93)90217-6
Almaraz, M., Wong, M. Y., and Yang, W. H. (2020). Looking back to look ahead: a vision for soil denitrification research. Ecology 101, e02917. doi:10.1002/ecy.2917
Baldock, J. A., Oades, J. M., Nelson, P. N., Skene, T. M., Golchin, A., and Clarke, P. (1997). Assessing the extent of decomposition of natural organic materials using solid-state 13C NMR spectroscopy. Aust. J. Soil Res. 35, 1061–1083. doi:10.1071/S97004
Beauchamp, E. G., Trevors, J. T., and Paul, J. W. (1989). “Carbon sources for bacterial denitrification,” in Advances in Soil Science. Editor B. A. Stewart (New York NY, Springer), 113–142. doi:10.1007/978-1-4613-8847-0_3
Böttcher, J., Springob, G., and Duijnisveld, W. H. M. (1999). Sandige Böden und deren Wasser- und Stoffhaushalt unter Acker und Nadelwald im Fuhrberger Feld. Mitt. Dtsch. Bodenkundlichen Ges. 90, 405–424.
Bremner, J. M., and Shaw, K. (1958). Denitrification in soil. II. Factors affecting denitrification. J. Agric. Sci. 51, 40–52. doi:10.1017/S0021859600032779
Burford, J. R., and Bremner, J. M. (1975). Relationships between the denitrification capacities of soils and total, water-soluble and readily decomposable soil organic matter. Soil Biol. Biochem. 7, 389–394. doi:10.1016/0038-0717(75)90055-3
Chin, Y. P., Aiken, G., and O'Loughlin, E. (1994). Molecular weight, polydispersity, and spectroscopic properties of aquatic humic substances. Environ. Sci. Technol. 28, 1853–1858. doi:10.1021/es00060a015
Cleveland, C. C., and Liptzin, D. (2007). C:N:P stoichiometry in soil: is there a “redfield ratio” for the microbial biomass? Biogeochemistry 85, 235–252. doi:10.1007/s10533-007-9132-0
Čuhel, J., Šimek, M., Laughlin, R. J., Bru, D., Chèneby, D., Watson, C. J., et al. (2010). Insights into the effect of soil pH on N2O and N2 emissions and denitrifier community size and activity. Appl. Environ. Microbiol. 76, 1870–1878. doi:10.1128/AEM.02484-09
deCatanzaro, J. B., and Beauchamp, E. G. (1985). The effect of some carbon substrates on denitrification rates and carbon utilization in soil. Biol. Fert. Soils 1, 183–187. doi:10.1007/BF00257635
Don, A., and Kalbitz, K. (2005). Amounts and degradability of dissolved organic carbon from foliar litter at different decomposition stages. Soil Biol. Biochem. 37, 2171–2179. doi:10.1016/j.soilbio.2005.03.019
Gaillard, V., Chenu, C., and Recous, S. (2003). Carbon mineralisation in soil adjacent to plant residues of contrasting biochemical quality. Soil Biol. Biochem. 35, 93–99. doi:10.1016/S0038-0717(02)00241-9
Groffman, P. M., Butterbach-Bahl, K., Fulweiler, R. W., Gold, A. J., Morse, J. L., Stander, E. K., et al. (2009). Challenges to incorporating spatially and temporally explicit phenomena (hotspots and hot moments) in denitrification models. Biogeochemistry 93, 49–77. doi:10.1007/s10533-008-9277-5
Henry, S., Texier, S., Hallet, S., Bru, D., Dambreville, C., Chèneby, D., et al. (2008). Disentangling the rhizosphere effect on nitrate reducers and denitrifiers: insight into the role of root exudates. Environ. Microbiol. 10, 3082–3092. doi:10.1111/j.1462-2920.2008.01599.x
Jäger, H.-J., Schmidt, S. W., Kammann, C., Grünhage, L., Müller, C., and Hanewald, K. (2003). The University of Giessen free-air carbon dioxide enrichment study: description of the experimental site and of a new enrichment system. J. Appl. Bot. 77, 117–127.
Jahn, R., and Guggenberger, G. (2003). Bodenbasisdaten des SPP1090-Standorts Rotthalm¨unster. Schriftl. Mitteilung. Halle (Germany): University of Halle.
Kaiser, K., and Guggenberger, G. (2000). The role of DOM sorption to mineral surfaces in the preservation of organic matter in soils. Org. Geochem. 31, 711–725. doi:10.1016/S0146-6380(00)00046-2
Kaiser, K., Kaupenjohann, M., and Zech, W. (2001). Sorption of dissolved organic carbon in soils: effects of soil sample storage, soil-to-solution ratio, and temperature. Geoderma 99, 317–328. doi:10.1016/S0016-7061(00)00077-X
Kaiser, M., Ellerbrock, R. H., and Sommer, M. (2009). Separation of coarse organic particles from bulk surface soil samples by electrostatic attraction. Soil Sci. Soc. Am. J. 73, 2118–2130. doi:10.2136/sssaj2009.0046
Kalbitz, K., Schmerwitz, J., Schwesig, D., and Matzner, E. (2003a). Biodegradation of soil-derived dissolved organic matter as related to its properties. Geoderma 113, 273–291. doi:10.1016/S0016-7061(02)00365-8
Kalbitz, K., Schwesig, D., Schmerwitz, J., Kaiser, K., Haumaier, L., Glaser, B., et al. (2003b). Changes in properties of soil-derived dissolved organic matter induced by biodegradation. Soil Biol. Biochem. 35, 1129–1142. doi:10.1016/S0038-0717(03)00165-2
Kalbitz, K., Schwesig, D., Rethemeyer, J., and Matzner, E. (2005). Stabilization of dissolved organic matter by sorption to the mineral soil. Soil Biol. Biochem. 37, 1319–1331. doi:10.1016/j.soilbio.2004.11.028
Kleber, M., Eusterhues, K., Keiluweit, M., Mikutta, C., Mikutta, R., and Nico, P. S. (2015). “Mineral–organic associations: formation, properties, and relevance in soil environments,” in Advances in Agronomy. Editor D. L. Sparks 130, 1–140. doi:10.1016/bs.agron.2014.10.005
Knowles, R. (1982). Denitrification. Microbiol. Rev. 46, 43–70. doi:10.1146/annurev.mi.42.100188.001311
Knowles, R. (1990). “Acetylene inhibition technique: development, advantages, and potential problems,” in Denitrification in soil and sediment, federation of European microbiological societies symposium series. Editors N. P. Revsbech, and J. Sørensen (Boston, MA: Springer US), 151–166. doi:10.1007/978-1-4757-9969-9_9
Kögel-Knabner, I., Guggenberger, G., Kleber, M., Kandeler, E., Kalbitz, K., Scheu, S., et al. (2008). Organo-mineral associations in temperate soils: integrating biology, mineralogy, and organic matter chemistry. J. Plant Nutr. Soil Sci. 171, 61–82. doi:10.1002/jpln.200700048
Lavallee, J. M., Soong, J. L., and Cotrufo, M. F. (2020). Conceptualizing soil organic matter into particulate and mineral-associated forms to address global change in the 21st century. Glob. Chang Biol. 26, 261–273. doi:10.1111/gcb.14859
Leinemann, T., Mikutta, R., Kalbitz, K., Schaarschmidt, F., and Guggenberger, G. (2016). Small scale variability of vertical water and dissolved organic matter fluxes in sandy Cambisol subsoils as revealed by segmented suction plates. Biogeochemistry 131, 1–15. doi:10.1007/s10533-016-0259-8
Liebmann, P., Wordell-Dietrich, P., Kalbitz, K., Mikutta, R., Kalks, F., Don, A., et al. (2020). Relevance of aboveground litter for soil organic matter formation – a soil profile perspective. Biogeosciences 17, 1–29. doi:10.5194/bg-17-3099-2020
Liu, X. J. A., Finley, B. K., Mau, R. L., Schwartz, E., Dijkstra, P., Bowker, M. A., et al. (2020). The soil priming effect: consistent across ecosystems, elusive mechanisms. Soil Biol. Biochem. 140, 107617. doi:10.1016/j.soilbio.2019.107617
Marschner, B., and Kalbitz, K. (2003). Controls of bioavailability and biodegradability of dissolved organic matter in soils. Geoderma 113, 211–235. doi:10.1016/S0016-7061(02)00362-2
Mastný, J., Kaštovská, E., Bárta, J., Chroňáková, A., Borovec, J., Šantrůčková, H., et al. (2018). Quality of DOC produced during litter decomposition of peatland plant dominants. Soil Biol. Biochem. 121, 221–230. doi:10.1016/j.soilbio.2018.03.018
McClain, M. E., Boyer, E. W., Dent, C. L., Gergel, S. E., Grimm, N. B., Groffman, P. M., et al. (2003). Biogeochemical hot spots and hot moments at the interface of terrestrial and aquatic ecosystems. Ecosystems 6, 301–312. doi:10.1007/s10021-003-0161-9
Mikutta, R., Kaiser, K., Dörr, N., Vollmer, A., Chadwick, O. A., Chorover, J., et al. (2010). Mineralogical impact on organic nitrogen across a long-term soil chronosequence (0.3–4100 kyr). Geochim. Cosmochim. Acta 74, 2142–2164. doi:10.1016/j.gca.2010.01.006
Mikutta, R., Mikutta, C., Kalbitz, K., Scheel, T., Kaiser, K., and Jahn, R. (2007). Biodegradation of forest floor organic matter bound to minerals via different binding mechanisms. Geochim. Cosmochim. Acta 71, 2569–2590. doi:10.1016/j.gca.2007.03.002
Mikutta, R., Schaumann, G. E., Gildemeister, D., Bonneville, S., Kramer, M. G., Chorover, J., et al. (2009). Biogeochemistry of mineral–organic associations across a long-term mineralogical soil gradient (0.3–4100 kyr), Hawaiian Islands. Geochim. Cosmochi. Acta 73, 2034–2060. doi:10.1016/j.gca.2008.12.028
Miller, M. N., Zebarth, B. J., Dandie, C. E., Burton, D. L., Goyer, C., and Trevors, J. T. (2008). Crop residue influence on denitrification, N2O emissions and denitrifier community abundance in soil. Soil Biol. Biochem. 40, 2553–2562. doi:10.1016/j.soilbio.2008.06.024
Ottow, J. C. G. (2011). Mikrobiologie von Böden. Berlin, Heidelberg: Springer Berlin Heidelberg Available at: http://link.springer.com/10.1007/978-3-642-00824-5.
Palmer, K., Biasi, C., and Horn, M. A. (2012). Contrasting denitrifier communities relate to contrasting N2O emission patterns from acidic peat soils in arctic tundra. ISME J. 6, 1058–1077. doi:10.1038/ismej.2011.172
Parkin, T. B. (1987). Soil microsites as a source of denitrification variability. Soil Sci. Soc. Am. J. 51, 1194. doi:10.2136/sssaj1987.03615995005100050019x
Parry, S., Renault, P., Chadœuf, J., Chenu, C., and Lensi, R. (2000). Particulate organic matter as a source of variation in denitrification in clods of soil. Eur. J. Soil Sci. 51, 271–281. doi:10.1046/j.1365-2389.2000.00298.x
Philippot, L., Hallin, S., and Schloter, M. (2007). Ecology of denitrifying prokaryotes in agricultural soil. Adv. Agron. 96, 249–305. doi:10.1016/S0065-2113(07)96003-4
Rashid, G. H., and Schaefer, R. (1988). The influence of glucose and other sources of carbon on nitrate reduction rates in two temperate forest soils. Plant Soil 106, 43–48. doi:10.1007/BF02371193
Senbayram, M., Chen, R., Budai, A., Bakken, L., and Dittert, K. (2012). N2O emission and the N2O/(N2O + N2) product ratio of denitrification as controlled by available carbon substrates and nitrate concentrations. Agric. Ecosyst. Environ. 147, 4–12. doi:10.1016/j.agee.2011.06.022
Surey, R., Lippold, E., Heilek, S., Sauheitl, L., Henjes, S., Horn, M. A., et al. (2020a). Differences in labile soil organic matter explain potential denitrification and denitrifying communities in a long-term fertilization experiment. Appl. Soil Ecol. 153. doi:10.1016/j.apsoil.2020.103630
Surey, R., Schimpf, C. M., Sauheitl, L., Mueller, C. W., Rummel, P. S., Dittert, K., et al. (2020b). Potential denitrification stimulated by water-soluble organic carbon from plant residues during initial decomposition. Soil Biol. Biochem. 147, 107841. doi:10.1016/j.soilbio.2020.107841
Swanston, C. W., Caldwell, B. A., Homann, P. S., Ganio, L., and Sollins, P. (2002). Carbon dynamics during a long-term incubation of separate and recombined density fractions from seven forest soils. Soil Biol. Biochem. 34, 1121–1130. doi:10.1016/S0038-0717(02)00048-2
Terry, R. E., and Duxbury, J. M. (1985). Acetylene decomposition in soils. Soil Sci. Soc. Am. J. 49, 90–94. doi:10.2136/sssaj1985.03615995004900010018x
Valera, C. L., and Alexander, M. (1961). Nutrition and physiology of denitrifying bacteria. Plant Soil 15, 268–280. doi:10.1007/BF01400460
Yamashita, T., Flessa, H., John, B., Helfrich, M., and Ludwig, B. (2006). Organic matter in density fractions of water-stable aggregates in silty soils: effect of land use. Soil Biol. Biochem. 38, 3222–3234. doi:10.1016/j.soilbio.2006.04.013
Yeomans, J. C., and Beauchamp, E. G. (1978). Limited inhibition of nitrous oxide reduction in soil in the presence of acetylene. Soil Biol. Biochem. 10, 517–519. doi:10.1016/0038-0717(78)90046-9
Keywords: denitrification potential, nitrous oxide, carbon dioxide, organic matter fractions, water-extractable OM, particulate OM, mineral-associated OM, agricultural soils
Citation: Surey R, Kaiser K, Schimpf CM, Mueller CW, Böttcher J and Mikutta R (2021) Contribution of Particulate and Mineral-Associated Organic Matter to Potential Denitrification of Agricultural Soils. Front. Environ. Sci. 9:640534. doi: 10.3389/fenvs.2021.640534
Received: 11 December 2020; Accepted: 02 February 2021;
Published: 07 April 2021.
Edited by:
Lu-Jun Li, Chinese Academy of Science, ChinaReviewed by:
Tim J. Clough, Lincoln University, New ZealandYansheng Li, Chinese Academy of Science, China
Copyright © 2021 Surey, Kaiser, Schimpf, Mueller, Böttcher and Mikutta. This is an open-access article distributed under the terms of the Creative Commons Attribution License (CC BY). The use, distribution or reproduction in other forums is permitted, provided the original author(s) and the copyright owner(s) are credited and that the original publication in this journal is cited, in accordance with accepted academic practice. No use, distribution or reproduction is permitted which does not comply with these terms.
*Correspondence: Ronny Surey, cm9ubnkuc3VyZXlAbGFuZHcudW5pLWhhbGxlLmRl
†Present address: Carsten W. Mueller, Department of Geosciences and Natural Resource Management, University of Copenhagen, Copenhagen, Denmark