- 1Institute of Biology, Freie Universität Berlin, Berlin, Germany
- 2Berlin-Brandenburg Institute of Advanced Biodiversity Research, Berlin, Germany
Previous microplastic research under laboratory conditions has focused on microplastics that are homogeneously mixed into test media, in order to maximize test reproducibility and uniform bio-accessibility. Here we specifically focused on testing the idea that microplastics in soil could affect adjacent soil layers not containing microplastic themselves. We included two different microplastics (low-density polyethylene films and polyacrylonitrile fibers) and carried out a soil column test consisting of three different vertical layers (0–3 cm, top, control soil; 3–6 cm, middle, microplastic-containing soil; 6–9 cm, bottom, control soil). Our study shows that microplastic-containing soil layers can act as an anthropogenic barrier in the soil column, interrupting the vertical water flow. These changes directly affected the water content of adjacent layers, and changes in the proportion of soil aggregate sizes occurred for each depth of the soil columns. We also observed that these physical changes trigger changes in soil respiration, but do not translate to effects on enzyme activities. These results imply that the soil environment in non-contaminated parts of the soil can be altered by microplastic contamination in adjacent layers, as might occur for example during ploughing on agricultural fields. More generally, our results highlight the need to further examine effects of microplastic in experiments that do not treat this kind of pollution as uniformly distributed.
Introduction
Scientists estimate that less than 5% of plastic production is recycled (Sutherland et al., 2019), and a considerable amount of plastic waste is accumulating in the environment (Jambeck et al., 2015; Rillig and Lehmann, 2020). One of the main concerns about plastic pollution is that plastic waste can be slowly fragmented into smaller size under environmental conditions such as UV-radiation and mechanical weathering (Arthur et al., 2009). These tiny particles (<5 mm), defined as “microplastics,” are ubiquitously observed in freshwater (Sarijan et al., 2021), oceans (Andrady, 2011), atmospheres (Chen et al., 2020), and soils (Rillig, 2012). An annual input rate of microplastics into European agricultural lands has been estimated to be 125–850°tons per million inhabitants, and 427 thousand°tons of plastic mulch films are used every year in European farmlands (Nizzetto et al., 2016). Previous studies have reported that 300–67,500 mg kg−1 or 40–18,760 particles kg−1 of microplastics are observed in agricultural (Liu et al., 2018; Piehl et al., 2018; Zhang et al., 2018; Zhang and Liu, 2018; Ding et al., 2020), coastal (Zhou et al., 2018), floodplain (Scheurer and Bigalke, 2018), and industrial lands (Fuller and Gautam, 2016).
Research on microplastics effects has been mainly conducted under highly controlled laboratory conditions since this provides more accurate results, and many studies have mixed microplastic into test media as homogeneously as possible to keep variability of results low. In liquid media, homogenous dispersion of insoluble test substances (e.g., nanomaterials and microplastics) is an important requirement to reduce agglomeration or sedimentation, and the use of dispersants is often adopted as an efficient strategy (Potthoff et al., 2017). For soil, it is also recommended for target material to be mixed thoroughly and homogenized (Thomas et al., 2020). A recent study explained that the “homogeneity of exposure” is a crucial criterion to guarantee the reproducibility and uniform bio-accessibility during laboratory tests in microplastic research (de Ruijter et al., 2020).
Here, we were specifically interested in testing if microplastics in soil can affect adjacent soil layers not even containing microplastic themselves. Microplastics can induce changes in soil physicochemical and biological parameters, and these effects have been well-established in previous studies (Rillig and Lehmann, 2020). For instance, microplastic fibers can interfere with soil aggregate formation due to their linear shape (de Souza Machado et al., 2018; de Souza Machado et al., 2019; Zhang et al., 2019), and microplastic films influence soil tensile strength (Wan et al., 2019). It is likely that such physical changes in microplastic-containing soils would become more intense with time (de Souza Machado et al., 2018; de Souza Machado et al., 2019; Lehmann et al., 2020b), and that flows of water and nutrients into adjacent soil layers can be influenced. This would be important, because such indirect effects would suggest that previous work might have underestimated the extent of microplastic effects in soil. To capture this situation, we designed an experiment in which we added microplastic in a layer of a soil column, and this afforded us the opportunity to study effects on adjacent soil layers that are themselves not contaminated. We selected two different microplastics as target materials; low-density polyethylene (LDPE) films and polyacrylonitrile (PAN) fibers. The soil column was constructed with three layers (control soil; microplastic-containing soil; control soil), and two different levels of water addition (low and high) were included in the experimental design. To evaluate biophysical parameters at each depth of the soil columns, water content, water flow, soil aggregates sizes, soil respiration, and enzyme activities were measured after short- (1 day) and long-term (60 days) incubation periods.
Materials and Methods
Preparation and Characterization of Microplastics
LDPE films and PAN fibers were prepared using commercial mulching films (thickness, 13.66 ± 2.32°μm, Ihlshin Chemical Co., Ltd., Ansan, South Korea) and knitting wool (100% PAN, DIKTAS Sewing & Knitting Yarns Co., Turkey) (Kim et al., 2020). Each material was cut using sterilized scissors, and then passed through a 630 μm-sieve. Each microplastic was observed under a microscope, and close-up photographs were captured to determine average sizes using image analysis (ImageJ, 1.52a, National Institutes of Health, United States) (Supplementary Figure S1). The average area of LDPE films was calculated as 1.5 ± 0.8 mm−2 (n = 100), and the average length of PAN fibers was 2.4 ± 0.6 mm (n = 100). Target microplastics were stored at room temperature before main experiments. To characterize the actual nature of each material, a spectrophotometer (Jasco, model FT/IR-4100, ATR mode) was used, and each sample was scanned 32 times from 4000 to 600 cm−1, with a resolution of 4 cm−1 (Supplementary Figure S2).
Soil Column Test
Test soil was collected from a grassland site of the Institute of Biology of Freie Universität, Berlin, Germany (52.45676N, 13.30240E) on January 20, 2020. The soil was passed through a 2 mm-sieve, and then dried at 60°C for 24 h. The texture of test soil was a sand (sand 93.3%, silt 5.0%, and clay 1.7%), and pH and water holding capacity (WHC) were 6.7 ± 0.2 and 0.34 ± 0.10 ml g−1, respectively (n = 3). In order to prepare microplastic soils (LDPE films and PAN fibers), 100 mg of each microplastic and 99.9 g of dry test soil were mixed using laboratory tweezers and a spatula, and each mixture was shaken using an overhead shaker (Reax 2, Heidolph, Germany) for 5 min (0.1% based on dry weight). The control soil was treated by an equivalent process (shaking), but not containing microplastics, and each soil was directly used for the soil column test. To prepare the soil column, 10 g of test soil was placed into 50 ml-test tubes (bottom layer), and 10 g of each microplastic-containing soil (LDPE films and PAN fibers, 0.1%) were added (microplastic-containing soil layer), after which additional test soil (10 g) was placed into the test tube (top layer) (n = 3). A control treatment was prepared with no microplastic-containing soil layer, but using an otherwise equivalent process (n = 3). The total soil depth of the soil column was approximately 9 cm, and the depth of each layer (top, microplastic-containing soil, and bottom) was 3 cm (Figure 1). To moisten the soil columns, 3 ml (low level of irrigation) or 6 ml (high) of deionized water was carefully injected into surface soil (<1 cm) using a syringe needle, and these water levels were regarded as 10 and 20% of total soil weight. Each soil column was covered by a vented cap and incubated at 20°C-laboratory incubator (PP110plus, Memmert GmbH, Schwabach, Germany) in the dark for 1 day or 60 days, respectively. Changes in biological parameters are expected to be observed after long-term incubation, while the water infiltration occurs within 1–2 days (Schneider et al., 2018). We determined two test periods (1 and 60 days) to check both parameters in the soil columns. Since the different water content in soil can influence our measurement, parameters such as soil respiration and enzyme activities, water content was replenished every 3 days to keep uniform moisture during incubation periods.
At the end of each incubation period, soil samples of each depth (1 cm) were carefully collected using laboratorial spatula. The weights of each soil sample were recorded before and after drying at 60°C for 24 h to calculate water content (%). Soil structure of each depth were assessed as reported in previous study (de Souza Machado et al., 2019; Lehmann et al., 2020a). Shortly, the whole soil was gently passed through a set of stacked sieves (4,000, 2,000, 1,000, and 212 μm), and the weights of four separated fractions were recorded to determine the proportions (%) of each soil aggregate size class. Bulk density was computed by measuring the volume of soils within the plastic pot and soil dry weight (g cm−3). We measured the soil respiration of three layers (top, microplastic-containing soil, and bottom), as CO2 production rate (ppm h−1) after 60 days of the experiment. Before the measurement, we flushed each of the tubes with CO2-free air for five minutes to standardize among experimental units (Rillig et al., 2019). After 18 h, we sampled 1 ml of air from the headspace of each tube and injected this sample into an infrared gas analyzer (LiCOR 6400xt). Extracellular soil enzyme activities, acid phosphatase and β-D-glucosidase were measured after the 60 days incubation (Jackson et al., 2013). Briefly, 5 g of each soil sample (top, microplastic-containing soil, and bottom) was placed into a 50 ml test tube and mixed with 10 ml of 50 mM acetate buffer (pH 5.0–5.4), and 150 µl soil slurry was pipetted into each of six wells on a 96-well plate after vortexing. Then 150 µl acetate buffer was added into the last two wells of each samples (sample buffer control), and 150 µl substrate solutions (p-nitrophenyl-phosphate and p-nitrophenyl-β-glucopyranoside; Sigma, Germany) to the first four wells. Then the plates were kept in an incubator at 25°C for 2–4 h. After incubation, the microplates were centrifuged at 3000 x g for 5 min, and then 100 µl supernatant from each well was added into the new microplates with 10 µl 1 M NaOH and 190 µl distilled water in each well. Finally, the absorbance was recorded at 410 nm by a microplate reader (Benchmark Plus Microplate Spectrophotometer System, BioRad Laboratories, Hercules, CA, United States).
Dye Tracer Test
To observe the spatial patterns of water flow in the soil columns, dye tracer experiments were conducted with starting and 60 days incubated soil columns. The starting soil columns (0 days, before irrigation) were directly used for dye tracer test, and the 60 days incubated columns were dried at 60°C for 48 h. We employed Brilliant Blue dye as a tracer since it is highly visible (Schneider et al., 2018). Although dye transport is slower than the advance of infiltrating water, dye-stained soil patterns are generally considered to reasonable reflect flow patterns in soil experiments (Cey and Rudolph, 2009). We dissolved 100 mg of Brilliant Blue powder in 100 ml of deionized water, and 3 ml or 6 ml of dye solutions were applied to each soil column. After 24 h, the soil was carefully separated from the soil column, and vertically excavated to observe the soil profiles. To study the distribution of dye tracer in the soil profiles, photographs were captured. For each profile, the close-up photographs were adjusted for analyzing the relative pixel intensity of Brilliant Blue dyed path using ImageJ software (ImageJ, 1.52a, National Institutes of Health, United States).
Statistical Analyses
Data were analyzed using the SPSS statistical software (Ver. 24.0, SPSS Inc., Chicago, IL, United States). One-way analysis for variance (ANOVA) and Turkey’s tests were conducted to determine the significance (p < 0.05) of multiple comparisons.
Results And Discussion
Effects on Water Contents and Flows
We observed each soil sample at each depth to examine the potential migration of microplastics during the soil column tests. As shown in Supplementary Figures S3–S6, microplastic-contaminated soil layers contained numerous LDPE films and PAN fibers, while only a few microplastic particles were found in top and bottom layers. We assume that the microplastics ended up in adjacent layers during the layer separation or soil analysis steps. Although the microplastics may migrate to the adjacent soil layers with longer time or different conditions, we concluded here that the microplastics were not transported in our soil column tests. Water contents of each depth were considerably different already after the 1 day incubation. In the control treatment, after low-level irrigation, water content of the top layer (0–3 cm) was relatively higher than the bottom layer (6–9 cm), and this difference significantly increased in microplastic-containing soil layer treatments. Water contents increased to 13.03 ± 0.29 (LDPE films) and 12.98 ± 0.28 (PAN fibers) % in the top transition layer (3–4 cm), while the control treatment had a water content of 11.32 ± 0.19%. In the bottom transition layer (6–7 cm), water contents were 1.67 ± 0.19 (LDPE films) and 1.57 ± 0.23 (PAN fibers) %, while control treatment had 4.72 ± 0.57% (Figure 2A). The gaps of water contents between top and bottom layers were reduced after the 60 days of incubation, but significant differences among depths remained in the soil column containing PAN fiber layer (Figure 2B). In high-level irrigation treatments, only the soil layer with PAN fibers significantly influenced the vertical water distribution (Figure 2C), and the difference of water content in the top layer disappeared after the 60 days incubation, but remained in the bottom layer (Figure 2D).
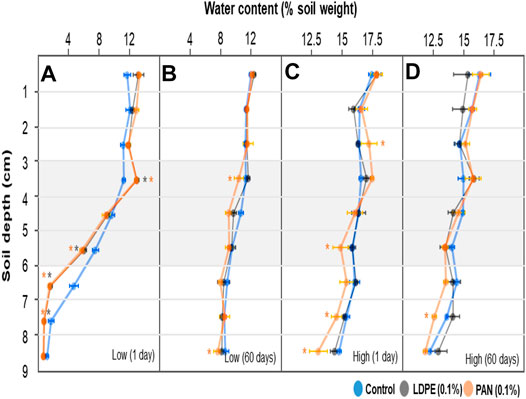
FIGURE 2. Water contents (% soil dry weight) at each soil depth (0–9 cm) of each soil column (control and microplastic-containing soil layer treatments). Each soil column was irrigated either at a low level (A,B) or with higher water volume (C,D), and incubated for 1 day (A,C) and 60 days (B,D). Asterisk represents significance at the level of 5% (p = 0.05) between control and microplastic-containing soil layer treatments.
The infiltrated dye stain patterns for vertical soil profiles are shown in Figure 3. In the control treatment after low-level irrigation, the dye tracer solution had uniformly infiltrated into soil depth 3–4 cm for both incubation periods (1 and 60 days), while uneven dye distributions and several discontinuities were observed in microplastic-containing soil layer treatments (yellow arrows in Figures 3A,B). After high-level irrigation, the maximum depth of dyed soil in the control treatment increased to 4–5 cm for both incubation periods (1 and 60 days) (Figures 3C,D). Paths of preferential flow appeared in microplastic-containing soil layer treatments (LDPE films and PAN fibers), and these patterns were observed below a soil depth of 6 cm (yellow arrows in Figure 3C). Although the dye transport does not exactly match the infiltrating water volume, the preferential flow indicates that the microplastic-containing soil layer might block and influence the water flow path in the soil column. These preferential flows were not observed after 60 days of incubation, and uneven dye distributions were observed in the top layers (yellow arrows in Figure 3D).
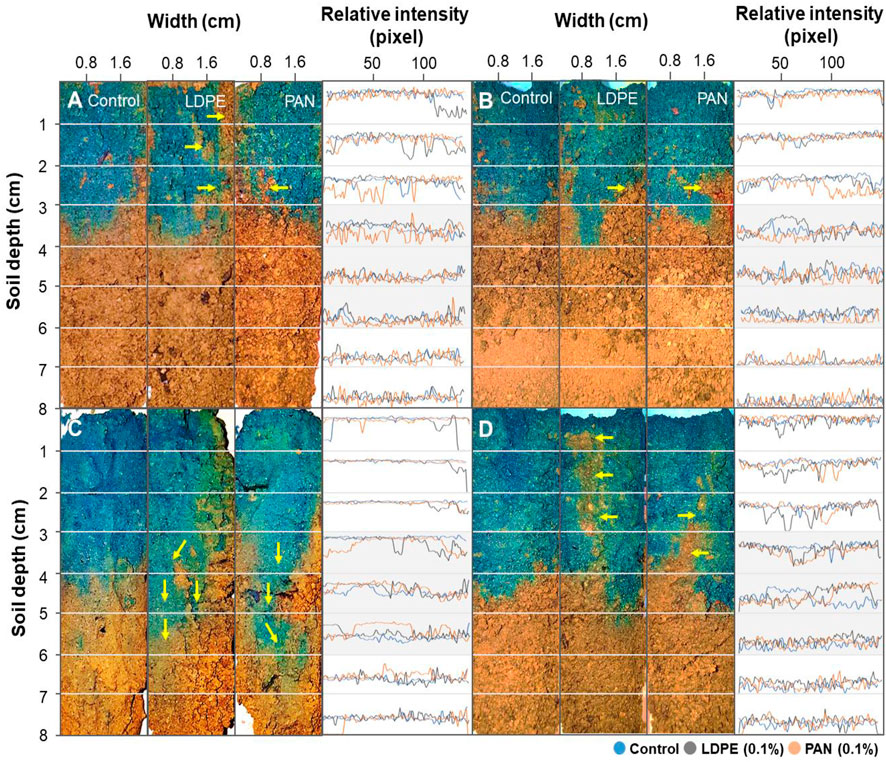
FIGURE 3. Infiltrated dye stain patterns for vertical soil profiles. Each soil column was incubated for 0 days (A,C) and 60 days (B,D), and irrigated either at a low level (A,B) or with higher water volume (C,D).
Soil water content plays an important role in hydrological and biological processes, and spatial variability, both horizontally and vertically, is typically present in soil profiles (Wang and Liu, 2013). In anthropogenically modified soils, a high heterogeneity of substrates and unique patterns of water infiltration are often observed, such as in mine spoil soils, tilled soils, and biochar-containing soils (Andreini and Steenhuis, 1990; Badorreck et al., 2010; Schneider et al., 2018). A high spatial heterogeneity of pore volumes can be associated with anthropogenic (e.g., relict charcoal hearths) or natural fragments (e.g., organic matter and plant roots), and these can affect water flows in soil profiles (Schneider et al., 2018). There are several previous studies reporting that microplastics can influence water dynamics (de Souza Machado et al., 2018; de Souza Machado et al., 2019; Wan et al., 2019). Polyethylene films and polyester fibers induced changes in soil aggregation and pore sizes, and these phenomena can be directly or indirectly linked with water evaporation and soil cracking (Wan et al., 2019; Zhang et al., 2019). Alterations in soil structure can affect pore space in soils, which can simultaneously alter water holding capacity and water availability (de Souza Machado et al., 2019). Our study here shows that microplastic-containing soil layers can affect water contents and flows in adjacent soil layers, even if total water contents in the soil columns were kept the same in each treatment (control, LDPE films, and PAN fibers) (Supplementary Figure S7).
Effects on Soil Physical Structure
With low-level irrigation (1 day incubation) in the control treatment, large soil aggregate size fractions (2–4 mm) decreased with increasing soil depth, while intermediate sized fractions (1–2 and 0.1–1 mm) increased. This difference was more pronounced in microplastic-containing soil layer treatments, and mainly occurred in the microplastic-containing soil and bottom layers (<4 cm soil depth) (Figures 4A–C). After 60°days, the differences in soil aggregate size fractions between each soil depth were noticeably reduced in the control treatment, but significant differences among soil depths were still observed in the microplastic-containing soil layer treatments (Figures 4E–G). With high-level irrigation, large and intermediate sized soil aggregate fractions (2–4 and 1–2 mm) showed similar levels at each soil depth, but the PAN fiber layer influenced other size fractions (0.2–1 and <0.2 mm) (Figures 5A–D). After 60°days, the proportion of small soil aggregate size fractions (<0.2 mm) was dramatically changed by LDPE films and PAN fiber layers. The proportion of the small size fraction significantly increased in the top layers and decreased in the bottom layers of PAN fiber treatment, and LDPE film layers had a significantly lower level than the control treatment (Figure 5H). Soil bulk density in the bottom layer seemed to be slightly influenced by microplastic-containing soil layers, but overall levels were similar in each treatment and depth (Figure 6).
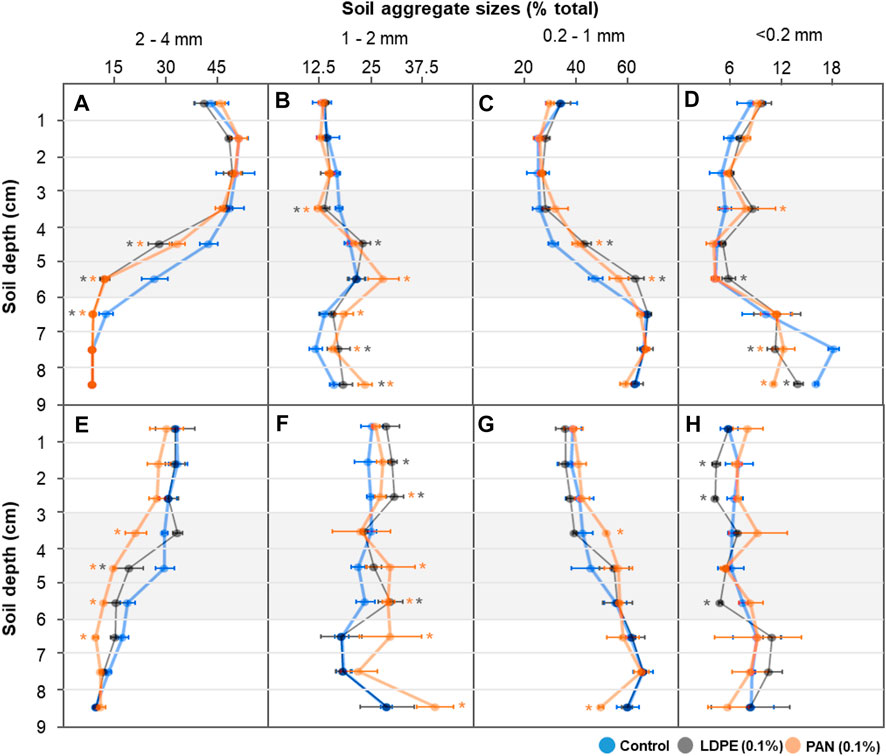
FIGURE 4. Soil aggregate size fractions (2–4; 1–2; 0.2–1; <0.2 mm) at each depth (0–9 cm) after low-level irrigation. Each soil column was incubated for 1 day (A–D) and 60 days (E–H). Asterisk represents significance at the level of 5% (p = 0.05) between control and microplastic-containing soil layer treatments.
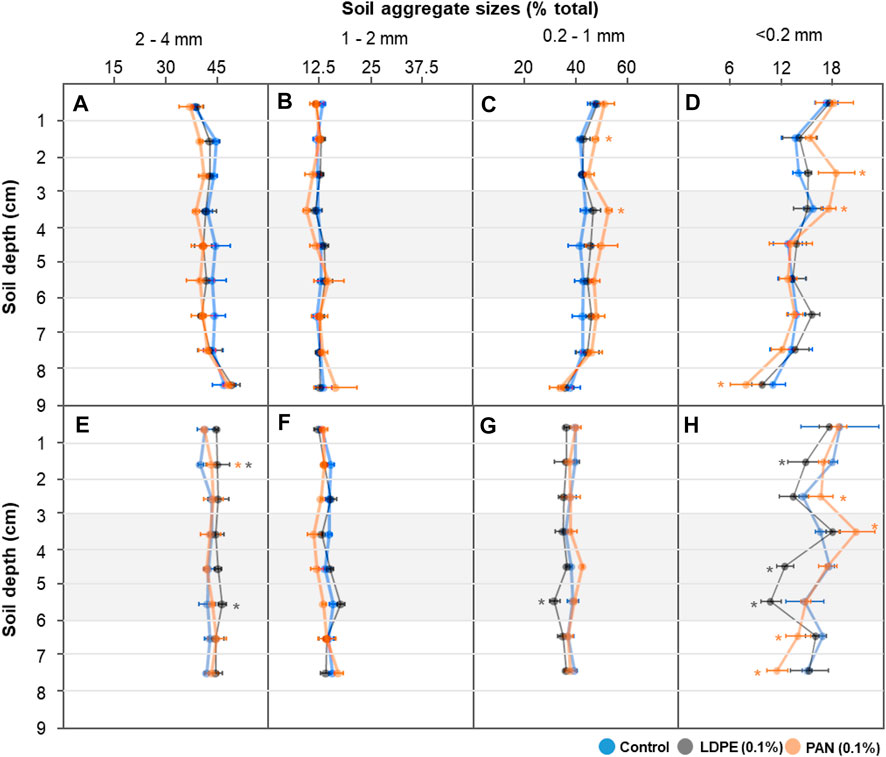
FIGURE 5. The fractions of each soil aggregate size (2–4; 1–2; 0.2–1; <0.2 mm) in each depth (0–9 cm) after high-level irrigation. Each soil column was incubated for 1 day (A–D) and 60 days (E–H). Asterisk represents significance at the level of 5% (p = 0.05) between control and microplastic-containing soil layer treatments.
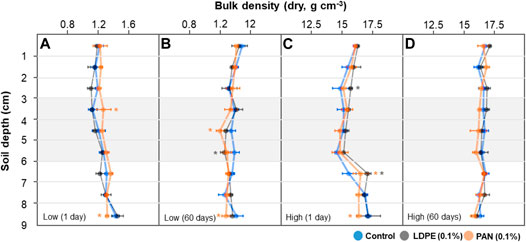
FIGURE 6. Bulk density (dry, g cm−3) at each soil depth (0–9 cm) of each soil column (control and microplastic-containing soil layer treatments). Each soil column was irrigated either at a low level (A,B) or with higher water volume (C,D), and incubated for 1 day (A,C) and 60 days (B,D). Asterisk represents significance at the level of 5% (p = 0.05) between control and microplastic-containing soil layer treatments.
The relative proportion of micro- (<0.2 mm) and larger macro-aggregates (2–4 mm) is crucial for pore size distribution (Horn and Smucker, 2005), and thus directly and indirectly influence the movement of water, gas, and nutrients (Jayarathne et al., 2021). We observed that the differences in size fractions between adjacent layers were less pronounced after the 60 days incubation since the water started to slowly infiltrate into the whole soil column from the soil surface (Figures 2, 4). In microplastic-containing soil treatments with low-level irrigation, the significant differences in large and intermediate sized soil aggregate fractions (2–4, 1–2, and 0.2–1 mm) were still observed after 60 days of incubation (Figures 4E–G). With high-level irrigation, each size fraction in the soil columns after the 60 days incubation showed similar levels in control and microplastic treatments due to relatively homogenous water contents (Figures 5E–G), but fluctuations were observed in small soil aggregate size fractions (<0.2 mm) (Figure 5H). Since more intense irrigation can increase the dispersion of water and the mobility of clay particles (Horn and Dexter, 1989), the soil fraction in the size range of micro-aggregates seems to be influenced by both clay contents (Schweizer et al., 2019) and microplastics (Rillig and Lehmann, 2020).
The effects of microplastic fibers on soil aggregation have been well established in previous studies (de Souza Machado et al., 2019; Rillig and Lehmann, 2020). Aggregate water stability decreased by polyamide and polyester fibers in sandy loam soil (de Souza Machado et al., 2018; de Souza Machado et al., 2019; Lehmann et al., 2019), however, the contrary result that macro-aggregate fractions increased by polyester fibers addition in clayey soil (Zhang et al., 2019) was also observed. Films, which is one of the two microplastic shapes we use here, reduce tensile strength of soil, and desiccation shrinkage and cracking can be induced, depending on film particle size (Wan et al., 2019). In the present study, changes in each soil aggregate size fraction occurred in both microplastic-containing soil and bottom layers, and larger macro-aggregate fractions (2–4 mm) decreased while micro-aggregates (<0.2 mm) were more variable. Our results here show that the microplastic-containing soil layer acts as an anthropogenic barrier, disrupting water flow paths into the bottom soil layer, and the different water contents in each layer seemed to be highly linked with the changes in the soil aggregate size fraction. Regarding microplastic target concentration, the changes in soil aggregate fractions by microplastic addition were induced in the ranges of 0.1–0.4% in previous reports (de Souza Machado et al., 2018; de Souza Machado et al., 2019; Lehmann et al., 2019). In our study, the soil aggregate size fractions were influenced not only in 0.1% of LDPE films or PAN fibers containing soil layers, but also in adjacent layers. Since many previous studies have focused on the homogeneous microplastic distribution in test soil and the effects in themselves, the observed changes in non-contaminated adjacent layers might mean that the effects of microplastics have been underestimated.
Effects on Biological Parameters
The results for soil respiration and enzyme activities are shown in Supplementary Table S1. A part of β-galactosidase data are missing due to experimental errors during measurements. With low-level irrigation, soil respiration rates (CO2 production) in the top layers (0–3 cm) were 5.10–5.25 ppm h−1, and those of the middle layers (3–6 cm) were 3.78–4.45 ppm h−1. Significant changes were observed in the bottom layer (6–9 cm), as LDPE films and PAN fibers treatments had lower respiration (2.54 ± 0.12 (LDPE films), 2.75 ± 0.36 (PAN fibers) ppm h−1) than control (3.27 ± 0.45 ppm h−1). With high-level irrigation, soil respiration rates increased in the bottom layers with microplastic-containing soil layers, with 6.26 ± 0.80 (control), 7.64 ± 0.79 (LDPE films), and 7.86 ± 0.38 (PAN fibers) ppm h−1, respectively. Enzyme activities in the bottom layers showed no significant differences between control and microplastic-containing soil layer treatments. Although acid phosphatase in soil columns containing PAN fibers tended to have higher activity (8.90 ± 5.02 and 8.32 ± 6.06 μmol mg−1 h−1 for low- and high-level irrigations, respectively), these values were not significantly different from the control (5.40 ± 3.05 and 4.09 ± 0.35 μmol mg−1 h−1). The activities of β-D-glucosidase in each treatment were calculated as 1.92–3.68 (for low-level irrigation) and 2.10–3.08 (for high-level irrigation) μmol mg−1 h−1, and there were no significant differences compared with control treatment.
Broad and extensive microbial responses to microplastic exposure have been reported in many previous studies (Liu et al., 2017; Yang et al., 2018; Huang et al., 2019). LDPE films and PAN fibers, the target microplastics in this study, can affect the rate of fluorescein diacetate hydrolysis (Huang et al., 2019; Liang et al., 2019). Microplastic fibers could provide more porosity, and their effects on soil respiration and enzyme activities can depend on soil water conditions (Lozano et al., 2021). Microplastic films can strongly influence soil respiration (Ng et al., 2020), and could reduce activity of aerobic microbes by affecting soil aeration due to their planar shape (Lehmann et al., 2020b). Previous studies have suggested that changes in soil structure can be a trigger for a series of events (de Souza Machado et al., 2018; de Souza Machado et al., 2019). Changes in soil structure can influence pore spaces, which can alter water dynamics and soil aeration, and this microplastic-driven physical change is particularly linked to biological or chemical processes. In our study, microplastic-containing soil layers interrupted water flow in soil and changed soil physical structure. These differences would be directly or indirectly linked with microbial activities: water content in soil has a linear relationship with soil respiration (Cook and Orchard, 2008), and soil aggregate size class is highly correlated with biological soil parameters since each size fraction has a different available organic matter content and C-N ratio (Ashman et al., 2003). We found evidence that microplastic-containing soil layers can affect a biological parameter (soil respiration) in the non-contaminated bottom layer. Despite the changes in soil respiration in the bottom layer, these changes did not translate to overall changes in the rate of enzyme activities.
Conclusion
Microplastics have unique properties compared with more traditional pollutants, such as heavy metals or organic chemicals, and many previous studies have reported effects of microplastic on soil properties. We here examined that microplastics-containing soil can affect adjacent soil layers not containing microplastic. We conducted a simple soil column test taking a phenomenological approach. Our results provide crucial evidence that microplastics-containing soil layers could act as an anthropogenic barrier, leading to vertically interrupted soil water flows and changes in physical structure. These effects occurred not only in microplastic-containing soil layers, but also in adjacent layers (top and bottom). Our results imply that the indirect effects on adjacent soils might be underestimated, and soil systems can be altered by microplastic contamination in unexpected ways. While our study was intended as a proof-of-concept, it also has relevance to real world situations, for example when plastic-containing soil surface layers are flipped during certain ploughing operations in agricultural systems. Overall, we argue that future research should also consider heterogenous distribution of microplastic pollutants in ecosystems.
Data Availability Statement
The original contributions presented in the study are included in the article/Supplementary Material, further inquiries can be directed to the corresponding author.
Author Contributions
SK: conceptualization, design of the study, experiment set up, analysis of data, and writing. TZ and YL: analysis and writing. MR: review and editing. All authors contributed to the article and approved the submitted version.
Conflict of Interest
The authors declare that the research was conducted in the absence of any commercial or financial relationships that could be construed as a potential conflict of interest.
Acknowledgments
We acknowledge support by the Open Access Publication Initiative of Freie Universität Berlin. This work was supported by a post-doctoral grant from the National Research Foundation of Korea funded by the Ministry of Science, ICT, and Future Planning (2019R1A6A3A03031386). MR acknowledges support from an ERC Advanced Grant (grant no. 694368). YL acknowledges a scholarship from the China Scholarship Council. TZ acknowledges the China Scholarship Council for a scholarship (CSC No. 201608260012).
Supplementary Material
The Supplementary Material for this article can be found online at: https://www.frontiersin.org/articles/10.3389/fenvs.2021.681934/full#supplementary-material
References
Andrady, A. L. (2011). Microplastics in the Marine Environment. Mar. Pollut. Bull. 62, 1596–1605. doi:10.1016/j.marpolbul.2011.05.030
Andreini, M. S., and Steenhuis, T. S. (1990). Preferential Paths of Flow under Conventional and Conservation Tillage. Geoderma 46, 85–102. doi:10.1016/0016-7061(90)90009-x
Arthur, C., Baker, J., and Bamford, H. (2009). Proceedings of the International Research Workshop on the Occurrence, Effects, and Fate of Microplastic Marine Debris, September 9–11 (Tacoma, WA, United States: University of Washington Tacoma).
Ashman, M. R., Hallett, P. D., and Brookes, P. C. (2003). Are the Links between Soil Aggregate Size Class, Soil Organic Matter and Respiration Rate Artefacts of the Fractionation Procedure? Soil Biol. Biochem. 35, 435–444. doi:10.1016/s0038-0717(02)00295-x
Badorreck, A., Gerke, H. H., and Vontobel, P. (2010). Noninvasive Observations of Flow Patterns in Locally Heterogeneous Mine Soils Using Neutron Radiation. Vadose Zone J. 9, 362–372. doi:10.2136/vzj2009.0100
Cey, E. E., and Rudolph, D. L. (2009). Field Study of Macropore Flow Processes Using Tension Infiltration of a Dye Tracer in Partially Saturated Soils. Hydrol. Process 23, 1768–1779. doi:10.1002/hyp.7302
Chen, G., Feng, Q., and Wang, J. (2020). Mini-review of Microplastics in the Atmosphere and Their Risks to Humans. Sci. Total Environ. 703, 135504. doi:10.1016/j.scitotenv.2019.135504
Cook, F. J., and Orchard, V. A. (2008). Relationships between Soil Respiration and Soil Moisture. Soil Biol. Biochem. 40, 1013–1018. doi:10.1016/j.soilbio.2007.12.012
de Ruijter, V. N., Redondo-Hasselerharm, P. E., Gouin, T., and Koelmans, A. A. (2020). Quality Criteria for Microplastic Effect Studies in the Context of Risk Assessment: A Critical Review. Environ. Sci. Technol. 54, 11692–11705. doi:10.1021/acs.est.0c03057
de Souza Machado, A. A., Lau, C. W., Kloas, W., Bergmann, J., Bachelier, J. B., Faltin, E., et al. (2019). Microplastics Can Change Soil Properties and Affect Plant Performance. Environ. Sci. Technol. 53, 6044–6052. doi:10.1021/acs.est.9b01339
de Souza Machado, A. A., Lau, C. W., Till, J., Kloas, W., Lehmann, A., Becker, R., et al. (2018). Impacts of Microplastics on the Soil Biophysical Environment. Environ. Sci. Technol. 52, 9656–9665. doi:10.1021/acs.est.8b02212
Ding, L., Zhang, S., Wang, X., Yang, X., Zhang, C., Qi, Y., et al. (2020). The Occurrence and Distribution Characteristics of Microplastics in the Agricultural Soils of Shaanxi Province, in North-Western China. Sci. Total Environ. 720, 137525. doi:10.1016/j.scitotenv.2020.137525
Fuller, S., and Gautam, A. (2016). A Procedure for Measuring Microplastics Using Pressurized Fluid Extraction. Environ. Sci. Technol. 50, 5774–5780. doi:10.1021/acs.est.6b00816
Horn, R., and Dexter, A. R. (1989). Dynamics of Soil Aggregation in an Irrigated Desert Loess. Soil Tillage Res. 13, 253–266. doi:10.1016/0167-1987(89)90002-0
Horn, R., and Smucker, A. (2005). Structure Formation and its Consequences for Gas and Water Transport in Unsaturated Arable and Forest Soils. Soil Tillage Res. 82, 5–14. doi:10.1016/j.still.2005.01.002
Huang, Y., Zhao, Y., Wang, J., Zhang, M., Jia, W., and Qin, X. (2019). LDPE Microplastic Films Alter Microbial Community Composition and Enzymatic Activities in Soil. Environ. Pollut. 254, 112983. doi:10.1016/j.envpol.2019.112983
Jackson, C. R., Tyler, H. L., and Millar, J. J. (2013). Determination of Microbial Extracellular Enzyme Activity in Waters, Soils, and Sediments Using High Throughput Microplate Assays. JoVE 80, 50399. doi:10.3791/50399
Jambeck, J. R., Geyer, R., Wilcox, C., Siegler, T. R., Perryman, M., Andrady, A., et al. (2015). Plastic Waste Inputs from Land into the Ocean. Science 347, 768–771. doi:10.1126/science.1260352
Jayarathne, J. R. R. N., Chamindu Deepagoda, T. K. K., Clough, T. J., Thomas, S., Elberling, B., and Smits, K. M. (2021). Effect of Aggregate Size Distribution on Soil Moisture, Soil-Gas Diffusivity, and N2O Emissions from a Pasture Soil. Geoderma 383, 114737. doi:10.1016/j.geoderma.2020.114737
Kim, S. W., Waldman, W. R., Kim, T.-Y., and Rillig, M. C. (2020). Effects of Different Microplastics on Nematodes in the Soil Environment: Tracking the Extractable Additives Using an Ecotoxicological Approach. Environ. Sci. Technol. 54, 13868–13878. doi:10.1021/acs.est.0c04641
Lehmann, A., Fitschen, K., and Rillig, M. (2019). Abiotic and Biotic Factors Influencing the Effect of Microplastic on Soil Aggregation. Soil Syst. 3, 21. doi:10.3390/soilsystems3010021
Lehmann, A., Leifheit, E. F., Feng, L., Bergmann, J., Wulf, A., and Rillig, M. C. (2020a). Microplastic Fiber and Drought Effects on Plants and Soil Are Only Slightly Modified by Arbuscular Mycorrhizal Fungi. Soil Ecol. Lett. doi:10.1007/s42832-020-0060-4
Lehmann, A., Leifheit, E. F., Gerdawischke, M., and Rillig, M. C. (2020b). Microplastics Have Shape-And Polymer-dependent Effects on Soil Processes. bioRxiv. doi:10.1101/2020.06.02.130054
Liang, Y., Lehmann, A., Ballhausen, M.-B., Muller, L., and Rillig, M. C. (2019). Increasing Temperature and Microplastic Fibers Jointly Influence Soil Aggregation by Saprobic Fungi. Front. Microbiol. 10, 2018. doi:10.3389/fmicb.2019.02018
Liu, H., Yang, X., Liu, G., Liang, C., Xue, S., Chen, H., et al. (2017). Response of Soil Dissolved Organic Matter to Microplastic Addition in Chinese Loess Soil. Chemosphere 185, 907–917. doi:10.1016/j.chemosphere.2017.07.064
Liu, M., Lu, S., Song, Y., Lei, L., Hu, J., Lv, W., et al. (2018). Microplastic and Mesoplastic Pollution in Farmland Soils in Suburbs of Shanghai, China. Environ. Pollut. 242, 855–862. doi:10.1016/j.envpol.2018.07.051
Lozano, Y. M., Aguilar‐Trigueros, C. A., Onandia, G., Maaß, S., Zhao, T., and Rillig, M. C. (2021). Effects of Microplastics and Drought on Soil Ecosystem Functions and Multifunctionality. J. Appl. Ecol. doi:10.1111/1365-2664.13839
Ng, E. L., Lin, S. Y., Dungan, A. M., Colwell, J. M., Ede, S., Huerta Lwanga, E., et al. (2020). Microplastic Pollution Alters Forest Soil Microbiome. J. Hazard. Mater. 409, 124606. doi:10.1016/j.jhazmat.2020.124606
Nizzetto, L., Futter, M., and Langaas, S. (2016). Are Agricultural Soils Dumps for Microplastics of Urban Origin? Environ. Sci. Technol. 50, 10777–10779. doi:10.1021/acs.est.6b04140
Piehl, S., Leibner, A., Löder, M. G. J., Dris, R., Bogner, C., and Laforsch, C. (2018). Identification and Quantification of Macro- and Microplastics on an Agricultural Farmland. Sci. Rep. 8, 17950. doi:10.1038/s41598-018-36172-y
Potthoff, A., Oelschlägel, K., Schmitt-Jansen, M., Rummel, C. D., and Kühnel, D. (2017). From the Sea to the Laboratory: Characterization of Microplastic as Prerequisite for the Assessment of Ecotoxicological Impact. Integr. Environ. Assess. Manag. 13, 500–504. doi:10.1002/ieam.1902
Rillig, M. C., and Lehmann, A. (2020). Microplastic in Terrestrial Ecosystems. Science 368, 1430–1431. doi:10.1126/science.abb5979
Rillig, M. C. (2012). Microplastic in Terrestrial Ecosystems and the Soil?. Environ. Sci. Technol. 46, 6453–6454. doi:10.1021/es302011r
Rillig, M. C., Ryo, M., Lehmann, A., Aguilar-Trigueros, C. A., Buchert, S., Wulf, A., et al. (2019). The Role of Multiple Global Change Factors in Driving Soil Functions and Microbial Biodiversity. Science 366, 886–890. doi:10.1126/science.aay2832
Sarijan, S., Azman, S., Said, M. I. M., and Jamal, M. H. (2021). Microplastics in Freshwater Ecosystems: a Recent Review of Occurrence, Analysis, Potential Impacts, and Research Needs. Environ. Sci. Pollut. Res. 28, 1341–1356. doi:10.1007/s11356-020-11171-7
Scheurer, M., and Bigalke, M. (2018). Microplastics in Swiss Floodplain Soils. Environ. Sci. Technol. 52, 3591–3598. doi:10.1021/acs.est.7b06003
Schneider, A., Hirsch, F., Raab, A., and Raab, T. (2018). Dye Tracer Visualization of Infiltration Patterns in Soils on Relict Charcoal Hearths. Front. Environ. Sci. 6, 143. doi:10.10.3389/fenvs.2018.00143
Schweizer, S. A., Bucka, F. B., Graf-Rosenfellner, M., and Kögel-Knabner, I. (2019). Soil Microaggregate Size Composition and Organic Matter Distribution as Affected by Clay Content. Geoderma 355, 113901. doi:10.1016/j.geoderma.2019.113901
Sutherland, W. J., Fleishman, E., Clout, M., Gibbons, D. W., Lickorish, F., Peck, L. S., et al. (2019). Ten Years on: A Review of the First Global Conservation Horizon Scan. Trends Ecol. Evol. 34, 139–153. doi:10.1016/j.tree.2018.12.003
Thomas, D., Schütze, B., Heinze, W. M., and Steinmetz, Z. (2020). Sample Preparation Techniques for the Analysis of Microplastics in Soil-A Review. Sustainability 12, 9074. doi:10.3390/su12219074
Wan, Y., Wu, C., Xue, Q., and Hui, X. (2019). Effects of Plastic Contamination on Water Evaporation and Desiccation Cracking in Soil. Sci. Total Environ. 654, 576–582. doi:10.1016/j.scitotenv.2018.11.123
Wang, Y., Shao, M. a., and Liu, Z. (2013). Vertical Distribution and Influencing Factors of Soil Water Content within 21-m Profile on the Chinese Loess Plateau. Geoderma 193-194, 300–310. doi:10.1016/j.geoderma.2012.10.011
Yang, X., Bento, C. P. M., Chen, H., Zhang, H., Xue, S., Lwanga, E. H., et al. (2018). Influence of Microplastic Addition on Glyphosate Decay and Soil Microbial Activities in Chinese Loess Soil. Environ. Pollut. 242, 338–347. doi:10.1016/j.envpol.2018.07.006
Zhang, G. S., and Liu, Y. F. (2018). The Distribution of Microplastics in Soil Aggregate Fractions in Southwestern China. Sci. Total Environ. 642, 12–20. doi:10.1016/j.scitotenv.2018.06.004
Zhang, G. S., Zhang, F. X., and Li, X. T. (2019). Effects of Polyester Microfibers on Soil Physical Properties: Perception from a Field and a Pot Experiment. Sci. Total Environ. 670, 1–7. doi:10.1016/j.scitotenv.2019.03.149
Zhang, S., Yang, X., Gertsen, H., Peters, P., Salánki, T., and Geissen, V. (2018). A Simple Method for the Extraction and Identification of Light Density Microplastics from Soil. Sci. Total Environ. 616–617, 1056–1065. doi:10.1016/j.scitotenv.2017.10.213
Keywords: aggregates, fibers, films, heterogeneous pollution, water path
Citation: Kim SW, Liang Y, Zhao T and Rillig MC (2021) Indirect Effects of Microplastic-Contaminated Soils on Adjacent Soil Layers: Vertical Changes in Soil Physical Structure and Water Flow. Front. Environ. Sci. 9:681934. doi: 10.3389/fenvs.2021.681934
Received: 17 March 2021; Accepted: 26 April 2021;
Published: 12 May 2021.
Edited by:
Hongbiao Cui, Anhui University of Science and Technology, ChinaReviewed by:
Xuetao Guo, Northwest A and F University, ChinaYuyi Yang, Chinese Academy of Sciences, China
Copyright © 2021 Kim, Liang, Zhao and Rillig. This is an open-access article distributed under the terms of the Creative Commons Attribution License (CC BY). The use, distribution or reproduction in other forums is permitted, provided the original author(s) and the copyright owner(s) are credited and that the original publication in this journal is cited, in accordance with accepted academic practice. No use, distribution or reproduction is permitted which does not comply with these terms.
*Correspondence: Shin Woong Kim, c3draW1AemVkYXQuZnUtYmVybGluLmRl