Assessment of Conventional Full-Scale Treatment for the Removal of Endocrine Disruptors and Pharmaceuticals Present in the Tibagi River (Paraná State, Brazil)
- 1Departamento de Ambiental, Universidade Tecnológica Federal do Paraná (UTFPR), Campus Londrina, Londrina, Brazil
- 2Programa de Pós-Graduação em Engenharia Ambiental (PROAMB), Universidade Federal de Ouro Preto (UFOP), Campus Morro do Cruzeiro, Ouro Preto, Brazil
The concentrations of 25 pharmaceuticals and endocrine disruptors were monitored in the water coming from the Tibagi River (State of Paraná, Brazil) and in a conventional water treatment plant over 13 sampling campaigns. In raw water, only 3 compounds (bisphenol A, dexamethasone and losartan) were detected with high frequency (>75%) and 4 drugs (estradiol, diclofenac, loratadine and naproxen) were found with moderate frequency (between 30 and 70%). In addition, 7 micropollutants (paracetamol, ethinylestradiol, caffeine, propanolol, diltiazem, benzafibrate and promethazine) were not detected in any of the samples analyzed and 11 other compounds were quantified at low frequency (up to 25%). The conventional treatment process employed at WTP- Jataizinho has proven to be very efficient in removing dexamethasone (∼99%), moderately efficient in reducing bisphenol A (∼47%) concentration and inefficient in removing losartan (∼22%) and loratadine (not removed). The greatest removals were observed during the water clarification stage using aluminum sulfate as coagulant. In general, the dry and rainy seasons did not influence the concentrations of pharmaceuticals and endocrine disruptors in raw water. In terms of the 5 most prevalent micropollutants in treated water (bisphenol A, losartan, dexamethasone, loratadine and naproxen), the human health risk associated with ingesting contaminated water was assessed and considered negligible.
Introduction
Contaminants of emerging concern (CECs) are compounds of natural or synthetic origin that have been detected in different environmental compartments on all continents (Küster and Adler, 2014; Gothwal and Shashidhar, 2015; Yang et al., 2017; Bexfield et al., 2019; Patel et al., 2019) The CECs, also known as micropollutants and microcontaminants, can be classified into various groups according to their properties and purposes, such as pharmaceuticals and endocrine disruptors. Pharmaceuticals generally include antibiotics, hormones, analgesics, anti-inflammatories, lipid regulators, beta-blockers, among others (Hu et al., 2017). Endocrine disruptors comprise synthetic substances (alkylphenols, agrochemicals, phthalates, polychlorinated biphenyls, bisphenol A, trace metals, among others) and natural substances (natural estrogens and phytoestrogens) (Yang et al., 2017).
In recent decades, the incidence of pharmaceuticals, personal care products and endocrine disruptors has received attention due to their occurrence, persistence and potential effect on human health and ecosystems (Wu et al., 2012; Tran et al., 2018). These micropollutants are introduced into the aquatic environment by different routes, which include the discharge of sanitary sewage, hospital effluents and pharmaceutical industries (Tran et al., 2018; Patel et al., 2019), landfill leachate (Buszka et al., 2009; Li et al., 2016), runoff from urban or agricultural areas and aquaculture activities (Nikolaou et al., 2007; Lees et al., 2016; Burns et al., 2018).
Because Wastewater Treatment Plants (WWTPs) are inefficient in removing pharmaceuticals and endocrine disruptors (World Health Organization, 2012; Archer et al., 2017), these have frequently been detected in treated sewage in concentrations ranging from ng L−1 to μg L−1 (Tran et al., 2018). Thus, the release of sewage, treated or in natura, is considered one of the main sources of such contaminants in the aquatic environment. The concern is mainly because the discharge into the environment is generally continuous, since many pharmaceuticals are consumed over a long period and in large part of the user’s life. After use, pharmaceuticals can be absorbed, partially metabolized and excreted in feces and urine of humans and animals (Jjemba, 2006).
The presence of pharmaceutical drugs in water varies regionally, partly due to prescription practices, socio-economic conditions, hydrology, water resource management, urbanization, age composition of the population, among others (Burns et al., 2018; Tran et al., 2018; Santos et al., 2020). Moreover, seasonal variations can be attributed to flow fluctuations (dry and rainy periods) and the prevalence of certain diseases in different seasons (Patel et al., 2019).
In view of the adverse effects of pharmaceuticals and endocrine disruptors already observed in aquatic biota (Brozinski et al., 2013; Rand-Weaver et al., 2013; Li et al., 2016; Xu et al., 2019) special attention has been given to the presence of these micropollutants in surface waters used for public supply, since drinking water (DW) can be an important route of human exposure to these contaminants (Machado et al., 2016). One of the first assessments on emerging contaminants in water supplies was conducted by Focazio et al. (2008) in the United States. They assessed the presence of 100 substances in 49 samples from surface and DW sources, supplying populations in several American states. Out of the 100 compounds tested, 63 were detected, including cholesterol, metolachlor, carbamazepine and bisphenol A. Other comprehensive studies were also carried out by Watkinson et al. (2009) in Australia and Meffe and de Bustamante (2014) in Italy, Carmona et al. (2014) in Spain, de Jesus Gaffney et al. (2015) in Portugal, aus der Beek et al. (2016) in several countries, among others.
In Brazil, Machado et al. (2016) analyzed 100 samples of DW, collected in 22 Brazilian State capitals, and 7 of these samples came from surface springs. Caffeine and atrazine were the most frequently detected micropollutants, both in springs and in public water supply. In turn, Reis et al. (2019) analyzed 28 drugs, in superficial springs and water treatment plants (WTPs) in the State of Minas Gerais. Eighteen drugs were detected in superficial springs and 11 in treated water. The concentrations in the treated water were lower than those present in the springs, thus demonstrating that at least part of the contaminants can be removed in conventional treatment.
Although WTPs can be an important barrier to reducing the risk of involuntary human intake of micropollutants in natural waters, in general, WTPs have limited efficiency in removing pharmaceuticals and endocrine disruptors (Huerta-Fontela et al., 2011; Yang et al., 2017; Reis et al., 2019; Santos et al., 2020; World Health Organization, 2012). Studies conducted in conventional WTPs have shown that coagulation is not very efficient in removing pharmaceutical products, while better results are obtained during water disinfection, which is usually performed with chlorine, chlorine dioxide and ozone (World Health Organization, 2012).
Low percentage removal of diclofenac (30%), ibuprofen (10%), carbamazepine (insignificant), bezafibrate (∼10%), and sulfamethoxazole (insignificant) was observed in the coagulation step in a dose of 50 mg L−1 of ferric chloride and a concentration range of 30–40 μg L−1 of the pharmaceuticals. On the other hand, water chlorination decreased the amount of acetaminophen, sulfamethoxazole and erythromycin by almost 75% (Vieno et al., 2006). Stackelberg et al. (2007) analyzed water and sludge samples in a conventional WTP and observed, in general, that the clarification step accounted for only 15% of the removal, while disinfection (32%) and filtration with activated carbon (53%) were more efficient.
Hence, the quantitative investigation of micropollutants in water sources and DW constitutes a fundamental aspect for the understanding of the existing gaps regarding the possible effects of these substances on biota and human health. In the literature, there have been reported more studies on WWTPs than on WTPs, and often pharmaceuticals removal processes were conducted in laboratory experiments (Yang et al., 2017; Kim et al., 2020). Furthermore, studies considering the temporal variability of micropollutants in supply sources are infrequent (Paíga et al., 2016; Hu et al., 2017; Burns et al., 2018; Santos et al., 2020).
Therefore, this work aimed to analyze the presence of 25 drugs and endocrine disruptors in raw water (RW) of the Tibagi River, to verify the removal of these compounds in the conventional treatment plant in the city of Jataizinho (PR) in 13 sampling campaigns during the dry and rainy seasons. In addition, it has been evaluated whether the remaining concentration of these compounds in the treated water poses significant risk to the human health. The hypothesis behind these objectives is that although Brazilian rivers are polluted with contaminants of emerging concern due to the discharge of sewage (raw or partially treated), specially in the dry season, the technologies employed in the WTP can reduce the concentration of such compounds to levels deemed safe for human health.
Materials and Methods
Study Area and Collection Points
The Tibagi River basin (TRB) is situated in the central-eastern portion of Paraná State and has an area of 25,239 km2, which corresponds to approximately 13% of the State surface. The Tibagi River rises in the south of the state of Paraná, in the southern region of Brazil, at 1,060 m of altitude and travels 550 km in a northerly direction, having its mouth at the lake of the Capivara hydroelectric power plant in the river Paranapanema at 298 m of altitude. Along its way there are 91 jumps and waterfalls, and it receives waters from 65 direct tributaries and hundreds of subtributaries (SEMA, 2015).
The average annual rainfall of the basin varies between 1,200 mm and 1900 mm (IAPAR-EMATER, 2018). The distribution of the average monthly precipitation values shows regularity along the basin and a period of low precipitation between the months of April and August (dry period), with a total average of 93 mm, while the maximum expected is around 112 mm. During the months of September to March (rainy period), the average and minimum values are 149 and 111 mm, close to the maximum expected for the dry period.
The land-use of the TRB is characterized by industries, intensive agricultural activities and a medium concentration of cities (upper Tibagi); agropastoral activity and low concentration of cities (middle Tibagi); and industries, agricultural activities and high concentration of cities (lower Tibagi). It is estimated that the basin has 3.8% native forests, 27.6% secondary vegetation, 9.4% reforestation, 18.1% pastures, 40.1% agriculture and 1% other uses, among them urban centers. The TRB extends over 49 municipalities, with a resident population of approximately 2 million inhabitants, of which 88% in urban areas (IPARDES, 2018).
Sewage collection and treatment systems in the Tibagi River basin cover 82% of the urban population and 54 WWTPs are in operation. Near the collection point used in this study (20 km upstream of the city of Jataizinho), the Tibagi river receives treated sewage from four WWTPs located in southern Londrina, with a total average flow of 670 L/s (Figure 1.).
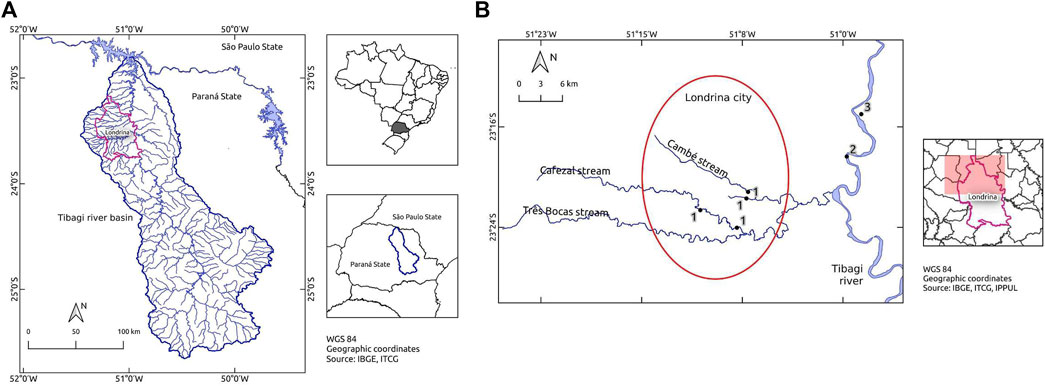
FIGURE 1. Map of Tibagi river basin and Tibagi River location indicating: 1) Sewage treatment plants (23o21′23´´ e 51o07′03´´; 23o21′52´´e 51o07′11´´, 23o22′43´´e 51o10′37´´, 23o24′02´´e 51o07′54´´) 2) Tibagi river collection point and (23o28′43´´,50o59′43´´) 3) the Jataizinho water treatment plant (23o15′33´´ e 50o58′38´´).
Currently, the flow rate of ETA Jataizinho on the Tibagi river is 60 L s−1. The WTP is of the complete cycle type and has a hydraulic flocculator of vertical flow (descending/ascending), with wooden chicanes and hydraulic retention time of 35 min to perform the floculation process. Three conventional decanters are used with horizontal flow, surface application rate of 2 m3 m−2 h−1 and hydraulic retention time of 2.5 h, whereby the washing of each decanter occurs every 45–60 days. The WTP has three rapid filters, with sand filter media (diameter ranging 0.4–0.6 mm and 0.6 m depth). The filters operate at a variable declining rate (average 10 m3 m−2 h−1). In periods of low rainfall, the turbidity of RW reaches values in the range of 10 uT, and filtering intervals are between 5 and 7 days, with one filter being washed every 2 days with treated water from an elevated reservoir (filters backwashed with water only). Finally, the filtered water (FW) is sent to a 50 m3 contact tank, where disinfection occurs by adding chlorine gas. Subsequently, the water is stored in a 1700 m3 supporting reservoir, from where it is pumped to a 50 m3 elevated reservoir and then distributed to the city. The total volume of reservoir (1800 m3) allows maintaining the water supply to the city for more than 17 h (IPARDES, 2018).
Sample Collection and Physicochemical Analysis
As previously mentioned, the WTP operates at a flow rate of 60 L s−1, resulting in operating times between 10 and 14 h per day (intermittence in water production). In general, the station operates with less interruptions in the morning. Hence, it was chosen to collect four 500 ml aliquots, at hourly frequency, to make up a 2 L composite sample during such period.
RW samples were collected in the inlet channel, upstream of the Parshall flume, using a 500 ml graduated glass cylinder, previously prepared (washed with 10% nitric acid). Among the collections, the glass cylinder was washed with RW for five times. Each aliquot (500 ml) was added to a 2-L glass container and kept under refrigeration. At the WTP, the filtered water is directed through a closed pipe to the contact chamber, where disinfection occurs, and without access for collection. At the outlet of each filter, pumps were installed that take the FW through pipes to the operational and quality control laboratory. The filter chosen for sampling was the one that had been washed a short time ago. During collection of aliquots, the pump was turned on for 3 min, then the graduated cylinder was washed five times with FW, and a volume of 500 ml was collected to constitute the composite sample. Each aliquot (500 ml) was added to a 2-L glass container and kept under refrigeration (4°C). Samples of the filter washing water (FWW) were collected in the outlet channel of the washing water. After the floodgate was opened, the initial outlet water was discarded (1–2 min) and a sample (approximately 2 L) of the washing water was collected.
The collection of DW was performed through a tap, available in the laboratory, which receives treated water from the raised reservoir. During collection of the aliquots, the tap was opened for 3 min, then the graduated cylinder was washed five times with the DW and a volume of 500 ml was collected to constitute the composite sample. Each aliquot (500 ml) was added to a 2-L glass container and kept under refrigeration (4°C).
The methods described in Standard Methods for the Examination of Water and Wastewater, 22nd edition (APHA, AWWA, WEF, 2012), indicated in Table 1, were used to perform physicochemical characterization of water samples collected at WTP- Jataizinho.
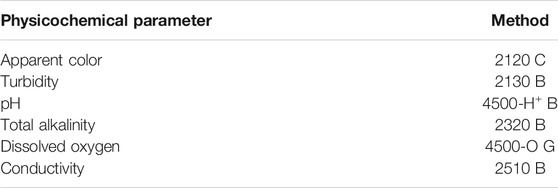
TABLE 1. Parameters and methods used for the physicochemical analysis of the water collected at Jataizinho WTP.
Extraction of Micropollutants
The analyses of pharmaceuticals and endocrine disruptors were based on the work of Corrêa et al. (2021) and Sanson. (2012), developed at the Laboratory of Molecular Characterization and Mass Spectrometry (LABMASSAS) of Universidade Federal de Ouro Preto (UFOP). For this purpose, the water samples collected in composed form immediately underwent vacuum filtration processes using fiberglass membranes of different porosities (8 μm, followed by 2 and 0.45 µm) for the removal of particulate material.
Filtered samples had their pH corrected to 2.0 ± 0.2 using hydrochloric acid (25%), followed by the addition of 500 mg of ethylenediaminetetraacetic acid (EDTA). The samples were kept at rest with stirring every 20 min and were ready for solid phase extraction (SPE) after 60 min of EDTA addition. Subsequently, the samples were submitted to extraction processes using solid phase extraction (SPE) cartridges filled with the modified divinylbenzene adsorber phase consisting of lipophilic and hydrophilic sites (Strata X®, Phenomenex). The extraction procedure was performed under positive nitrogen pressure according to the methodology described by Sanson et al. (2014).
The SPE cartridges containing the compounds of interest were identified and stored in a freezer for subsequent sending to the LABMASSAS of UFOP. In LABMASSAS the SPE cartridges were submitted to the elution of the analytes using 9 ml of ethyl acetate in a 12-port vacuum manifold. Immediately after the elution, the resulting extracts were dried with gaseous nitrogen by means of a concentrating apparatus that maintained the extract at room temperature during drying. The vials containing the dried extracts were kept in a freezer until analysis.
Gas Chromatography Coupled to Mass Spectrometry Analysis
The extracts from the SPE were redissolved in 500 µL of HPLC grade methanol (JTBaker), agitated in vortex for approximately 30 s and transferred to two vials containing volume restrictor. The vial (A) contained 100 µL sample and the second vial (Aspike) 70 µL sample +30 µL standard solution of 100 μg L−1 of analytes in methanol. The extracts were then dried completely under nitrogen gas flow and kept in a freezer (−26°C) until analysis (Corrêa et al., 2021).
For the analytical curves, HPLC grade methanol stock solutions (JTBaker) of each analyte (ibuprofen, paracetamol, 4-nonylphenol, 4-octylphenol, bisphenol A, genfibrozil, estrone, estradiol, ethinylestradiol and estriol) were prepared in 1 g L−1 concentration. From these solutions, working solutions were prepared at a concentration of 1 mg L−1 in methanol and containing all the analytes, which were also stored in a freezer. For the analytical curves, dilutions with methanol were performed from the working solution on the day of the analysis, being the concentration range from 2.5 to 100 μg L−1. Vials containing the solutions for constructing the analytical curves were also submitted to dryness under nitrogen gas flow. On the day of analysis, all vials were submitted to derivatization and then redissolved with 75 µL of N,O-Bis(trimethylsilyl)trifluoroacetamide: Trimethylchlorosilane (BSTFA:TMCS, 99:1, GCMS, Sigma Aldrich) and 25 µL of pyridine solution (Merck) containing 200 μg L−1 of 4-n-nonylphenol-2,3,5,6-d4 (CDN Isotopes), used as internal standard (IS). Subsequently, they were maintained at 80°C for 30 min and then subjected to gas chromatography coupled to mass spectrometry (GC-MS) analysis (Sanson, 2012).
All analyses were performed in a gas chromatograph coupled to a mass spectrometer, model GCMS-QP2010 Plus (Shimadzu), at LABMASSAS of UFOP. The injection of 1 µL of the samples was performed by the automatic injector model AOC-20i (Shimadzu) and the chromatographic column used was the Zebron ZB-5MSi (30 m × 0.25 mm × 0.25 µm, Phenomenex). The temperature ramp used was 120°C for 1 min, rising to 227°C with a heating rate of 15°C min−1, then increasing to 240°C with a rate of 10°C min−1 and finally reaching 330°C at 15°C min−1 and remaining at this temperature for 2 min. Injection mode was splitless for 0.5 min, followed by 1:20 split rate, purge flow 5.0 ml min−1 and injector temperature 280°C. The carrier gas used was grade 5.0 helium with a total flow of 25.9 ml min−1 and a linear velocity of 36.5 cm s−1. For the mass spectrometer temperatures of 280°C were used at the interface and 250°C at the ionization source, employing 70 eV for electron ionization. The mass/charge ratios (m/z) monitored for each derivatized analyte are described in Table 2, as well as their retention times (Corrêa et al., 2021).
The response used to construct the analytical curves was the ratio of analyte peak area to internal standard peak area (IS) (y-axis) against analyte concentration (x-axis). Thus, for the samples, the ratio between the areas of the analyte and IS was also used. Eq. (1) was used for the evaluation of the matrix effect (ME), in which Aspike is the area of the analyte in the spike vial, A is the area of the analyte in the sample vial and in the denominator is represented the area of the analyte at 30 μg L−1 according to the equation of the analytical curve.
LC-MS/MS Analysis
The same extracts obtained in item 2.4 after redissolving the SPE extract in 500 µL of HPLC grade methanol (J.T. Baker), was transferred (100 µL) to a vial containing a volume restrictor. This extract was then dried under nitrogen gas flow and kept in a freezer (−26°C) until analysis.
For the analytical curves, stock solutions in methanol HPLC grade (J.T. Baker) of each of the analytes (metformin, acyclovir, atenolol, caffeine, linezolid, propanolol, diltiazem, promethazine, losartan, bezafibrate, diclofenac, dexamethasone, loratadine, sulfamethoxazole and naproxen) were prepared in 1 g L−1 concentration. The working solutions containing all the analytes were prepared from these solutions at a concentration of 1 mg L−1, in methanol and containing all the analytes, which were also stored in a freezer. For the analytical curves, dilutions were made with methanol containing 0.1% v/v formic acid (88%, J.T. Baker) from the working solution on the day of analysis with a concentration range of 2.5–50 μg L−1. In addition, vial named standard solution (Astandard) were prepared, containing standard solution in methanol with 0.1% v/v formic acid with all the analytes at a concentration of 30 μg L−1, and the vial named solvent (Asolvent) containing only methanol with 0.1% v/v formic acid.
On the day of sample analysis, the vials containing the dry extract were redissolved with 100 µL of methanol with 0.1% v/v formic acid. The analyses were performed using the LCMS-8040 equipment (Shimadzu) coupled with the UHPLC model Nexera (Shimadzu) with the following modules: CBM-20A controller, 3 LC-30AD pumps, SIL-30AC sampler, CTO-30A column oven, and DGU-20As degasser. A C18 chromatographic column model Kinetex (Phenomenex) 100 mm × 2.1 mm × 2.6 µm was used. The mobile phase consisted of 1) ultrapure water with 0.1% v/v formic acid; 2) acetonitrile (HPLC grade, J. T. Baker) with 0.1% v/v formic acid, using the following gradient steps of solvents: 5% B from 0 to 1 min, reaching 60% B at 12 min, rising to 95% B at 13 min and returning to the initial condition at 14 min. This resulted in a total running time of 16 min under a flow rate of 0.250 ml min−1. A third mobile phase 3), acetonitrile solution containing 3.5 mM ammonium hydroxide (28%, Sigma Aldrich), was subsequently added post column at a flow rate of 0.03 ml min−1.
Each sample was injected twice: (Asolvent) 10 µL sample +5 µL solvent and (Astandard) 10 µL + 5 µL standard solution. This analysis was performed to calculate the punctual matrix effect for each sample and each analyte. The same injection model was performed with standard solutions of the analytical curves, whereby the calibration curve was constructed using the ratio estimated by Eq. 2 and the analyte concentration.
The general conditions employed in the method used for the mass spectrometer were: 230 kPa of 5.0 argon at collision-induced dissociation cell, 350°C for interface temperature, 200°C for desolvation line temperature, 400°C for heat block temperature, nebulizing nitrogen gas flow of 1.5 L min−1 and 15.00 L min−1 for drying gas. The precursor ions, products monitored in Multiple Reaction Monitoring (MRM) mode and the retention time used for each analyte are described in Table 3. After the LC-MS/MS analyses, data were treated considering the recovery percentage of each analyte. The matrix effect was corrected using the ratio as a response, i.e., the correction of the effect was punctual in relation to the sample and the analyte.
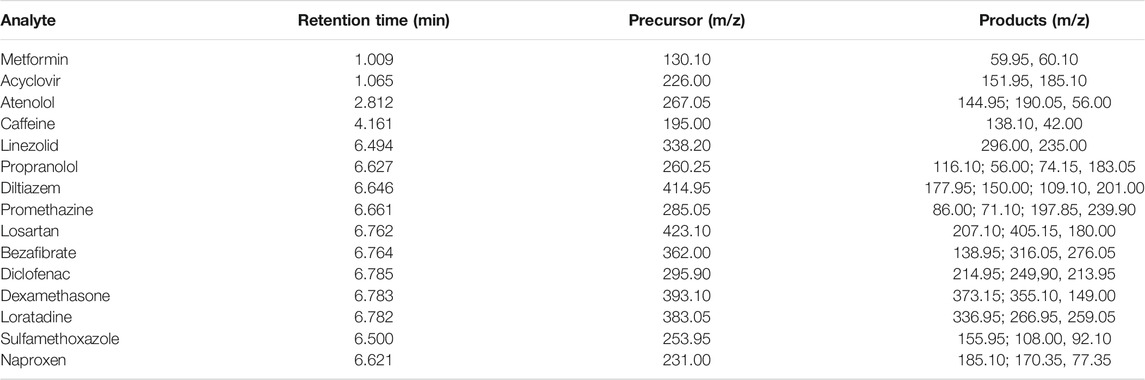
TABLE 3. Mass/charge ratio (m/z) of precursor and products monitored in LC/MS in Multiple Reaction Monitoring (MRM) mode.
Risk Analysis
Risk analysis of the population exposure to pharmaceuticals and endocrine disruptors by consumption of treated water was performed by calculating the margin of exposure (MOE). The MOE was estimated by the ratio between the guide value (calculated from epidemiological and/or toxicological information) and the concentration of the contaminant (maximum reported value or 95th percentile) in the water distributed to the population (EPHC NHMRC NRMMC, 2008; USEPA, 2016) as per Eq. 3. The MOE represents whether the occurrence of the compound in question is lower or higher than its guide value, and was used for risk classification as follows: 1) MOE ≤1 (imminent risk) for micropollutants in treated water at concentrations higher than or equal to the guide values and therefore pose health risks; 2) 1 < MOE <10 (high risk) for micropollutants found in treated water in concentrations lower than the guide values, but in the same order of magnitude as the concentrations which would represent health risks; 3) 10 < ME ≤ 100 (moderate risk) for micropollutants occurring in treated water in concentrations lower than the guide values in up to two orders of magnitude; 4) 100 < ME (low risk) for micropollutants found in treated water at concentrations at least one hundred times lower than the guide values.
Where: MOE is the margin of exposure; GV is the guide value (calculated by Eq. 4 using the lowest tolerable daily intake value reported in the literature) and OC is the occurrence of the micropollutant in treated water (in this work the 95th percentile of the concentration of the pharmaceutical/endocrine disruptor in treated water was considered).
Where: TDI is the tolerable daily intake (μg/kg/d), derived from epidemiological or toxicological studies and, in the case of drugs. The TDI can be estimated by the therapeutic doses reported by manufacturers or pharmacopoeias considering an uncertainty factor, as presented in Eq. 5; BW means body weight (60 kg for the Brazilian population); AF means allocation factor (proportion of TDI attributed to water intake, which varies according to the contaminant) and V is the daily average water consumption (2 L/d for the Brazilian population).
Where: MDTD means the minimum daily therapeutic dose (mg d−1) and UF is the uncertainty factor (EPHC NHMRC NRMMC, 2008).
The TDI values used in the risk assessment performed in this work were obtained from the compilation presented by (Brandt et al., 2019).
Results
Study Area and Physicochemical Analysis
The monitoring network of the water resources of the Tibagi basin is composed of 71 rain stations, 13 climatological, 41 fluviometric, 25 sediment and 26 monitoring stations of water quality. The monitoring station “Chácara Ana Cláudia" (coordinates 23° 18' 43″ and 50° 59' 43″), implemented on January 07, 1977, is located between the point of effluent discharge of the WWTPs from the cities of Londrina, Cambé and Jataizinho (Figure 1). In the area near the monitoring station there is great urban concentration and land use diversity with predominance of intensive agriculture. The water quality data in this station, in the period between 1998 and 2007 (18 samples), showed Water Quality Index (WQI) values between 59 and 83, of which 90% of the time with good quality and 10%, optimum (World Health Organization, 2011). Comparing with the Class 2 quality standard, established by Conama Resolution 357/2005, of the 18 samples analyzed, all meet pH and dissolved oxygen (DO) standards, 84% biochemical oxygen demand (BOD), 95% turbidity, 89% phosphorus and 92% thermotolerant coliforms. More recent data (October 2016 to March 2019) indicated WQI values in the range of 52–69, with E. Coli and total phosphorus above the quality standards. Despite a slight worsening, the water quality is considered compatible with class 2, with no restriction of use for public supply. Table 4 includes the values of physicochemical parameters measured in the samples collected, as well as the number of micropollutants with concentrations above the limit of detection for each sample.
Supplementary Figure S1 shows the average monthly precipitation values at the IAPAR Station, located in southern Londrina. It can be noticed a clear difference in the average monthly precipitation between dry and rainy periods. It was found that the average monthly flow of the Tibagi River was 582 m3 s−1 during the rainy period and 251 m3 s−1 in the dry period.
Occurrence of Micropollutants
The concentrations of pharmaceuticals and endocrine disruptors detected in RW supplying WTP-Jataizinho is shown in the Supplementary Table S1. In general, of the 25 pharmaceuticals and endocrine disruptors analyzed, in 67% of the samples they were not detected or were found with low frequency (in up to 23%). The seven microcontaminants which were not detected in RW were: paracetamol, ethinylestradiol, caffeine, propanolol, diltiazem, benzafibrate and promethazine. Only three compounds (bisphenol A (BPA), dexametazone (DXM) and losartan (LST)) were detected with a high frequency (above 77%) and four pharmaceuticals (estradiol, diclofenac, loratadine (LRT), naproxen (NPX)) were found with moderate frequency (between 30 and 69%). Among the five most prevalent substances in RW, only the endocrine disruptor BPA (chemical input used in the manufacture of resins and plastics) is not a pharmaceutical, being the four pharmaceuticals belonging to the classes of steroidal (DXM) and non-steroidal (NPX) anti-inflammatories, antihypertensive (LST) and antihistamine (LRT).
The concentrations of the 5 microppolutants mostly detected in the different collection points in the WTP are presented in Table 5. It is seen that dexametazone and naproxen occurred in higher concentrations in RW during the dry season when compared to the rainy season, while for the other compounds the differences observed were not statistically significant.

TABLE 5. Values of median concentrations of the 5 micropollutants most frequently detected in the dry (_D) and rainy (_R) periods in raw water (RW), filtered water (FW) and drinking water (DW) of WTP-Jataizinho.
Table 6 depicts endocrine disruptors and pharmaceuticals that have been quantified in at least 30% of the filter washing water (FWW) samples over the 13 sample campaigns. Out of the 8 compounds meeting this criterion, four of them (4-octylphenol, estrone, diclofenac and dexamethasone) occurred in the FWW at a median concentration equal to half the limit of detection (criterion normally used to replace < LOD by numerical values) of the analytical method. The BPA and naproxen compounds were found in the FWW in median concentrations higher than those observed in DW, while for loratadine and losartan compounds no significant differences in their median concentrations were observed in FWW and DW.
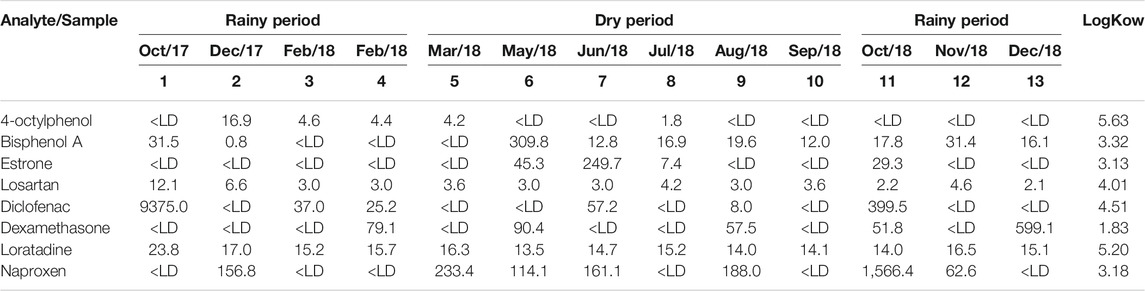
TABLE 6. Values of concentrations (ng L−1) of pharmaceuticals and endocrine disruptors which occurred in the filter washing water (FWW) of WTP-Jataizinho with a minimum frequency of 30%.
Discussion
Micropollutants in Raw Water
During the dry period (March to September), 38 occurrences of micropollutants with concentration values above limit of detection (LOD) or limit of quantification (LOQ) were identified (6.3 per collection), while during the rainy period there were 36 occurrences (5.1 per collection). Analysis of data of the 3 compounds occurring with high frequency in the water of the Tibagi River indicated that there were no significant differences between the average concentrations calculated for the rainy and dry periods for the BPA (dry: 18.6 ng L−1; rainy: 17.3 ng L−1) and losartan compounds (dry: 3.3 ng L−1; rainy: 4.8 ng L−1), whereas for dexamethasone compound (dry: 598.4 ng L−1; rainy: 182.6 ng L−1) the median concentration observed in the dry period was 3.3 times higher than that observed in the rainy period. This may suggest that the main source of such a corticoid input to the Tibagi River is the discharging of domestic sewage, therefore being more affected by the dilution factor caused by the increased river flowrate during the rainy season. Although sewage is more diluted in the rainy season, increased runoff can also contribute to diffuse pollution of the water body by carrying solid material containing micropollutants, especially in livestock areas (e.g. veterinary drugs) and urban solid waste disposal (e.g. micropollutants leached from packaging). Nevertheless, water quality monitoring data collected at the Chácara Ana Cláudia station, conducted by the Paraná Water Institute (AGUASPARANÁ), indicated stable WQI values in the range of 52–69 throughout the monitoring period carried out on the Tibagi River.
As noted in Table 4 three to seven pharmaceuticals and/or endocrine disruptors were detected in the samples collected over the 13 sample campaigns performed. One can notice that the highest numbers of microcontaminants (6 and 7) were detected during the dry and rainy periods, both in samples with high and low turbidity levels or with high or low dissolved oxygen content, thus indicating no apparent correlation between the frequency of detection of microcontaminants and the usual water quality parameters. Multivariate analysis of these data was carried out in order to verify if there was any correlation between the characterization parameters and the pharmaceutical drugs and endocrine disruptors monitored in this work. Figure 2 shows the results of the multivariate analysis corresponding to the monitoring data of microcontaminants and the usual parameters of characterization in RW.
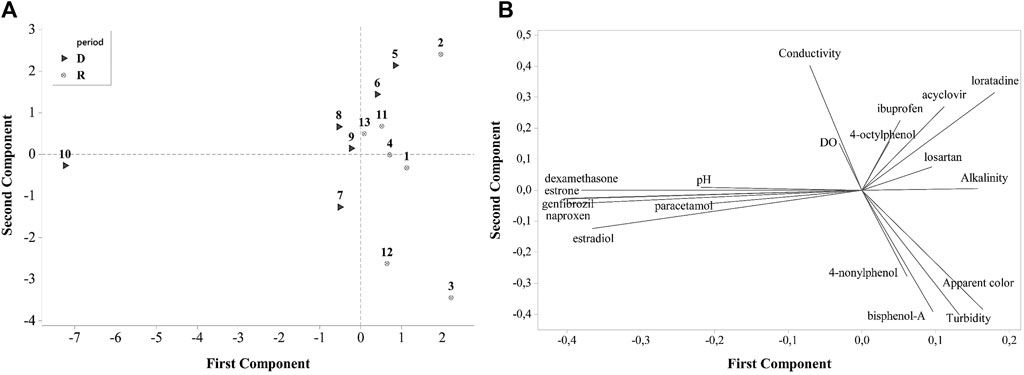
FIGURE 2. Analysis of principal components (PCA) of the contaminants present in the WTP-Jataizinho: (A) scores plot, (B) loading plot.
Figure 2Ashows that RW samples are not distinguished according to collection periods, although there was a remarkable pluviometric difference between the “dry” and “rainy” periods, as seen in Supplementary Figure S1. The sample collected in the 10th campaign during the dry season stands out from the others probably due to the high concentrations of estrone, dexamethasone and naproxene observed (see Supplementary Table S1). Samples collected in the 3rd and 12th campaigns in the rainy season are also out of cluster probably due to their high apparent color and low dissolved oxygen concentrations (Table 4).
The analysis of the score graph (Figure 2B) indicates a large dispersion of the micropollutants monitored and low correlation with the water quality indicator parameters which were widespread in the four quadrants of the PCA graph. However, the formation of a cluster including the parameters turbidity, apparent color, bisphenol A and 4-octylphenol was clearly noted. These compounds are well-known endocrine disruptors of hydrophobic nature which tend to adsorb onto suspended organic matter that causes turbidity and apparent color. It was also noted that, overall, there was no significant apparent correlation between the parameters dissolved oxygen, conductivity and alkalinity, and the micropollutants monitored. For the parameter pH it was observed that it tended to cluster with six micropollutants of which four of them (DMX, E1, E2, PCT) have pKa values higher than 9. In other words, the pH tended to cluster with compounds which exhibited no charge at neutral pH, except for NPX and GEN which are acidic compounds.
Micropollutants in Water Treatment Plant
Comparison of the concentration values of pharmaceuticals and endocrine disruptors, during dry and rainy periods, along the treatment stages used in WTP-Jataizinho were evaluated by the medians, as shown in Table 5. Figure 3 presents the changes in concentration along the treatment of the most prevalent compounds in WTP-Jataizinho, disregarding the separation between the dry and rainy periods.
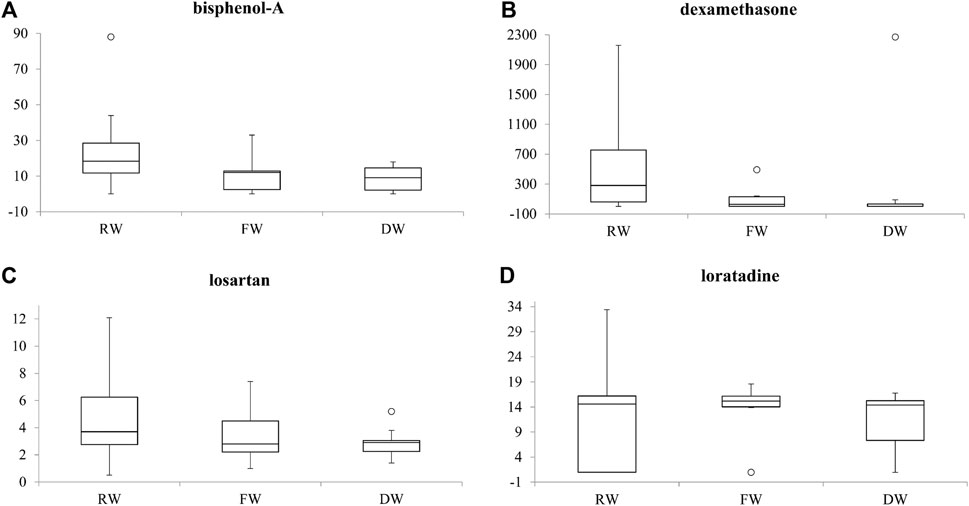
FIGURE 3. Changes in concentration (ng∙L−1) of the compounds most frequently detected in raw water at the WTP-Jataizinho: (A) bisphenol-A, (B) dexamethasone, (C) losartan and (D) loratadine.
It was verified that the micropollutants removed with greater efficiency in WTP-Jataizinho were dexamethasone (∼99% in dry or rainy season) and BPA (46% in dry season and 48% in rainy season), whereas the antiallergic loratadine was not removed significantly in any of the two seasons (3.4% in dry and −4.8% in rainy season). In turn, the antihypertensive losartan was moderately removed only in the rainy season (9% dry and 46% rainy), while the anti-inflammatory naproxen was only moderately removed in the dry season (56.9% dry and 0% rainy). The removal of pharmaceuticals was mainly in the clarification stage, and chlorination was only important for dexamethasone removal in the rainy period as in this period the median concentration of such corticoid in the sand filters effluent still accounted for 30% that was found in RW (Table 6). It is important to highlight that a simple change in the structure of a given target compound would make its detection unfeasible in the developed method. Thus, the removal of a given microcontaminant was evaluated based on the reduction in the concentration of the target compound, disregarding the possible formation of related compounds.
The high removal of dexamethasone at the clarification stage is somewhat surprising since such compound has moderate Kow and Koc values and, therefore, is considered moderately mobile, with greater affinity for water than for solids suspended therein. On the other hand, the removal in the clarification stage of about 35% of the BPA present in RW is consistent with its greater hydrophobicity and tendency to adhere to the flocs formed in the flocculator and retained in the decanter and filters of WTP-Jataizinho.
In general, it was observed that the more hydrophobic compounds (log Kow > 3) were present in the FWW, although some of them were not found with high frequency or concentration in RW or DW (used for filter washing). This indicates that such compounds were probably removed preferably via adsorption to the flocs retained in the decanter and filter material. The presence of these compounds in the FWW possibly results from the desorption process due to the equilibrium displacement promoted by the use, in the washing, of water containing lower concentration of hydrophobic contaminants retained in the flocs. The exception was dexamethasone (log Kow = 1.83), which was repeatedly detected in the filter washing water and cannot be considered hydrophobic. In the specific case of dexamethasone, the presence in the FWW probably arises from its frequent presence in the DW which was used to wash the filters.
Huerta-Fontela et al. (2011) evaluated the removal of 55 drugs in a WTP comprising primary chlorination, clarification, sand filtration, ozonation, activated carbon filtration and post chlorination. Thirty-five contaminants were identified in the supply source and only 5 of these were completely removed at the clarification stage. The removal efficiency was less than 30% for most of the compounds. Regarding chlorination, high removals were obtained for beta-blockers and estrogen hormones, whereas only 5% of atenolol was removed. In the treated water, only 5 drugs were detected, the efficiency being attributed to chlorination and ozonation, while filtration with activated carbon was important in the removal of hydrophobic compounds. On the other hand, Nam et al. (2017) found that chlorination did not remove caffeine, carbamazepine, metoprolol and sulfamethoxazole, which are substances frequently found in surface waters. In fact, the removal efficiency of endocrine disruptors and pharmaceuticals varies widely depending on the different oxidation processes employed. As an example, chlorination was highly efficient in removing estriol, triclosan and estrone, although it was ineffective in removing ibuprofen and iopromide (Wu et al., 2012). Similarly, Hu et al. (2017) investigated 25 drugs in supply waters, and observed removals in the range of 16.7–100% in the WTP which included ozonation and activated carbon and 2.9–100% in conventional station. In general, the WTP with advanced treatment processes, including ozonation and filtration of granular activated carbon presented better performance than the station with conventional treatment processes, except for sulfonamides. Indeed, according to Arnold et al. (2013), water treatment technologies involving clarification, chlorination and activated carbon usually remove between 76 and 99% of bisphenol A present in RW. In another study, conventional DW treatment processes achieved low removal efficiencies for pharmaceutical products such as antibiotics and beta-blockers (Kim et al., 2020), while advanced treatment processes provided higher removal efficiencies (Yang et al., 2017).
Risk Analysis
Considering the 5 contaminants (BPA, LST, DXM, LRT and NPX) that occurred most frequently in the chlorinated water (DW) distributed by WTP-Jataizinho (see Table 5), a risk analysis was performed by calculating the MOE. As previously described, MOE was estimated by the ratio of the guide value (calculated from epidemiological and/or toxicological information) to the concentration of the contaminant in the distributed water (Eq. 3). The guide value was estimated using information reported in Brandt et al. (2019) that compiled TDI values for several pharmaceuticals and endocrine disruptors. Regarding the compounds of interest in this study, the lowest TDI values (in μg kg−1 d−1) reported by Brandt et al. (2019) are the following: DXM–0.008; LRT–0.17; LST–0.42; BPA–4; NPX–7.3. Using such values in Eq. 4 and considering allocation factors (AF) values equal to 0.6 for BPA (EFSA, 2015); 0.1 for DXM (used in human and veterinary medicine); and 1.0 for NPX, LRT and LST (used in human medicine only), according to the EPHC NHMRC NRMMC (2008) approach, guideline values (GV) for such micropollutants were estimated at 0.025 μg L−1 (25 ng L−1) for DXM; 5.1 μg L−1 (5,100 ng L−1) for LRT; 12.6 μg L−1 (12,600 ng L−1) for LST; 72 μg L−1 (72,000 ng L−1) for BPA and 219 μg L−1 (219,000 ng L−1) for NPX.
Utilizing the calculated GV and considering the 95th percentile values of LRT, LST, BPA, NPX and DXM concentration in the DW of WTP-Jataizinho, estimated at 16.3 ng L−1; 4.4 ng L−1; 17.8 ng L−1; 1,631 ng L−1 and 962 ng L−1, respectively, Eq. 3 provides the following MOE values: 312.8 for LRT; 2,863.6 for LST; 4044.9 for BPA; 0.025 for DXM and 134.3 for NPX. These results indicated that the concentration of pharmaceuticals and endocrine disruptors in the treated water of WTP-Jataizinho is approximately 130 to 4,000 times lower than the concentration which would cause some adverse effect, except for DXM. In the case of DXM, its high concentration in chlorinated water was due to the high concentration observed during the 5th collection campaign, which is probably an isolated event. The median DXM concentration in the water distributed by WTP-Jataizinho, considering all data (rainy and dry seasons) was estimated at 1.40 ng L−1, which is equivalent to half the quantification limits for this compound. In other words, 50% of the DXM values in the treated water from WTP-Jataizinho were below the limit of quantification of the analytical method used. Thus, considering the median concentration in Eq. 3, the margin of exposure to DXM would be 17, thereby indicating that the calculated risk is low, as observed for the other micropollutants.
This approach has assumed, in addition to the conservative 95th percentile of monitoring data (OC), uncertainty factors (UFs) of at least 1,000 (10 for differences in response among humans, including sensitive individuals (intra-species variation); 10 for the protection of sensitive subgroups, including children and infants (inter-species variation); 10 for the fact that the lowest daily therapeutic dose is not an effect-free level (EPHC NHMRC NRMMC, 2008) for the calculation of TDI (see Eq. 5), hence, risk analysis performed here was quite conservative. Therefore, it can be affirmed that the risk to human health would be negligible for the most prevalent pharmaceuticals and endocrine disruptors in the water treated by WTP-Jataizinho and future works should pay attention on the occurrence of dexametazone which, despite being efficiently removed by conventional treatment might exhibit adverse effects at concentrations as low as 25 ng L−1.
Conclusion
The monitoring of 25 pharmaceuticals and endocrine disruptors carried out in the Tibagi River (Paraná/Brazil) over 13 sample campaigns comprising dry and rainy periods indicated that 7 micropollutants were not detected in any sample analyzed. Only 3 compounds (BPA-bisphenol A, DXM–dexamethasone and LST–losartan) were detected with a high frequency (> 10/13) in the RW, at median concentrations ranging from 17.3 ng L−1 (rainy) to 18.6 ng L−1 (dry) for BPA; 182.6 ng L−1 (rainy) to 598.4 ng L−1 (dry) for DXM; and 3.2 ng L−1 (dry) to 4.8 ng L−1 (rainy) for LST. There was no significant correlation between the micropollutants monitored and the usual water quality parameters although it was observed that the endocrine disrupters BPA and 4-nonylphenol (4NP) followed the behavior of turbidity and apparent color.
The conventional treatment process (coagulation/flocculation/settling/sand filtration/chlorination) used on WTP-Jataizinho was very efficient in removing DXM (∼99%), moderately efficient in removing BPA (∼47%) and not very efficient for LST removal (∼22%). Despite the persistent presence of 5 microcontaminants (BPA, DXM, LRT, LST, and NPX) in the treated water distributed to the population, the risk analysis carried out indicated that they occurred in concentrations (95th percentile) about 130–4,000 times lower than that which would cause some adverse effect, thus evidencing low risk of human exposure to such contaminants by the consumption of treated water.
Data Availability Statement
The original contributions presented in the study are included in the article/Supplementary Material, further inquiries can be directed to the corresponding author.
Author Contributions
All authors listed have made a substantial, direct, and intellectual contribution to the work and approved it for publication.
Conflict of Interest
The authors declare that the research was conducted in the absence of any commercial or financial relationships that could be construed as a potential conflict of interest.
Publisher’s Note
All claims expressed in this article are solely those of the authors and do not necessarily represent those of their affiliated organizations, or those of the publisher, the editors and the reviewers. Any product that may be evaluated in this article, or claim that may be made by its manufacturer, is not guaranteed or endorsed by the publisher.
Acknowledgments
The authors would like to thank the following agencies for their financial support: Coordenação de Aperfeiçoamento de Pessoal de Nível Superior (CAPES), Fundação de Amparo à Pesquisa do Estado de Minas Gerais (FAPEMIG), Conselho Nacional de Desenvolvimento Científico e Tecnológico (CNPq), Fundação Nacional de Saúde (FUNASA), Universidade Federal de Ouro Preto (UFOP) and Universidade Tecnológica Federal do Paraná (UTFPR).
Supplementary Material
The Supplementary Material for this article can be found online at: https://www.frontiersin.org/articles/10.3389/fenvs.2021.715772/full#supplementary-material
References
Archer, E., Petrie, B., Kasprzyk-Hordern, B., and Wolfaardt, G. M. (2017). The Fate of Pharmaceuticals and Personal Care Products (PPCPs), Endocrine Disrupting Contaminants (EDCs), Metabolites and Illicit Drugs in a WWTW and Environmental Waters. Chemosphere 174, 437–446. doi:10.1016/j.chemosphere.2017.01.101
Arnold, S. M., Clark, K. E., Staples, C. A., Klecka, G. M., Dimond, S. S., Caspers, N., et al. (2013). Relevance of Drinking Water as a Source of Human Exposure to Bisphenol A. J. Expo. Sci. Environ. Epidemiol. 23, 137–144. doi:10.1038/jes.2012.66
aus der Beek, T., Weber, F.-A., Bergmann, A., Hickmann, S., Ebert, I., Hein, A., et al. (2016). Pharmaceuticals in the Environment-Global Occurrences and Perspectives. Environ. Toxicol. Chem. 35, 823–835. doi:10.1002/etc.3339
Bexfield, L. M., Toccalino, P. L., Belitz, K., Foreman, W. T., and Furlong, E. T. (2019). Hormones and Pharmaceuticals in Groundwater Used as a Source of Drinking Water across the United States. Environ. Sci. Technol. 53, 2950–2960. doi:10.1021/acs.est.8b05592
Brandt, E. M. F., Aquino, S. F., and Bastos, R. K. X. (2019). Substâncias Químicas: Fármacos e Desreguladores Endócrinos. Subsídios para Discussão e Orientações para Revisão do Anexo XX da Portaria de Consolidação n. 5 de 2017. Relatório apresentado ao Grupo de Trabalho do Tema II. Brasil: Ministério da Saúde do Governo do Brasil.
Brozinski, J.-M., Lahti, M., Meierjohann, A., Oikari, A., and Kronberg, L. (2013). The Anti-inflammatory Drugs Diclofenac, Naproxen and Ibuprofen Are Found in the Bile of Wild Fish Caught Downstream of a Wastewater Treatment Plant. Environ. Sci. Technol. 47, 342–348. doi:10.1021/es303013j
Burns, E. E., Carter, L. J., Kolpin, D. W., Thomas-Oates, J., and Boxall, A. B. A. (2018). Temporal and Spatial Variation in Pharmaceutical Concentrations in an Urban River System. Water Res. 137, 72–85. doi:10.1016/j.watres.2018.02.066
Buszka, P. M., Yeskis, D. J., Kolpin, D. W., Furlong, E. T., Zaugg, S. D., and Meyer, M. T. (2009). Waste-indicator and Pharmaceutical Compounds in Landfill-Leachate-Affected Ground Water Near Elkhart, Indiana, 2000-2002. Bull. Environ. Contam. Toxicol. 82, 653–659. doi:10.1007/s00128-009-9702-z
Carmona, E., Andreu, V., and Picó, Y. (2014). Occurrence of Acidic Pharmaceuticals and Personal Care Products in Turia River Basin: From Waste to Drinking Water. Sci. Total Environ. 484, 53–63. doi:10.1016/j.scitotenv.2014.02.085
Corrêa, J. M. M., Sanson, A. L., Machado, C. F., Aquino, S. F., and Afonso, R. J. C. F. (2021). Occurrence of Contaminants of Emerging Concern in Surface Waters from Paraopeba River Basin in Brazil: Seasonal Changes and Risk Assessment. Environ. Sci. Pollut. Res. 28, 30242–30254. doi:10.1007/s11356-021-12787-z
de Jesus Gaffney, V., Almeida, C. M. M., Rodrigues, A., Ferreira, E., Benoliel, M. J., and Cardoso, V. V. (2015). Occurrence of Pharmaceuticals in a Water Supply System and Related Human Health Risk Assessment. Water Res. 72, 199–208. doi:10.1016/j.watres.2014.10.027
EPHC NHMRC NRMMC, (2008). Australian Guidelines for Water Recycling. Managing Health and Environmental Risks (Phase 2). Canberra: Augmentation of Drinking Water Supplies.
Focazio, M. J., Kolpin, D. W., Barnes, K. K., Furlong, E. T., Meyer, M. T., Zaugg, S. D., et al. (2008). A National Reconnaissance for Pharmaceuticals and Other Organic Wastewater Contaminants in the United States - II) Untreated Drinking Water Sources. Sci. Total Environ. 402, 201–216. doi:10.1016/j.scitotenv.2008.02.021
Gothwal, R., and Shashidhar, T. (2015). Antibiotic Pollution in the Environment: A Review. Clean. Soil Air Water 43, 479–489. doi:10.1002/clen.201300989
Hu, X.-L., Bao, Y.-F., Hu, J.-J., Liu, Y.-Y., and Yin, D.-Q. (2017). Occurrence of 25 Pharmaceuticals in Taihu Lake and Their Removal from Two Urban Drinking Water Treatment Plants and a Constructed Wetland. Environ. Sci. Pollut. Res. 24, 14889–14902. doi:10.1007/s11356-017-8830-y
Huerta-Fontela, M., Galceran, M. T., and Ventura, F. (2011). Occurrence and Removal of Pharmaceuticals and Hormones through Drinking Water Treatment. Water Res. 45, 1432–1442. doi:10.1016/j.watres.2010.10.036
IAPAR-EMATER (2018). Instituto de Desenvolvimento Rural Do Paraná - IAPAR. Available at: http://www.iapar.br/modules/conteudo/conteudo.php?conteudo=1828. (Accessed October 6, 2020).
IPARDES (2018). Instituto Paranaense de Desenvolvimento Econômico e Social - Secretaria Do Planejamento e Projetos Estruturantes Do Governo Do Estado Do Paraná. Available at: http://www.ipardes.pr.gov.br.
Jjemba, P. K. (2006). Excretion and Ecotoxicity of Pharmaceutical and Personal Care Products in the Environment. Ecotoxicology Environ. Saf. 63, 113–130. doi:10.1016/j.ecoenv.2004.11.011
Kim, K. Y., Ekpe, O. D., Lee, H.-J., and Oh, J.-E. (2020). Perfluoroalkyl Substances and Pharmaceuticals Removal in Full-Scale Drinking Water Treatment Plants. J. Hazard. Mater. 400, 123235. doi:10.1016/j.jhazmat.2020.123235
Küster, A., and Adler, N. (2014). Pharmaceuticals in the Environment: Scientific Evidence of Risks and its Regulation. Phil. Trans. R. Soc. B 369, 20130587. doi:10.1098/rstb.2013.0587
Lees, K., Fitzsimons, M., Snape, J., Tappin, A., and Comber, S. (2016). Pharmaceuticals in Soils of Lower Income Countries: Physico-Chemical Fate and Risks from Wastewater Irrigation. Environ. Int. 94, 712–723. doi:10.1016/j.envint.2016.06.018
Li, Q., Wang, P., Chen, L., Gao, H., and Wu, L. (2016). Acute Toxicity and Histopathological Effects of Naproxen in Zebrafish (Danio rerio) Early Life Stages. Environ. Sci. Pollut. Res. 23, 18832–18841. doi:10.1007/s11356-016-7092-4
Machado, K. C., Grassi, M. T., Vidal, C., Pescara, I. C., Jardim, W. F., Fernandes, A. N., et al. (2016). A Preliminary Nationwide Survey of the Presence of Emerging Contaminants in Drinking and Source Waters in Brazil. Sci. Total Environ. 572, 138–146. doi:10.1016/j.scitotenv.2016.07.210
Meffe, R., and de Bustamante, I. (2014). Emerging Organic Contaminants in Surface Water and Groundwater: A First Overview of the Situation in Italy. Sci. Total Environ. 481, 280–295. doi:10.1016/j.scitotenv.2014.02.053
Nam, S.-W., Yoon, Y., Chae, S., Kang, J.-H., and Zoh, K.-D. (2017). Removal of Selected Micropollutants during Conventional and Advanced Water Treatment Processes. Environ. Eng. Sci. 34, 752–761. doi:10.1089/ees.2016.0447
Nikolaou, A., Meric, S., and Fatta, D. (2007). Occurrence Patterns of Pharmaceuticals in Water and Wastewater Environments. Anal. Bioanal. Chem. 387, 1225–1234. doi:10.1007/s00216-006-1035-8
Paíga, P., Santos, L. H. M. L. M., Ramos, S., Jorge, S., Silva, J. G., and Delerue-Matos, C. (2016). Presence of Pharmaceuticals in the Lis River (Portugal): Sources, Fate and Seasonal Variation. Sci. Total Environ. 573, 164–177. doi:10.1016/j.scitotenv.2016.08.089
Patel, M., Kumar, R., Kishor, K., Mlsna, T., Pittman, C. U., and Mohan, D. (2019). Pharmaceuticals of Emerging Concern in Aquatic Systems: Chemistry, Occurrence, Effects, and Removal Methods. Chem. Rev. 119, 3510–3673. doi:10.1021/acs.chemrev.8b00299
Rand-Weaver, M., Margiotta-Casaluci, L., Patel, A., Panter, G. H., Owen, S. F., and Sumpter, J. P. (2013). The Read-Across Hypothesis and Environmental Risk Assessment of Pharmaceuticals. Environ. Sci. Technol. 47, 11384–11395. doi:10.1021/es402065a
Reis, E. O., Foureaux, A. F. S., Rodrigues, J. S., Moreira, V. R., Lebron, Y. A. R., Santos, L. V. S., et al. (2019). Occurrence, Removal and Seasonal Variation of Pharmaceuticals in Brasilian Drinking Water Treatment Plants. Environ. Pollut. 250, 773–781. doi:10.1016/j.envpol.2019.04.102
Sanson, A. L., Baêta, B. E. L., Rodrigues, K. L. T., and Afonso, R. J. C. F. (2014). Equipamento de baixo custo para extração em fase sólida em amostras aquosas de grande volume utilizando pressão positiva de N. Quím. Nova 37, 150–152. doi:10.1590/S0100-40422014000100024
Sanson, A. L. (2012). Estudo da Extração e Desenvolvimento de Metodologia para Determinação Simultânea de Microcontaminantes Orgânicos em Água Superficial por GC-MS e Métodos Quimiométricos. Ouro Preto: Universidade Federal de Ouro Preto.
Santos, A. V., Couto, C. F., Lebron, Y. A. R., Moreira, V. R., Foureaux, A. F. S., Reis, E. O., et al. (2020). Occurrence and Risk Assessment of Pharmaceutically Active Compounds in Water Supply Systems in Brazil. Sci. Total Environ. 746, 141011. doi:10.1016/j.scitotenv.2020.141011
Sema, (2015). Secretaria de Estado Do Meio Ambiente e Recursos Hídricos. Bacias Hidrográficas Do Paraná – Série Histórica. Available at: https://www.paranagua.pr.gov.br/imgbank2/file/meio_ambiente/material-didatico/Revista_Bacias_Hidrograficas_2015.pdf. (Accessed July 17, 2021).
Stackelberg, P. E., Gibs, J., Furlong, E. T., Meyer, M. T., Zaugg, S. D., and Lippincott, R. L. (2007). Efficiency of Conventional Drinking-Water-Treatment Processes in Removal of Pharmaceuticals and Other Organic Compounds. Sci. Total Environ. 377, 255–272. doi:10.1016/j.scitotenv.2007.01.095
Tran, N. H., Reinhard, M., and Gin, K. Y.-H. (2018). Occurrence and Fate of Emerging Contaminants in Municipal Wastewater Treatment Plants from Different Geographical Regions-A Review. Water Res. 133, 182–207. doi:10.1016/j.watres.2017.12.029
USEPA (2016). Contaminant Candidate List (CCL) and Regulatory Determination. Washington: United States Environmental Protection Agency.
Vieno, N., Tuhkanen, T., and Kronberg, L. (2006). Removal of Pharmaceuticals in Drinking Water Treatment: Effect of Chemical Coagulation. Environ. Techn. 27, 183–192. doi:10.1080/09593332708618632
Watkinson, A. J., Murby, E. J., Kolpin, D. W., and Costanzo, S. D. (2009). The Occurrence of Antibiotics in an Urban Watershed: From Wastewater to Drinking Water. Sci. Total Environ. 407, 2711–2723. doi:10.1016/j.scitotenv.2008.11.059
World Health Organization (2011). Guidelines for Drinking-Water Quality. 4th ed. Switzerland: WHO Graphics.
Wu, Q., Shi, H., Adams, C. D., Timmons, T., and Ma, Y. (2012). Oxidative Removal of Selected Endocrine-Disruptors and Pharmaceuticals in Drinking Water Treatment Systems, and Identification of Degradation Products of Triclosan. Sci. Total Environ. 439, 18–25. doi:10.1016/j.scitotenv.2012.08.090
Xu, C., Niu, L., Guo, H., Sun, X., Chen, L., Tu, W., et al. (2019). Long-term Exposure to the Non-steroidal Anti-inflammatory Drug (NSAID) Naproxen Causes Thyroid Disruption in Zebrafish at Environmentally Relevant Concentrations. Sci. Total Environ. 676, 387–395. doi:10.1016/j.scitotenv.2019.04.323
Keywords: endocrine disruptors, pharmaceuticals, water treatment, risk assessment, micropollutants
Citation: Fazolo A, Batista LFA, Nonaka FM, Sanson AL, Alves MCP, Afonso RJdCF and Aquino SFd (2021) Assessment of Conventional Full-Scale Treatment for the Removal of Endocrine Disruptors and Pharmaceuticals Present in the Tibagi River (Paraná State, Brazil). Front. Environ. Sci. 9:715772. doi: 10.3389/fenvs.2021.715772
Received: 27 May 2021; Accepted: 31 July 2021;
Published: 02 September 2021.
Edited by:
Henrietta Dulai, University of Hawaii at Manoa, United StatesReviewed by:
Mohamed Hassaan, National Institute of Oceanography and Fisheries (NIOF), EgyptNeyson Martins Mendonça, Federal University of Pará, Brazil
Copyright © 2021 Fazolo, Batista, Nonaka, Sanson, Alves, Afonso and Aquino. This is an open-access article distributed under the terms of the Creative Commons Attribution License (CC BY). The use, distribution or reproduction in other forums is permitted, provided the original author(s) and the copyright owner(s) are credited and that the original publication in this journal is cited, in accordance with accepted academic practice. No use, distribution or reproduction is permitted which does not comply with these terms.
*Correspondence: Ananda Lima Sanson, ananda_lima@ufop.edu.br