- 1Center for Ecological Genomics and Wildlife Conservation, Department of Zoology, University of Johannesburg, Auckland Park, South Africa
- 2Department of Pest-Management and Conservation, Faculty of Agriculture and Life Sciences, Lincoln University, Lincoln, New Zealand
- 3Hungarian University of Agriculture and Life Sciences, Institute for Wildlife Management and Nature Conservation, Gödöllő, Hungary
- 4Department of Nature Conservation and Wildlife Management-Institute of Medical Microbiology, University of Debrecen, Debrecen, Hungary
- 5Bio-Protection Research Centre, Lincoln University, Lincoln, New Zealand
Large-scale monitoring of wild populations in remote areas using traditional live-capturing methods is logistically and financially challenging. Devices that can be used to obtain biological material remotely and store it for an extended period have considerable potential to monitor population densities and health status, but their applicability remains largely unexplored. The present study describes a device that collects trace amounts of DNA from the saliva of small mammals that is deposited on the surface of a collection medium (WaxTags®). The device’s performance was evaluated on Australian brushtail possums (Trichosurus vulpecula), an invasive pest species and the most significant vector of bovine tuberculosis infective agent (Mycobacterium bovis), under field conditions in Canterbury, New Zealand. The retrieved DNA was used to amplify eight possum-specific microsatellite markers and bacterial 16S rRNA. The design is mechanically robust, and the quality of the recovered DNA was adequate for microsatellite-based identification of individual possums, estimation of population density, and partial reconstruction of their oral microbiomes as a potential indicator of health. Several medically important bacteria, including strains of environmental Mycobacterium sp., were detected. The design can be refined to monitor other animals’ populations proactively and provide different levels of information necessary to manage wild populations.
Introduction
Recent developments in DNA sequencing technologies have made it possible to detect species’ presence from trace amounts of DNA they leave in the environment (Biggs et al., 2015; Thomsen and Willerslev, 2015; Webster et al., 2020).
Compared to the quality of the DNA retrieved from blood and tissue samples, environmental samples contain less template DNA, have lower amplification rates for genetic markers, are more prone to genotyping errors, and are likely to be contaminated by the presence of non-target DNA (Taberlet et al., 1996; Henry et al., 2011). In addition, exposure to environmental conditions, such as high temperature, wind, humidity, and UV radiation, negatively impact the recovery and sequencing of DNA from environmental samples (Nsubuga et al., 2004; Vargas et al., 2009; Duenas-Serrano, 2013; Harrison et al., 2019; Stewart, 2019).
Several techniques have been developed to collect DNA from marine and terrestrial animals. The common objective of these methods is to obtain high-quality template DNA from biological materials without the need for sedation or the risk of causing significant injury, and to minimize interfering with the target animals’ natural behaviour (Lefort et al., 2018).
The literature on the potential source of DNA for genetic studies is extensive and focuses primarily on biological materials, such as using faeces or regurgitates for the reconstruction of dietary items (Emami-Khoyi et al., 2016; Ntshudisane et al., 2021), genotyping for individual assignment or populations genetics using faeces (Mondol et al., 2009), urine (Valiere and Taberlet, 2000), hair (Mills et al., 2000; Hanke and Dickman, 2013), feathers (Peters et al., 2020), eggshells (Beja-Pereira et al., 2009), nail and horns (Gilbert et al., 2007), water-soluble adhesive tapes on the body surface (Sloane et al., 2000), footprints (Kinoshita et al., 2019), saliva (Nichols et al., 2012), gnawed wood (Aylward et al., 2018), blowhole exhalation (Frère et al., 2010), for the individual assignment or population genetics, and body swabs for microbiome studies (Solarz et al., 2020) (Figure 1). However, application of these methods has been hampered by the technical limitations of obtaining and preserving specimens under field conditions (Taberlet and Luikart, 1999; Bohmann et al., 2014). One such limitation is the need for optimal storage and preservation of samples until DNA extraction can be undertaken.
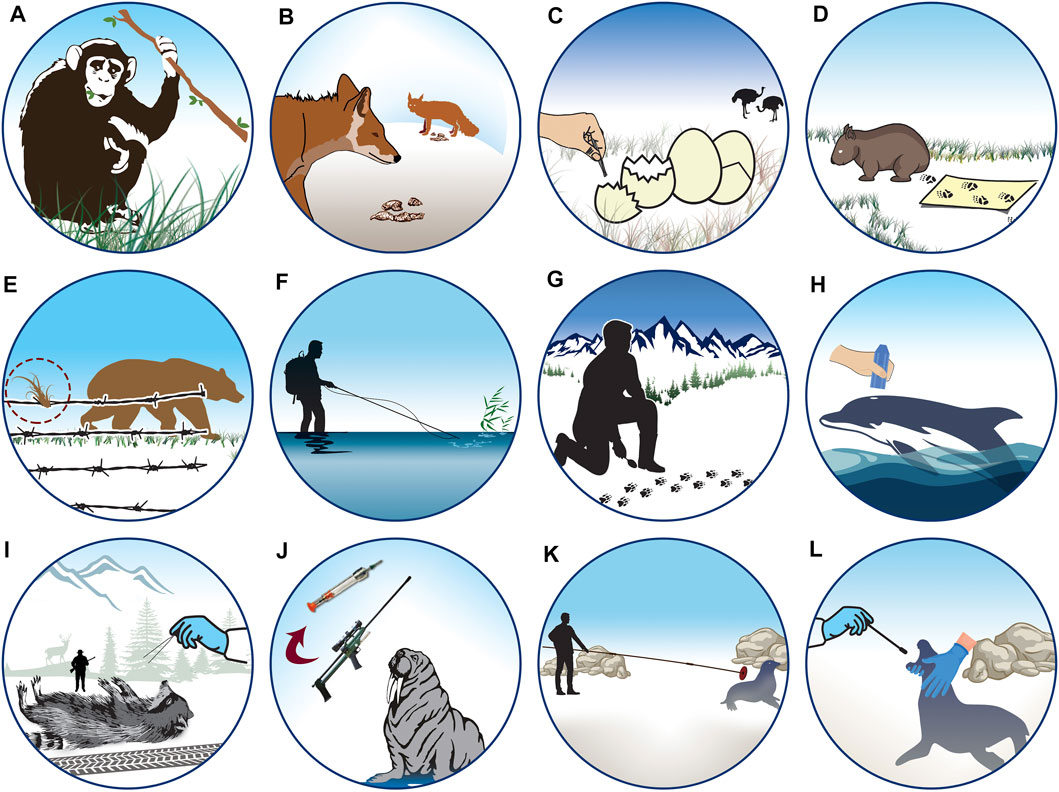
FIGURE 1. Depiction of some of the major means of non-intrusive eDNA sampling; (A) chewed sticks, (B) faeces, (C) eggshells, (D) adhesive tape trap, (E) hair snare, (F) filtering of water, (G) snow imprints, (H) blowhole exhalation, (I) body parts, (J) non-lethal remote dart biopsy, (K) hair stripping discs, (L) oral swabbing.
Large-scale application of DNA that has been collected non-invasively to study wildlife species requires the invention and optimization of methods that collect biological samples in the field, and subsequently protect them to yield DNA of the desired quality. The degree of preservation needed for environmental samples varies based on the type of tissue used. Furthermore, some non-invasive DNA collection methods require that researchers collect the specimens within a short time interval after biological materials are deposited in the environment, demanding intensive field presence and observation.
Mondol et al. (2009) reported individual genotyping limitation using DNA from faecal samples of tiger (Panthera tigris), whereas Wilson et al. (2003) had a higher success rate in individual genotyping when only DNA from freshly deposited badger (Meles meles) scats was used. In an experimental field study, Murphy et al. (2007) exposed freshly deposited brown bear (Ursus arctos) faeces to different environmental conditions in terms of humidity and ambient temperature, and found that the duration of time that specimens were exposed to the elements, and the average daily temperature, negatively impacted the recovery and the quality of retrieved DNA.
Several authors discussed the effectiveness of chemical buffers in preserving DNA from different types of tissues (Seutin et al., 1991; Al-Griw et al., 2017; Garg et al., 2018). However, physical protection of specimens from the elements under field condition has not received the same level of interest. The development of such methods extends the period for which DNA can be effectively used for genetic analysis, and eliminates the need for constant field presence in remote locations.
As efforts to eradicate zoonotic diseases have intensified globally, the potential of samples that can be collected non-invasively as a tool for the simultaneous monitoring of the vector populations and their disease status has remained mostly unexplored.
Our study region, New Zealand, was one of the last places on Earth to be colonized by humans (Wilmshurst et al., 2008). The archipelago’s ecological remoteness prevented mammals from achieving the same level of dominance as on other major continents, allowing wildlife to evolve under distinct evolutionary dynamics. However, two consecutive waves of human colonization resulted in large-scale hunting of marine and terrestrial animals (Emami-Khoyi et al., 2018), extensive habitat alteration (Taylor and Taylor, 1989; Meyer et al., 2015), and the introduction of several invasive species (Murphy et al., 2019). Among the introduced species, the negative impacts of the omnivorous Australian brushtail possum (Trichosurus vulpecula) on biodiversity have been particularly severe (Clout and Ericksen, 2000; Cowan and Glen, 2021). The common brushtail possum was introduced into New Zealand from Australia in the 19th century to establish a fur industry (Cowan and Glen, 2021). Following multiple introductions, large breeding populations established themselves throughout most of New Zealand’s diverse landscapes, and in the absence of natural predators and major competitors, the brushtail possum reached densities far beyond those found in their native Australian range. Brushtail possums heavily browse native forests and prey on endemic bird and insect species, which possess few adaptations to evade mammalian predators (Dowding and Murphy, 2001; Cowan and Glen, 2021). Furthermore, brushtail possums form the most significant wildlife reservoir for the causative agent of bovine tuberculosis (bTB), Mycobacterium bovis, which threatens New Zealand’s extensive dairy and farming industries, as well as posing a significant risk to public health (Livingstone et al., 2015).
The estimation of the population density and the prevalence of disease in vector populations has traditionally relied on the live capture of animals, followed by subsequent necroscopy, immunological, histopathological, and microbiological assays to identify the pathogenic agent or its biochemical signatures in the respiratory, digestive, and circulatory systems (Ramos et al., 2015). However, field monitoring of wild populations, especially in remote areas, tends to be logistically and financially challenging. Recently, new monitoring tools such as passive sensors, camera traps, Chew Cards or WaxTags®, have been tested successfully (Forsyth et al. 2018; Ahumada et al., 2020). These new methods are comparatively easy to deploy and monitor in the field; however, a trade-off is that new tools only report the presence or absence of a vector species in the environment and provide no information on the genetics or vector’s health status. While DNA has been extracted from the surface of both Chew Cards or WaxTags® with some success in enclosure trials (Vargas et al., 2009; Duenas-Serrano, 2013), the genetic profile of individuals that were obtained under field conditions is typically a mixed profile of multiple individuals that bit into a collection medium, and cannot be reliably used for genetic analysis.
This study describes the development and testing of a non-invasive method for the collection of trace amounts of DNA from saliva that small mammals deposit on the surface of a collection medium and which protects the DNA within the device’s housing unit until DNA extraction can be undertaken. The performance of the new method was tested on both captive and wild brushtail possums that bit into a specific collection medium, WaxTags®, and we investigated the applicability and adequacy of the recovered DNA to distinguish between different individuals, to estimate population density, and to partially reconstruct the oral microbiome to monitor disease status.
Method and Materials
Registering Weather Conditions During the Enclosure Trial
To identify any unusual weather conditions during the trial (such as high temperature, rainfall, wind and UV light) that could potentially affect the reproducibility of our results, the weather readings for the 14-days trial period were compared to the 10-year average reported for the same area and time of the year, registered by the Lincoln Broadfield Environmental Weather Station. Statistical significance of variation in weather readings during the trial period compared to the ten year average was tested using a t-test.
Testing of WaxTags® as a DNA Collection Medium
Prior to developing the DNA collection device, the suitability of WaxTags® as a DNA collection medium to extract DNA of desired quality following exposure to the environment was tested under captive conditions. Five brushtail possums (three males and two females) were live-captured and transferred to the Johnstone Memorial Laboratory (JML) at Lincoln University, New Zealand. The wildlife veterinary team assessed the health status of the animals upon arrival. Animals were then kept in separate outdoor enclosures, and all individuals were fed the same diet before the start of the trial. On the day of sample collection, 10 WaxTags® were presented in front of each animal’s mouth to trigger their natural biting behaviour. We allowed each animal to bite a sufficient portion of each WaxTag® before removing the tags. Five animals bit a total of 50 WaxTags®.
Five WaxTags® from each individual were directly exposed to the outdoor environmental conditions by hanging them from the top bars of a large metal cage. The remaining five WaxTags® were sheltered inside blue waterproof, UV protective plastic covers before exposing the covered tags to the same outdoor environmental conditions (Supplementary Figure S1).
WaxTags® were collected after 14 days of exposure to the elements, and the surface of each WaxTag® was thoroughly swabbed using sterile rayon-tip swabs (COPAN, Italy). The swab tips were immediately transferred into a 2 ml vial containing 0.70 ml Longmire lysis buffer. The solutions were treated with DXT and DX enzyme (Qiagen®, Germany) and incubated overnight at 56°C. Following the incubation step, each lysate was transferred into an automated QIAxtractor system (Qiagen®, Germany), and DNA was extracted from lysates following the standard protocols provided by the device’s manufacturer. Replicate extractions from protected (n = 5) and unprotected (n = 5) WaxTag® belonging to each animal were pooled into two separate tubes before microsatellite amplification.
Seven possum-specific microsatellite markers, Tv16, Tv19, Tv53, Tv54, Tv58, Tv64 and TvM1 (Taylor and Cooper 1998; Lam et al., 2000), were amplified from each extraction in a 10 μl Qiagen Type-it Microsatellite PCR master mix, containing 0.15 μM of each primer and 2 μl of template DNA. To increase the accuracy of the genotyping, amplicons from each individual were genotyped four times using a 3100 Genetic Analyzer (Applied Biosystems, United States). The Quality Index (QI) of genotyping for each locus, the number of successfully amplified microsatellite markers per individual, and a consensus genetic profile for each animal were reported using GeneMapper V4 (Chatterji and Pachter, 2006).
Development and Description of the DNA Collection Device
Following the testing of WaxTag® on captive possums, a device that could be used to remotely replicate the process of sample collection under both captive and field conditions was developed. The collection device consists of a cylindrical PVC tube with a baiter, lead sinker, catcher, and a magnet (Figure 2, Supplementary Figures S2, S3). The PVC pipe is freestanding. A medium-strength magnet that requires either 0.30 kg or 0.49 kg of force for removal and horizontal movement, respectively, are attached to the top half of the PVC tube by fixing a metal strap outside the device’s body. A series of metal lines connect the WaxTags® baiter to the magnet from one end, and to a lead sinker within the device’s central housing unit from other end. Once an animal’s bite triggers the device, the magnet moves forward, releases the baiter, and the sinker’s weight pulls the baiter around a 90° elbow bend and down into the shaft of the apparatus. Then, the collected samples are protected from the elements in the device’s central housing unit and multiple animals cannot reach and retrieve the bait source.
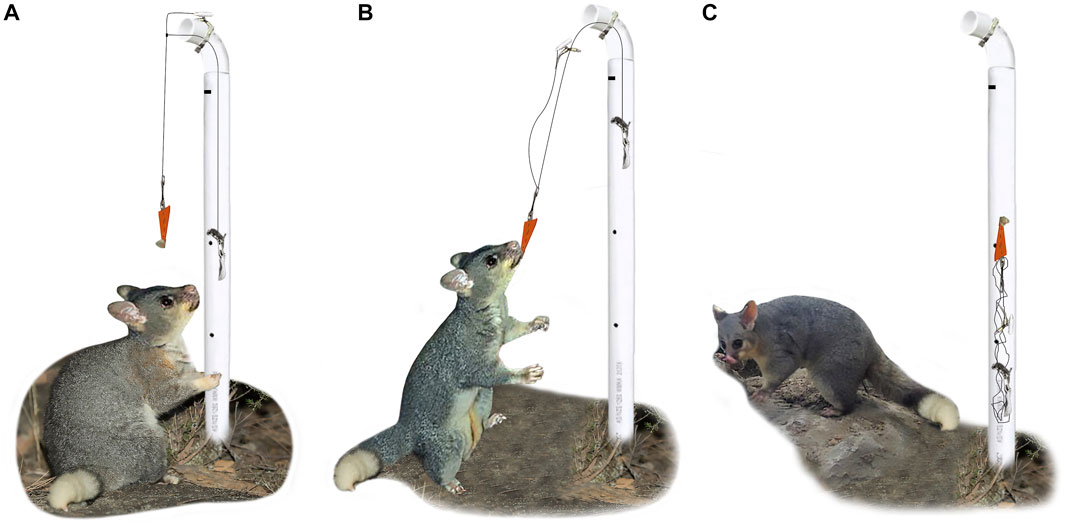
FIGURE 2. Sequence of events resulting in sample collection by the DNA collection device; (A) the animal inspects the device, (B) bites into the WaxTag® attached to the baiter (orange) and pulls the magnet away from the metal band, (C) gravity pulls the sinker into the central housing unit and the collected DNA is protected within the shaft of the apparatus (for more details see Supplementary Figures S2, S3).
The device’s simple design allows for adjustments depending on the species targeted, including the selection of the DNA collection medium, the magnet’s strength, the shape and distance of the baiter from ground level, and the PVC housing unit’s diameter.
Mechanical Testing of the Device Under Small-Scale Field Conditions
The efficacy of the DNA collection device in terms of field feasibility and practicality, as well as the behaviour of both target and non-target animals in response to its presence in the wild were tested under field conditions.
To this end, ten device prototypes were deployed in two semi-natural habitats in Hororata (n = 5, 13.7 hectares) and Burnham (n = 5, 4.0 hectares) in Canterbury, New Zealand, for three consecutive nights. The focus of the small-scale field evaluation was to test the design’s mechanical robustness and document animal interactions with the device. No genetic analysis was performed on the baiters at this stage.
Animals’ interactions with the DNA collection devices were recorded using a trail camera (Model Ltl-5210A 940 nm infrared, Ltl Acorn Outdoors, New Zealand) mounted adjacent to each collection station. Camera records from each interaction with the DNA collection devices were visually investigated, and behaviours were reported. Successful identification of interacting individuals was only reported when a trained observer could visually distinguish an individual based on its size, colouration, visible ear notches or other distinctive characteristics by analysing the camera records.
Microbiome Sample Collection and Amplification Under Captive Conditions
Following the field evaluation of the device’s performance, microbiome sample collection was conducted to determine if the same method can be applied to partially reconstruct the bacterial community present within saliva residues that animals leave on the surface of WaxTags®.
For this purpose, four WaxTags® were treated under UV light overnight to eliminate non-target DNA, and then were transferred to the JML laboratory. At the beginning of the enclosure trial, the WaxTags® were appended to the collection devices and placed separately into one of the enclosures. At the same time, four unprotected WaxTags® were suspended in each enclosure to serve as controls to quantify microorganisms present in the environment. WaxTags® from triggered devices were collected after successful interaction within 14 h of deployment, and portions that showed clear dental impressions (Figure 3) were swabbed using a sterile rayon-tip swab, and were subsequently excised. Swab tips and excised sections were immediately transferred into separate 5 ml tubes, each containing 200 mg of ≤106 µm glass beads (Sigma, United States) and 0.3 ml of Qiagen ATL buffer, supplemented with 20 mg/ml lysozyme (Thermo Fisher, United States). The suspension was incubated at 37° for 1 h, with moderate agitation using a stirrer plate. At the next step, 600 IU of Qiagen proteinase K was added to the suspension, and incubated at 60°C for 1 h; then, 0.3 ml of Qiagen AL buffer was added, and the samples were incubated at 70° for an additional 10 min. A Qiagen TissueLyser II was used for 3 min at 30 Hz to disrupt the solution by bead-beating, and the supernatants were separated by a brief centrifugation step, before being transferred to a tube containing 0.3 μl of ethanol. DNA was purified using a standard on-column purification method with Qiagen buffers AW1 and AW2 as washing agents. The final extractions were eluted in 70 μl 10 mM Tris buffer (pH 8.0). Extractions from each swab and excised WaxTags® were pooled into a single tube before marker amplification.
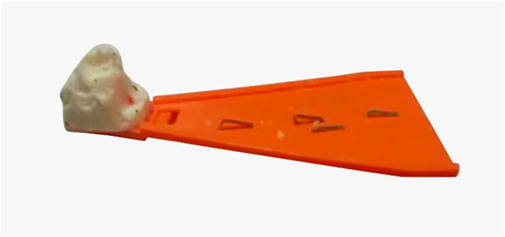
FIGURE 3. A baiter-holder (the orange object) with an attached WaxTag® (the white object on the left) showing a possum’s dental impressions.
The hypervariable regions (V1 and V2) of the 16S rRNA marker were amplified using primer pair 8F (5'-TCG TCG GCAGCG TCA GAT GTG TAT AAG AGA CAG AGA GTT TGA TCC TGG CTC AG-3') (Lane, 1991) and 338R (5'-GTC TCG TGG GCTCGG AGA TGT GTA TAA GAG ACA GGC TGC CTC CCG TAG GAG T-3') (Forney et al., 2010) as described in Emami-Khoyi et al. (2019). Three negative PCR controls that were prepared from chemical consumables without template DNA alongside three positive controls, which was metagenomic DNA extracted from human buccal swabs available commercially, were included during the amplification steps. The genomic libraries were prepared from amplicons, indexed separately, and sequenced on an Illumina MiSeq sequencing platform (Illumina, San Diego, United States) using 2 × 250 bp MiSeq Reagent Kit v3 at Chapel Hill Microbiome Core Facility, North Carolina University.
The resulting sequences were demultiplexed and analyzed using the QIIME2 v2020.8 pipeline (Bolyen et al., 2019). Briefly, the QIIME 2 Cutadapt v1.18 plugin (Martin, 2011) was used to remove the Illumina sequencing adapters, contaminants, and over-represented sequences. Sequences were then quality filtered, denoised and, when possible, merged into unique sequence features using the QIIME2 DADA2 plugin (Callahan et al., 2016). A taxonomic rank was assigned to each sequence feature based on the four best matches using QIIME2 “blast-consensus feature classifier plugin” by searching the Greengenes 13_8 99% OTUs full-length database (McDonald et al. 2012). Default settings were used except for minimum percentage identity and the p-value, which were set to 80% and 0.001, respectively.
To visualize the variation in the bacterial taxa identified from the collection media, a circular phylogenetic tree was constructed using the PhyloT online server (Letunic, 2015) and visualized in ITol v33 (Letunic and Bork, 2016). To estimate α and β diversity indices, all samples were first rarefied to a sequencing depth of 65,000. Rarefaction at this depth resulted in approximately 48.47% of the total sequences being retained in all specimens. Then, Shannon’s index (Shannon and Weaver, 1949), Faith’s phylogenetic diversity (Faith, 1992), Pielou’s evenness (Pielou, 1966), Bray-Curtis dissimilarity (Sørensen, 1948), Jaccard’s similarity index (Jaccard, 1908), unweighted Unifrac distance (Lozupone and Knight, 2005), and weighted normalized Unifrac distance (Lozupone et al., 2007) were estimated for each specimen.
Large-Scale Evaluation of the DNA Collection Device Under Field Condition
For a large-scale field trial, the final version of the DNA collection device was tested in a 146-ha podocarp-broadleaf forest in Omahu Bush on the Port Hills of Banks Peninsula. The study site was initially monitored using Chew Cards (Forsyth et al. 2018), and six brushtail possum “hot-spots” within the area were identified (Agnew, 2016). These sites were separated from each other by distances of more than 130 m, and DNA collection devices were deployed in clusters of 5–7 units in a cross-shaped pattern in each location.
All devices were checked daily for a week, followed by checks every seventh day for an additional 3 weeks. WaxTags® from successfully triggered devices were collected upon each field inspection and preserved in an isometric tube containing Longmire buffer until DNA extraction was undertaken within 24 h.
After completion of the 28-day trial, all devices were replaced with 42 leg-hold possum traps (Victor N1, hard-catch traps) for a total of six nights. All captured possums were humanely euthanized, and a tissue sample from the ear was collected for microsatellite marker genotyping. The DNA extraction and PCR procedure were identical to that described earlier, except for the addition of an extra microsatellite marker, Tv27 (Taylor and Cooper 1998; Lam et al., 2000), that was only optimized after the end of the enclosure trial, to the suite of microsatellite markers.
Consensus genetic profiles were generated from tissue samples, and basic descriptive statistics, including the number of alleles, observed heterozygosity, and expected heterozygosity, were estimated in GenAlEx v.6501 (Peakall and Smouse, 2012). The genetic profiles from tissue samples were compared to those genotyped from DNA devices, using Cervus v3.0.7 (Kalinowski et al., 2007).
To evaluate the device’s performance in the estimation of the population density, Huggins’ estimator of the population density incorporated in program MARK v8.2 (Cooch and White, 2002) was used. This method uses a mark-recapture framework and reports a maximum likelihood estimate of the population density that is based on the total number of uniquely identified animals (i.e., the total number of individuals uniquely identified based on the genetic profile) and the probability that an individual is re-encountered, one or more time during the course of the trial.
Results
None of the subject animals in the enclosure trial displayed any pathological signs during the initial clinical examination. Only minor gum tissue inflammation was observed, which is typical of wild possum populations. No pronounced weather deviations from the 10-year local average were observed over the enclosure trial; therefore, it was concluded that weather conditions have not negatively influenced the reproducibility of the observed results (Supplementary Figure S4).
Recovery of DNA From WaxTags®
The enclosure trial results showed that high-quality DNA could be extracted from the collection medium, and the chemical make-up of WaxTags® did not interfere with the extraction of DNA, using the protocols described in the Method and Materials.
At the end of the trial, three samples from the unprotected WaxTags® trial failed to produce any genetic profile, whereas all samples from protected WaxTags® were amplified for one or more microsatellite markers (Supplementary Figure S5). On average, the number of successfully amplified markers per individual from covered WaxTags® was higher than those genotyped from unprotected samples (22 versus 37%), although the number was highly variable. A sample size larger than that used in the current study is required to compare the two treatments conclusively. Nevertheless, enclosure trial results confirmed that DNA retrieved from the surface of WaxTags® could be effectively used for genetic analysis under both conditions; we therefore proceeded to the evaluation of the device under field conditions.
Mechanical Performance of the DNA Collection Device and Analysis of the Camera Records
Camera records in two small-scale field trials showed that individual possums were attracted to the device, and none of the observed interactions resulted in damage or removal of the device from mounting poles. At the first interaction, brushtail possums spent on average 152 s investigating the device before triggering it. Subsequent interactions lasted only 79 s on average. Eighty-seven percent of all devices were triggered after the first interactions, and the remaining 13% were triggered after subsequent interaction.
Minimal interaction from non-target species, mainly feral cats and wild birds, was observed, but these interactions did not result in the premature triggering of the DNA devices (Supplementary Table S1, S2). The camera records conclusively identified four individual possums from Hororata and seven individuals from Burnham, respectively. At the Burnham site, the population density estimate based on the number of individuals interacting with DNA collection devices was consistent with two other methods, Bite Mark Index (BMI, Forsyth et al., 2018) and Residual Trap Catch Index (RTCI, Glen, 2014), which were carried out at approximately the same time (Agnew, 2016). However, at the larger Hororata site, the estimated population density was significantly lower, suggesting that some individuals did not interact with the DNA collection device (Supplementary Table S3) (see Discussion). Overall, no major flaw in the mechanical design, or unusual avoidance behaviour of brushtail possums that could hamper large-scale deployment of the collection device, were observed.
Partial Oral Microbiome Sequencing and Analysis in the Enclosure Trial
Brushtail possums triggered all devices within 14 h in all enclosures. DNA was successfully extracted and amplified from all four samples. The Illumina sequencing produced 507,611 paired-end, quality-filtered sequences with a mean frequency of 126,902 sequences per WaxTag® (Supplementary Table S4). No amplification was observed from the three negative PCR controls that were made using PCR consumables without any template DNA, and all positive PCR controls from commercially available human buccal swabs were successfully amplified. Quality-filtered sequences were dereplicated into 4,171 unique sequence features by the DADA2 plugin. Bacteria belonging to 30 phyla, 65 classes, 100 orders, 163 families, 271 genera, and 100 known species were identified (Figure 4). Strains of Acidobacteria, Actinobacteria, Armatimonadetes, Bacteroidetes, Chlorobi, Chloroflexi, Cyanobacteria, Fibrobacteres, Firmicutes, Fusobacteria, Gemmatimonadetes, Planctomycetes, Proteobacteria, Spirochaetes, Synergistetes, Tenericutes, Thermotogae, Verrucomicrobia, and Thermi; as well as the candidate phyla: AD3, BRC1, FBP, GN02, OD1, OP11, SR1, TM6, TM7, WPS-2, and WS3, were identified from the surfaces of the four WaxTags®. The six dominant bacterial phyla identified from WaxTags® were Proteobacteria, Cyanobacteria, Actinobacteria, Firmicutes, Bacteroidetes, and Fusobacteria. Other taxa were comparatively less abundant, accounting for less than 2% of the total abundance.
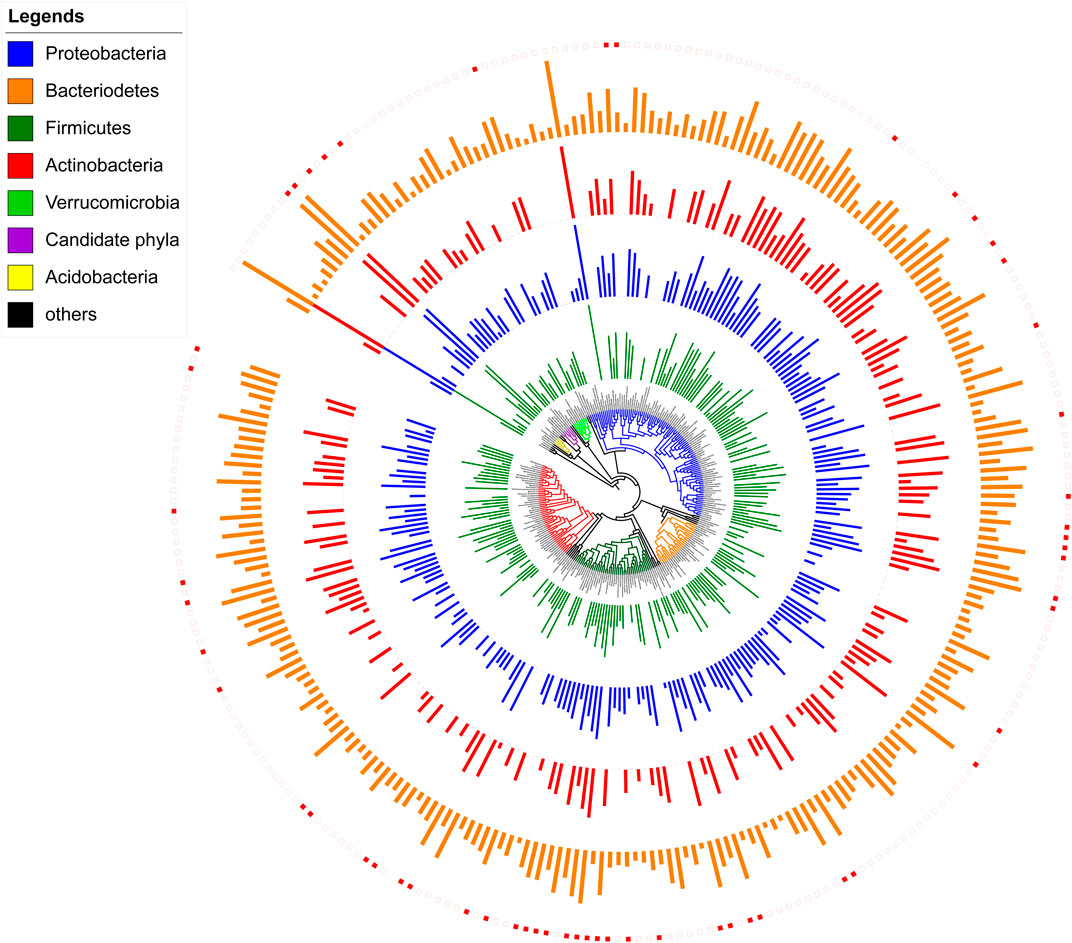
FIGURE 4. A phylogenetic tree reconstructed from 16S rRNA sequences illustrating different bacterial taxa and their abundance from DNA within brushtail possum’s saliva, deposited on the surface of the WaxTags®. The four circles of bars surrounding the central phylogeny (in red, blue, green and orange) represent the four possums. The height of each bar is proportional to the relative abundance of each taxon, at a logarithmic scale. Solid encircling rectangle boxes represent taxa previously reported from the oral cavity of brushtail possums, and clear boxes represent novel taxa reported in this study. The raw data for generating this figure are available in the Supplementary Materials, p_microbiome.txt.
DNA extractions from WaxTags® that were used to quantify the presence of non-target DNA in the environment failed to produce any sequence. It is likely that the small surface of the WaxTags®, which limits the number of environmental microorganisms deposited on the surface of the collection medium, combined with a higher rate of DNA degradation, or an unidentified methodological artefact during metagenomic library preparation, reduced the size of DNA into fragments smaller than the size of the selected genetic marker. As a consequence, we could not quantify the presence of non-target DNA; however, the adequacy and applicability of this method to monitor vector populations does not require quantification of the non-target DNA present in the environment (see Discussion).
Alpha and beta diversity indices varied noticeably between different WaxTags® (Supplementary Figures S6–9). While part of this variation is due to intra-individual variation in the diversity of oral communities, the remainder may reflect the stochasticity in reconstructing the oral microbiota from the surface of collection media and non-target DNA present in the environment (Supplementary Tables S5, S6).
Large-Scale Evaluation of the DNA Collection Device Under Field Conditions
In the large-scale Omahu Bush trial, all tissue samples from trapped possums (n = 22) were successfully genotyped for the selected suite of microsatellite markers (Supplementary Table S7). Individual assignment analysis indicated that genotyping at least seven microsatellite markers is required to identify non-related individuals with a probability of misidentification (PI) < 0.00003. Similarly, a minimum of eight markers is necessary to differentiate between siblings with a PI < 0.004 (Supplementary Figure S10). Therefore, the selected panel of eight microsatellite markers was informative to distinguish between individuals in the study area.
Out of a total of 122 collected WaxTags®, DNA was successfully extracted and genotyped from 83 (68%) samples. The microsatellite profiles from eight WaxTags® showed a mixed pattern, probably due to delayed retraction or multiple animals squabbling at the time of triggering the device, and these were removed from the rest of the analysis, bringing the total number of analysed WaxTags® to 75.
To increase the accuracy of individual assignment and population density estimates, only a subset of 35 genetic profiles for which the quality of genotyping was estimated to be more than 0.5, was subselected for downstream analysis.
The genetic profile of 10 samples (29%) matched those obtained from the trapped possums, and the remaining 25 samples (71%) originated from individuals not trapped during the live-capture trial. Ten different individuals were reliably identified, one of which was found to have triggered twelve devices in the various locations in the area. Among others, 30% of identified possums re-encountered DNA collection devices more than once; they were used for the density estimation, resulting in a population density estimation of 32.4 (±11.8 SE) individuals for the study site (Supplementary Table S8).
Discussion
The current study is the first to describe a DNA collection method that simultaneously collects trace amounts of DNA within the saliva of small-sized mammals, and then protects the collected DNA within the device until the downstream genetic analysis is undertaken. The current design’s performance and adequacy for individual identification, estimation of the population density, and partial reconstruction of oral microbiomes were successfully tested on invasive Australian brushtail possums in New Zealand.
Our study adds to the growing body of evidence that emphasises the need to develop new methodologies to expand the application of DNA that has been collected non-invasively under field conditions, and to mitigate the impacts of long-term exposure to the elements on the integrity and recovery of DNA from environmental samples (Ravant et al., 2001; Nsubuga et al., 2004; McKelvey and Schwartz, 2005; Vargas et al., 2009; Duenas-Serrano, 2013; Strickler et al., 2015; Stewart, 2019).
Agnew (2016) found that the negative effects of exposure to the elements on unprotected WaxTags® were more pronounced as exposure exceeded 7 days. In the same study, only 20% of the possums were identified from unprotected WaxTags®, whereas shielding the WaxTags® resulted in the identification of all individuals in the trial.
Individuals differ in the size and intensity of their bites (Sakata, 2011), and this variation potentially affects the amount of saliva deposition, DNA recovery, as well as the accuracy of genetic profiles that are created from WaxTags®. A large quantity of DNA within the saliva originates from oral epithelial cells. Forensic studies on human “touch DNA” report significant inter-individual variation in the number and frequency of cells that an individual may shed (Tan et al., 2019; Goray and van Oorschot, 2021). We suggest that a similar variation in the “shedder status” among individual brushtail possums could exist, causing lower genotyping success in individuals with weaker bite force. Observations further substantiate this idea, as bites from some individuals consistently fail to produce reliable genetic profiles even on the day of specimen collection, when no significant DNA degradation is expected (James Ross, personal communication).
The analysis of the camera records in two small-scale field trials confirmed that the current design was mechanically robust, and the interference from non-target animals was minimal. Moreover, animals were attracted to the device, and they did not demonstrate any avoidance behaviour. At the Burnham site, the population density estimate based on the number of individuals that interacted with the devices was consistent with those obtained from the other two methods. However, at the larger Hororata site, the population density estimates were lower, indicating that some individuals did not interact with the devices or, alternatively, they could not be identified based on visual features. The density of the DNA devices at this site was lower than at the Burnham site (0.36 versus 1.25 devices per hectare), partly explaining the downward bias in estimation.
Brushtail possums exhibit complex behaviours in response to unfamiliar objects in their environment (Sjoberg, 2013). The DNA collection device requires a high level of interaction by the subject animals. The Hororata site was subjected to intensive population control operations before the start of the field trial, and we cannot rule out that the development of “trap shyness” (Nugent et al., 2010) could have caused temporary avoidance behaviours towards the DNA devices in the remaining individuals.
Larger-scale evaluation of the DNA collection device in the 146-hectare Omahu Bush indicated that the 68% success rate in genotyping possum-specific microsatellite markers from DNA collected during our study was a significant improvement compared to earlier studies that used unprotected saliva (Duenas-Serrano, 2013), or brushtail possums’ faecal samples that were collected under field conditions after 2 days exposure to the elements (Morgan et al., 2007).
Individual brushtail possums in Omahu Bush varied in their attraction to the DNA collection devices and leg-hold traps, and this variation in individual behaviour needs to be taken into consideration to achieve a more accurate estimation of the population density.
Transmission of infectious disease in vector populations is typically density-dependent, and inaccurate estimation of the survival rate based on a single method can result in a premature end to population control operations and the emergence of new vector populations.
The combined application of various methods will undoubtedly provide environmental managers with the information necessary to implement a successful ecosystem management plan.
Large-scale deployment of the DNA collection device in an area makes it possible to create an encounter history for uniquely identified individuals, and this information can be used to estimate population density.
We estimated a population density of 32.4 (95% CI 21.1–75.6) possums for Omahu Bush, which was consistent with the total number of trapped possums (n = 22) and those uniquely identified from the DNA device (n = 10); however, this estimate had moderate precision due to the limited number of secondary encounters with DNA collection devices, resulting in an average probability of detection of 16%.
Optimizing the deployment of the DNA collection devices in terms of greater density, increased duration of the monitoring period, improved device visibility, and allure to the target animals will improve the probability of its detection and the accuracy of population density estimates.
Partial Oral Microbiome Reconstruction
Individuals in the current study showed no clinical sign of bTB infection before the start of the enclosure trial, and this assessment was confirmed by the apparent absence of Mycobacterium bovis from the oral microbiome. However, the identification of several medically significant bacteria, such as members of HACEK, Pasteurellaceae, Brucellaceae, nontuberculous Mycobacterium spp. (NTM), Glaesserella parasuis (the causative agent of swine Glässer’s disease), Pseudomonas sp., Clostridium sp., Staphylococcus sp., Streptococcus sp., Capnocytophaga sp., and Prevotella sp. from the surface of the WaxTags®, suggests that the same methodology can potentially be applied to test for the presence of Mycobacterium bovis and other pathogenic organisms that reside within the oral cavity. Some of the bacterial taxa from the surface of WaxTags® are asymptotic inhabitants of the upper respiratory system and the oral cavity in mammals (Talan et al., 1999; Dewhirst et al., 2012; Cheng et al., 2015; Gomez et al., 2017; Emami-Khoyi et al., 2020), while others are environmental microorganisms that live ubiquitously in different terrestrial and aquatic habitats. In a subset of hosts with compromised immune system, these microorganisms can cause severe localised or systemic diseases, and infected individuals form new reservoirs to spread the infection.
One major limitation of reconstructing the oral microbiome from environmental samples is a large quantity of non-target DNA that is sequenced concurrently. This is particularly relevant for some pathogens, such as Mycobacterium spp., as the genus comprises numerous strains, and each strain has a differing degree of pathogenicity and colonizes a different habitat (Tortoli et al., 2017; Gupta et al., 2018). Moreover, comparatively lower concentrations of M. bovis in the oral cavity compared to their concentration in the mucus originating deeper within the respiratory system, such as sputum from the trachea or bronchi, make direct detection by mean of sequencing a challenging task. To differentiate between different strains, the development of shorter, strain-specific genetic markers (Ullah et al., 2020) is paramount, and for this purpose, more comparative genomic studies on different pathogenic strains are required (Engel et al., 2012; Wilbur et al., 2012; Wood et al., 2015). The confounding effects of non-target DNA are more concerning in comprehensive surveys of microbiome diversity from a specific organ. These adverse effects are of less concern when a particular group of pathogenic taxa can be selectively targeted in a pool of target and non-target DNA; however, the performance of mixed DNA from the surface of WaxTags® to distinguish the source of an infective agent that shares habitat between a host and its surrounding environments remains inconclusive.
Detection of sequences that are matched bioinformatically to a known pathogen does not necessarily mean that the hosts are clinically ill, nor does it indicate that the source of the pathogen’s DNA was a living cell. Furthermore, it does not explain whether the vector animal is currently present in the area or whether it has dispersed after initial identification. A conclusive link between infection and infective agent requires specific immunological, histopathological, and biochemical diagnostic tests on animals suspected of being infected (Emami-Khoyi et al., 2019). Nevertheless, early identification of sequences with significant similarity to a pathogenic agent, independent of its exact aetiology, merits an in-depth survey of populations suspected to be infected, and more rigorous testing is required to prevent the emergence of new reservoirs of the pathogenic agent.
Conclusion
The DNA collection device described in the current study provides a cost-efficient and easy to deploy tool for monitoring wild populations of small mammals under field conditions. The current design is heavier than other widely used non-invasive methods, such as WaxTags® and Chew Cards, yet lighter than traditional leg-holds or cage traps. This makes it particularly suitable for large-scale monitoring of wild populations in remote areas. The successful amplification of mammalian DNA (microsatellites) and bacterial 16S rRNA confirm that the current design can provide information on individuals’ genetics, relatedness and, potentially, disease status. Similar methods can be refined to study other invasive, rare, and elusive species in remote areas. In addition, a design that uses remotely triggered camera traps adjacent to the DNA collection stations can simultaneously provide information about target species’ behaviours.
Data Availability Statement
The datasets presented in this study can be found in online repositories. The names of the repository/repositories and accession number(s) can be found at: BioProject ID PRJNA725379.
Ethics Statement
Animal handling and microbiome specimen collection for this study was approved by the Lincoln University Animal Ethics Committee (SOP 29–14).
Author Contributions
Conceptualization, JR, EM,TA, AP, HA, and AE-K; methodology, JR, EM, TA, AE-K, AP, and HA; validation, JR, EM, TA, and AE-K; formal analysis, TA, AE-K, MA, AB, DM, SP, DM, CS, RR, and TG; resources, JR, BV, EM, PT, and SC; writing original draft preparation, AE-K, PT, TA, MA, AB, DM, SP, DM, CS, RR, TG, JR, EM, BV, CS, MH, and HA; funding acquisition, JR, BV, EM, PT, and SC; all authors have read and agreed to the current version of the manuscript.
Conflict of Interest
The authors declare that the research was conducted in the absence of any commercial or financial relationships that could be construed as a potential conflict of interest.
Publisher’s Note
All claims expressed in this article are solely those of the authors and do not necessarily represent those of their affiliated organizations, or those of the publisher, the editors and the reviewers. Any product that may be evaluated in this article, or claim that may be made by its manufacturer, is not guaranteed or endorsed by the publisher.
Acknowledgments
We would like to thank AS, AB, NS, Sina Rhmaty, and an anonymous reviewer for their constructive comments on the initial version of the manuscript. Ali Labbaf Tehrani is acknowledged for the creation of the graphical illustrations. AE-K and DM received bursaries from South Africa’s National Antarctic Program (SANAP) Grant No. 110728 to BJ. The computational resources for the current study were provided by the Center for High-Performance Computing (CHPC) in Cape Town, the University of Johannesburg IT services, and the Hungarian University of Agriculture and Life Science.
Supplementary Material
The Supplementary Material for this article can be found online at: https://www.frontiersin.org/articles/10.3389/fenvs.2021.701033/full#supplementary-material
References
Agnew, T. W. (2016). Assessing Proposed Designs for a Non-invasive DNA Collection Device Suitable for Use in Possum (Trichosurus vulpecula) Population Monitoring. Master’s Thesis. Canterbury: Lincoln University.
Ahumada, J. A., Fegraus, E., Birch, T., Flores, N., Kays, R., and O’Brien, T. G. (2020). Wildlife Insights: A Platform to Maximize the Potential of Camera Trap and Other Passive Sensor Wildlife Data for the Planet. Environ 47 (1), 1–6. doi:10.1017/S0376892919000298
Al-Griw, H. H., Zraba, Z. A., Al-Muntaser, S. K., Draid, M. M., Zaidi, A. M., Tabagh, R. M., et al. (2017). Effects of Storage Temperature on the Quantity and Integrity of Genomic DNA Extracted from Mice Tissues: A Comparison of Recovery Methods. Open J. Vet. Med. 7 (3), 239–243. doi:10.4314/ovj.v7i3.7
Aylward, M. L., Sullivan, A. P., Perry, G. H., Johnson, S. E., and Louis, E. E. (2018). An Environmental DNA Sampling Method for Aye-Ayes from Their Feeding Traces. Ecol. Evol. 8 (10), 9229–9240. doi:10.1002/ece3.4341
Beja-Pereira, A., Oliveira, R., Alves, P. C., Schwartz, M. K., and Luikart, G. (2009). Advancing Ecological Understandings through Technological Transformations in Noninvasive Genetics. Mol. Ecol. Resour. 9 (5), 1279–1301. doi:10.1111/j.1755-0998.2009.02699.x
Biggs, J., Ewald, N., Valentini, A., Gaboriaud, C., Dejean, T., Griffiths, R. A., et al. (2015). Using eDNA to Develop a National Citizen Science-Based Monitoring Programme for the Great Crested Newt (Triturus Cristatus). Biol. Conserv. 183, 19–28. doi:10.1016/j.biocon.2014.11.029
Bohmann, K., Evans, A., Gilbert, M. T. P., Carvalho, G. R., Creer, S., Knapp, M., et al. (2014). Environmental DNA for Wildlife Biology and Biodiversity Monitoring. Trends Ecol. Evol. 29 (6), 358–367. doi:10.1016/j.tree.2014.04.003
Bolyen, E., Rideout, J. R., Dillon, M. R., Bokulich, N. A., Abnet, C. C., Al-Ghalith, G. A., et al. (2019). Reproducible, Interactive, Scalable and Extensible Microbiome Data Science Using QIIME 2. Nat. Biotechnol. 37 (9), 852–857. doi:10.1038/s41587-019-0209-9
Callahan, B. J., McMurdie, P. J., Rosen, M. J., Han, A. W., Johnson, A. J. A., and Holmes, S. P. (2016). Dada2: High-Resolution Sample Inference from Illumina Amplicon Data. Nat. Methods 13 (7), 581–583. doi:10.1038/nmeth.3869
Chatterji, S., and Pachter, L. (2006). Reference Based Annotation with GeneMapper. Genome Biol. 7 (4), R29. doi:10.1186/gb-2006-7-4-r29
Cheng, Y., Fox, S., Pemberton, D., Hogg, C., Papenfuss, A. T., and Belov, K. (2015). The Tasmanian Devil Microbiome-Implications for Conservation and Management. Microbiome 3, 76. doi:10.1186/s40168-015-0143-0
Clout, M., and Ericksen, K. (2000). “Anatomy of a Disastrous Success: The Brushtail Possum as an Invasive Species,” in The Brushtail Possum: Biology, Impact and Management of an Introduced Marsupial. Editor L. Montague (New Zealand: Manaaki Whenua Press), 1–9.
Cowan, P. E., and Glen, A. S. (2021). “Family Phalangeridae: Trichosurus vulpecula,” in The Handbook of New Zealand Mammals. Editors C.M. King, and D.M. Forsyth. 3rd ed. (Melbourne: CSIRO Publishing)), 43–77.
Dewhirst, F. E., Klein, E. A., Thompson, E. C., Blanton, J. M., Chen, T., Milella, L., et al. (2012). The Canine Oral Microbiome. PLoS One 7 (4), 1–12. doi:10.1371/journal.pone.0036067
Dowding, J. E., and Murphy, E. C. (2001). The Impact of Predation by Introduced Mammals on Endemic Shorebirds in New Zealand: A Conservation Perspective. Biol. Conserv. 99 (1), 47–64. doi:10.1016/S0006-3207(00)00187-7
Duenas-Serrano, J. F. (2013). Development and Evaluation of a Protocol to Identify Individuals of Trichosurus vulpecula with Non-invasively Recovered DNA. Master’s ThesisCanterbury: Lincoln University.
Emami-Khoyi, A., Benmazouz, I., Paterson, A. M., Ross, J. G., Murphy, E. C., Bothwell, J., et al. (2020). Oral Microbiome Metabarcoding in Two Invasive Small Mammals from New Zealand. Diversity 12 (7), 278. doi:10.3390/d12070278
Emami-Khoyi, A., Benmazouz, I., Ross, J. G., Boren, L. J., Murphy, E. C., Jansen van Vuuren, B., et al. (2019). A Survey of the Oral Cavity Microbiome of New Zealand Fur Seal Pups (Arctocephalus Forsteri). Mar. Mam. Sci. 36 (1), 334–343. doi:10.1111/mms.12639
Emami-Khoyi, A., Hartley, D. A., Paterson, A. M., Boren, L. J., Cruickshank, R. H., Ross, J. G., et al. (2016). Identifying Prey Items from New Zealand Fur Seal (Arctocephalus Forsteri) Faeces Using Massive Parallel Sequencing. Conserv. Genet. Resour. 8 (3), 343–352. doi:10.1007/s12686-016-0560-9
Emami-Khoyi, A., Paterson, A. M., Hartley, D. A., Boren, L. J., Cruickshank, R. H., Ross, J. G., et al. (2018). Mitogenomics Data Reveal Effective Population Size, Historical Bottlenecks, and the Effects of Hunting on New Zealand Fur Seals (Arctocephalus Forsteri). Mitochondrial DNA A DNA Mapp. Seq. Anal. 29 (4), 567–580. doi:10.1080/24701394.2017.1325478
Engel, G. A., Wilbur, A. K., Westmark, A., Horn, D., Johnson, J., and Jones-Engel, L. (2012). Naturally Acquired Mycobacterium tuberculosis Complex in Laboratory Pig-Tailed Macaques. Emerg. Microbes Infect. 1, 10. doi:10.1038/emi.2012.31
Faith, D. P. (1992). Conservation Evaluation and Phylogenetic Diversity. Biol. Conserv. 61 (1), 1–10. doi:10.1016/0006-3207(92)91201-3
Forney, L. J., Gajer, P., Williams, C. J., Schneider, G. M., Koenig, S. S., McCulle, S. L., et al. (2010). Comparison of Self-Collected and Physician-Collected Vaginal Swabs for Microbiome Analysis. J. Clin. Microbiol. 48 (5), 1741–1748. doi:10.1128/JCM.01710-09
Forsyth, D. M., Perry, M., Moloney, P., McKay, M., Gormley, A. M., Warburton, B., et al. (2018). Calibrating Brushtail Possum (Trichosurus vulpecula) Occupancy and Abundance Index Estimates from Leg-Hold Traps, Wax Tags and Chew Cards in the Department of Conservation’s Biodiversity and Monitoring Reporting System. N. Z. J. Ecol. 42 (2), 179–191. doi:10.20417/nzjecol.42.20
Frère, C. H., Krzyszczyk, E., Patterson, E. M., Hunter, S., Ginsburg, A., and Mann, J. (2010). Thar She Blows! A Novel Method for DNA Collection from Cetacean Blow. PLoS ONE 5, 8. doi:10.1371/journal.pone.0012299
Garg, R. K., Sengar, K., and Singh, R. K. (2018). Comparative Analyses of Genomic DNA Extracted from Freshwater Fish Tissues Preserved in Formaldehyde and Alcohol in Different Periods of Time. J. Appl. Biol. Biotechnol. 6 (4), 26–31. doi:10.9790/300810.7324/jabb.2018.60405
Gilbert, M. T. P., Djurhuus, D., Melchior, L., Lynnerup, N., Worobey, M., Wilson, A. S., et al. (2007). mtDNA from Hair and Nail Clarifies the Genetic Relationship of the 15th century Qilakitsoq Inuit Mummies. Am. J. Phys. Anthropol. 133 (2), 847–853. doi:10.1002/ajpa.20602
Glen, Al. (2014). Animal Pests: Residual Trap Catch Index for Possums. Department of Conservation. DOCDM-1414383.
Gomez, A., Espinoza, J. L., Harkins, D. M., Leong, P., Saffery, R., Bockmann, M., et al. (2017). Host Genetic Control of the Oral Microbiome in Health and Disease. Cell Host Microbe 22 (3), 269–278. doi:10.1016/j.chom.2017.08.013
Goray, M., and van Oorschot, R. A. H. (2021). Shedder Status: Exploring Means of Determination. Sci. Justice 61 (4), 391–400. doi:10.1016/j.scijus.2021.03.004
Gupta, R. S., Lo, B., and Son, J. (2018). Phylogenomics and Comparative Genomic Studies Robustly Support Division of the Genus Mycobacterium into an Emended Genus Mycobacterium and Four Novel Genera. Front. Microbiol. 9 (67), 1–41. doi:10.3389/fmicb.2018.00067
Hanke, P. U., and Dickman, C. R. (2013). Sniffing Out the Stakes: Hair-Snares for Wild Cats in Arid Environments. Wildl. Res. 40 (1), 45–51. doi:10.1071/WR12210
Harrison, J. B., Sunday, J. M., and Rogers, S. M. (2019). Predicting the Fate of eDNA in the Environment and Implications for Studying Biodiversity. Proc. R. Soc. B. 286, 1–9. doi:10.1098/rspb.2019.1409
Henry, P., Henry, A., and Russello, M. A. (2011). A Non-invasive Hair Sampling Technique to Obtain High Quality DNA from Elusive Small Mammals. J. Vis. Exp. 49, 49. doi:10.3791/2791
Jaccard, P. (1908). Nouvelles Recherches Sur la Distribution Florale. Bull. de La Soc. Vaud. Des Sci. Nat. 44 (163), 223–270. doi:10.5169/seals-268384
Kalinowski, S. T., Taper, M. L., and Marshall, T. C. (2007). Revising How the Computer Program CERVUS Accommodates Genotyping Error Increases Success in Paternity Assignment. Mol. Ecol. 16, 1099–1106. doi:10.1111/j.1365-294X.2007.03089.x
Kinoshita, G., Yonezawa, S., Murakami, S., and Isagi, Y. (2019). Environmental DNA Collected from Snow Tracks Is Useful for Identification of Mammalian Species. Zool. Sci. 36 (3), 198–207. doi:10.2108/zs180172
Lam, M. K-P., Hickson, R. E., Cowan, P. E., and Cooper, D. W. (2000). A Major Histocompatibility (MHC) Microsatellite Locus in Brushtail Possums (Trichosurus vulpecula). Onl. J. Vet. Res. 4 (4), 139–141.
Lane, D. (1991). “16s/23s rRNA Sequencing,” in Nucleic Acid Techniques in Bacterial Systematics. Editors E. Stackebrandt, and M. Goodfellow (New York: John Wiley & Sons).
Lefort, M. C., Cruickshank, R. H., Descovich, K., Adams, N. J., Barun, A., Emami-Khoyi, A., et al. (2018). Blood, Sweat and Tears: A Review of Non-invasive DNA Sampling. bioRxiv [Preprint] Available at: https://www.biorxiv.org/content/10.1101/385120v4.
Letunic, I., and Bork, P. (2016). Interactive Tree of Life (Itol) V3: An Online Tool for the Display and Annotation of Phylogenetic and Other Trees. Nucleic Acids Res. 44 (W1), W242–W245. doi:10.1093/nar/gkw290
Letunic, I. (2015). Phylot: Phylogenetic Tree Generator. Available at: https://phylot.biobyte.de/ (Accessed, 2020).
Livingstone, P. G., Hancox, N., Nugent, G., and De Lisle, G. W. (2015). Toward Eradication: The Effect of Mycobacterium Bovis Infection in Wildlife on the Evolution and Future Direction of Bovine Tuberculosis Management in New Zealand. N. Z. Vet. J. 63, 4–18. doi:10.1080/00480169.2014.971082
Lozupone, C. A., Hamady, M., Kelley, S. T., and Knight, R. (2007). Quantitative and Qualitative Beta Diversity Measures Lead to Different Insights into Factors that Structure Microbial Communities. Appl. Environ. Microbiol. 73 (5), 1576–1585. doi:10.1128/AEM.01996-06
Lozupone, C., and Knight, R. (2005). UniFrac: A New Phylogenetic Method for Comparing Microbial Communities. Appl. Environ. Microbiol. 71 (12), 8228–8235. doi:10.1128/AEM.71.12.8228-8235.2005
Martin, M. (2011). Cutadapt Removes Adapter Sequences from High-Throughput Sequencing Reads. Embnet. J. 17 (1), 10–12. doi:10.14806/ej.17.1.200
McDonald, D., Price, M. N., Goodrich, J., Nawrocki, E. P., DeSantis, T. Z., Probst, A., et al. (2012). An Improved Greengenes Taxonomy with Explicit Ranks for Ecological and Evolutionary Analyses of Bacteria and Archaea. ISME J. 6 (3), 610–618. doi:10.1038/ismej.2011.139
McKelvey, K. S., and Schwartz, M. K. (2005). DROPOUT: A Program to Identify Problem Loci and Samples for Non-invasive Genetic Samples in a Capture-Mark-Recapture Framework. Mol. Ecol. Notes 5 (3), 716–718. doi:10.1111/j.1471-8286.2005.01038.x
Meyer, S., Robertson, B. C., Chilvers, B. L., and Krkošek, M. (2015). Population Dynamics Reveal Conservation Priorities of the Threatened New Zealand Sea Lion Phocarctos Hookeri. Mar. Biol. 162 (8), 1587–1596. doi:10.1007/s00227-015-2695-8
Mills, L. S., Citta, J. J., Lair, K. P., Schwartz, M. K., and Tallmon, D. A. (2000). Estimating Animal Abundance Using Non-invasive DNA Sampling: Promise and Pitfalls. Ecol. Appl. 10 (1), 283–294. doi:10.2307/264100210.1890/1051-0761(2000)010[0283:eaaund]2.0.co;2
Mondol, S., Karanth, K. U., Kumar, N. S., Gopalaswamy, A. M., Andheria, A., and Ramakrishnan, U. (2009). Evaluation of Non-invasive Genetic Sampling Methods for Estimating Tiger Population Size. Biol. Conserv. 142 (10), 2350–2360. doi:10.1016/j.biocon.2009.05.014
Morgan, D. R., Nugent, G., Gleeson, D., and Howitt, R. (2007). Are Some Possums Untrappable, Unpoisonable, and Therefore Unmonitorable. Landcare Research Contract Report: LC0607/143 for the Animal Health Board.
Murphy, E. C., Russell, J. C., Broome, K. G., Ryan, G. J., and Dowding, J. E. (2019). Conserving New Zealand’s Native Fauna: A Review of Tools Being Developed for the Predator Free 2050 Programme. J. Ornithol. 160, 883–892. doi:10.1007/s10336-019-01643-0
Murphy, M. A., Kendall, K. C., Robinson, A., and Waits, L. P. (2007). The Impact of Time and Field Conditions on Brown Bear (Ursus arctos) Faecal DNA Amplification. Conserv. Genet. 8 (5), 1219–1224. doi:10.1007/s10592-006-9264-0
Nichols, R. V., Königsson, H., Danell, K., and Spong, G. (2012). Browsed Twig Environmental DNA: Diagnostic PCR to Identify Ungulate Species. Mol. Ecol. Resour. 12 (6), 983–989. doi:10.1111/j.1755-0998.2012.03172.x
Nsubuga, A. M., Robbins, M. M., Roeder, A. D., Morin, P. A., Boesch, C., and Vigilant, L. (2004). Factors Affecting the Amount of Genomic DNA Extracted from Ape Faeces and the Identification of an Improved Sample Storage Method. Mol. Ecol. 13 (7), 2089–2094. doi:10.1111/j.1365-294X.2004.02207.x
Ntshudisane, O. K., Emami-Khoyi, A., Gouws, G., Weiss, S.-E., James, N. C., Oliver, J.-C., et al. (2021). Dietary Specialization in a Critically Endangered Pipefish Revealed by Faecal eDNA Metabarcoding. bioRxiv [Preprint] Available at: https://www.biorxiv.org/content/10.1101/2021.01.05.425398v1.
Nugent, G., Whitford, J., Sweetapple, P., Duncan, R., and Holland, P. (2010). Effect of One-Hit Control on the Density of Possums (Trichosurus vulpecula) and Their Impacts on Native Forest. Sci. Conserv. 304, 5–64.
Peakall, R., and Smouse, P. E. (2012). GenALEx 6.5: Genetic Analysis in Excel. Population Genetic Software for Teaching and Research-an Update. Bioinformatics 28, 2537–2539. doi:10.1093/bioinformatics/bts460
Peters, C., Nelson, H., Rusk, B., and Muir, A. (2020). A Novel Method to Optimize the Utility of Underused Moulted Plumulaceous Feather Samples for Genetic Analysis in Bird Conservation. Conserv. Genet. Resour. 12 (3), 457–467. doi:10.1007/s12686-019-01117-8
Pielou, E. (1966). The Measurement of Diversity in Different Types of Biological Collections. J. Theor. Biol. 13, 131–144. doi:10.1016/0022-5193(66)90013-0
Ramos, D. F., Silva, P. E. A., and Dellagostin, O. A. (2015). Diagnosis of Bovine Tuberculosis: Review of Main Techniques. Braz. J. Biol. 75 (5), 830–837. doi:10.1590/1519-6984.23613
Ravant, J. L., Douki, T., and Cadet, J. (2001). Direct and Indirect Effects of UV Radiation on DNA and its Components. J. Photochem. Photobiol. B. 63 (1), 88–102.
Sakata, K. (2011). Forensic Approaches to Monitoring and Individually Identifying New Zealand Vertebrate Pests. PhD dissertation. New Zealand: Lincoln University.
Seutin, G., White, B. N., and Boag, P. T. (1991). Preservation of Avian Blood and Tissue Samples for DNA Analyses. Can. J. Zool. 69 (1), 82–90. doi:10.1139/z91-013
Shannon, C. E., and Weaver, W. (1949). The Mathematical Theory of Communication. Champaign, Illonois: University of Illonois Press.
Sjoberg, T. (2013). Possum (Trichosurus vulpecula) Responses and Preferences to Novel Objects in Their Environment. Master’s Thesis. Canterbury: Lincoln University.
Sloane, M. A., Sunnucks, P., Alpers, D., Beheregaray, L. B., and Taylor, A. C. (2000). Highly Reliable Genetic Identification of Individual Northern Hairy-Nosed Wombats from Single Remotely Collected Hairs: A Feasible Censusing Method. Mol. Ecol. 9 (9), 1233–1240. doi:10.1046/j.1365-294x.2000.00993.x
Solarz, W., Najberek, K., Wilk-Woźniak, E., and Biedrzycka, A. (2020). Raccoons Foster the Spread of Freshwater and Terrestrial Microorganisms-Mammals as a Source of Microbial eDNA. Divers. Distrib. 26, 453–459. doi:10.1111/ddi.13027
Sørensen, T. J. (1948). A Method of Establishing Groups of Equal Amplitude in Plant Sociology Based on Similarity of Species Content and its Application to Analyses of the Vegetation on Danish Commons. København: I kommission hos E. Munksgaard.
Stewart, K. A. (2019). Understanding the Effects of Biotic and Abiotic Factors on Sources of Aquatic Environmental DNA. Biodivers. Conserv. 28 (5), 983–1001. doi:10.1007/s10531-019-01709-8
Strickler, K. M., Fremier, A. K., and Goldberg, C. S. (2015). Quantifying Effects of UV-B, Temperature, and pH on eDNA Degradation in Aquatic Microcosms. Biol. Conserv. 183, 85–92. doi:10.1016/j.biocon.2014.11.038
Taberlet, P., Griffin, S., Goossens, B., Questiau, S., Manceau, V., Escaravage, N., et al. (1996). Reliable Genotyping of Samples with Very Low DNA Quantities Using PCR. Nucl. Acids Res. 24 (16), 3189–3194. doi:10.1093/nar/24.16.3189
Taberlet, P., and Luikart, G. (1999). Non-Invasive Genetic Sampling and Individual Identification. Biol. J. Linn. Soc. 68 (1–2), 41–55. doi:10.1006/bijl.1999.032910.1111/j.1095-8312.1999.tb01157.x
Talan, D. A., Citron, D. M., Abrahamian, F. M., Moran, G. J., and Goldstein, E. J. (1999). Bacteriologic Analysis of Infected Dog and Cat Bites. Emergency Medicine Animal Bite Infection Study Group. N. Engl. J. Med. 340, 85–92. doi:10.1056/NEJM199901143400202
Tan, J., Lee, J. Y., Lee, L. Y. C., Aw, Z. Q., Chew, M. H., Ishak, N. I. B., et al. (2019). Shedder Status: Does it Really Exist?. Forensic Sci. Int. Genet. Suppl. Ser. 7, 360–362. doi:10.1016/j.fsigss.2019.10.012
Taylor, A. C., and Cooper, D. W. (1998). Microsatellite Markers for the Phalangerid Marsupial, the Common Brushtail Possum (Trichosurus vulpecula). Mol. Ecol. 7 (12), 1780–1782. doi:10.1046/j.1365-294x.1998.00517.x
Taylor, R. H., and Taylor, G. A. (1989). Re-Assessment of the Status of Southern Elephant Seals (Mirounga leonina) in New Zealand. N. Z. J. Mar. Freshw. Res. 23 (2), 201–213. doi:10.1080/00288330.1989.9516357
Thomsen, P. F., and Willerslev, E. (2015). Environmental DNA – an Emerging Tool in Conservation for Monitoring Past and Present Biodiversity. Biol. Conserv. 183, 4–18. doi:10.1016/j.biocon.2014.11.019
Tortoli, E., Fedrizzi, T., Meehan, C. J., Trovato, A., Grottola, A., Giacobazzi, E., et al. (2017). The New Phylogeny of the Genus Mycobacterium: The Old and the News. Infect. Genet. Evol. 56, 19–25. doi:10.1016/j.meegid.2017.10.013
Ullah, A., Sadique, U., Ayaz, S., Qureshi, M. S., and Khan, F. A. (2020). Bovine Tuberculosis (bTB)-Isolation and Species-specific Identification of Mycobacterium Bovis from Bovine Raw Milk in Pakistan. Sarhad J. Agric. 36 (2), 489–498. doi:10.17582/journal.sja/2020/36.2.489.498
Valiere, N., and Taberlet, P. (2000). Urine Collected in the Field as a Source of DNA for Species and Individual Identification. Mol. Ecol. 9, 2150–2152. doi:10.1046/j.1365-294x.2000.11142.x
Vargas, M. L., Cruickshank, R. H., Ross, J. G., Holyoak, A. J., Ogilvie, S. C., and Paterson, A. M. (2009). Non-invasive Recovery and Detection of Possum Trichosurus vulpecula DNA from Bitten Bait Interference Devices (WaxTags). Mol. Ecol. Resour. 9 (2), 505–515. doi:10.1111/j.1755-0998.2008.02498.x
Webster, H. J., Emami-Khoyi, A., Van Dyk, J. C., Teske, P. R., and Van Vuuren, B. J. (2020). Environmental DNA Metabarcoding as a Means of Estimating Species Diversity in an Urban Aquatic Ecosystem. Animals 10 (11), 1–18. doi:10.3390/ani10112064
Wilbur, A. K., Engel, G. A., Rompis, A., Putra, I. G. A. A., Lee, B. P. Y. H., Aggimarangsee, N., et al. (2012). From the Mouths of Monkeys: Detection of Mycobacterium tuberculosis Complex DNA from Buccal Swabs of Synanthropic Macaques. Am. J. Primatol. 74 (7), 676–686. doi:10.1002/ajp.22022
Wilmshurst, J. M., Anderson, A. J., Higham, T. F., and Worthy, T. H. (2008). Dating the Late Prehistoric Dispersal of Polynesians to New Zealand Using the Commensal Pacific Rat. PNAS 105 (22), 7676–7680. doi:10.1073/pnas.0801507105
Wilson, G. J., Frantz, A. C., Pope, L. C., Roper, T. J., Burke, T. A., Cheeseman, C. L., et al. (2003). Estimation of Badger Abundance Using Faecal DNA Typing. J. Appl. Ecol. 40 (4), 658–666. doi:10.1046/j.1365-2664.2003.00835.x
Keywords: non-invasive DNA, disease monitoring, Australian brushtail possum, DNA degradation, zoonosis, population density
Citation: Emami-Khoyi A, Agnew TW, Adair MG, Murphy EC, Benmazouz I, Monsanto DM, Parbhu SP, Main DC, Le Roux R, Golla TR, Schnelle C, Alizadeh H, Csányi S, Heltai M, Jansen van Vuuren B, Paterson AM, Teske PR and Ross JG (2021) A New Non-invasive Method for Collecting DNA From Small Mammals in the Field, and Its Application in Simultaneous Vector and Disease Monitoring in Brushtail Possums. Front. Environ. Sci. 9:701033. doi: 10.3389/fenvs.2021.701033
Received: 27 April 2021; Accepted: 20 July 2021;
Published: 30 August 2021.
Edited by:
Attila D. Sándor, University of Agricultural Sciences and Veterinary Medicine of Cluj-Napoca, RomaniaReviewed by:
Aleksandra Biedrzycka, Institute of Nature Conservation (PAN), PolandNaiara Sales, University of Lisbon, Portugal
Copyright © 2021 Emami-Khoyi, Agnew, Adair, Murphy, Benmazouz, Monsanto, Parbhu, Main, Le Roux, Golla, Schnelle, Alizadeh, Csányi, Heltai, Jansen van Vuuren, Paterson, Teske and Ross. This is an open-access article distributed under the terms of the Creative Commons Attribution License (CC BY). The use, distribution or reproduction in other forums is permitted, provided the original author(s) and the copyright owner(s) are credited and that the original publication in this journal is cited, in accordance with accepted academic practice. No use, distribution or reproduction is permitted which does not comply with these terms.
*Correspondence: Arsalan Emami-Khoyi, ZWthcnNhbGFuQGdtYWlsLmNvbQ==
†These authors have contributed equally to this work.