- 1Agronomy and Crop Science, Institute of Crop Science and Plant Breeding, Christian-Albrechts-University, Kiel, Germany
- 2Thünen-Institute of Climate-Smart Agriculture, Braunschweig, Germany
- 3Agricultural Science, Technische Hochschule Ostwestfalen-Lippe, Lemgo, Germany
After the harvest of winter oilseed rape and faba bean crops, considerable high soil nitrate values may be built up before winter in central to north European regions. High precipitation and a low N uptake by the subsequent crop in fall cause a high risk of N2O emissions and nitrate leaching. Microbial decomposition of crop residues or high carbon amendments may immobilize mineral N temporarily and may prevent losses by direct N2O emissions. Five treatments, including crop residue removal and application of different organic amendments after harvest, were tested in a field trial in Northern Germany to elucidate the potential of this mechanism as a mitigation option. N2O emissions and the soil mineral nitrogen status were monitored from August to March for three consecutive years. Observed emissions ranged from 0.1 to 3.4 kg N ha−1 in 180 days. An empirical model approach was applied to separate the impact of spatially and temporally heterogeneous environmental conditions between the plots of the field experiment from treatment effects in the subsequent statistical analysis of N2O emissions. Results show that the exchange of the initial crop residues with organic amendments with high C:N ratios (i.e., winter wheat straw and sawdust) after the harvest of faba bean or oilseed rape can reduce N2O emission during fall and winter by up to 45%.
Introduction
Nitrous oxide (N2O) emissions from agriculture are the largest source of anthropogenic greenhouse gas (GHG) emissions after the combustion of fossil fuels and industrial processes (IPCC, 2014). The largest contributors are direct and indirect emissions of N2O originating from managed soils (IPCC, 2019). Peaks of N₂O emissions from arable soils generally occur when mineral nitrogen (N) concentrations are high, for example, directly after fertilization and after soil cultivation, when increased aeration boosts organic matter turnover (Mosier et al., 1998). In particular, crops that are harvested early and leave behind crop residues with a low C:N ratio create an increased risk of high nitrate accumulation and subsequent N loss during fall and winter (Beaudoin et al., 2005; Rathke et al., 2006; Walter et al., 2015a). This risk results from high mineralization and nitrification rates after harvest and a low N uptake by the following crops in fall (Sieling and Kage, 2006; Henke et al., 2008). In central Europe, the common cultivation of winter oilseed rape (WOSR) with a subsequent winter cereal exhibits all these factors (Sieling and Kage, 2010). Similar conditions can be found after the harvest of faba beans (Sylvester-Bradley and Cross, 1991; Kage, 1997). Various studies have observed that off-season emissions contribute significantly to overall N2O budgets in agricultural soils of the cool–temperate zone (Flessa et al., 1995; Ruser et al., 2001; Tatti et al., 2014). N2O emissions may be even higher after the harvest of WOSR than during the growing season (Walter et al., 2015b).
Mitigation measures lowering N2O emissions can be expected to be most effective if they focus on reducing the availability of mineral N (Di and Cameron, 2002). Less intense tillage can decrease mineralization rates (Goss et al., 1993; Henke et al., 2008) and is already widely practiced. However, no tillage creates drawbacks concerning phytopathological aspects that may lead to a higher use of herbicides (Triplett and Dick, 2008). As an alternative, catch crops are an option to take up available N, retain it, and then release it later via mineralization (Justes et al., 1999; Sieling, 2019). Nevertheless, this approach is limited by the length of the growing period after the harvest of the previous crop. If also a valuable winter crop must be replaced by a spring crop there are economic disadvantages.
Another process decreasing mineral N is immobilization: microbial decomposition of crop residue requires N. If the N demand by microbes exceeds the N input with residues, the available mineral N will be metabolized and converted into organic forms (Chen et al., 2013). The resulting organic N is thereby temporarily saved from loss. A later shift to net mineralization at times of high N uptake rates by the subsequent crop (spring) would improve the temporal synchronization of N supply and N demand. This effect has already been demonstrated (Mitchell et al., 2001; Congreves et al., 2013; Cong et al., 2015) even regarding crop rotations featuring WOSR (Jensen et al., 1997; Trinsoutrot et al., 2000). There is a lack of studies regarding the effect of faba bean residues.
Consequently, the objective of this study was to test the impact of the application of different C-rich amendments after the harvest of WOSR and faba beans in field scale. In focus was the reduction of direct N2O emissions during emission-critical post-harvest and winter periods. Since the C:N ratio of the organic input is supposed to be the main driver of the process, the field trial feature amendments cover a gradient of C:N ratios that are of practical relevance (i.e., local availability).
Spatial and temporal variability of abiotic conditions governing N2O emissions (i.e., soil temperature and soil moisture) cause typically heterogeneous N2O dynamics (Kaiser and Ruser, 2000; Butterbach-Bahl et al., 2013; Lammirato et al., 2018), especially if the data is derived from large experimental areas and over long periods. To disentangle those interactions and account for the large variance of flux rates, abiotic factors were included in the statistical analysis.
Materials and Methods
Site Description
The field trial was conducted from 2015 to 2018 at the experimental station Hohenschulen near Kiel (Northern Germany). The long-term average annual temperature (1993–2018) is 9°C, and the average annual precipitation is 730 mm. During the three observation periods (each year from the harvest of preceding crops in August till the first fertilization in March), daily mean air temperature was between −7.3 and 23.2°C. Air temperatures below 0°C occurred in 2015/16 on 23, in 2016/17 on 35, and in 2017/18 on 22 days. The fall and winter average daily mean temperatures of 2015/16 and 2017/18 were above the long-term average (7.3 and 7.6 compared to 7°C; Figure 1), whereas 2016/17 was below the average (6.7°C). Soil temperatures at 5 cm depth were between −1.3 and 24.2°C. Soil temperatures below 0°C often could not be measured due to impenetrable soil. In terms of precipitation, the 2017/18 fall/winter season was average, whereas 2015/16 was wetter and 2016/17 was rather dry compared to the 25-year average (Figure 1). Single precipitation events up to 43 mm took place, resulting in up to 100% water filled pore space (WFPS).
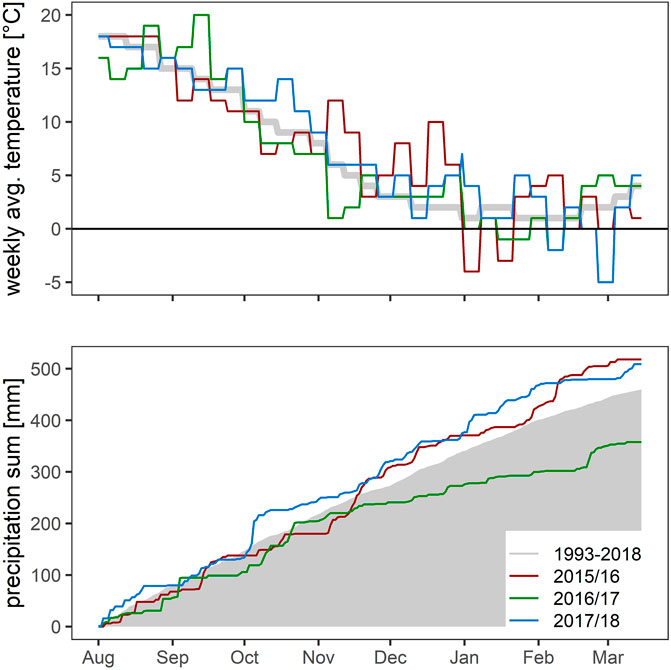
FIGURE 1. Weekly average temperature (°C) and precipitation sum (mm) from August 1 to March 15 per season compared to the long-term average.
The soil is a sandy to clayey loam with the high small-scale heterogeneity typical of the hilly Young Drift moraine landscape. Average pH (CaCl2) was 6.5 ± 0.17, and the soil organic carbon (SOC) content was 1.3 ± 0.1% (Reichel et al., 2018).
Experimental Design and Treatments
The field trial was set up as a split-plot design (Supplementary Figures 1, 2 of the Supplementary Material). Two common local crop rotations were studied: WOSR as preceding crops to winter wheat (WW) followed by winter barley and faba beans–WW–winter barley. In all three experimental years, each crop of both crop rotations was established on main plots. Within each main plot, four amendment treatments (Figure 2) were randomly distributed in subplots. The amendments were:
• keeping the preceding crop residues (control)
• replacement of crop residues with winter wheat straw
• replacement of crop residues with sawdust
• removal of above-ground residues
Within each amendment subplot, four fertilizer intensity treatments to WW (0, 80, 200, and 280 kg N ha−1) were randomly distributed in 3 × 15 m2 sub-subplots. As this study focused on interactions of amendments with soil organic matter, only results prior to fertilizer application will be shown in the following. Thus, the design was crop (n = 2 × 3)/post-harvest amendment (n = 4)/fertilization intensity (n = 4) and replication (n = 4) summing up to 384 plots. The treatment subplots were separated from each other and from the surrounding area by marginal sub-subplots.
WOSR and faba beans were managed according to the common local practice including mineral fertilization of WOSR: 240 kg N ha−1 as calcium ammonium nitrate (CAN) divided into two split-applications during spring. After harvest, above-ground crop residues were removed, and the amendments were applied prior to sowing of subsequent WW (Figure 2). The amendments used in the trial were chosen by practical aspects, that is, local availability and the possibility for coupled use of (agricultural) resources: keeping the preceding crop residues on the field as the control treatment as this is the common local practice. Typically, there is no alternative use for WOSR and faba bean straw. However, those residues were considered for biogas production (e.g., Lönnqvist et al., 2013). Furthermore, WOSR straw is sometimes used in the dairy cow diet (Moss, 2002). Winter wheat straw can be made available from farm internal translocation and shows a considerable higher C:N ratio than both WOSR and faba bean residues (Table 1). Sawdust application was used as a third treatment in order to intensify the approach of C:N ratio–driven immobilization. Contrasting treatment was the sole removal of aboveground residues. The applied amounts of preceding crop residues correspond to actual yield of the preceding crop (averaged per year over the whole experimental site). For winter wheat, an idealized yield of 10 t ha−1 (dry matter) with a harvest index of 0.5 was assumed. Accordingly, the amount of sawdust was the dry matter equivalent to the applied wheat straw. Consequently, amendment treatments differed not only in the C:N ratio but also in the absolute amount of C and N applied.
The straw was chopped during removal and application resulting in particle sizes of 1–10 cm length. Sawdust was already delivered fine-grained, approximately 2–5 mm in diameter. Directly after the application all plots were tilled using a short disk harrow to incorporate the amendments into the first 10 cm of soil. Prior to sowing of WW fields were plowed 30 cm deep. Sowing was combined with seed-bed preparation resulting in further soil perturbation (approximately 10 cm deep). During the growing period, WW received three applications of calcium ammonium nitrate in all treatments, according to the fertilizer intensity treatments. All other crop management (e.g., P and K supply, sowing dates, and pesticide application) was done according to local recommendations for optimal yield.
N2O Flux Measurements
From August (harvest of WOSR) till March (prior to first fertilization of WW), N2O fluxes were measured almost weekly (8 days interval on average), which resulted in >20 measurement campaigns per season. In 2015/16 and 2016/17 in both crop rotations one sub-subplot per treatment and replication was sampled (n = 32). To gain statistical robustness, sample number was doubled in 2017/18 (two sub-subplots per amendment treatment and replication, n = 64) as advised by Lammirato et al. (2018). The fluxes from these expanded samplings were averaged per treatment and replication to ensure a uniform analysis together with the data from the other years. Interactions of N2O emissions with actual fertilization were not in focus as the study aimed on the dynamics of the soil organic C compartments.
N2O emissions were quantified with the manual static chamber method (Hutchinson and Mosier, 1981). In 2015/16, white PVC-chambers of 0.59 m2 area and 0.3 m3 volume were used. In the other two years, smaller rectangular chambers (A = 0.03 m2, V = 0.003 m3) were used. Both chamber types were equipped with rubber lip seals, a thermometer, and a fan. In addition, the smaller chambers had a vent for pressure equalization. At least one day prior to the first sampling, PVC-collars were pushed 3–4 cm into the soil. Remaining above-ground space inside each collar was determined to calculate the overall chamber volume. At the beginning of the sampling, chambers were placed on the collars and kept closed for 1 h. The first gas sample was collected with a 30 ml-syringe from the chamber headspace 1 min after closing of the chamber. The samples were then transferred to pre-evacuated 20 ml glass vials, which were sealed with a rubber septum. Three further samples were taken at constant sampling intervals of 20 min. Using eight chambers at a time one complete replication (all treatments of both crop rotations) could be sampled in one run of 1.3 h. Gas sampling of all replications had to be divided into four sampling runs between 8:30 am and 4:30 pm (fixed plot order throughout the observation period). Parallel to the gas sampling, chamber temperature and soil temperatures in 5 and 10 cm depth were recorded with insertion thermometers (Testo 0560, Testo SE & Co.). The four gas samples per plot were analyzed for N2O concentrations via gas chromatography (GC) and an electron capture detector (ECD). Four standard gases (Linde Gas, Pullach, Germany) in the range 300–3,000 ppb were used for calibration. Performance of the GC (Shimadzu GC-2014, Shimadzu, Kyoto, Japan) and auto-sampler (Greenhouse Workstation, SRI Instruments Europe, Bad Honnef, Germany) system were checked regularly by 10 consecutive measurements of the lowest standard. The resulting coefficient of variation was always below 3% and generally below 2%. Mole fractions were converted to mass concentrations using the ideal gas law. Fluxes were calculated from mass concentration, chamber size, and temperature with the R package gas fluxes (Fuß, 2017). An integrated selection algorithm (Leiber et al., 2014) decided between the nonlinear HMR-model (Hutchinson and Mosier, 1981; Pedersen et al., 2010), the robust linear (Huber, 1981) and the simple linear model. Cumulative emissions were calculated by linear interpolation between two samplings and integration over the entire measurement period for each treatment, replication, and season.
Soil Sampling and Soil Mineral N Analysis
Soil samples were taken from the upper 30 cm soil layer with a 1 cm inner diameter probe and four cores per plot were pooled. Samples down to 90 cm depth were taken with a 2 cm inner diameter probe and three cores were pooled. All samples were kept cool in the field in insulated ice boxes and subsequently stored frozen at −18°C until processing in the lab. Soil mineral nitrogen (SMN, sum of NH4+ -N and NO3− -N contents) was determined with a spectrophotometer after extraction of 50 g soil with 400 ml of a 125 mM CaCl2 solution and 45 min of mechanical shaking followed by centrifugation. A subsample of each soil sample was used for determination of gravimetric water content (dried at 105°C), which was converted to volumetric water content via multiplication with estimated bulk densities of the three soil layers: 1.45 g cm-for 0–30 cm, 1.60 g cm−3 for 30–60 cm, and 1.70 g cm−3 for 60–90 cm.
Due to logistical reasons and sometimes due to impenetrable frozen soil, SMN sampling could not always take place parallel to the N2O sampling. Fall net mineralization was calculated as difference of SMN in 0–90 cm depth in December and at the preceding crop harvest.
Data Processing
Different weather conditions per year and during the observation period as well as the soil heterogeneity of the site caused a wide variation of environmental conditions affecting the N2O emissions at a particular plot. In order to consider this variance in the emission analysis, an evaluation of these conditions was conducted.
Since the abiotic driving factors have a complex, nonlinear influence on the different N2O production processes (Butterbach-Bahl et al., 2013), they were assessed with a procedure based on the approach of Hansen et al. (1990). Empirical functions were used to evaluate the impact of the driving forces on N2O production, that is, soil water content and soil temperature, on a scale from 0 (no emissions) to 1 (optimum for N2O production). The resulting factors were multiplied with the concentration of available mineral N (as substrate for the emission processes).
The general formula for the impact rating is expressed in Eq. 1, which uses the assumption that there is no interaction between the effect of soil temperature and soil water status and that their combined effect is multiplicative (Hansen et al., 1990).
where fabiot is a function describing the impact of abiotic conditions on emissions (abiotic factor), N is the amount of available mineral N (kg N ha−1), f(W) and f(T) are functions that reflect the limitations imposed by moisture and temperature for the topsoil layer (upper 10 cm), respectively. Because this procedure originates from mechanistic modeling of N2O emissions this analysis is a semi-mechanistic model.
The impact of the driving forces differs for nitrification and denitrification. Therefore, the evaluation was carried out separately for both processes with different specific functions as follows:
The indicators fnit and fden reflect the emission potential under the given environmental conditions. However, in contrast to a full mechanistic model, the data evaluation described here was not intended to predict the actual emissions. The soil moisture and temperature functions are defined by Eqs 4–7 (Hansen et al., 1990; Thorburn et al., 2010; Mielenz et al., 2016). The nonlinear assessment of moisture and temperature with respect to N2O emissions is shown exemplarily for a specific soil texture in the Supplementary Material.
pF = decimal logarithm of the matric potential in hPa derived from the actual soil water content (SWC; see the Supplementary Material for details), T = soil temperature in °C, SWC = actual volumetric SWC in %, SWC(WFPScrit_den) = volumetric SWC at critical WFPS above which denitrification takes place (%), and SWCsat = volumetric SWC at saturation (%).
The lower threshold for denitrification SWC(WFPScrit_den) in Eq. 6 was set to the drained upper limit (DUL ≙ field capacity) of the specific soil. For simplification, we determined soil texture on main plot level and used tabulated values for the corresponding DULs (Boden, 2005). SWC, T, and pF values were derived from measurements. Although the treatment factor did not show a significant effect on the measured values of soil moisture and soil temperature, an inter-dependency of the amendment treatment and those parameters, soil moisture, soil temperature, and SMN content, was assumed (details can be seen in the Supplementary Material). Since this might mask the treatment effect, plot-level values of these parameters were averaged per main plot. Daily values were generated by linear interpolation between two consecutive sampling events. The daily values for the abiotic factors were then cumulated over the entire observation period. The resulting indicators quantify the abiotic conditions, separately for the main N2O production processes, under which the observed emissions occurred. The indicators also reflect the different lengths of the observation periods that occurred due to different harvest dates of the preceding crops and due to different field conditions per year. Statistical analyses were carried out with R (R Core Team, 2019). We fitted a linear mixed effects model using the package nlme (Pinheiro et al., 2019). A subsequent multiple comparisons of groups were performed with the package multcomp (Hothorn et al., 2008). A detailed description of the process including R code can be found in the Supplementary Material. Results are presented as arithmetic mean ± 1 standard deviation if not stated differently in the text. Statistical significance was set to α = 0.05.
Results
Soil Mineral N Dynamics
After WOSR, which had been fertilized with 240 kg N ha−1, residual N (= fertilizer N – grain N content x yield) of the years 2015–2017 on average exceeded 100 kg N ha−1. Since faba beans were not fertilized, the residual N calculation without consideration of the N fixation was always negative. However, SMN contents after harvest (Figure 3) also suggest a high potential for N loss after faba beans. In fall, SMN contents in the topsoil layer (0–30 cm) usually increased, reaching a peak shortly after sowing of WW (see Figure 4 and the SMN dynamics of all plots in the Supplementary Material, chapter 4). This was most apparent in the residue-free treatment. Although the differences at the peak between the residue-free treatment and the other treatments were small (9–13 kg N ha−1), a buffer effect of amendment application was apparent. A temporal delayed appearance of the SMN peaks with increasing soil depth indicated the translocation of NO3− with soil water flow. Shortly before the first fertilizer application in March, SMN contents of all treatments and layers leveled off to 15 ± 9 kg ha−1.
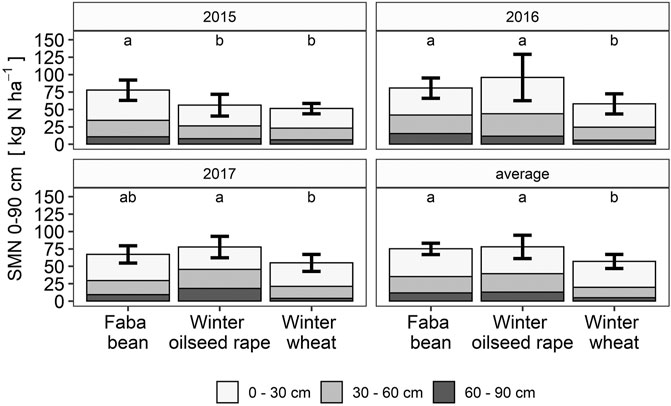
FIGURE 3. SMN contents in 0–90 cm after harvest for each experimental year; FB, faba bean, WOSR, winter oilseed rape (240 kg N/ha), WW, winter wheat (200 kg N/ha); error bars indicate standard deviation of total SMN in 0–90 cm; letters indicate significant differences per season (linear mixed-effects model, p < 0.05).
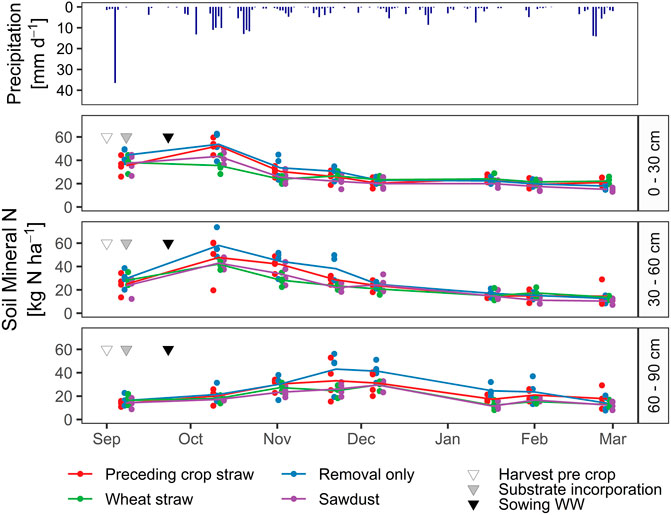
FIGURE 4. Example SMN dynamics from 2016/17 after faba bean for the three soil layers 0–30, 30–60, and 60–90 cm depth; points indicate measured contents; lines indicate linear interpolated mean values.
Averaged over all treatments, calculated fall net mineralization was negative with a mean of −21 ± 22 kg N ha−1 (Figure 5). Averaged over all three years, fall net mineralization in WOSR rotation after application of winter wheat residues was significantly smaller than without residues (difference of 25 ± 6 kg N ha−1; see the Supplementary Material for details). N2O flux dynamics.
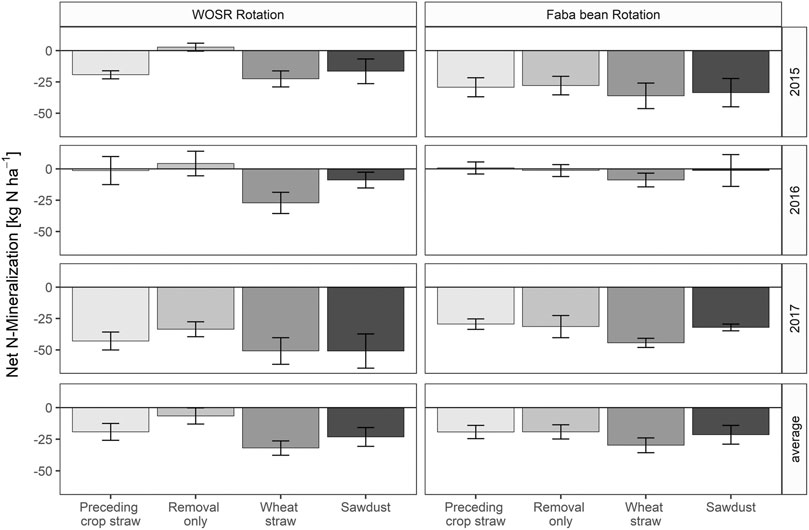
FIGURE 5. Difference of SMN concentrations in 0–90 cm depth at the preceding crop harvest and the beginning of December (= Net N-Mineralization); error bars indicate standard error.
On 69 measurement dates 2861 flux rates were determined. For the majority (n = 2759) the robust linear regression was used for flux rate calculation, HMR was rarely selected (64 times). Linear regression was used 38 times because one out of four gas samples had been lost. Mean observed flux rate was 14 ± 54 μg N2O-N m−2 h−1. This can be considered low to moderate although emission peaks up to 1,665 μg N2O-N m−2 h−1 occurred on single plots (Figure 6). Peaks of average emissions were observed in 2016 and 2017 in the warm period between mid of August and beginning of September with average emissions of 76 ± 95 and 83 ± 95 μg N2O-N m−2 h−1, respectively. Therefore, this particular period might be identified as a literal hot moment for post-harvest emissions: on average of the experimental years the daily mean temperature between August 15 and September 15 was 16 ± 2°C, which caused increased emissions. The average temperature dropped in the following 30 days to 12 ± 3°C, while correspondingly lower emissions occurred (Figure 6). N2O emission data did not cover this period in 2015 due to a delayed start of measurement. However, peaks in 2015/16 had an average magnitude of 49 ± 151 μg N2O-N m−2 h−1 and occurred in January 2016, presumably due to a freeze–thaw event (Song et al., 2017). Such incidents also occurred in the other trial years but with a less intense increase of emissions (Figure 6).
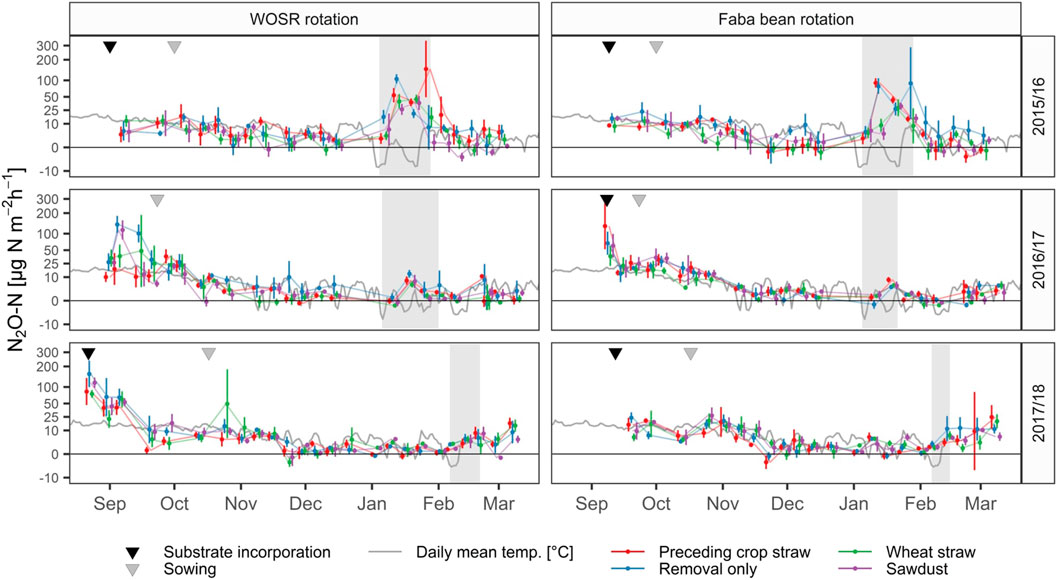
FIGURE 6. N2O-N flux rates per treatment and season; points are mean values; lines represent linear interpolation; error bars indicate ± standard error; y-axis scaled after modulus transformation (John and Draper, 1980); freeze–thaw events are highlighted with gray background.
N2O emissions and corresponding interpolated SMN contents are slightly positively correlated. The Spearman rank correlation coefficient is 0.37 with p < 2.2e−16. Flux rates were not immediately sensitive to soil perturbation events, that is, substrate incorporation (30 cm deep by plowing) and sowing (10 cm deep), or heavy rainfall events (precipitation data not shown). These events were not followed by a substantial increase (Figure 6).
Cumulated N2O Emissions
Cumulated N2O emissions of the treatment/year combinations within the observation period of roughly over 6 months were between 0.1 and 3.4 kg N ha−1 with a mean of 0.54 ± 0.55 kg N ha−1 (Table 2). In addition to the varying length of the observation period and the influence of annual weather conditions, soil texture heterogeneity across the experimental site contributed to a high variability of N2O emissions, leading to a few captured extreme fluxes that govern the cumulated emissions.
A multi-factor ANOVA showed that the cumulated value of the abiotic factor for nitrification fnit is sensitive (p < 0.01) to 1) the inter-annual variability of climatic conditions, 2) the different lengths of measurement periods per crop rotation and season, as well as 3) the spatial variability of the site and the different sampling times in dependency of the replications. The same analysis for the factor of denitrification fden suggested dependency on the preceding crop (p < 0.01; for details and underlying data, see Supplementary Section S5).
The linear mixed effect model for evaluation of cumulative N2O fluxes includes the preceding crop (rotation) and the amendment treatment as main factors and the abiotic factors as numeric covariates (fixed effects). Interactions between the fixed effects factors were included except between both abiotic factors. Season (i.e., the year of harvest of winter wheat), the replication ID, and preceding crop were set as nested random effect, which reflects the structure of the split-plot design of the trial and allows individual intercept estimation per level combination. To achieve (approximate) normal distribution of residuals log-transformation of the dependent variable (cumulated N2O emissions) was conducted as it was done for similar models (for example, Walter et al., 2015a; Ruser et al., 2017). The wide 95% confidence intervals of the model estimates (Supplementary Table 19 in the Supplementary Material and retransformed in Figure 7) indicate a large amount of uncertainty of the effects.
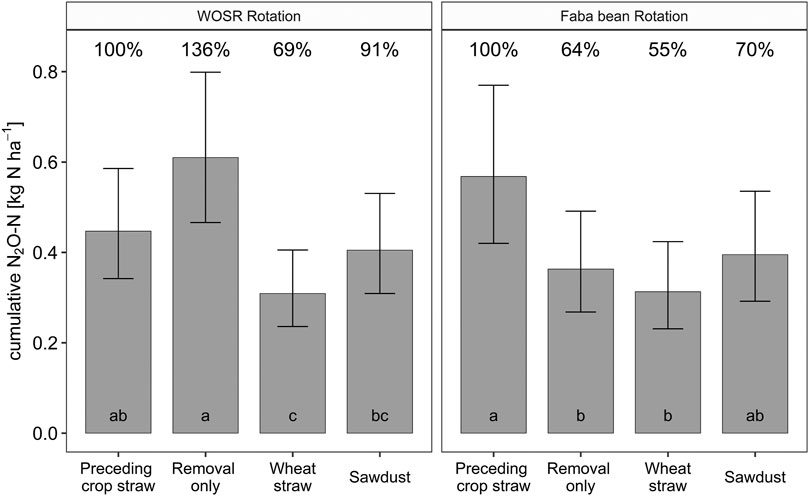
FIGURE 7. Cumulative N2O-N emissions calculated with the regression model at average abiotic emission conditions (i.e., average period length, temperature sum, moisture status, and N-source status) and re-transformed from log-transformation; minor letters indicate significant differences of the model estimates (p < 0.05) per crop rotation; error bars indicate the 95% confidence interval; percent values refer to the control treatment.
An analysis of co-variance (marginal ANCOVA) was employed subsequently to obtain p-values (Supplementary Table 18). The significant interactions of treatment factors (crop rotation and amendment treatment) and the covariates cause different slope estimations for each combination of the treatment factor levels. Consequently, the differences between groups depend on the actual level of the covariates. A regular multiple comparison of groups would compare the intercepts at a covariate level of 0, which is not meaningful as the covariates reflect the emission conditions. To solve this issue and additionally reduce co-linearity as assessed by generalized variance-inflation factors (VIF), the abiotic factors were centered (see for example Garcia et al., 2016). This way the intercepts of each rotation–amendment combination were compared at average emission conditions.
The retransformed emission values derived from the model estimates after centering of covariates (Figure 7) can be understood as an estimate of the potential emissions at average abiotic emission conditions. The modeled emissions can hardly be compared with the observed values, since the field conditions never matched this average. The abiotic emission conditions cover the length of the observation period, the temperature sum and moisture content as well as the N availability for the respective N2O formation process. The model estimates were compared per rotation–amendment group. A significant difference between the treatment with wheat straw amendment and the treatments where the WOSR residues were kept or completely removed was revealed (30 and 67% reduction, respectively). Removal of the WOSR residues resulted in the highest N2O emissions at average abiotic conditions with an increase by almost 40% compared to the control treatment (p < 0.1).
In contrast, in the faba bean rotation, keeping the bean residues increased emissions (p < 0.05). Removal of the legume residues as well as the exchange with wheat straw produced less N2O (36 and 45% reduction, respectively, p < 0.001). Although sawdust application lowered emissions compared to the control treatment in both rotations, the difference was not significant.
Discussion
Soil Mineral N Dynamics
Residual SMN after the harvest of preceding crops confirmed a high risk of N losses. The high N surplus after WOSR can be partially attributed to a low N removal with harvest due to low seed yields, which were remarkably below the average seed yield of the previous years (Sieling et al., 1999). SMN contents correlated strongly with dry matter crop yield, as it determined both, N withdrawal from the soil and the total dry matter of residues (detailed yield parameters are available in the Supplementary Material).
Despite a net mineralization in the first 6–8 weeks after harvest on all plots, a buffering of the SMN peaks as well as a less pronounced NO3− translocation on plots with amendments clearly indicated fall N-immobilization-mineralization as proposed by Chen et al. (2014). This hypothesis was further supported generally by a negative net N mineralization calculated for the period from harvest to December. Lowest net N mineralization could be observed after application of winter wheat straw which features the highest C:N ratio leaving sawdust aside. The effect size decreases with a decrease in the C:N ratio of the amendments. Occasionally, even positive net mineralization was observed on plots without any amendment. This emphasizes the role of the C:N ratio as a main driver of immobilization. However, sawdust is an exception: Its small effect on SMN dynamics despite its extremely high C:N ratio was probably due to the high lignin content of wood. Lignin causes low C mineralization rates and thus lowers N immobilization (Jensen et al., 2005; Popa et al., 2008). In contrast, Reichel et al. (2018) demonstrated the immobilization potential of the same material in an incubation experiment including additional N fertilization. Latter might be necessary to induce the microbial decomposition of the otherwise recalcitrant sawdust.
It should be noted that precipitation data suggest a contribution of N leaching losses (translocation of SMN deeper than 90 cm) to the change of SMN in fall (Di and Cameron, 2002). Lack of data prevents leaching quantification. Gaseous losses were a minor factor since the average cumulated N2O emissions for the entire observation period hardly exceeded 1.0 kg N ha−1. It can be assumed that emissions of other N gases (N2, NO) were not much larger (e.g., Ruser et al., 2006; Wu et al., 2018; Liao et al., 2020). In addition, N-uptake by winter wheat could be assumed to be negligibly small (5 ± 1.6 kg N ha−1 based on destructive measurements in December 2015).
N2O Emissions
The emissions presented were quite common for this climate zone, the observation period, and the agricultural system. Ruser et al. (2017) measured N2O fluxes at the same site in winter wheat after WOSR from sowing till end of December in 2013–2015 and observed only mean fluxes <100 μg N2O-N m−2 h−1. Vinzent et al. (2017), in a study in southern Germany in 2013 and 2014, rarely observed flux rates above 50 μg N2O-N m−2 h−1 in the post-harvest periods after WOSR. Resulting cumulative emissions were between 0.3 and 2.0 kg N2O-N ha−1. Likewise, Walter et al. (2015a) found only cumulative emissions <0.5 kg N2O-N ha−1 in winter 2012/13 after WOSR at a site in central Germany.
Since soil moisture was no limiting factor (almost constantly above 64% WFPS), low temperatures in fall/winter are most likely to cause the low N2O emissions (Butterbach-Bahl et al., 2013). Higher emissions were observed after WOSR than after faba bean (n.s.). While average post-harvest SMN contents were at a similar level indicating a high N2O loss potential for both crops, the fallow periods till WW sowing were longer after WOSR due to an earlier harvest compared to faba beans (2–3 weeks). The higher daily mean temperatures and the already abundant precipitation favored N2O production and affected substantially the cumulated emissions of that particular period. Due to this fact, a further comparison of the amendment treatment effect on crop rotation level was considered to be not meaningful.
Although considerable temporal and spatial heterogeneity of N2O dynamics was suggested in the literature, the observed variability was larger than expected. Consequently, differences between the treatments in terms of absolute emissions were inconclusive. The linear mixed effects model adequately took into account random effects and variance heterogeneity in dependency of the experimental year and the location, helping to identify the treatment effects under average conditions.
The abiotic factors as a proxy of the effect of environmental conditions over time explained a significant part of the variance among the data. Spatial variation of fden was noticeably higher than that of fnit (Figure 8). Presumably, this is a consequence of the sensitivity of the denitrification factor to the actual SWC: the outcome of the assessment depended considerably on the selected threshold for denitrification conditions.
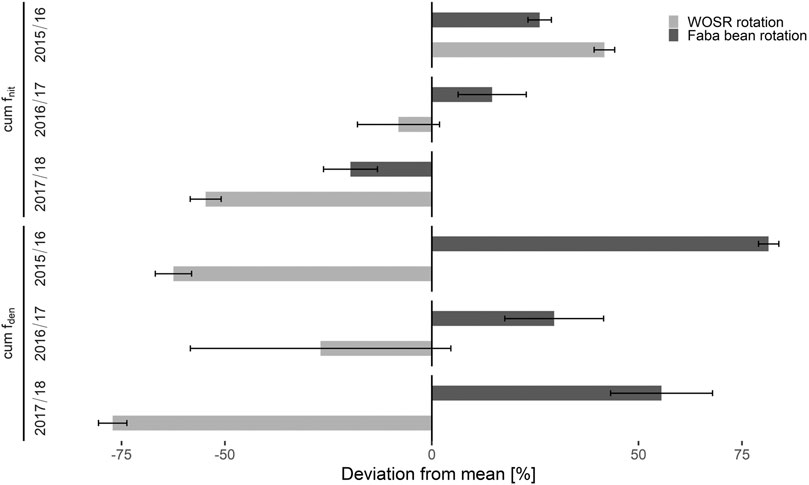
FIGURE 8. Deviation from the respective mean of the cumulated daily abiotic factors for nitrification and denitrification, averaged per season; error bars indicate ± standard error across replications. High values of the abiotic factors equal conditions favoring N2O emissions.
Unfortunately, the lower limit of SWC for beginning of denitrification is not uniformly defined (Barton et al., 1999; Heinen, 2006). It seems to be highly site and soil specific. Hence, the critical threshold in Eq. 7 was generalized as suggested by Thorburn et al. (2010). This generalization offered the option to consider denitrification below DUL. Still, due to a lack of more accurate data we used WFPS at DUL for the specific soil texture. Consequently, fden varied with the local soil texture (and its DUL) and the current SWC. Both varied highly on a small scale at the given site. However, given that nitrate as well as organic carbon as a prerequisite for denitrification was abundant and temperatures above 2°C already allow the reaction, SWC as the dominant environmental controller for O2 availability was the main governing factor of denitrification (Firestone and Davidson, 1989).
The model indicated a 30% reduction in N2O emissions when WOSR residues were replaced with WW straw as hypothesized. Still, the conventional practice of leaving the residues on the field causes lower emissions compared to residue removal, for which 40% higher emissions were estimated. Similar to the SMN dynamics, the relatively recalcitrant sawdust affected the N2O dynamics only to a small extent.
Baggs et al. (2003) reported higher N2O emissions after incorporation of faba bean residues, compared to a cereal straw treatment as well as to a treatment without residues. Most likely the quite low C:N ratio of faba bean residues favored fast microbial decomposition and hence temporary anaerobic microsites might have been created where emissions by denitrification are dominant (Gök and Ottow, 1988; Huang et al., 2004; Jensen et al., 2005). In treatments with slow-reacting amendments (WW straw and sawdust) as well as in the soils without additional amounts of organic matter, this mechanism may not have been initiated during the time of observation.
The absolute differences of average direct N2O emissions under the control treatment and the application of WW residues were 137 g N2O-N ha−1 in 182 days. This is equivalent to 40.5 kg CO2-eq. emission, assuming a GWP100 for N2O of 296 (Myhre et al., 2013). The magnitude of typical N2O emissions under a whole year of WOSR cultivation in Germany has been reported to be 1 kg N2O-N (Ruser et al., 2017). Roughly estimated, a post-harvest treatment with WW residues could lower direct annual N2O emissions from WOSR cultivation by almost 14%. It is noteworthy that this calculation does not consider the effect on mitigating N-leaching and related indirect emissions. Also, NH3 emissions have been neglected. Former studies at the same site showed that noteworthy NH3 emissions only occur after manure/slurry application (Räbiger et al., 2020), which is not applicable for the presented experiment. Annual production emissions, that is, the sum of CO2-equivalent emission for tillage, harvest operations, and N fertilization of WOSR cropping are about 1,500–2000 kg CO2eq ha−1 (Neeft et al., 2012; BioGrace-I GHG calculation tool-version 4d, 2015). Consequently, a reduction of 40.5 kg CO2-eq ha−1 would reduce the total greenhouse gas emissions by up to 2.7%. However, for a comprehensive assessment, the effects on other greenhouse gases must also be taken into account.
Conclusion
Residual SMN after the harvest of WOSR and faba beans is higher than that after cereals. So is the risk of gaseous N losses. At sites with comparable conditions as described in the present study, removal of WOSR residues (e.g., for biogas production or dairy cow diet) would increase N2O emissions and should be avoided. In contrast, faba bean residues seem to stimulate N2O emissions after harvest. The present study shows that the replacement of preceding crop residues with winter wheat straw, which features a higher C:N ratio, buffers SMN increase in fall and mitigates N2O emissions by up to 45%.
The analysis method featuring abiotic environmental conditions as explaining covariates assesses the varying environmental conditions and adjusts for them. Hence, it allows performing uniform comparisons of emissions from a dataset covering various observation periods and a heterogeneous site.
Although the findings confirm the C:N ratio as a main driver of the immobilization process, there are further aspects like the recalcitrance of the main amendment constituents and the abiotic factors ruling the N2O emissions. Therefore, recommendations for farmers have to be site-specific, considering various features of the available amendments and the environmental conditions.
Data Availability Statement
The datasets presented in this study can be found in BonaRes online repository https://doi.org/10.20387/bonares-25h2-a5yp, https://doi.org/10.20387/BonaRes-zdw4-083t.
Author Contributions
SR wrote the manuscript. IP, HK, and SR designed the study. SR performed the sampling, data preparation, and analysis. RF supervised the trace gas analysis. IP and HK contributed central ideas. RF, IP, and HK revised the manuscript.
Funding
This work was supported by the German Federal Ministry of Education and Research (BMBF) (grant number 031A561G). Also, we acknowledge financial support by DFG within the funding programme Open Access Publizieren.
Conflict of Interest
The authors declare that the research was conducted in the absence of any commercial or financial relationships that could be construed as a potential conflict of interest.
Acknowledgments
We would like to thank all our INPLAMINT project partners for fruitful meetings, Kerstin Gilke and Andrea Oehns-Rittgerodt of Thünen Institute for the GC-analysis, Mario Hasler for statistical advice, our technical assistant Anja Wolff, and the numerous research assistants for their help with the field work. The corresponding author thanks Insa Kühling and Klaus Sieling for proofreading.
Supplementary Material
The Supplementary Material for this article can be found online at: https://www.frontiersin.org/articles/10.3389/fenvs.2021.712013/full#supplementary-material
References
Baggs, E. M., Stevenson, M., Pihlatie, M., Regar, A., Cook, H., and Cadisch, G. (2003). Nitrous Oxide Emissions Following Application of Residues and Fertiliser under Zero and Conventional Tillage. Plant Soil 254, 361–370. doi:10.1023/A:1025593121839
Barton, L., McLay, C. D. A., Schipper, L. A., and Smith, C. T. (1999). Annual Denitrification Rates in Agricultural and forest Soils: a Review. Soil Res. 37, 1073–1094. doi:10.1071/sr99009
Beaudoin, N., Saad, J. K., Van Laethem, C., Machet, J. M., Maucorps, J., Mary, B., et al. (2005). Nitrate Leaching in Intensive Agriculture in Northern France: Effect of Farming Practices, Soils and Crop Rotations. Agric. Ecosyst. Environ. 111, 292–310. doi:10.1016/j.agee.2005.06.006
BioGrace-I GHG calculation tool-version 4d (2015). Align Biofuel GHG Emission Calculations in Europe (BioGrace), Project Funded by Intelligent Energy Europe Programme. Available from: https://www.biograce.net/home (accessed 04 06, 2020).
Boden, A.-H.-A. (2005). Bodenkundliche Kartieranleitung. 5th Edn. Hannover, Germany: Schweizerbart Science Publishers.
Butterbach-Bahl, K., Baggs, E. M., Dannenmann, M., Kiese, R., and Zechmeister-Boltenstern, S. (2013). Nitrous Oxide Emissions from Soils: How Well Do We Understand the Processes and Their Controls? Phil. Trans. R. Soc. B 368, 20130122. doi:10.1098/rstb.2013.0122
Chen, B., Liu, E., Tian, Q., Yan, C., and Zhang, Y. (2014). Soil Nitrogen Dynamics and Crop Residues. A Review. Agron. Sustain. Dev. 34, 429–442. doi:10.1007/s13593-014-0207-8
Chen, H., Li, X., Hu, F., and Shi, W. (2013). Soil Nitrous Oxide Emissions Following Crop Residue Addition: a Meta-Analysis. Glob. Change Biol. 19, 2956–2964. doi:10.1111/gcb.12274
Cong, W.-F., Hoffland, E., Li, L., Janssen, B. H., and van der Werf, W. (2015). Intercropping Affects the Rate of Decomposition of Soil Organic Matter and Root Litter. Plant Soil 391, 399–411. doi:10.1007/s11104-015-2433-5
Congreves, K., Vyn, R., and Van Eerd, L. (2013). Evaluation of Post-Harvest Organic Carbon Amendments as a Strategy to Minimize Nitrogen Losses in Cole Crop Production. Agronomy 3, 181–199. doi:10.3390/agronomy3010181
Di, H. J., and Cameron, K. C. (2002). Nitrate Leaching in Temperate Agroecosystems: Sources, Factors and Mitigating Strategies. Nutr. Cycl. Agroecosystems 64, 237–256. doi:10.1023/A:1021471531188
IPCC (2019). 2019 Refinement to the 2006 IPCC Guidelines for National Greenhouse Gas Inventories. Editors E. Calvo Buendia, K. Tanabe, A. Kranjc, J. Baasansuren, M. Fukuda, S. Ngarizeet al. (Switzerland: Published: IPCC).
Firestone, M. K., and Davidson, E. A. (1989). Microbiological Basis of NO and N2O Production and Consumption in Soil. Exch. Trace Gases Terr. Ecosyst. Atmosphere 47, 7–21.
Flessa, H., Dörsch, P., and Beese, F. (1995). Seasonal Variation of N2O and CH4fluxes in Differently Managed Arable Soils in Southern Germany. J. Geophys. Res. 100, 23115–23124. doi:10.1029/95JD02270
Fuß, R., 2017. Gasfluxes: Greenhouse Gas Flux Calculation from Chamber Measurements. R package version 0.2-1.
García, J., Salmerón, R., García, C., and López Martíndel, M. d. M. M. L. (2016). Standardization of Variables and Collinearity Diagnostic in Ridge Regression. Int. Stat. Rev. 84, 245–266. doi:10.1111/insr.12099
Gök, M., and Ottow, J. C. G. (1988). Effect of Cellulose and Straw Incorporation in Soil on Total Denitrification and Nitrogen Immobilization at Initially Aerobic and Permanent Anaerobic Conditions. Biol. Fertil. Soils 5, 317–322. doi:10.1007/BF00262139
Goss, M. J., Howse, K. R., Lane, P. W., Christian, D. G., and Harris, G. L. (1993). Losses of Nitrate-Nitrogen in Water Draining from under Autumn-Sown Crops Established by Direct Drilling or Mouldboard Ploughing. J. Soil Sci. 44, 35–48. doi:10.1111/j.1365-2389.1993.tb00432.x
Hansen, S., Jensen, H. E., Nielsen, N. E., and Svendsen, H. (1990). NPo-Research, A10: DAISY: Soil Plant Atmosphere System Model. Copenhagen, Denmark: Miljoestyrelsen.
Heinen, M. (2006). Application of a Widely Used Denitrification Model to Dutch Data Sets. Geoderma 133, 464–473. doi:10.1016/j.geoderma.2005.08.011
Henke, J., Böttcher, U., Neukam, D., Sieling, K., and Kage, H. (2008). Evaluation of Different Agronomic Strategies to Reduce Nitrate Leaching after winter Oilseed Rape (Brassica Napus L.) Using a Simulation Model. Nutr. Cycl Agroecosyst 82, 299–314. doi:10.1007/s10705-008-9192-0
Hothorn, T., Bretz, F., and Westfall, P. (2008). Simultaneous Inference in General Parametric Models. Biom. J. 50, 346–363. doi:10.1002/bimj.200810425
Huang, Y., Zou, J., Zheng, X., Wang, Y., and Xu, X. (2004). Nitrous Oxide Emissions as Influenced by Amendment of Plant Residues with Different C:N Ratios. Soil Biol. Biochem. 36, 973–981. doi:10.1016/j.soilbio.2004.02.009
Huber, P. J. (1981). Robust Statistics, Wiley Series in Probability and Statistics. New York: John Wiley and Sons. doi:10.1002/0471725250.ch7
Hutchinson, G. L., and Mosier, A. R. (1981). Improved Soil Cover Method for Field Measurement of Nitrous Oxide Fluxes. Soil Sci. Soc. America J. 45, 311–316. doi:10.2136/sssaj1981.03615995004500020017x
IPCC (2014). Climate Change 2014: Mitigation of Climate Change: Working Group III Contribution to the Fifth Assessment Report of the Intergovernmental Panel on Climate Change. New York, NY: Cambridge University Press.
Jensen, L. S., Mueller, T., Magid, J., and Nielsen, N. E. (1997). Temporal Variation of C and N Mineralization, Microbial Biomass and Extractable Organic Pools in Soil after Oilseed Rape Straw Incorporation in the Field. Soil Biol. Biochem. 29, 1043–1055. doi:10.1016/S0038-0717(97)00014-X
Jensen, L. S., Salo, T., Palmason, F., Breland, T. A., Henriksen, T. M., Stenberg, B., et al. (2005). Influence of Biochemical Quality on C and N Mineralisation from a Broad Variety of Plant Materials in Soil. Plant Soil 273, 307–326. doi:10.1007/s11104-004-8128-y
John, J. A., and Draper, N. R. (1980). An Alternative Family of Transformations. Appl. Stat. 29, 190–197. doi:10.2307/2986305
Justes, E., Mary, B., and Nicolardot, B. (1999). Comparing the Effectiveness of Radish Cover Crop, Oilseed Rape Volunteers and Oilseed Rape Residues Incorporation for Reducing Nitrate Leaching. Nutr. Cycl. Agroecosystems 55, 207–220. doi:10.1023/A:1009870401779
Kage, H. (1997). Is Low Rooting Density of Faba Beans a Cause of High Residual Nitrate Content of Soil at Harvest?. Plant Soil 190, 47–60. doi:10.1023/a:1004250905262
Kaiser, E.-A., and Ruser, R. (2000). Nitrous Oxide Emissions from Arable Soils in Germany - an Evaluation of Six Long-Term Field Experiments. J. Plant Nutr. Soil Sci. 163, 249–259. doi:10.1002/1522-2624(200006)163:3
Lammirato, C., Lebender, U., Tierling, J., and Lammel, J. (2018). Analysis of Uncertainty for N2 O Fluxes Measured with the Closed-Chamber Method under Field Conditions: Calculation Method, Detection Limit, and Spatial Variability. J. Plant Nutr. Soil Sci. 181, 78–89. doi:10.1002/jpln.201600499
Leiber, K., Fuß, R., Voigt, C., and Freibauer, A., 2014. High CO2 Fluxes from Grassland on Histic Gleysol along Soil Carbon and Drainage Gradients. Biogeosciences 11:749–761. doi:10.5194/bg-11-749-2014
Liao, X., Niu, Y., Liu, D., Chen, Z., He, T., Luo, J., et al. (2020). Four-year Continuous Residual Effects of Biochar Application to a sandy Loam Soil on Crop Yield and N2O and NO Emissions under maize-wheat Rotation. Agric. Ecosyst. Environ. 302, 107109. doi:10.1016/j.agee.2020.107109
Lönnqvist, T., Silveira, S., and Sanches-Pereira, A. (2013). Swedish Resource Potential from Residues and Energy Crops to Enhance Biogas Generation. Renew. Sust. Energ. Rev. 21, 298–314. doi:10.1016/j.rser.2012.12.024
Mielenz, H., Thorburn, P. J., Scheer, C., De Antoni Migliorati, M., Grace, P. R., and Bell, M. J. (2016). Opportunities for Mitigating Nitrous Oxide Emissions in Subtropical Cereal and Fiber Cropping Systems: A Simulation Study. Agric. Ecosyst. Environ. 218, 11–27. doi:10.1016/j.agee.2015.11.008
Mitchell, R., Webb, J., and Harrison, R. (2001). Crop Residues Can Affect N Leaching over at Least Two winters. Eur. J. Agron. 15, 17–29. doi:10.1016/S1161-0301(00)00088-5
Mosier, A., Kroeze, C., Nevison, C., Oenema, O., Seitzinger, S., and van Cleemput, O. (1998). Closing the Global N2O Budget: Nitrous Oxide Emissions through the Agricultural Nitrogen Cycle. Nutr. Cycl. Agroecosystems 52, 225–248. doi:10.1023/A:1009740530221
Moss, A. (2002). The Effects of Long-Term Feeding of Extracted Rapeseed Meal and Whole Rapeseed on the Physical and Financial Performance, Health and Welfare of High Yielding Dairy Cows (No. OS59). Reading, GB: ADAS.
Myhre, G., Shindell, D., Bréon, F.-M., Collins, W., Fuglestvedt, J., Huang, J., et al. (2013). Anthropogenic and Natural Radiative Forcing, in: Climate Change 2013: The Physical Science Basis. Contribution of Working Group I to the Fifth Assessment Report of the Intergovernmental Panel on Climate Change. Editors T. F. Stocker, D. Qin, G.-K. Plattner, M. Tignor, S. K. Allen, J. Boschunget al. (Cambridge, United Kingdom and New York, NY: Cambridge University Press), 82.
Neeft, J., Gagnepain, B., Bacovsky, D., Ludwiczek, N., Lavelle, P., Thonier, G., et al. (2012). BioGrace Publishable Final Report 28. Heidelberg, Germany
Pedersen, A. R., Petersen, S. O., and Schelde, K. (2010). A Comprehensive Approach to Soil-Atmosphere Trace-Gas Flux Estimation With Static Chambers. Eur. J. Soil Sci. 61, 888–902. doi:10.1111/j.1365-2389.2010.01291.x
Pinheiro, J., Bates, D., DebRoy, S., and Sarkar, D. (2019). Nlme: Linear and Nonlinear Mixed Effects Models. R. Package Version 3, 1–131.
Popa, V. I., Dumitru, M., Volf, I., and Anghel, N. (2008). Lignin and Polyphenols as Allelochemicals. Ind. Crops Prod. 27, 144–149. doi:10.1016/j.indcrop.2007.07.019
Rathke, G., Behrens, T., and Diepenbrock, W. (2006). Integrated Nitrogen Management Strategies to Improve Seed Yield, Oil Content and Nitrogen Efficiency of Winter Oilseed Rape (Brassica Napus L.). A review. Agric. Ecosyst. Environ. 117, 80–108. doi:10.1016/j.agee.2006.04.006
R Core Team (2019). R: A Language and Environment for Statistical Computing. Vienna, Austria: R Foundation for Statistical Computing.
Räbiger, T., Andres, M., Hegewald, H., Kesenheimer, K., Köbke, S., Quinones, T. S., et al. (2020). Indirect Nitrous Oxide Emissions from Oilseed Rape Cropping Systems by NH3 Volatilization and Nitrate Leaching as Affected by Nitrogen Source, N Rate and Site Conditions. Eur. J. Agron. 116, 126039. doi:10.1016/j.eja.2020.126039
Reichel, R., Wei, J., Islam, M. S., Schmid, C., Wissel, H., Schröder, P., et al. (2018). Potential of Wheat Straw, Spruce Sawdust, and Lignin as High Organic Carbon Soil Amendments to Improve Agricultural Nitrogen Retention Capacity: An Incubation Study. Front. Plant Sci. 9. doi:10.3389/fpls.2018.00900
Ruser, R., Flessa, H., Russow, R., Schmidt, G., Buegger, F., and Munch, J. C. (2006). Emission of N2O, N2 and CO2 from Soil Fertilized with Nitrate: Effect of Compaction, Soil Moisture and Rewetting. Soil Biol. Biochem. 38, 263–274. doi:10.1016/j.soilbio.2005.05.005
Ruser, R., Flessa, H., Schilling, R., Beese, F., and Munch, J. C. (2001). Effect of Crop-specific Field Management and N Fertilization on N2O Emissions from a fine-loamy Soil. Nutr. Cycl. Agroecosystems 59, 177–191. doi:10.1023/A:1017512205888
Ruser, R., Fuß, R., Andres, M., Hegewald, H., Kesenheimer, K., Köbke, S., et al. (2017). Nitrous Oxide Emissions from winter Oilseed Rape Cultivation. Agric. Ecosyst. Environ. 249, 57–69. doi:10.1016/j.agee.2017.07.039
Sieling, K., Günther-borstel, O., Teebken, T., and Hanus, H. (1999). Soil mineral N and N Net Mineralization during Autumn and winter under an Oilseed Rape - winter Wheat - winter Barley Rotation in Different Crop Management Systems. J. Agric. Sci. 132, 127–137. doi:10.1017/s0021859698006273
Sieling, K. (2019). Improved N Transfer by Growing Catch Crops – a challenge. J. Für Kult. 71, 145–160. doi:10.5073/JfK.2019.06.01
Sieling, K., and Kage, H. (2010). Efficient N Management Using winter Oilseed Rape. A Review. Agron. Sustain. Dev. 30, 271–279. doi:10.1051/agro/2009036
Sieling, K., and Kage, H. (2006). N Balance as an Indicator of N Leaching in an Oilseed Rape - winter Wheat - winter Barley Rotation. Agric. Ecosyst. Environ. 115, 261–269. doi:10.1016/j.agee.2006.01.011
Song, Y., Zou, Y., Wang, G., and Yu, X. (2017). Altered Soil Carbon and Nitrogen Cycles Due to the Freeze-Thaw Effect: A Meta-Analysis. Soil Biol. Biochem. 109, 35–49. doi:10.1016/j.soilbio.2017.01.020
Sylvester-Bradley, R., and Cross, R. B. (1991). Nitrogen Residues from Peas and Beans and the Response of the Following Cereal to Applied Nitrogen. Asp. Appl. Biol. 27, 293–298.
Tatti, E., Goyer, C., Chantigny, M., Wertz, S., Zebarth, B. J., Burton, D. L., et al. (2014). Influences of over winter Conditions on Denitrification and Nitrous Oxide-Producing Microorganism Abundance and Structure in an Agricultural Soil Amended with Different Nitrogen Sources. Agric. Ecosyst. Environ. 183, 47–59. doi:10.1016/j.agee.2013.10.021
Thorburn, P. J., Biggs, J. S., Collins, K., and Probert, M. E. (2010). Using the APSIM Model to Estimate Nitrous Oxide Emissions from Diverse Australian Sugarcane Production Systems. Agric. Ecosyst. Environ. 136, 343–350. doi:10.1016/j.agee.2009.12.014
Trinsoutrot, I., Nicolardot, B., Justes, E., and Recous, S. (2000). Decomposition in the Field of Residues of Oilseed Rape Grown at Two Levels of Nitrogen Fertilisation. Effects on the Dynamics of Soil mineral Nitrogen between Successive Crops. Nutr. Cycl. Agroecosystems 56, 125–137. doi:10.1023/A:1009838618133
Triplett, G. B., and Dick, W. A. (2008). No-Tillage Crop Production: A Revolution in Agriculture!. Agron. J. 100, S-153-S-165. doi:10.2134/agronj2007.0005c
Vinzent, B., Fuß, R., Maidl, F.-X., and Hülsbergen, K.-J. (2017). Efficacy of Agronomic Strategies for Mitigation of After-Harvest N 2 O Emissions of winter Oilseed Rape. Eur. J. Agron. 89, 88–96. doi:10.1016/j.eja.2017.06.009
Walter, K., Don, A., and Flessa, H. (2015a). Net N 2 O and CH 4 Soil Fluxes of Annual and Perennial Bioenergy Crops in Two central German Regions. Biomass and Bioenergy 81, 556–567. doi:10.1016/j.biombioe.2015.08.011
Walter, K., Don, A., Fuß, R., Kern, J., Drewer, J., and Flessa, H. (2015b). Direct Nitrous Oxide Emissions from Oilseed Rape Cropping - a Meta‐analysis. GCB Bioenergy 7, 1260–1271. doi:10.1111/gcbb.12223
Keywords: residue management, N2O emission, N loss mitigation, organic soil amendments, vicia faba, brassica napus, triticum aestivum
Citation: Rothardt S, Fuß R, Pahlmann I and Kage H (2021) Post-Harvest N2O Emissions Can Be Mitigated With Organic Amendments. Front. Environ. Sci. 9:712013. doi: 10.3389/fenvs.2021.712013
Received: 19 May 2021; Accepted: 18 June 2021;
Published: 16 July 2021.
Edited by:
Rajni Singh, Amity University, IndiaReviewed by:
Anjana Srivastava, Govind Ballabh Pant University of Agriculture and Technology, IndiaNicola Dal Ferro, University of Padua, Italy
Copyright © 2021 Rothardt, Fuß, Pahlmann and Kage. This is an open-access article distributed under the terms of the Creative Commons Attribution License (CC BY). The use, distribution or reproduction in other forums is permitted, provided the original author(s) and the copyright owner(s) are credited and that the original publication in this journal is cited, in accordance with accepted academic practice. No use, distribution or reproduction is permitted which does not comply with these terms.
*Correspondence: S. Rothardt, cm90aGFyZHRAcGZsYW56ZW5iYXUudW5pLWtpZWwuZGU=