- Pacific Northwest National Laboratory, Earth Systems Science Division, Richland, WA, United States
Commercially available particulate amendments demonstrate high reactivity for effective treatment of water soluble organic and inorganic contaminants in laboratory studies; however, transport of these particles is constrained in the subsurface. In many field applications, particulate amendments are mixed with organic polymers to enhance mobility for direct push applications or stabilize suspensions for high mass loadings. As such, the interactions between particulate amendments, organic polymers and contaminant species need to be systematically investigated to properly understand mechanistic processes that facilitate predictive performance metrics for specific applications in situ. In this study, batch experiments were conducted to quantify the effects of organic polymers (xanthan gum, guar gum, and sodium alginate), polymer concentration (800 and 4,000 mg/L), and aging (up to 28 days) on chromate treatment rate and capacity by two classes of amendments: reductants [granular zero-valent iron (gZVI), micron-ZVI (mZVI), sulfur modified iron (SMI)], and an adsorbent (bismuth sub-nitrate). When particulate amendments were suspended in polymer solutions, reductants retained between 84–100% of the amendment treatment capacity. Conversely, the adsorbent maintained 63–97% relative treatment capacity of the no-polymer control. Polymer solutions had a more pronounced impact on the rate of chromate removal; first order rates of chemical reduction decreased by as much as 70% and adsorption by up to 81% relative to the no-polymer controls. Polymer–amendment aging experiments also showed decreased Cr(VI) treatment capacity; reductants decreased by as much as 24% and adsorption decreased by as much as 44% after 28 days of incubation. While polymer suspensions are needed to aid the injection of particulate amendments into the subsurface, the results from this study indicate potential losses of treatment capacity and a decrease in the rate of remedial performance due to the physical and chemical interactions between polymer suspensions and reactive particulate amendments. Simple batch systems provide baseline characterization of tripartite interactions for the removal of Cr(VI). Additional work is needed to quantify the full impact of polymers on remedial outcomes under site relevant conditions at field scale.
1 Introduction
In situ remediation of sediment and groundwater often necessitates the introduction of reagents or reactive amendments to promote the degradation, mobilization, or stabilization of organic and inorganic contaminants within an acceptable timeframe (Muller et al., 2020). While the underlying mechanism(s) of a remediation technology may show promise at bench scale, flow behavior or other physical constraints can impede mass loading and the transport of remedial amendments through contaminant source zones (Kitanidis and McCarty, 2012). In practice, subsurface injection of remedial reagents can achieve reasonable coverage of high-permeability unsaturated sediment or aquifer strata; however, effective penetration into low-permeability zones remains a major challenge for in situ remediation (e.g., Orozco et al., 2015; Fan et al., 2017). Incomplete treatment of these less accessible regions is important because contamination will persist well after active remediation and will likely contribute to a rebound in contaminant concentrations in site groundwater (e.g., Switzer and Kosson, 2007; Thomson et al., 2008; Brusseau et al., 2011).
Numerous particulate amendments and suspensions are commercially available for the remediation of sediment and groundwater; however, the application of particulates is fundamentally limited by poor subsurface mobility (O’Carroll et al., 2013; Tosco et al., 2014a; Kocur et al., 2014). Grain size, permeability, heterogeneity of the geological formation, and groundwater velocity are the primary physical parameters that dictate particle transport, distribution, and retention in porous media. These parameters define the permissible particle size and injection pressures needed to successfully emplace amendments into the subsurface. Moreover, the electrostatic, chemical, and magnetic properties of the reactive particles and soil grain surfaces play an important role in the practical application of these remedial amendments in subsurface environments (Tufenkji and Elimelech, 2004). To overcome mobility challenges facing particulate amendment delivery in field implementation, particle size can be reduced, surface properties modified, or chemical additives used to enhance suspension stability, and extend particle penetration in heterogenous media (e.g., Fan et al., 2017; Lowry and Phenrat, 2019; Pavelková et al., 2020; Tiraferri et al., 2008; Tosco et al., 2014a; Truex et al., 2011; Velimirovic et al., 2012; Velimirovic et al., 2016). Such modifications introduce tradeoffs in remedial efficiency, treatment or reaction time, and potential cost. To help better understand these tradeoffs in advance, the complex and varied interactions between chemical additives, remedial amendments, contaminant species, and representative site conditions can be systematically investigated to gain a more predictive understanding of the mechanistic interactions that control subsurface reactions and influence remedial outcomes.
Natural and synthetic polymers have been used for borewell drilling in the petroleum industry for decades. More recently, similar techniques have been adopted by the remediation industry to improve applications for subsurface cleanup. For example, guar gum has been utilized extensively to transport hydraulic fracturing proppant, and xanthan gum has been used, though to a lesser extent, for direct push applications (Fink, 2015, Hasan and Abdel-Raouf, 2018; Smith et al., 2008; Tiraferri et al., 2008; Truex et al., 2011; Vecchia et al., 2009; Velimirovic et al., 2012; Velimirovic et al. 2014a; Velimirovic et al. 2014b; Velimirovic et al., 2016; Zhong et al., 2008; Zhong et al., 2013). Polymers assist subsurface injection of particulates in two ways-by increasing suspension stability and improving subsurface sweeping efficiencies (Hasan and Abdel-Raouf, 2018; Tosco et al., 2014b; Wu et al., 2017; Zhong et al., 2011; Zhong et al., 2013). Viscous polymer fluids reduce sedimentation and aggregation of particulate amendments, resulting in suspensions that are stable for hours and suitable for injection (Velimirovic et al., 2012). Furthermore, some bio-polymers exhibit non-Newtonian shear thinning properties which have been shown to extend penetration distances of particulate reactants through porous media in laboratory 1D columns (Zhong et al., 2008, Zhong et al., 2013), 2D wedges (Oostrom et al., 2007; Mondino et al., 2020), and modeling simulations (Tosco and Sethi, 2010; Bianco et al., 2016). These studies demonstrate that shear thinning polymers establish a more uniform injection front, decrease fingering in heterogenous media, and improve micron ZVI particle transport distances by as much as 40% from the point of injection. While the positive attributes of polymer additives are clear, systematic evaluation of the interactions between remedial components under site relevant conditions remains an important gap to ensure the efficacious treatment of contaminant species (e.g., Chuang et al., 2017; Velimirovic et al., 2012; Velimirovic et al., 2016).
The overarching objective of this work was to quantify the influence of polymer suspensions on the reaction kinetics and capacity of commercially available particulate remedial reactants. The selected polymer solutions represent industry accepted formulations and concentrations of guar gum, xanthan gum, and sodium alginate. Selected particulate amendments include two sizes of zero valent iron (ZVI) (granular [gZVI] and micron [mZVI]), sulfur modified iron (SMI), and bismuth sub-nitrate, wherein suspension combinations were evaluated for contaminant removal. Bismuth sub-nitrate was selected as a promising adsorbent material which consists of layered structures that effectively treat a variety of inorganic contaminant species and mixtures (Pearce et al., 2020). These studies incorporated chromate because it is a prevalent groundwater contaminant worldwide due to metals plating, textiles, wood preservation, and corrosion prevention (Testa et al., 2004; Gharbi et al., 2018). Hexavalent chromium, Cr(VI), is highly soluble in water and can be effectively removed from the aqueous phase by chemical reduction of Cr(VI) to the highly insoluble Cr(III), or adsorption. In this study, the interference of polymer additives was quantified against a selection of remedial amendments that vary in particle size, properties, and specific mode of action (i.e., reduction vs sorption); providing new insights into the tradeoffs between beneficial remedial delivery strategies and treatment efficiencies and outcomes.
2 Materials and Methods
2.1 Remedial Amendments
Commercial grade particulate amendments, granular 325 mesh Zero-Valent Iron, gZVI, (Hepure), 20–80 mesh Sulfur-modified Iron, SMI (Hydrex™ 9670), and 1–5 µm micron-sized ZVI (mZVI) (Ashland) were used for the experiments described herein. Bismuth sub-nitrate, 100 mesh, was selected as the adsorbent material (Sigma-Aldrich). Experimental testing and reactivity measurements were conducted using hexavalent chromium as a model groundwater contaminant, capable of both being adsorbed and reduced. Various amounts of a 320 mg/L aqueous chromium stock solution (K2CrO4, Sigma Aldrich) were used to achieve initial Cr to amendment ratios of 4.5–18.5 mg K2CrO4/g solid amendment.
2.2 Chromium Assay
Aqueous samples were immediately processed for quantification of soluble Cr(VI). Samples were passed through a 0.2 µm syringe filter to remove particulate amendments prior to analysis and diluted in artificial groundwater. Aqueous concentration of chromium was measured spectrophotometrically following the optimized assay method described by Lace et al. (2019). Briefly, 0.5 ml of an aqueous sample was mixed with 0.25 ml of 0.2 M sulfuric acid, and 0.25 ml 0.5%(w/v) 1,5-diphenylcarbazide, and the absorbance was measured (λ = 540 nm). Potassium chromate standards were freshly prepared and analyzed with every set of experiments. Quantification standards were prepared by the dilution-method in artificial groundwater and had a linear range from 0.2–6.5 mg/L (slope = 11.4 ± 1.2, R2 > 0.994).
2.3 Bio-Polymer Solution Properties
Xanthan gum, guar gum, and alginate are natural polymers that possess useful properties which are exploited for a variety of applications ranging from food additives, tissue bio-engineering, and geotechnical engineering. Relevant features to this study are summarized in Table 1. Typical polymer formations used to suspend particulate amendments range in concentration from 0.5−4 g/L (e.g., Tosco and Sethi, 2010; Truex et al., 2011; Tosco et al., 2014a; Velimirovic et al., 2016).
Particulate amendments were suspended in polymer solutions that were consistent with industry standard applications in both composition and concentration. Polymers included: 1) xanthan gum (MP Biomedicals); 2) guar gum (Sigma-Aldrich); and 3) sodium alginate (MP Biomedicals).
Two concentrations of xanthan gum, guar gum and sodium alginate solutions (800 mg/L and 4,000 mg/L) were tested in an artificial groundwater (AGW). The AGW has been modeled after typical groundwater compositions encountered at the Department of Energy’s Hanford Site in Richland, Washington, United States (Truex et al., 2017). AGW composition is as follows: silicic acid (H2SiO3∙nH2O, 15.3 mg/L), potassium chloride (KCl, 8.2 mg/L), magnesium carbonate (MgCO3, 13 mg/L), sodium chloride (NaCl, 15 mg/L), calcium sulfate (CaSO4, 67 mg/L), and calcium carbonate (CaCO3, 150 mg/L). The AGW medium was supersaturated with CaCO3 (solubility is 14 mg/L) and stirred for one week to reach equilibrium (pH 7.8). The density of polymer solutions prepared in AGW was measured via a pycnometer. At 800 mg/L, polymer solution densities were near water at 0.9965 g/mL, 0.9967 g/mL, and 0.9968 g/mL, for xanthan gum, guar gum, and sodium alginate, respectively.
2.4 Batch Experiments With Particulate Amendment–Polymer Suspensions
Batch experiments were systematically conducted with pairwise combinations of remedial amendments (gZVI, mZVI, Bi, and SMI) suspended in polymer solutions (xanthan gum, guar gum, and sodium alginate) to measure the reaction kinetics and capacity for removal of K2CrO4 (aq) from solution. Batch experiments were composed in 20 ml borosilicate glass scintillation vials. Briefly, 0.1 g of particulate amendment was added directly to the prepared polymer solutions and well mixed to disperse amendments. Vials were spiked with a hexavalent chromium stock solution to produce a final Cr(VI) concentration of 23, 46, or 96 mg/L in a total aqueous volume of 20 ml. Vials were placed on a platform shaker (125 rpms, room temperature, in the dark) in between measurements to ensure reactants remained well mixed. Aqueous samples were collected at 2, 6, 24, and 48 h and processed immediately to measure the aqueous concentration of Cr(VI). Negative controls consisted of AGW and polymer solutions without particulate amendment to ensure no loss of K2CrO4 occurred. The no amendment control experiments (also sampled at 2, 6, 24 and 48 h) had average Cr recoveries of 104 ± 12, 100 ± 7, 102 ± 7, and 94 ± 6%, for no polymer, xanthan gum, guar gum, and sodium alginate solutions, respectively. Amendments without biopolymer solutions (no polymer controls) were also included to provide measurement of maximum reactivity and quantify interference from the polymer solutions. The relative error for all experimental replicates ranged from 0–11%, with an average 5 ± 6%.
2.5 Remedial Amendment Aging Experiments
A pairwise matrix of particulate amendments and polymer solutions was evaluated to quantify the temporal interactions between specific polymer fluid–amendment combinations by measuring the preservation and longevity of treatment (reduction or adsorption) capacity. Particulate amendments were added directly to prepared polymer solutions in borosilicate glass scintillation vials. Batch experiments were placed on a platform shaker (125 rpms, room temperature, in the dark) for an aging duration of 1, 2, and 4 weeks. After the prescribed aging period, the K2CrO4 stock solution was added (4.5–18.5 mg K2CrO4/g solid amendment) and the reaction kinetics and capacity were quantified by collecting aqueous samples after 24 and 48 h. Samples were processed immediately as described to measure the aqueous concentration of Cr(VI). Since the relative error among replicates for the treatment rate and capacity experiments described above was consistently low and never exceeded 11% (mean = 5 ± 6%), temporal aging measurements were collected as singlets.
2.6 Modeling Reactive Performance of Remedial Amendment Suspensions
Aqueous Cr(VI) concentrations for each temporal data series (t = 0, 2, 6, 24, and 48 h) were fit together to Eq. 1 to quantify both the treatment capacity and kinetic rates. This model is derived from a first order rate expression that is modulated by a capacity term.
where: C(t) = aqueous concentration at time t [mg/L]; Ceq is aqueous equilibrium concentration [mg/L]; C0 is initial concentration [mg/L]; k is first order rate coefficient [hr−1]; t is time [hr].
Ceq and k were fit to Eq. 1 using experimental results for C(t) and the known experimental starting condition for C0. Reported errors are the standard error of parameter fit. Amendment capacity (C in mg Cr/g-amendment) was calculated from Ceq and the uncertainty was determined through error propagation. Both treatment capacity and first order rate coefficients were compared to the appropriate controls to quantify the influence of the polymer solution.
3 Results and Discussion
Polymer composition, concentration, and background fluid characteristics (e.g., pH, net charge, and ionic strength) can have an important influence on remedial amendment properties in suspension. For example, that stability of milled ZVI (average size = 12 µm) suspensions were found to be inversely correlated with guar gum concentration (0, 0.5, 1 g/L), which was reflected by zeta potential which increased from −22 mV, to −15 mV, to −4 mV, respectively (Velimirovic et al., 2016). On the other hand, Velimirovic et al. (2016) found milled ZVI suspension stability was slightly improved with increased agar concentration (zeta potential decreased from −22 mV, −33 mV, and −30 mV respectively for 0, 0.5, and 1 g/L solutions), indicating the stability difference invoked by different types of polymers. Generally, zeta potential values < −30 mV or > +30 mV are considered stable while values falling between −30 mV and +30 mV are considered unstable. Based on reported literature values for polymer zeta potentials (Zhang et al., 2014; Li et al., 2020) and the measured pH values of our remedial solutions, xanthan gum and alginate suspensions are expected to be stable at pH > 4; while guar gum is unlikely to produce a stable suspension at any pH.
3.1 Amendment Reactivity
3.1.1 Reductants in Polymer Solutions
The overall treatment capacity (C) and first order rate of K2CrO4 reduction (k) were determined by fitting the experimental data to Eq. 1 (Figure 1; Table 2).
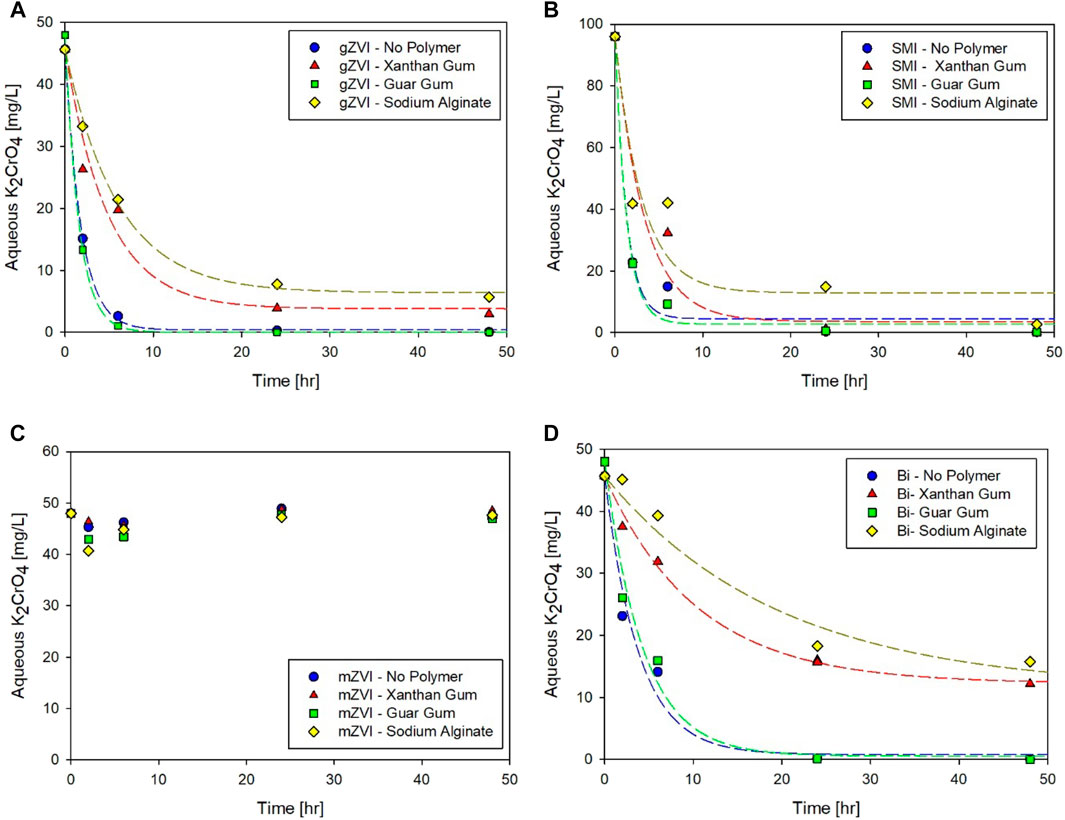
FIGURE 1. Impact of polymer (800 mg/L) on K2CrO4 treatment via (A) gZVI, (B) SMI, (C) mZVI, and (D) Bismuth sub-nitrate. Symbols indicate experimental data points for each polymer type (circle = no polymer; triangle = xanthan gum, square = guar gum, diamond = sodium alginate). Dashed lines indicate corresponding kinetic model fits (Eq. 1). The relative error associated with experimental replicates ranged from 0–11% (average 5%).
Slight decreases in the treatment capacity of gZVI were measured in suspensions of 800 mg/L (final concentration) xanthan gum, guar gum, and sodium alginate; corresponding to 7.0 ± 0.1, 0.8 ± 0.1, and 12 ± 0.0%, respectively, less capacity than the gZVI no-polymer control (Figure 1A; Table 2). However, gZVI reduction rate coefficients were strongly influenced by the presence of the polymer, with Cr(VI) reduction rates decreasing 62 ± 9.3%, 41 ± 12, and 70 ± 4.0% in xanthan gum, guar gum, and sodium alginate solutions, respectively, relative to the gZVI no-polymer control.
The treatment capacity of SMI decreased 2.5 ± 0.2, 2.6 ± 0.2, and 16 ± 0.3%, in the presence of 800 mg/L xanthan gum, guar gum, and sodium alginate, respectively (Figure 1B; Table 2). Similar to the behavior of gZVI, polymer suspensions had a much more pronounced effect on the treatment rate coefficient for SMI where rates decreased 61 ± 29, 2 ± 28, and 58 ± 35% in the presence of xanthan gum, guar gum, and sodium alginate, respectively.
The effect of polymers on particle reactivity has not been fully characterized in the literature, though studies have described a temporal delay or loss of reactivity (Velimirovic et al., 2014a; Gastone et al., 2014). The significance of these implications remains to be determined, though the consensus is that the practical benefits, which range from delayed surface corrosion to enhanced transport behavior, potentially outweigh any negative interactions which may be inherently overcome by groundwater flow and biodegradation in situ (Velimirovic et al., 2012; Velimirovic et al., 2014a).
Micron sized ZVI was unresponsive for the reduction of Cr(VI) during these short duration experiments (Figure 1C; Table 2). Regardless of the polymer used, the aqueous concentration of Cr(VI) remained unchanged for up to 48 h of incubation in these batch experiments. This ZVI product is commercial grade, not lab grade purity, however the vendor specifications do not offer any clear explanation for the relatively slow reactivity.
3.1.2 Adsorbent in Polymer Solutions
In contrast to particulate reductants, the treatment capacity and rate of bismuth subnitrate to adsorb K2CrO4 from solution was generally more impaired by the polymer suspension (Figure 1D; Table 2). The adsorption capacity of bismuth decreased 37 ± 0.2, 2.9 ± 0.1, and 23 ± 0.1%, and adsorption rates decreased 30 ± 35, 81 ± 27, and 81 ± 27% in the presence of xanthan gum, guar gum, and sodium alginate, respectively. These results are consistent with studies showing that polymer suspensions can have substantial interference on the kinetics and capacity of remedial amendments. For example, the rate of trichloroethylene (TCE) reduction by milled ZVI decreased an order of magnitude, relative to controls, when suspended in agar (1 g/L) (Velimirovic et al., 2016). Similarly, reduction rates of TCE and other chlorinated aliphatics were 1–8 times slower when micron sized ZVI was suspended in guar gum (2, 4, or 6 g/L) (Velimirovic et al., 2012). In these studies, the loss of amendment reactivity was described as a reversible interaction between the polymer matrix and reaction sites on the ZVI particle surface, in which full reactivity could be restored by repeated rinsing or enzymatic treatment to eliminate the polymer from those sites (Velimirovic et al., 2012; Velimirovic et al., 2013). Polymers have also been used to form a hydrodynamic barrier on ZVI and SMI particle surfaces to slow oxidative reactions (Messali et al., 2017; Balachandramohan and Sivasankar, 2018; Palumbo et al., 2019). This explanation that polymers create a physical barrier to particle reaction sites is consistent with our results showing reduced performance metrics that indicate delayed reactivity with Cr(VI) over these short duration experiments. Longer temporal studies are needed, however, to determine whether treatment capacity (reduction or adsorption) is genuinely lost due to chemical reaction with the polymer or simply delayed due to the physical blocking of reaction sites.
The strength of electrostatic interactions between the polymer matrix, an amendment, and/or a contaminant ion, could compound the physical interferences between reactants. It is worth noting that polymers may also alter the surface charge of a particle (Barany, 2015). In this case, if the net surface charge is negative then repulsion of negatively charged contaminant species could thereby reduce treatment capacity and retard reaction kinetics. This interpretation could partially explain why the highly negative charge of the alginate had the most Cr(VI) interference, as Cr predominately exists as CrO42−, followed by the slightly negatively charged xanthan gum. By extension, the lack of interference experienced with guar gum could be attributed to it having a net neutral charge (at pH ∼ 8). Zeta potential measurements were not included as part of this investigation but may be important for understanding specific mechanistic interactions between these remedial components.
3.2 Concentration Effects of Polymers on Amendment Reactivity
Many publications have evaluated the rheological properties of polymer solutions at relatively high concentrations; however, concentration dependent effects on amendment reactivity could be quite important depending on the remedial application. For example, highly viscous suspensions (4 to as high as 8 g/L) would be appropriate to emplace amendment mass in fractures and fissures, while shear thinning behavior at much lower polymer concentration is necessary for direct push injection of suspensions into the heterogenous subsurface. The effect of high polymer concentration (4,000 mg/L) on amendment treatment capacity (C) and first order rate of K2CrO4 reduction (k) were determined by fitting the experimental data to Eq 1 (Figure 2; Table 2). Batch experiments were conducted as described above allowing for direct comparison between the two polymer concentrations (800 and 4,000 mg/L). Since alginate provided little rheological benefit (i.e., little increase in overall viscosity and no shear thinning behavior), limited improvement in suspension stability for the amendments considered here (data not shown), and high interference was exhibited with the various amendments, no further testing was performed.
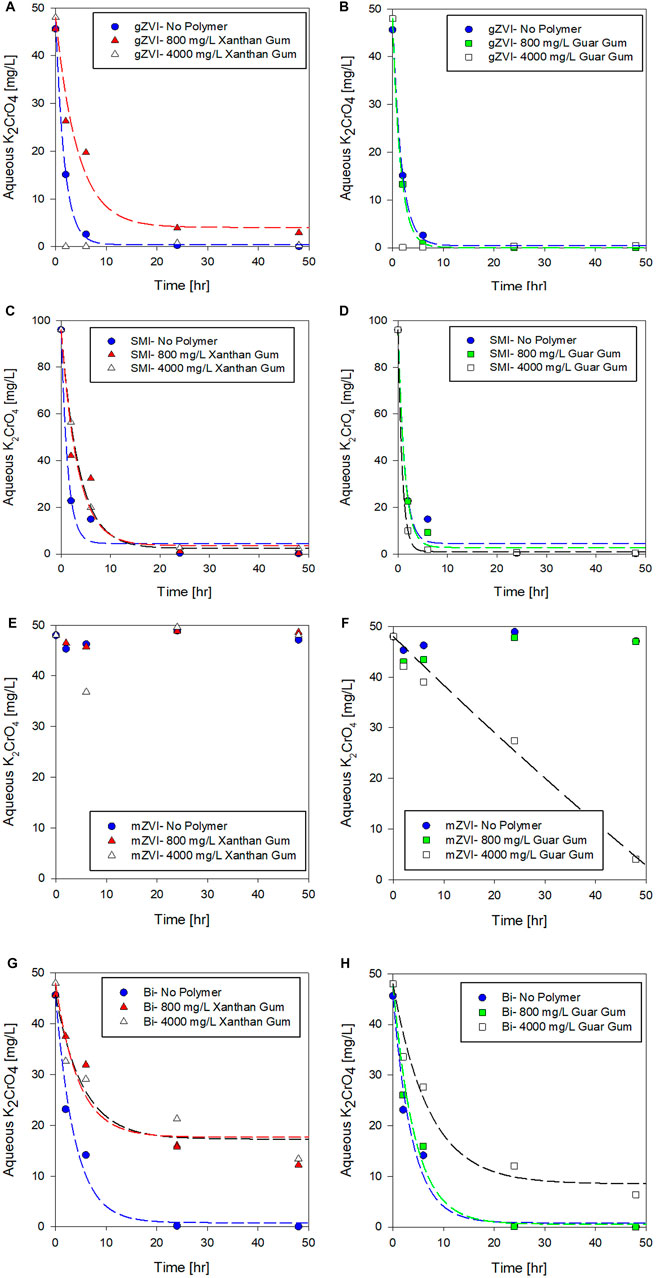
FIGURE 2. Effect of Xanthan Gum and Guar Gum Concentration Effects on Reactivity of (A,B) gZVI, (C,D) SMI, (E,F) mZVI and (G,H) Bismuth, respectively. Symbols designate experimental data; lines indicate corresponding kinetic model fits (Eq. 1). The relative error associated with experimental replicates ranged from 0–11% (average 5%).
3.2.1 Effect of Polymer Concentration on Reductants
Increasing the polymer concentration to 4,000 mg/L corresponded with a significant increase in gZVI treatment kinetics, which resulted in the reaction being too fast to successfully obtain fitted model values for capacity and kinetics (Table 2; Figure 2A,B). While parameters could not be fit for direct comparisons, due to the quick depletion of aqueous K2CrO4, results suggest that the higher polymer concentrations, for both xanthan and guar gum, created no capacity reduction and perhaps increased the reduction reaction rates compared to the no polymer control and experiments conducted at 800 mg/L polymer. Visual observations and suspension stability experiments for gZVI suggest that the high polymer concentrations created a more stable suspension (see supporting information). In this case, we surmise that the high polymer content reduced particle aggregation, thereby increasing effective surface area for rapid reduction of Cr(VI).
SMI treatment capacity was generally insensitive to increased polymer concentration; however, SMI reduction kinetics did show polymer specific trends (Table 2; Figures 2C,D). Xanthan gum, regardless of concentration (i.e., 800 or 4,000 mg/L), decreased treatment rates to 36–39% of the no polymer control kinetic rate. Guar gum showed the opposite trend, Cr(VI) removal kinetics increased by about 53% in a 4,000 mg/L guar gum suspension compared to the no polymer control and 56% compared to the lower concentration guar gum solution.
3.2.2 Effect of Polymer Concentration on Adsorbent
For bismuth subnitrate, increasing xanthan gum concentration from 800 mg/L to 4,000 mg/L did not create additional interference in terms of treatment capacity or treatment rate. The results summarized in Figure 2G suggest that maximum interference by xanthan gum on bismuth subnitrate was achieved at a concentration of 800 mg/L and thus, no further polymer concentration effects on Cr(VI) reduction were measured. This trend may suggest electrostatic interactions between the slightly positively charged bismuth and the strongly negatively charged xanthan gum are playing an important role. On the other hand, bismuth subnitrate adsorption capacity was reduced from 97 to 83% with increasing concentration of guar gum (Figure 2H), which may indicate that physical interactions predominate for net neutral guar gum, thus eliminating electrostatic forces from consideration. Clearly, the maximum interference displayed by guar gum was not exceeded at 800 mg/L, as was observed for xanthan gum, and concentration dependent effects were measured at 4,000 mg/L.
As with gZVI, a higher polymer concentration produced faster reaction kinetics for Cr(VI) removal. Bismuth treatment kinetics increased from 0.05 to 0.14 h−1 with increased guar gum concentration. Similarly, the fitted rate coefficient was higher for the 4,000 mg/L xanthan gum solution compared to the 800 mg/L solution, although the increase was within the estimated parameter error. As postulated for gZVI, polymer enhanced stability can improve reaction kinetics by minimizing particle aggregation and agglomeration; however, the obvious tradeoff for faster reaction kinetics is, presumably, time dependent impairment of treatment capacity. These types of experiments not only illustrate clear tradeoffs, but may also be used to quickly determine an optimal carrier concentration for an amendment-carrier pair, providing a balance between the ability of the carrier to increase suspension stability without creating significant interferences that reduce or delay treatment capacity.
We presume the primary interference for adsorbent materials, like Bi, is due to physical blocking of the surface reaction sites by a polymer. This is supported by published studies showing that particulate amendment reactivity can be restored if the polymer is removed (e.g., Velimirovic et al., 2012, Velimirovic et al., 2013), although it is unclear whether polymer interaction with reductants is strictly physical or possibly chemical as well. The implication of potentially reversible interference behavior is that particulate amendment reactivity could be fully restored through dilution, desorption, and/or degradation in situ. The former processes will be largely controlled by groundwater flow conditions and groundwater chemistry, and the latter by microbial densities and relative activity. Guar gum has been shown to be susceptible to degradation, while xanthan gum appears more refractory, though detailed degradation studies with quantitative data are lacking (e.g., Cadmus et al., 1982). Consequently, interferences and complex interactions between remedial reagents need to be systematically characterized to reliably understand in situ performance and temporal behavior, as well as to identify actionable interventions that ensure remedial goals are met.
3.3 Aging Effects on Amendment Reactivity
In application, emplaced amendments may naturally age in situ if exposure to contaminants is primarily controlled by slow groundwater transport. As such, this series of aging experiments provide insights into whether amendment reactivity is preserved over time in polymer suspensions, or if some amount of an amendment’s remedial capacity is lost as it ages in a polymer suspension before contacting contaminant. To investigate the temporal effects of polymers on amendment reactivity, amendments were aged in polymer solutions for 7, 14, and 28 days prior to measuring Cr(VI) removal over a 24-h reaction period. Results from the aging experiments are summarized in Figure 3 where the performance metrics and results described above (Table 2) were used as a baseline (t = 0 days) for comparison.
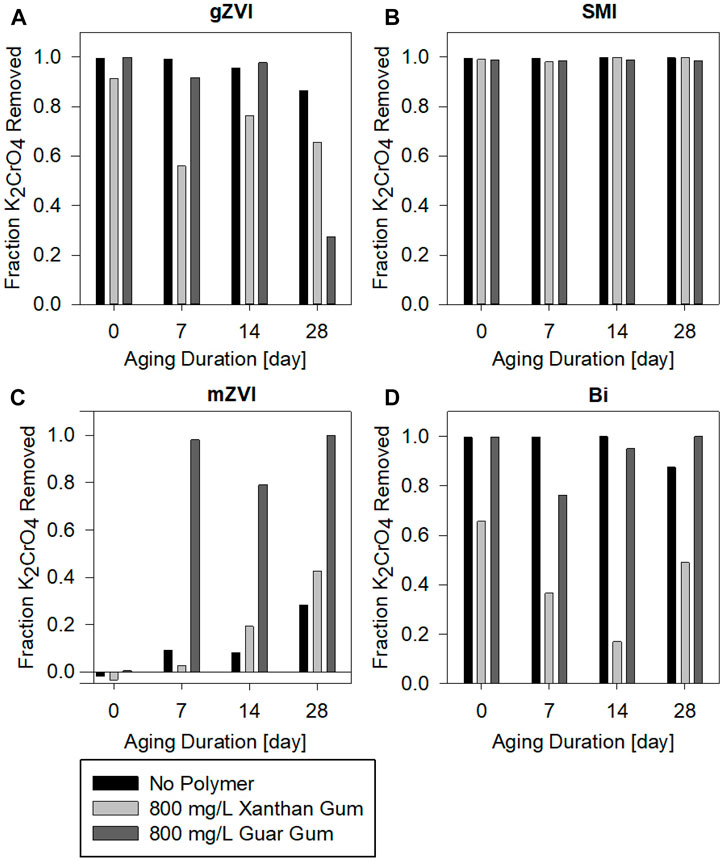
FIGURE 3. Effect of polymer (800 mg/L)–amendment aging on K2CrO4 removal via (A) gZVI, (B) SMI, (C) mZVI, and (D) Bismuth sub-nitrate. Post aging, chromate was added to the polymer-amendment system and Cr(VI) concentration was measured after 24 h.
3.3.1 Aging of Reductants in Polymer Solutions
After 28 days of aging particulate amendments, approximately 86% of the gZVI treatment capacity was preserved in the no-polymer controls, whereas in xanthan gum, reduction capacity decreased from 91 to 66% by 28 days. Similarly, guar gum suspensions of gZVI maintained reduction capacity for up to 14 days of aging, reducing 98% of the aqueous Cr(VI); however, between 14 and 28 days of incubation, gZVI reduction capacity declined to only 27%.
The ability of SMI to effectively reduce Cr(VI) was not affected following 28 days of aging in any of the polymer suspensions. In all SMI aging tests at least 98% of the treatment capacity was retained, relative to no polymer baseline control, indicating the robust nature of SMI in the tested polymers. SMI is a potent chemical reductant, utilizing the dual action of zero-valent iron and reduced sulfur compounds, capable of rapidly neutralizing a variety of groundwater contaminants (Allred 2012). In particular, sulfidation of nZVI has been shown to minimize nonspecific, competitive reactions that deplete reducing potential, chiefly corrosive reactions that predominate during aging in water (Su et al., 2015), thereby improving overall treatment selectivity and reactivity for target compounds (Rajajayavel and Ghoshal, 2015; Fan et al., 2016).
Interestingly, mZVI was not only activated in response to aging, but the temporal activation period appeared shorted when suspended in a polymer compared to the no polymer control. In the mZVI–no polymer control, Cr(VI) concentration slowly declined after 7–14 days (8–9% Cr(VI) reduced) with a 28% decrease in Cr(VI) concentration after 28-days of aging. Lawter et al. (2018) demonstrated effective reduction of 99Tc and 129I with the same mZVI product over comparable time scales (days-weeks). For comparison, the mZVI–guar gum suspension removed over 98% Cr(VI) after 7 days of aging, and 100% after the full 28 days of aging. Similar trends, though lower in magnitude, were measured when mZVI was aged in xanthan gum; 43% Cr(VI) was removed after 28 days of aging in xanthan gum, compared to 28% in the no polymer control (Figure 3). We surmise that the measured delay in mZVI response could, in part, reflect competing reactions for available mZVI reaction sites (and reductant) between water, O2, and Cr(VI). Additional work is needed to resolve the temporal delay in mZVI reduction activity, but this behavior contradicts conventional expectations relative to gZVI based on the established relationship between particle size and reactivity. It is most plausible that suspension stability plays a key role in mZVI reactive performance which clearly increased with high concentration of guar gum (Figure 2) and in response to aging which would increase particle disaggregation, creating more surface area for Cr(VI) reduction (Figure 3).
3.3.2 Aging of Adsorbent in Polymer Solutions
Consistent with the results summarized previously, adsorption capacity of bismuth subnitrate was more severely affected by aging compared to the chemical reductants investigated. When bismuth was suspended in xanthan gum, Cr(VI) removal capacity decreased from 65 to 37%–17% as suspensions were aged 0, 7, and 14 days, respectively. However, after 28 days, bismuth-xanthan gum capacity increased up to 49%, indicating a time-dependent behavior that seemingly restored some treatment capacity after 28 days of aging. Interestingly, bismuth suspended in guar gum did not exhibit this behavior. In fact, chromate removal capacity of bismuth suspended in guar gum remained 95 and 100%, after 14- and 28-days aging, respectively.
Overall, the batch experiment results illustrate how polymer specific attributes may differentially affect treatment capacity and reaction rate for reductants and adsorbents. Concentration dependent behavior and likely a multitude of complex physical and chemical interactions between polymers and remedial amendments are highlighted for simple batch systems. We anticipate the complexity of the system will likely increase further as site specific geological media and biotic processes are also considered. Thus, remedial suspensions need to be systematically characterized in order to more completely understand these dynamic systems to enable a more predictive and translational knowledge of in situ treatment performance and longevity.
4 Conclusion
The results indicate that polymer composition, concentration, and temporal aging may all have important impacts on the treatment capacity and reaction kinetics of particulate amendments. Specific interactions between polymer and amendment varied, suggesting that both physical and chemical interactions impede reactivity. The main findings from this study are that polymer interferences: 1) were more pronounced for adsorption of Cr(VI) compared to chemical reduction, 2) were generally lower and less severe with guar gum compared to other polymers tested, 3) resulted in capacity lost for gZVI, but not SMI, during aging while Bi treatment capacity was temporally delayed but restored with time.
While this study does contribute incremental knowledge of specific interactions between combinations of remedial reagents, we expect that these interactions will become much more complex and dynamic under site relevant conditions (e.g., high sediment to water ratio with little mixing, heterogeneity). As such, the performance metrics reported here likely represent best case, baseline determinations for Cr(VI) removal whereby increasing system complexity will exacerbate interferences and disrupt desired remedial reactions. Additional studies are needed to systematically characterize the processes that most significantly affect remedial outcomes to help enable application design improvements and our ability to forecast in situ treatment outcomes with increased precision.
For any given amendment-polymer combination, there are clear trade-offs between beneficial rheological attributes and temporal impairment of remedial activity that must be considered in light of site-specific conditions and the remedial objectives. While fluid property requirements will differ by remedial application, the lowest polymer concentration that meets fluid characteristics for successful injection should be selected for use to limit carrier interference. While interferences are highly dependent on the mode of action of the remedial amendment, potential interferences should be considered and built into remedial design strategies. Upfront experimental screening, as conducted here, could help to identify preparation conditions for amendment suspensions that tune remedial amendment performance for specific site conditions and subsurface applications.
Data Availability Statement
The raw data supporting the conclusion of this article will be made available by the authors, without undue reservation.
Author Contributions
KM and CB contributed to conception and design of the study. KM, LZ, and CB conducted the experiments and analyzed the results. KM and CB wrote the manuscript. KM, LZ, and CB contributed to manuscript revision and approved the submitted version.
Funding
This work was funded by the United States Department of Energy (DOE) Richland Operations Office to the Deep Vadose Zone–Applied Field Research Initiative at Pacific Northwest National Laboratory (PNNL). PNNL is managed by Battelle Memorial Institute under Contract No. DE-AC05-76RL01830 with the United States Department of Energy.
Conflict of Interest
The authors declare that the research was conducted in the absence of any commercial or financial relationships that could be construed as a potential conflict of interest.
Publisher’s Note
All claims expressed in this article are solely those of the authors and do not necessarily represent those of their affiliated organizations, or those of the publisher, the editors and the reviewers. Any product that may be evaluated in this article, orclaim that may be made by its manufacturer, is not guaranteed or endorsed by the publisher.
Acknowledgments
The coauthors would like to acknowledge Jim Szecsody and Carolyn Pearce for helpful discussion and meaningful contribution to the overall quality of this presentation.
Supplementary Material
The Supplementary Material for this article can be found online at: https://www.frontiersin.org/articles/10.3389/fenvs.2021.703851/full#supplementary-material
References
Allred, B. J. (2012). Laboratory Evaluation of Zero Valent Iron and Sulfur-Modified Iron for Agricultural Drainage Water Treatment. Groundwater Monit. Remediation 32, 81–95. doi:10.1111/j.1745-6592.2011.01379.x
Balachandramohan, J., and Sivasankar, T. (2018). Ultrasound Assisted Synthesis of Guar Gum-Zero Valent Iron Nanocomposites as a Novel Catalyst for the Treatment of Pollutants. Carbohydr. Polym. 199, 41–50. doi:10.1016/j.carbpol.2018.06.097
Barany, S. (2015). Polymer Adsorption and Electrokinetic Potential of Dispersed Particles in Weak and strong Electric fields. Adv. Colloid Interf. Sci. 222, 58–69. doi:10.1016/j.cis.2014.09.009
Bianco, C., Tosco, T., and Sethi, R. (2016). A 3-dimensional Micro- and Nanoparticle Transport and Filtration Model (MNM3D) Applied to the Migration of Carbon-Based Nanomaterials in Porous media. J. Contaminant Hydrol. 193, 10–20. doi:10.1016/j.jconhyd.2016.08.006
Brusseau, M. L., Carroll, K. C., Allen, T., Baker, J., Diguiseppi, W., Hatton, J., et al. (2011). Impact of In Situ Chemical Oxidation on Contaminant Mass Discharge: Linking Source-Zone and Plume-Scale Characterizations of Remediation Performance. Environ. Sci. Technol. 45 (12), 5352–5358. doi:10.1021/es200716s
Cadmus, M. C., Jackson, L. K., Burton, K. A., Plattner, R. D., and Slodki, M. E. (1982). Biodegradation of Xanthan Gum by Bacillus Sp. Appl. Environ. Microbiol. 44 (1), 5–11. doi:10.1128/aem.44.1.5-11.1982
Chuang, J. J., Huang, Y. Y., Lo, S. H., Hsu, T. F., Huang, W. Y., Huang, S. L., et al. (2017). Effects of pH on the Shape of Alginate Particles and its Release Behavior. Int. J. Polym. Sci. 2017, 3902704. doi:10.1155/2017/3902704
Fan, D., Gilbert, E. J., and Fox, T. (2017). Current State of In Situ Subsurface Remediation by Activated Carbon-Based Amendments. J. Environ. Manage. 204, 793–803. doi:10.1016/j.jenvman.2017.02.014
Fan, D., Johnson, G. O., Tratnyek, P. G., and Johnson, R. L. (2016). Sulfidation of Nano Zerovalent Iron (nZVI) for Improved Selectivity during In-Situ Chemical Reduction (ISCR). Environ. Sci. Technol. 50 (17), 9558–9565. doi:10.1021/acs.est.6b02170
Fink, J. K. (2015). Petroleum Engineer’s Guide to Oil Field Chemicals and Fluids. Second Edition. Houston, Texas: Gulf Professional Publishing, 567–651. Chapter 17, Fracturing Fluids.
Flores Orozco, A., Velimirovic, M., Tosco, T., Kemna, A., Sapion, H., Klaas, N., et al. (2015). Monitoring the Injection of Microscale Zerovalent Iron Particles for Groundwater Remediation by Means of Complex Electrical Conductivity Imaging. Environ. Sci. Technol. 49, 5593–5600. doi:10.1021/acs.est.5b00208
Gastone, F., Tosco, T., and Sethi, R. (2014). Green Stabilization of Microscale Iron Particles Using Guar Gum: Bulk Rheology, Sedimentation Rate and Enzymatic Degradation. J. Colloid Interf. Sci. 421, 33–43. doi:10.1016/j.jcis.2014.01.021
Gharbi, O., Thomas, S., Smith, C., and Birbilis, N. (2018). Chromate Replacement: What Does the Future Hold? Npj Mater. Degrad. 2, 12. doi:10.1038/s41529-018-0034-5
Hasan, A. M. A., and Abdel-Raouf, M. E. (2018). Applications of Guar Gum and its Derivatives in Petroleum Industry: A Review. Egypt. J. Pet. 27, 1043–1050. doi:10.1016/j.ejpe.2018.03.005
Kocur, C. M., Chowdhury, A. I., Sakulchaicharoen, N., Boparai, H. K., Weber, K. P., Sharma, P., et al. (2014). Characterization of nZVI Mobility in a Field Scale Test. Environ. Sci. Technol. 48, 2862–2869. doi:10.1021/es4044209
Lace, A., Ryan, D., Bowkett, M., and Cleary, J. (2019). Chromium Monitoring in Water by Colorimetry Using Optimised 1,5-Diphenylcarbazide Method. Ijerph 16, 1803. doi:10.3390/ijerph.16101803
Lawter, A. R., Garcia, W. L., Kukkadapu, R. K., Qafoku, O., Bowden, M. E., Saslow, S. A., et al. (2018). Technetium and Iodine Aqueous Species Immobilization and Transformations in the Presence of Strong Reductants and Calcite-Forming Solutions: Remedial Action Implications. Sci. Total Environ. 636, 588–595.
Li, J., Jin, W., Xu, W., Liu, G., Huang, Q., Zhu, Z., et al. (2020). Effect of Charge Density of Polysaccharide on Self-Assembly Behaviors of Ovalbumin and Sodium Alginate. Int. J. Biol. Macromolecules 154, 1245–1254. doi:10.1016/j.ijbiomac.2019.10.279
Lowry, G. V., and Phenrat, T. (2019). “State of Knowledge and Future Needs for NZVI Applications in Subsurface Remediation,” in Nanoscale Zerovalent Iron Particles for Environmental Restoration. Editors T. Phenrat, and G. Lowry (New York, United States: Springer, Cham), 563–579. doi:10.1007/978-3-319-95340-3_16
Messali, M., Lgaz, H., Dassanayake, R., Salghi, R., Jodeh, S., Abidi, N., et al. (2017). Guar Gum as Efficient Non-toxic Inhibitor of Carbon Steel Corrosion in Phosphoric Acid Medium: Electrochemical, Surface, DFT and MD Simulations Studies. J. Mol. Struct. 1145, 43–54. doi:10.1016/j.molstruc.2017.05.081
Mondino, F., Piscitello, A., Bianco, C., Gallo, A., de Folly D’Auris, A., Tosco, T., et al. (2020). Injection of Zerovalent Iron Gels for Aquifer Nanoremediation: Lab Experiments and Modeling. Water 12 (3), 826. doi:10.3390/w12030826
Muller, K. A., Johnson, C. D., Bagwell, C. E., and Truex, M. J. (2020)). Methods for Delivery and Distribution of Amendments for Subsurface Remediation: A Critical Review. Groundwater Monit. R. 41, 46–75. doi:10.1111/gwmr.12418
O’Carroll, D., Sleep, B., Krol, M., Boparai, H., and Kocur, C. (2013). Nanoscale Zero Valent Iron and Bimetallic Particles for Contaminated Site Remediation. Adv. Water Resour. 51, 104–122. doi:10.1016/j.advwatres.2012.02.005
Oostrom, M., Wietsma, T. W., Covert, M. A., and Vermeul, V. R. (2007). Zero-Valent Iron Emplacement in Permeable Porous Media Using Polymer Additions. Ground Water Monit. Remediation 27, 122–130. doi:10.1111/j.1745-6592.2006.00130.x
Palumbo, G., Berent, K., Proniewicz, E., and Banaś, J. (2019). Guar Gum as an Eco-Friendly Corrosion Inhibitor for Pure Aluminium in 1-M HCl Solution. Materials 12, 2620. doi:10.3390/ma12162620
Paveklová, A., Stejskal, V., Vološčuková, O., and Nosek, J. (2020). Cost-effective Remediation Using Microscale ZVI: Comparison of Commercially Available Products. Ecol. Chem. Engineer 27, 211–224. doi:10.2478/eces-2020-0014
Pearce, C. I., Cordova, E. A., Garcia, W. L., Saslow, S. A., Cantrell, K. J., Morad, J. W., et al. (2020). Evaluation of Materials for Iodine and Technetium Immobilization through Sorption and Redox-Driven Processes. Sci. Total Environ. 716, 136167. doi:10.1016/j.scitotenv.2019.136167
P. K. Kitanidis, and P. L. McCarty (Editors) (2012). Delivery and Mixing in the Subsurface: Processes and Design Principles for in Situ Remediation (Berlin, Germany: Springer Science & Business Media).
Rajajayavel, S. R. C., and Ghoshal, S. (2015). Enhanced Reductive Dechlorination of Trichloroethylene by Sulfidated Nanoscale Zerovalent Iron. Water Res. 78, 144–153. doi:10.1016/j.watres.2015.04.009
Smith, M. M., Silva, J. A. K., Munakata-Marr, J., and McCray, J. E. (2008). Compatibility of Polymers and Chemical Oxidants for Enhanced Groundwater Remediation. Environ. Sci. Technol. 42, 9296–9301. doi:10.1021/es800757g
Su, Y., Adeleye, A. S., Keller, A. A., Huang, Y., Dai, C., Zhou, X., et al. (2015). Magnetic Sulfide-Modified Nanoscale Zerovalent Iron (S-nZVI) for Dissolved Metal Ion Removal. Water Res. 74, 47–57. doi:10.1016/j.watres.2015.02.004
Switzer, C., and Kosson, D. S. (2007). Soil Vapor Extraction Performance in Layered Vadose Zone Materials. Vadose zone j. 6, 397–405. doi:10.2136/vzj2005.0131
Testa, S., Guertin, J., Jacobs, J., and Avakian, C. (2004). “Sources of Chromium Contamination in Soil and Groundwater,” in Chromium (VI) Handbook. Editors J. Guertein, J. Jacobs, and C. Avakian (Boca Raton, FL: CRC Press), pp143–164. doi:10.1201/9780203487969.ch4
Thomson, N. R., Fraser, M. J., Lamarche, C., Barker, J. F., and Forsey, S. P. (2008). Rebound of a Coal Tar Creosote Plume Following Partial Source Zone Treatment with Permanganate. J. Contaminant Hydrol. 102, 154–171. doi:10.1016/j.jconhyd.2008.07.001
Tiraferri, A., Chen, K. L., Sethi, R., and Elimelech, M. (2008). Reduced Aggregation and Sedimentation of Zero-Valent Iron Nanoparticles in the Presence of Guar Gum. J. Colloid Interf. Sci. 324, 71–79. doi:10.1016/j.jcis.2008.04.064
Tosco, T., Gastone, F., and Sethi, R. (2014a). Guar Gum Solutions for Improved Delivery of Iron Particles in Porous media (Part 2): Iron Transport Tests and Modeling in Radial Geometry. J. Contaminant Hydrol. 166 (0), 34–51. doi:10.1016/j.jconhyd.2014.06.014
Tosco, T., Petrangeli Papini, M., Cruz Viggi, C., and Sethi, R. (2014b). Nanoscale Zerovalent Iron Particles for Groundwater Remediation: a Review. J. Clean. Prod. 77, 10–21. doi:10.1016/j.jclepro.2013.12.026
Tosco, T., and Sethi, R. (2010). Transport of Non-newtonian Suspensions of Highly Concentrated Micro- and Nanoscale Iron Particles in Porous media: A Modeling Approach. Environ. Sci. Technol. 44, 9062–9068. doi:10.1021/es100868n
Truex, M. J., Vermeul, V. R., Mendoza, D. P., Fritz, B. G., Mackley, R. D., Oostrom, M., et al. (2011). Injection of Zero Valent Iron into an Unconfined Aquifer Using Shear-Thinning Fluids. Groundw. Monit. Rem. 31, 50–58. doi:10.1111/j.1745-6592.2010.01319.x
Truex, M. J., Szecsody, J. E., Qafoku, N., Strickland, C. E., Moran, J. J., Lee, B. D., et al. (2017). Contaminant Attenuation and Transport Characterization of 200-DV-1 Operable Unit Sediment Samples. PNNL-26208; RPT-DVZ-AFRI-037 830403000. Richland WA: Pacific Northwest National Laboratory.
Tufenkji, N., and Elimelech, M. (2004). Deviation from the Classical Colloid Filtration Theory in the Presence of Repulsive DLVO Interactions. Langmuir 20 (25), 10818–10828. doi:10.1021/la0486638
Vecchia, E. D., Luna, M., and Sethi, R. (2009). Transport in Porous media of Highly Concentrated Iron Micro- and Nanoparticles in the Presence of Xanthan Gum. Environ. Sci. Technol. 43, 8942–8947. doi:10.1021/es901897d
Velimirovic, M., Larsson, P.-O., Simons, Q., and Bastiaens, L. (2013). Reactivity Screening of Microscale Zerovalent Irons and Iron Sulfides towards Different CAHs under Standardized Experimental Conditions. J. Hazard. Mater. 252-253, 252–253. doi:10.1016/j.jhazmat.2013.02.047
Velimirovic, M., Chen, H., Simons, Q., and Bastiaens, L. (2012). Reactivity Recovery of Guar Gum Coupled mZVI by Means of Enzymatic Breakdown and Rinsing. J. Contaminant Hydrol. 142-143, 1–10. doi:10.1016/j.jconhyd.2012.09.003
Velimirovic, M., Schmid, D., Wagner, S., Micić, V., von der Kammer, F., and Hofmann, T. (2016). Agar agar-stabilized Milled Zerovalent Iron Particles for In Situ Groundwater Remediation. Sci. Total Environ. 563-564, 713–723. doi:10.1016/j.scitotenv.2015.11.007
Velimirovic, M., Simons, Q., and Bastiaens, L. (2014a). Guar Gum Coupled Microscale ZVI for In Situ Treatment of CAHs: Continuous-Flow Column Study. J. Hazard. Mater. 265, 20–29. doi:10.1016/j.jhazmat.2013.11.020
Velimirovic, M., Tosco, T., Uyttebroek, M., Luna, M., Gastone, F., De Boer, C., et al. (2014b). Field Assessment of Guar Gum Stabilized Microscale Zerovalent Iron Particles for Iin-Ssitu Remediation of 1,1,1-trichloroethane. J. Contaminant Hydrol. 164, 88–99. doi:10.1016/j.jconhyd.2014.05.009
Wu, J., Wang, X.-B., and Zeng, R. J. (2017). Reactivity Enhancement of Iron Sulfide Nanoparticles Stabilized by Sodium Alginate: Taking Cr (VI) Removal as an Example. J. Hazard. Mater. 333, 275–284. doi:10.1016/j.jhazmat.2017.03.023
Zhang, S., Zhang, Z., and Vardhanabhuti, B. (2014). Effect of Charge Density of Polysaccharides on Self-Assembled Intragastric Gelation of Whey Protein/polysaccharide under Simulated Gastric Conditions. Food Funct. 5 (8), 1829–1838. doi:10.1039/c4fo00019f
Zhong, L., Oostrom, M., Truex, M. J., Vermeul, V. R., and Szecsody, J. E. (2013). Rheological Behavior of Xanthan Gum Solution Related to Shear Thinning Fluid Delivery for Subsurface Remediation. J. Hazard. Mater. 244-245, 160–170. doi:10.1016/j.jhazmat.2012.11.028
Zhong, L., Oostrom, M., Wietsma, T. W., and Covert, M. A. (2008). Enhanced Remedial Amendment Delivery through Fluid Viscosity Modifications: Experiments and Numerical Simulations. J. Contaminant Hydrol. 101, 29–41. doi:10.1016/j.jconhyd.2008.07.007
Keywords: particulate reactants, adsorption, reduction, chromate, polymers, remediation
Citation: Muller KA, Zhong L and Bagwell CE (2021) Characterizing the Influence of Organic Polymers on the Specific Reactivity of Particulate Remedial Amendments. Front. Environ. Sci. 9:703851. doi: 10.3389/fenvs.2021.703851
Received: 30 April 2021; Accepted: 10 November 2021;
Published: 30 November 2021.
Edited by:
Balaji Seshadri, The University of Newcastle, AustraliaReviewed by:
Nicholas Kiprotich Cheruiyot, National Kaohsiung University of Science and Technology, TaiwanYanju Liu, The University of Newcastle, Australia
Copyright © 2021 Muller, Zhong and Bagwell. This is an open-access article distributed under the terms of the Creative Commons Attribution License (CC BY). The use, distribution or reproduction in other forums is permitted, provided the original author(s) and the copyright owner(s) are credited and that the original publication in this journal is cited, in accordance with accepted academic practice. No use, distribution or reproduction is permitted which does not comply with these terms.
*Correspondence: Christopher E. Bagwell, Q2hyaXN0b3BoZXIuQmFnd2VsbEBwbm5sLmdvdg==
†These authors have contributed equally to this work and share first authorship