Current Knowledge and Future Challenges on Bacterial Degradation of the Highly Complex Petroleum Products Asphaltenes and Resins
- 1Department of Bioengineering, King Fahd University of Petroleum and Minerals, Dhahran, Saudi Arabia
- 2Interdisciplinary Research Center for Membranes and Water Security, Dhahran, Saudi Arabia
- 3Department of Chemistry, King Fahd University of Petroleum and Minerals, Dhahran, Saudi Arabia
- 4Interdisciplinary Research Center for Refining and Advanced Chemicals, Dhahran, Saudi Arabia
Petroleum products consist mainly of aliphatics, aromatics, asphaltenes and resins. After oil exploitation, the concentrations of asphaltenes and resins are high in oil reservoirs; however, they are also the petroleum pollutants most recalcitrant to degradation, leading to high oil viscosity. A sizable amount of work has been dedicated to understand the degradation mechanisms of aliphatics and aromatics; however, in comparison, little work has been carried out on asphaltene and resin degradation. This review discusses our current knowledge on the understanding of asphaltene and resin degradation. More specifically, it sheds light on work carried out to date on the degradation of these pollutants, and highlights the major gaps that limit our understanding of their degradation pathways. It also presents new potential research areas that can be explored to fill in these gaps.
1 Introduction
Crude oil exploration and exploitation remain core operations in world economic activity, and around 80 million barrels of oil are produced daily worldwide (Fakhru’l-Razi et al., 2009). As a result of oil exploration, extraction, transport and storage, contamination of the environment with petroleum products is common, including accidental oil spills (de la Huz et al., 2011; Othumpangat and Castranova, 2014; Chen et al., 2019). For instance, during extraction, it is estimated that 3 L of reservoir water (also known as produced water) is generated for every liter of oil or gas (Fakhru’l-Razi et al., 2009). Thus, with an average of 80 million barrels of oil exploited daily, around a quarter of a billion barrels of produced water is generated (Fakhru’l-Razi et al., 2009). Most of the produced water is reutilized in the reservoir to extract more oil; however, 5–10% is released into the environment, an equivalent of 100 million liters daily (Fakhru’l-Razi et al., 2009). This highlights the role of the oil industry in contamination of the environment by petroleum products, thus their removal from the environment remains a major priority.
Crude oil consists mainly of four fractions: saturates or aliphatics, aromatics, resins and asphaltenes. Their separation is based on exploitation of their differential solubilities in various solvents, a process known as saturate, aromatic, resin, and asphaltene (SARA) analysis, as shown in Figure 1A (Akmaz et al., 2011; Fakher et al., 2020). Saturates and aromatics are soluble in propane, unlike resins and asphaltenes. Thus, liquid propane can be used to separate saturates/aromatics from asphaltenes/resins.
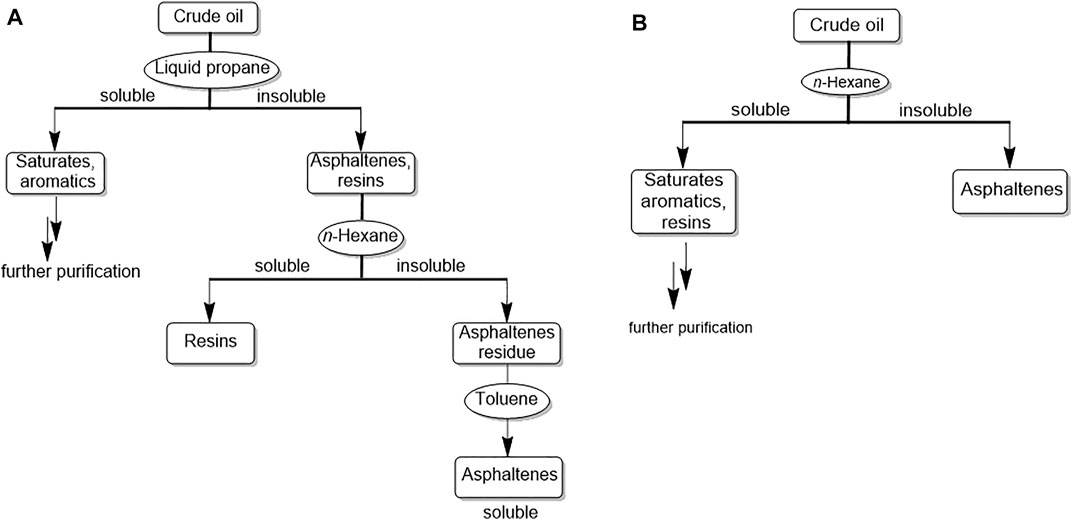
FIGURE 1. (A): Strategy for separation of oil components [saturates, aromatics, asphaltenes and resins (SARA)], adapted from Fakher et al., 2020 (B) Commonly used strategies for the separation of asphaltenes from the rest of the oil components, used in many publications discussed in this review.
Saturates (or alkanes) are aliphatic compounds that are generally in gas or liquid forms. Aromatics, on the one hand, consist of mono-aromatic hydrocarbons (MAHs), such as phenol and its derivatives, and on the other hand, they comprise polycyclic aromatic hydrocarbons (PAHs) with two or more rings, such as naphthalene [2 rings], phenanthrene [3 rings], pyrene [4 rings], and benzo [a]pyrene [5 rings].
Resins are complex compounds that have higher molecular masses than those of saturates and aromatics. They consist of aromatic and non-aromatic rings, small side chains of hydrocarbons, and sometimes one or more heteroatom(s) (Figure 2). The presence of heteroatoms favors their dissolution in polar solvents (e.g., methanol and dichloromethane). Resins play a significant role in stabilization of asphaltenes in crude oil (León et al., 2000). Asphaltenes are solid components of crude oil that have higher molecular weights than those of resins, with more aromatic and non-aromatic rings, associated with alkyl side chains of heteroatoms (Figure 3).
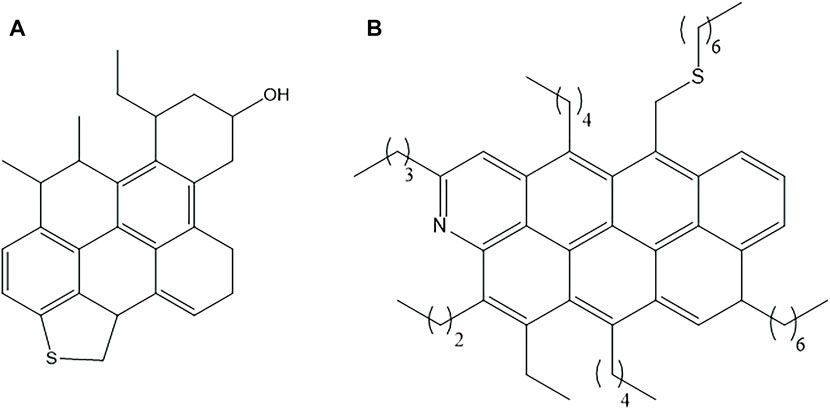
FIGURE 2. Proposed structures of resins, adapted from (A) Abdel-Raouf (2012); (B) Akmaz et al. (2011).
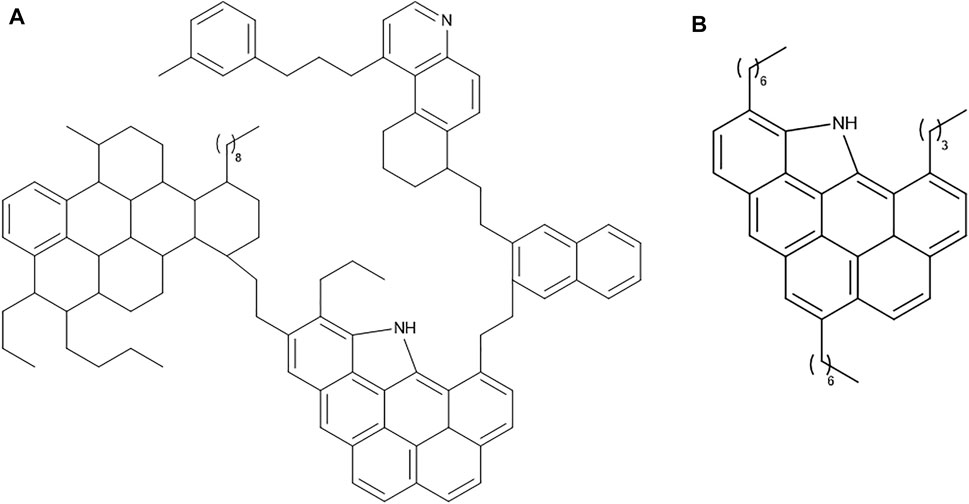
FIGURE 3. Proposed structures of asphaltenes, adapted from (A) Groenzin and Mullins (2000); (B) Kuznicki et al. (2008).
Asphaltenes and resins, which exhibit complex chemical compositions, have many proposed structures, and there is no report of a single purified asphaltene or resin compound that has been isolated or synthesized. So far, their chemical structures are only “proposed structures”, and there are as many “proposed structures” as the number of authors who have proposed them. Thus, they cannot be defined by specific chemical structures (Groenzin and Mullins, 2000; Kuznicki et al., 2008; Redelius, 2009; Akmaz et al., 2011; Abdel-Raouf, 2012; Fakher et al., 2020). The existence of pi-stacking, which results from the non-covalent interactions of phenyl rings, leading aggregate formation, is one of the main reasons for the difficulties in purifying various components of resins and asphaltenes. In addition, this tendency for aggregations varies from one solvent to the other, which complicates measurement of their molecular weights. Nevertheless, data indicate that the molecular weights of asphaltenes varies from 500 to >2000 g/ mol, and that of resins is 1,200–1700 g/ mol (Groenzin and Mullins, 2000; Speight, 2004)
These compounds are rather classified in terms of “solubility class”: asphaltenes are insoluble in n-alkanes but soluble in mono-aromatic solvents such as toluene and xylene; resins are insoluble in propane but soluble in n-hexane. Overall, asphaltenes are solid polar compounds and are the most complex oil components (Groenzin and Mullins, 2000; Kuznicki et al., 2008; Redelius, 2009; Akmaz et al., 2011; Abdel-Raouf, 2012; Fakher et al., 2020).
As shown in Figure 1A, the extraction of crude oil with propane leads to the separation of saturates/aromatics as the soluble fraction, and resins and asphaltenes as the insoluble fraction (SARA analysis). However, when crude oil is directly extracted with n-hexane, asphaltenes (insoluble fraction) are separated from the rest of the crude oil components (soluble fraction). This soluble fraction is known as maltene, and consists of saturates, aromatics and resins (Redelius, 2009), Figure 1B.
Crude oil also produces an important contaminant known as asphalt, which is derived from the non-destructive distillation of crude oil during petroleum refining. The oxidized form of this asphalt, which is obtained by blowing air through asphalt at elevated temperatures, produces asphalt that has interesting industrial applications, for instance in road paving and roofing. Outside the United States , asphalt is also referred to as bitumen, and a mixture of bitumen with mineral matter is referred to as asphalt (World Health Organization, 2004). Both asphalt and bitumen residues consist of the four components described above: saturates, aromatics, resins and asphaltenes (World Health Organization, 2004).
Several strategies have been developed to remove oil contaminants and these include chemical, physical and biological methods. The chemical and physical methods are expensive and generally produce secondary by-products as pollutants. Biological methods are the most attractive and are based on biodegradation, since they are more environmentally benign, and less costly. Biodegradation depends on exploitation of the ability of microorganisms to utilize pollutants as substrates for their growth, leading ultimately to their conversion to CO2. This concept has been explored and exploited in the removal of oil contaminants including aliphatics, MAHs and PAHs. Indeed, bioremediation or real life removal of oil pollutants by biodegradation has been carried out in various contaminated environments, including seawater, soil, groundwater (Farhadian et al., 2008; Scoma et al., 2016; Koshlaf and Ball, 2017; Davoodi et al., 2020; Mafiana et al., 2021; Sayed et al., 2021; Yap et al., 2021). In addition the mechanisms of degradation of aliphatics, MAHs and PAHs have been extensively studies and readers are referred to the following reviews on this topic (Haritash and Kaushik, 2009; Rojo, 2009; Seo et al., 2009; Meckenstock and Mouttaki, 2011; Nzila, 2018a, 2018b; Dhar et al., 2020; Nzila and Musa, 2020). Overall, aliphatics are easier to biodegrade, followed by MAHs, then PAHs, and within PAHs, microbial degradation performance decreases as the number of rings increase (Seo et al., 2009; Haritash and Kaushik, 2016).
The higher complexity of asphaltenes, and to a lesser extent resins, specifically make them the oil constituents most recalcitrant to biodegradation. So far, limited work has been dedicated to the understanding of the mechanisms of degradation of these recalcitrant components, when compared with those of saturates and aromatics. To fill this gap, work carried out to date on the biodegradation of asphaltenes and resins is presented in this review; challenges and gaps in our understanding of this degradation are summarized, and moreover, strategies to overcome these challenges are highlighted. New potential research avenues are also presented, for instance, in the Microbial Enhanced Oil Recovery (MEOR), an oil recovery approach that uses microorganisms to mobilize residual oil. Overall, this review provides new strategies that can be used to isolate microbes that can efficiently degrade these pollutants, and also improve our understanding of these microbes’ mechanisms of action.
2 Summary of Degradation Studies
2.1 Degradation Under Aerobic and Mesophilic Conditions
2.1.1 Asphaltene or Resin Degradation in the Context of Crude Oil as a Carbon Source
In this review, further reference details are listed in Supplementary Table S1 (ST1 to ST24) and Supplementary Table S2 (eST1-eST4). They will be referred to in the text by table entries (ST or eST).
In 1983, Bertrand et al. provided one of the first comprehensive reports on the bacterial degradation of asphaltenes from crude oil. In that work, the authors used a pre-isolated consortium of eight bacteria (E-8), and cultured them in a continuous bioreactor. Following filtration and weighing of the hexane-insoluble fraction of crude oil, which mainly corresponds to asphaltenes, the authors demonstrated that asphaltene content had been reduced by 74% (Bertrand et al., 1983; Supplementary Table S1, ST1). In another study using soil samples cultured in the presence of heavy oil, Minai-Tehrani et al. reported the degradation of asphaltenes and resins within a 12-month period (ST2); however, the degradation rate was relatively limited, less than 6%, especially in comparison with more than 42% for saturates and aromatics (Minai-Tehrani et al., 2015). The bacterial strain Pseudomonas aeruginosa was reported to degrade asphaltenes and resins in the presence of crude oil; however, only a 0.4% reduction in asphaltene was observed, while there was a c.10% reduction in aromatics (ST3). Interestingly, the reduction of asphaltene and aromatics was accompanied by an increase in saturates of around 10% (Etoumi et al., 2008), which could be explained by the degradation of aromatics and more complex compounds such as asphaltenes and resins to smaller moieties that exhibit characteristics similar to saturates.
2.1.2 Bitumen and Asphaltene Degradation in the Context of Using Bitumen as a Carbon Source
For many decades, there has been an interest in understanding the degradation of bitumen, partly because of its importance in the disposal of radioactive wastes. Since the 1950s, evidence for microbial degradation of this petroleum product has been generated; however, in these early studies, degradation was primarily monitored by the assessment of bacterial growth using bitumen as substrate (Drent, 1972). In addition to monitoring bacterial growth, and as proof of bacterial respiration, further studies have also monitored the generation of CO2 and biomethane under aerobic and anaerobic conditions, respectively (Brunner et al., 1987; Wolf and Bachofen, 1991b, 1991a; ST4-6).
As discussed earlier, bitumen consists of saturates, aromatics, resins and asphaltenes. Since saturates are the most amenable to biodegradation (this will be discussed in detail in Section 3), evidence of bacterial growth in the presence of bitumen does not necessarily imply that the recalcitrant asphaltenes and resins are also degraded. Thus, degradation of bitumen should be analyzed in the context of the degradation of each of its components, mainly the recalcitrant asphaltenes and resins. Such a study was reported by Ait-Langomazino et al., in which microbes consisting of the bacteria Pseudomonas aeruginosa, Pseudomonas putida, Arthrobacter sp., and Torulopsis holmii, as well as the yeast Saccharomycopsis lipolytica were shown to degrade bitumen. Overall, a 7–10% bitumen degradation was observed; detailed analysis indicated a degradation of saturates but not that of asphaltenes and resins, an indication of preferential degradation of saturates by these microbes (Ait-Langomazino et al., 1991; ST7). Morover, IR was used to confirm the appearance of bands that clearly indicate the presence of hydroxyl and carbonyl moities in the 3,640–3,100 cm−1 and 1720–1,690 cm−1 regions, respectively, upon degradation of bitumen using S. lipolytica. The degradation of bitumen has also been reported using a sediment slurryand monitored by CO2 production (respiration) and stable carbon isotopes. Evidence of bitumen degradation was reported, and this degradation was improved in the presence of an emulsifiying surfactant. However, no investigation was on the degradation of asphaltenes and resins (Lapham et al., 1999; ST8). In 2001, a report showed a degradation rate of up to 60% of bitumen within 120 days using sediment as inoculum. Fractionation experiments showed that most of the components from degradation of bitumen were saturates and aromatics; however, asphalatens and resins were not analyzed. Surprisingly, using molasses as part of the cometabolism to increase biodegradation yielded no benefit (Potter and Duval, 2001; ST9). This concept of metabolism will be discussed in Section 3.
In 2011, Olemebami et al. used an enrichment process and isolated five bacterial strains (Pseudomonas putrefaciens, Pseudomonas nigrificans, Bacillus licheniformis, Pseudomonas fragi, and Achromobacter aerogenes) that could grow in the presence of bitumen; their growth was associated with a decrease in the weight of bitumen, as measured by gravimetric analysis (Olabemiwo et al., 2011; ST10). Several years later, the same bacterial strains were shown to degrade the fraction of bitumen containing saturates and aromatics (Olabemiwo et al., 2014; ST11); however, in that study, the degradation of asphaltenes or resins was not reported, yet the authors concluded that these bacteria could be used to degrade bitumen. Bitumen degradation was also investigated in soil samples spiked with bitumen, using a preselected, but unidentified, bacterial consortium enriched in the presence of bitumen. In that study, degradation was ascertained by an increase in bacterial growth and the assessment of total carbon content. However, degradation of the recalcitrant asphaltenes or resins was not investigated in that study (Agarry and Oghenejoboh, 2014; ST12).
2.1.3 Asphaltene or Resin Degradation Using Asphalt as a Carbon Source
Two degradation studies have been carried out in the context of using asphalt as the carbon source. As stated earlier, the term asphalt is generally used interchangeably with bitumen, and consists of saturates, aromatics, resins and asphaltenes.
In 1998, Pendrys selected seven bacterial strains (belonging to the genera Pseudomonas, Acinetobacter, Alcaligenes, Flavimonas, and Flavobacterium) by an enrichment culture in the presence of asphalt (collected on the edge of an asphalt road). The authors demonstrated that each of these strains could grow in the presence of asphalt or its constituents of saturate, aromatic and asphaltene and resin fractions, as measured by bacterial counts (Pendrys, 1989; ST13). However, growth was more pronounced when using saturates and aromatics compared with asphaltenes and resins. These bacteria also produced biosurfactants, compounds that decrease the viscosity of recalcitrant pollutants, therefore promoting their biodegradation, as will be discussed in Section 3. Interestingly, that study indicated that active bacteria can be isolated from the asphalt of roads.
Two bacterial strains, Pseudomonas aeruginosa (Gx and Fx), have recently been proven to degrade “pure asphalt” (purchased from a commercial company) and an asphaltene fraction from heavy oil (Gao et al., 2017; ST14). In the same study, heavy crude oil was also used as a substrate. Around 10% of “pure asphalt” and 59–72% of crude oil asphaltene from heavy crude oil were degraded within 35 and 5 days, respectively (Gao et al., 2017). The use of these two substrates, “pure asphalt” and heavy crude oil, was associated with an increase in saturates and aromatics, as opposed to a decrease in asphaltenes, an indication that degradation of asphaltenes leads to aromatics or saturates. In addition, these two strains were shown to produce biosurfactants (Gao et al., 2017; ST14).
2.1.4 Asphaltene or Resin Degradation Using Asphaltenes as the Carbon Source
In the study ST1 (discussed in Section 2.1.1), a bacterial consortium EM-4 was used to investigate the degradation of asphaltenes using crude oil as a source of carbon, and, subsequently, Rontani et al. repeated the same experiment (with the same bacteria), but with asphaltenes as substrate and n-paraffins [(saturated hydrocarbons (C12-C18)] as co-substrate. The ability of this consortium to degrade the asphaltenic fraction was confirmed using mass spectrometry and nuclear magnetic resonance (NMR, both 1H and 13C), which showed a clear decrease in both aromaticity and length of side chains (Rontani et al., 1985; ST15). In another study, a strain of Pseudomonas sp. was shown to degrade up to 35% of 5,000 ppm resins within 15 days when cultured in a mineral medium using resins as the sole source of carbon (Venkateswaran et al., 1995; ST16).
Two bacterial strains, Bacillus sp. and Serratia liquesfaciens, isolated from oil contaminated soil, have also been reported to degrade asphaltenes, as measured by gravimetric methods (Rojas-Avelizapa et al., 2002; ST17).Similar results were reported using five bacterial strains, Pseudomonas spp. TMU2-5, Bacillus licheniformis TMU1-1, Bacillus lentus TMU5-2, Bacillus cereus TMU8-2, and Bacillus firmus TMU6-2 (Tavassoli et al., 2012; ST18). That study further showed a reduction in the proportion of elemental carbon, nitrogen and hydrogen after biodegradation. Another consortium of bacteria, consisting of Corynebacterium sp., Bacillus sp., Brevibacillus sp. and Staphylococcus sp. was shown to degrade asphaltenes when used as the sole source of carbon, as monitored by CO2 production (Pineda-Flores et al., 2004; ST19).
In another study, various bacterial consortia (Pseudomonas aeruginosa and Pseudomonas fluorescens; Citrobacter amalonaticus and Enterobactercloacae; Bacillus cereus and Lysinibacillus fusiformis), or the single bacterial strain Staphylococcus hominis, were proven to degrade asphaltenes, as measured by optical density, and changes in functional groups were observed using Fourier Transform Infrared (FT-IR) spectroscopy (Jahromi et al., 2014; ST20). The degradation of asphaltene has also been reported using Bacillus sp., Lysinibacillus fusiformes, Acinetobacter sp., and Mycobacterium sp. in the presence of asphaltenes and yeast extract (Aditiawati and Kamarisima, 2015; ST21).
Another study investigated the contribution of biosurfactant in biodegradation. Indeed, after enrichment of contaminated soil in the presence of asphaltenes, a strain of Bacillus cereus that produced biosurfactants and grew in the presence of asphaltenes as sole source of carbon was isolated. Further investigation showed that this strain could degrade up to 40% of 5.0 g.L−1 asphaltenes in 60 days (Asadollahi et al., 2016; ST22).
Likewise, the use of surfactant, sodium dodecyl benzene sulfonate, was associated with an increase in asphaltene degradation. Individual bacterial strains Enterobacter cloacae, Enterobacter cloacae, and Pseudomonas aeruginosacould degrade asphaltenes in mineral medium supplemented with yeast extract, and this degradation was augmented when the surfactant sodium dodecyl benzene sulfonate was added to the culture, a further illustration of the role of surfactants in the degradation of recalcitrant pollutants (Iraji and Ayatollahi, 2019; ST23).
Recently, the ability of various bacterial consortia to degrade asphaltenes was evaluated. Evidence of asphaltene degradation was provided using weight measurement, the assessment of elemental carbon, nitrogen, and hydrogen, and FT-IR spectrometry (Shahebrahimi et al., 2020; ST24).
2.2 Asphaltene or Resin Degradation Under Extremophilic Conditions
All studies reported above have been carried out under aerobic and mesophilic conditions. However, the ability of microorganisms to degrade oil products, including complex PAHs, has been documented in extreme conditions, which are conditions pertaining to the absence of oxygen (anaerobic), high temperature (thermophilic) and high salinity (halophilic) (Martins and Peixoto, 2012; Nzila, 2018b, 2018a). For instance, as discussed earlier, produced water, which results from oil exploitation is characterized by high salinity; likewise, high temperature, high salinity and also low or absence oxygen prevail in oil reservoirs. Thus, the application of biodegradation concept will require the use of extremophilic bacteria in these environments. A limited number of studies on the degradation of bitumen, asphaltenes, or resins have been performed under these conditions, and the results are summarized below and in Supplementary Table S2.
In 2009, a halophilic bacterial strain, TM-1, was isolated from a reservoir in an oil field, and was shown to degrade crude oil not only at high salinity (18% NaCl), but also at temperatures as high as 58°C. Specifically, when grown in the presence of heavy oil, this bacterium led to a reduction in the amount of resins and asphaltenes. However, in that study, the exact method of measurement of amounts of resins and asphaltenes was not provided (Hao and Lu, 2009; eST1).
The degradation of an oil asphaltenic fraction was also reported in three halotolerant bacteria, Bacillus sp. Asph1, Pseudomonas aeruginosa Asph2, and Micrococcus sp. that were isolated following enrichment experiments in the presence of the asphaltenic fraction. Using gas permeation chromatography, 83–96% of 2,500 mg L−1 asphaltene fraction was proven to be degraded within 21 days in the presence of 4.2% NaCl (g/v), and FT-IR analysis indicated an increase in hydroxyl, sulfoxide and sulfone functional groups (Ali et al., 2012; eST2).
The degradation of asphaltene and resin has also been tested under conditions of high temperature using a thermophilic bacterial strain TH-2, at 70°C; however, using crude oil as a source of carbon, the strain could only degrade 1–3% of asphaltenes and resins (Hao et al., 2004), eST3.
For the first time, Lavania et al. (Lavania et al., 2011) reported the degradation of the asphaltenic fraction of crude oil by a strain of Garciaella petrolearia (TERIG02) under anaerobic conditions that favored methanogenesis (CO2 as the main electron acceptor). Evidence of asphaltene degradation was provided, based on reduced viscosity and a change in functional groups (confirmed by FT-IR). Interestingly, H2 was produced, while biomethane was not (Lavania et al., 2011; eST4). The absence of biomethane was probably associated with the lack of methanogenic microorganisms in the culture medium.
3 General Findings and Further Perspectives
3.1 Mechanisms of Degradation of Asphaltenes and Resins
The general mechanisms of degradation of various saturates and aromatics have been proposed, and the literature is replete with reports on biochemical pathways for the degradation of various saturates and aromatics Haritash and Kaushik, 2009, 2016; Rojo, 2009; Seo et al., 2009; Nzila, 2018b; Nzila and Musa, 2020); however, virtually no information is available on the mechanisms of degradation of asphaltenes and resins.
Before discussing a possible mechanism of degradation of asphaltenes/resins, one needs to highlight the limitations that prevent the unraveling of these mechanisms. As discussed inSection 1, the structures of asphaltenes and resins are not well-defined. Rather, they occur as a mixture of various compounds that are characterized by high complexity, the presence of heteroatoms, and specific solubility patterns in n-alkanes and aromatic solvents; they also form nanoaggregates and aggregated clusters (Fakher et al., 2020). Generally, to decipher the mechanisms of biodegradation of a compound, one needs to subject a purified form of that compound to bacterial degradation, and identify its metabolites and enzymes that are involved in the degradation process; however, to date, there is no single study that has involved the use of a purified asphaltene or resin. Rather, all studies have used normal asphaltenes or resins, which are mixtures of many different compounds. Thus, under such circumstances, their mechanisms of degradation cannot be proposed.
However, based on the information that asphaltenes and resins consist of aliphatic moieties linked to aromatic and non-aromatic cyclic rings, sometimes with one or more heteroatoms, and our knowledge on the degradation pathways of saturates and aromatics, it is possible to propose a pathway for degradation of asphaltenes and resins, a concept that was initially proffered elsewhere (Pineda-Flores and Mesta-Howard, 2001).
First, in the order of biodegradation, alkanes (saturates) are easier to degrade than aromatics; these aromatics become more recalcitrant to degradation as the number of rings increase. Thus, it is conceivable that aliphatic moieties of asphaltenes and resins would be more amenable to degradation than aromatic or non-aromatic rings.
The degradation of saturates has been extensively studied under aerobic conditions. Saturates are not reactive compounds, and their degradation is initiated by the action of mono- or dioxygenases, enzymes that incorporate one or two oxygen atoms into the n-alkane chain, leading to the production of an alcohol through terminal or sub-terminal oxidation (Rojo, 2009). Further oxidation will generate intermediates of the Krebs cycle, such as ATP and CO2, for the production of energy through the central metabolism (Rojo, 2009).
The degradation of aromatic or non-aromatic rings is also initiated by their hydroxylation by mono- or di-oxygenases. Thereafter, a ring cleavage will take place to generate an aliphatic moiety, which generally has a terminal carboxylic group. For MAHs, the generated aliphatics join the Krebs cycle after further oxidation. In the case of PAHs, the ring opening process will continue in a stepwise fashion, until the last ring is opened, generating aliphatic intermediates that will eventually be transformed in the Krebs cycle, as in the case of MAHs. Intermediates from saturates or aromatics can present various functional groups, including carboxylic acid, aldehyde, ketone, or ether (Haritash and Kaushik, 2009, 2016; Rojo, 2009; Seo et al., 2009; Nzila, 2018b; Nzila and Musa, 2020).
The initial hydroxylation of aromatics and saturates is common in the biodegradation of these compounds under aerobic conditions. As proposed by Pineda-Flores and Mesta-Howard (Pineda-Flores and Mesta-Howard, 2001), it is conceivable that the aliphatic and aromatic moieties of asphaltenes and resins will be subjected to the same process of hydroxylation as the first step in the degradation pathway. Thus, mono- or dioxygenases should be key enzymes in the bacterial degradation of asphaltenes and resins. In support of this possibility, many studies on asphaltene degradation have involved the use of FT-IR spectrometry to monitor the functional groups of metabolites that result from degradation of asphaltenes. The results have shown that the degradation of asphaltenes is generally associated with the appearance of hydroxyl and carbonyl functional groups (ST10,18, eST2,4). Likewise, the oxidation of asphaltenes can also take place on the heteroatom, leading to a degradation process similar to that of the aromatic heterocyclic compounds (Pineda-Flores and Mesta-Howard, 2001).
In summary, the absence of a purified asphaltene or resin is a major limitation in studying the biodegradation pathways of these compounds; however, it is likely that mono- or di-oxygenases play an important role in the oxidation of these compounds before their degradation into small molecules. Discovering more strains that are able to efficiently degrade asphaltenes and resins is definitely important. However, efforts should be directed towards obtaining purified compounds of asphaltenes or resins with defined structures (either by extraction or synthesis), and then subject them to biodegradation. This will not only contribute to our understanding of the biodegradation mechanisms for these complex compounds, but also help in discovering new enzymes with unique functions.
3.2 Further Studies on Bitumen and Asphalt Biodegradation
As discussed earlier, generally both bitumen and asphalt are considered to be part of the complex petroleum products that are not amenable to degradation. Several studies have been reported on bacterial degradation of bitumen by monitoring bacterial growth, measuring the production of CO2, or monitoring the total carbon content or the change in bitumen/asphalt weight (ST4-12). The use of the term “bitumen biodegradation” is misleading in this context, since, in reality, bitumen is a mixture of saturates, aromatics, resins and asphaltenes. Thus, when exposed to bitumen, bacteria would degrade compounds that are more amenable to degradation, which are the saturates, followed by small aromatics. Therefore, evidence that a bacterium can grow in the presence of bitumen is not necessarily proof of the ability of this bacterium to degrade the most complex petroleum products (asphaltenes/resins). In support of this argument, studies on bitumen degradation, in which each bitumen component is assessed, clearly indicate that saturates and, to a lesser extent, aromatics are predominantly degraded compared to asphaltenes or resins (ST7,9).
The same observations have also been noted in the context of degradation of asphalt and crude oil. For instance, studies ST1, ST2, and ST13 showed that the degradation rate of saturates was higher in bioremediation of crude oil or asphalt. However, it is worth noting that, in two other studies, saturate levels were found to increase when crude oil was used as substrate (ST14, eST1). This could be due to an increase in the degradation of aromatics (mainly MAHs), which leads to ring opening and thus generation of saturates. Nevertheless, since saturates are more amenable to degradation, it is imperative to monitor the degradation of asphaltenes or resins, which are the components most recalcitrant to degradation, in biodegradation of crude oil, bitumen or asphalt.
3.3 Assessment of the Remaining Quantities of Asphaltenes/Resins Using Flash Chromatography Following Biodegradation
As discussed earlier, asphaltenes are separated from saturates, aromatics and resins by their insolubility in n-hexane. Resins and asphaltenes have heteroatoms, thus are more amenable to separation by flash chromatography, using polar solvents (such as methylene chloride or methanol) compared to the non- or less-polar saturates and aromatics. Thus, polar solvents have been employed in several studies to separate the polar (asphaltenes/resins) versus non-polar compounds, using flash chromatography (ST7, 9,11; ST16, eST4); however, this approach has limitations, especially when used to assess the remaining asphaltenes/resins in biodegradation. As discussed in Section 3.1, under aerobic conditions, the degradation of saturates and aromatics (also that of asphaltenes and resins) is initiated by the action of mono- or di-oxygenases, leading to mono- or di-hydroxyl metabolites, and these metabolites are converted further to carboxylic acid, aldehyde or ketone metabolites, which are also polar. Thus, when using polar solvents (in the context of flash chromatography) to assess the residual asphaltenes/resins after biodegradation, these metabolites will elute along with asphaltenes and resins, thus falsely increasing the amount of the remaining asphaltenes and resins. This shortfall makes the results emanating from this approach not to be accurate.
3.4 Cometabolism
The degradation of recalcitrant pollutants can be accelerated through a process known as cometabolism, which consists of using two substrates, the non-growth or recalcitrant substrate, and a growth substrate. This growth substrate is more amenable to degradation, thus is easily utilized to support bacterial growth and provide a source of energy. Thus, the presence of a growth substrate will spur the degradation of the non-growth substrate, and this concept of cometabolism has been evaluated in the degradation of various recalcitrant pollutants (Nzila, 2013).
When pollutants that consist of mixtures of various compounds are used for biodegradation, for instance, crude oil, bitumen and asphalt; this type of degradation can be referred to as cometabolism. Thus, as shown in Supplementary Table S1, in studies in which crude oil, bitumen and asphalt were used, it is possible to argue that the observed degradation of asphaltenes or resins is likely to have been contributed by saturates (present in these products) as growth substrates.
Likewise, cometabolism was also evaluated using asphaltenes (non-growth substrate) in the presence of the growth substrates paraffin (ST15), beef or yeast extract (ST17, 21, 23), or molasses (eST4). It is noteworthy that studies (ST14, ST9) that used asphalt and bitumen, which are mixtures of various compounds, also employed the growth substrates beef extract, peptone broth, or molasses.
Overall, it is important to note that, out of 28 studies reported in this review on the degradation of asphaltenes (and to a lesser extent resins), these compounds were used as the sole source of carbon in only six studies (ST16,18–20,22,24, eST2); this is probably due to the difficulties and challenges in the selection and isolation of bacteria that could degrade asphaltenes or resins when used as the sole source of carbon.
However, cometabolism can also be associated with drawbacks. For instance, the use of a growth substrate can inhibit the expression of enzymes involved in the degradation of the non-growth substrate, a phenomenon known as metabolite inhibition (Nzila, 2013). For instance, in the study in which molasses was used as a growth substrate, no increase in biodegradation of bitumen was observed (eST4). In all the other studies discussed in this review, no comparison was made on the rate of degradation in the presence versus absence of growth substrates, thus one cannot estimate the extent to which cometabolism increased the degradation rate of these petroleum pollutants. However, based on the benefit of this approach in previous work, one would expect that this approach would add value to the biodegradation process. Nevertheless, it is recommended that the effect of the addition of growth substrates be estimated before wide-scale use of this approach can be considered (Nzila, 2013).
3.5 Biomineralization of Asphaltenes
Under aerobic conditions, the complete microbial degradation of a pollutant would generate CO2 as the end product of the Krebs cycle, and this process is known as biomineralization, the ultimate objective of pollutant degradation. However, the generation of CO2 during biodegradation does not necessarily imply biomineralization. A bacterial strain can use part of the pollutant, and convert it to intermediate(s) in the Krebs cycle, which will generate CO2, while intermediates can remain and even accumulate to constitute secondary pollutants (Kiel and Engesser, 2015). In relation to asphaltene degradation (when used as the sole source of carbon), studies ST16,19 provide evidence of CO2 generation, and the authors claimed the occurrence of asphaltene biomineralization (ST19). This is arguable, since there is no evidence of conversion of all asphaltenes to CO2. Generally, mineralization is proven using radiolabeled carbon in a specific position in the pollutant (mainly carbon atoms at positions that are less amenable to biodegradation), and the detection of radiolabeled CO2 confirms the conversion of these carbons to CO2. For instance, biomineralization of the recalcitrant benzo [a]pyrene has been proven using this approach (Nzila and Musa, 2020). However, such evidence has not yet been provided with asphaltenes or resins, which is due to the complexity of their structures and the fact that most of the reported studies dealt with impure samples. As discussed earlier, it is possible that bacteria degrade the aliphatic parts of these compounds, leaving the most complex parts as intermediates. Thus, biomineralization of asphaltenes or resins still remains unresolved.
3.6 Degradation Using a Single Bacterial Strain or Bacterial Consortium
Pollutants in general, and recalcitrant pollutants in particular, are efficiently degraded by bacterial consortia rather than a single bacterial strain. A given bacterial strain needs to express a set of enzymes that are required for the biodegradation or biomineralization to take place. However, all these enzymes may not be present in a single bacterial strain, and in this context, the use of bacterial consortia will permit all these enzymes to be expressed in the presence of the pollutant. In addition, there is evidence that a product of biodegradation using one bacterial strain can be toxic to the same bacterial strain that led to its generation, but it can be a substrate for another bacterial strain, making bacterial consortia a better choice for biodegradation or biomineralization (Kim et al., 2009; Janbandhu and Fulekar, 2011; Kiel and Engesser, 2015).
As shown in Supplementary Tables S1, S2, only seven out of 26 studies on degradation of asphaltenes employed single bacterial stains (ST3,14,16,22; eST1,3–4). Because of the different experimental set-ups, it is not possible to compare degradation efficiency in the context of the use of consortia versus single strains; however, the large number of studies using consortia (75%) is a clear indication that biodegradation of such pollutants occurs more effectively in the context of consortia, as one would have expected. Thus, future studies should focus more on the use of bacterial consortia for degradation of recalcitrant complex compounds such as asphaltenes and resins.
3.7 Biodegradation Under Extreme Conditions
As discussed, the biodegradation of asphaltenes has primarily been investigated under aerobic conditions of normal temperatures and salinity. However, the contamination of asphaltenes and resins also occurs under extreme conditions characterized by high temperature (thermophilic conditions), high salinity (hypersaline conditions) and the absence of O2 (anaerobic conditions). For instance, produced water, generated from oil exploitation and which is contaminated with petroleum products, is characterized by high salinity. The oil reservoir environment in which the remnant of crude oil, which is characterized by heavy oil and a high content of asphaltenes (and resins), is associated with high temperatures and high salinity, and also low–or absence of–O2. Thus, the degradation of pollutants under these conditions requires the use of bacteria that can efficiently grow in extreme environments. For instance, the biodegradation of saturates and aromatics (MAHs and PAHs) has been studied in these extreme environments (Meckenstock et al., 2004; Meckenstock and Mouttaki, 2011; Martins and Peixoto, 2012; Fathepure, 2014; Nzila, 2018b, 2018a). So far, this topic has received little attention in relation to the biodegradation of asphaltenes or resins. For instance, the degradation of asphaltenes under halophilic conditions has been reported in only two studies, in which a single bacterial strain and a consortium were used (eST1,2). Likewise, biodegradation of asphaltenes under thermophilic conditions was reported in a single study, in which a thermophilic strain TH-2 was used at 70°C (eST3). These studies showed that, despite the recalcitrance of asphaltenes to degradation, bacteria that degrade these pollutants do exist in extreme environments. However, as is the case with the degradation of these pollutants under normal conditions, more studies with pure samples are needed to characterize the mechanisms of biodegradation under these extreme conditions.
Finally, investigations have also been carried out under anaerobic conditions that favor methanogenesis (production of methane as a final product of metabolism). In studies ST5 and ST6, bitumen biodegradation was also investigated under anaerobic conditions (in comparison with that under anaerobic conditions), while in eST4, anaerobic biodegradation of asphaltenes was investigated in the presence of molasses. However, evidence for methane production was provided in only one study (ST5). Methanogenesis is a complex process that requires the use of microbial consortia that exhibit individual substrate specificities. Indeed, a microbe is used to degrade a pollutant to a product, which is a substrate of another group of microbes, and this process continues until methane is produced by methanogens, which are archaeans (Merlin Christy et al., 2014; Nzila, 2017). Only one and two bacterial strains were used in ST6 and eST4, respectively, thus it is not surprising that methane was not detected in these studies. The lack of an appropriate consortium accounts for this failure. A consortium of microbes was used in ST5, and although the details of this consortium was not provided, one could conclude the existence of appropriate microbial groups for methanogenesis. Thus, studies on methanogenesis in the context of asphaltene and resin biodegradation await appropriate investigations.
3.8 Microbial Enhanced Oil Recovery and Asphaltene/Resin Biodegradation
As discussed earlier, our understanding of the biodegradation of asphaltenes/resins is still scanty, many gaps exist, and much work is needed to advance our knowledge of the degradation of these compounds. However, current knowledge of asphaltenes and resins can contribute to Microbial Enhanced Oil Recovery (MEOR), which is an oil recovery approach that uses microorganisms and their metabolites to mobilize residual oil (Niu et al., 2020).
With current technologies, it is estimated that less than 45% of oil is recovered during conventional extraction, and as a result, up to 55% of this original oil remains in oil reservoirs (Safdel et al., 2017; Nikolova and Gutierrez, 2020; Niu et al., 2020). This remaining oil is characterized by high viscosity. Strategies are employed to increase this oil recovery, and these involve thermal, chemical, and microbial approaches (i.e., MEOR). However, MEOR remains the most attractive approach since it is cost effective and the most environmentally benign among the different approaches (Nikolova and Gutierrez, 2020; Zhang et al., 2020). MEOR rests on allowing the growth of microbes after their injection into the oil reservoirs, along with nutrients. These microbes, upon growth, generate various metabolites, including biosurfactants, which increase oil emulsification, and decrease interfacial tension and viscosity, thus improving oil solubility and making it more amenable to extraction. Such active microbes are also expected to break down highly complex compounds (such as asphaltenes/resins), thus making oil more extractable.
Bacteria that degrade asphaltenes/resins could also have interesting properties for use in the context of MEOR. As shown in Supplementary Tables S1, S2 (ST13,14,22; eST1,4), studies in which the production of biosurfactants or a change in oil viscosity were investigated, the results have clearly indicated that biodegradation is associated with a reduction in oil viscosity, a central feature in MEOR. Thus, bacteria that degrade bitumen, asphalt or asphaltenes/resins can also be considered as candidate bacteria to be used in the context of MEOR.
3.9 Potential of “Omics” Approaches
An understanding of the mechanisms of degradation could open up the possibility of using novel “Omics” strategies to identify more efficient bacteria that can degrade asphaltenes and resins. In one such strategy, specific genes that encode key enzymes in the degradation of a pollutant can be used as probes to screen and isolate bacteria that carry these genes, and this concept has been used in the identification of bacteria that degrade various pollutants including naphthalene, phenanthrene and benzo [a]pyrene, among other PAHs (Debruyn et al., 2007; DeBruyn et al., 2012; Porter and Young, 2013, 2014; Chen et al., 2016; Ruan et al., 2016; Szewczyk and Kowalski, 2016).
An approach based on transcriptomics, or protein profiling, has also been explored to decipher the mechanisms of pollutant degradation. This technique allows the identification of enzymes that are expressed when bacteria are exposed to pollutants, and it has been used to study the degradation of the various PAHs (Mukherjee et al., 2017; Nzila et al., 2018; Zhu et al., 2019).
The aforementioned approaches necessitate the use of bacteria that can grow efficiently in vitro; However, the limitation imposed by in vitro culture can be overcome using functional genomic techniques, which are based on the cloning of large DNA fragments from uncultured microbes (that grow naturally in a contaminated environment) into specific vectors, and the resulting clones (also known as metagenomic clones) can be transformed into cells, such as Escherichia coli cells. Thereafter, these cells can be screened for their ability to degrade pollutants (Róźalska and Iwanicka-Nowicka, 2016; Szewczyk and Kowalski, 2016). This approach has been used to study the degradation of petroleum alkanes and PAHs (Vasconcellos et al., 2010, 2017; Dellagnezze et al., 2014; Ngara and Zhang, 2018). Although the application of these new “omics” techniques can contribute immensely to our understanding of asphaltene and resin degradation, the use of purified samples of asphaltenes will be needed to take full advantage of these approaches.
4 Concluding Remarks
This literature review has clearly shown that bacteria can degrade asphaltenes and resins, which are among the most recalcitrant oil pollutants. However, there are many gaps in our understanding of this process. For instance, evidence for this biodegradation is based on weight assessment and change in functional groups of asphaltenes and/or resins, but not on monitoring the degradation of a pure compound that can be detected by HPLC, as is carried out with other pollutants. There is no evidence for the complete degradation of asphaltenes and resins or for biomineralization to CO2. Biodegradation does occur, but it is likely to affect predominantly those parts of the asphaltene and resin molecules that contain aliphatic moieties, leaving complex and aromatic moieties, since aliphatic moieties are more amenable to biodegradation. More studies are needed to clarify these gaps.
One of the major limitations in our understanding of the mechanisms of biodegradation of asphaltenes and resins is that most bioremediation experiments for asphaltenes/resins were carried out in mixtures of different compounds. Therefore, it is almost impossible to decipher the pathway(s) of degradation when mixtures of compounds are simultaneously used as substrates. Our experience on the biodegradation of PAHs, and evidence based on the use of IR (presented in this review), point to the possibility of the action of mono- and di-oxygenases as the main initial enzymes in the degradation pathway. However, the detailed pathways of degradation can only be unraveled when a pure sample of asphaltene or resin is isolated or synthesized and used as a substrate. Thus, further efforts should be dedicated towards biodegradation of pure samples of well-defined structures of asphaltenes and resins. This will contribute to our understanding of their biodegradation mechanisms, and also to the discovery of new enzymes with unique functions.
The development of “omics” approaches offers excellent opportunities to better understand the molecular mechanisms of biodegradation of these pollutants. However, these approaches have not yet been explored in the context of asphaltene/resin biodegradation.
In summary, this review has highlighted important gaps that need to be explored in the context of asphaltene/resin biodegradation; moreover, understanding this biodegradation will contribute to improving strategies for oil recovery through MEOR.
Author Contributions
AN conceived the plan for this manuscript and prepared the first draft. MM finalized it, with a major contribution on information pertaining to analytical and organic chemistry. Both authors contributed to the article and approved the submitted version.
Funding
The authors acknowledge the support from the Ministry of Higher Education of Saudi Arabia, through the Deanship of Scientific Research (DSR) at the King Fahd University of Petroleum and Minerals (KFUPM), under grant number LS002505.
Conflict of Interest
The authors declare that the research was conducted in the absence of any commercial or financial relationships that could be construed as a potential conflict of interest.
Publisher’s Note
All claims expressed in this article are solely those of the authors and do not necessarily represent those of their affiliated organizations, or those of the publisher, the editors and the reviewers. Any product that may be evaluated in this article, or claim that may be made by its manufacturer, is not guaranteed or endorsed by the publisher.
Supplementary Material
The Supplementary Material for this article can be found online at: https://www.frontiersin.org/articles/10.3389/fenvs.2021.779644/full#supplementary-material
References
Abdel-Raouf, M. E.-S. (2012). “Factors Affecting the Stability of Crude Oil Emulsions,”. Chapter 10 in Crude Oil Emulsions - Composition Stability and Characterization (Rijeka, Croatia: Manar El-Sayed Abdel-Raouf IntechOpen), 183–204. doi:10.5772/35018
Aditiawati, P., and Kamarisima, K. (2015). Isolation of Asphaltene-Degrading Bacteria from Sludge Oil. Makara J. Sci. 19, 13–20. doi:10.7454/mss.v19i1.4477
Agarry, S. E., and Oghenejoboh, K. O. (2014). Biodegradation of Bitumen in Soil and its Enhancement by Inorganic Fertilizer and Oxygen Release Compound: Experimental Analysis and Kinetic Modelling. J. Microb. Biochem. Technol. 01, 1–8. doi:10.4172/1948-5948.s4-002
Ait-Langomazino, N., Sellier, R., Jouquet, G., and Trescinski, M. (1991). Microbial Degradation of Bitumen. Experientia 47, 533–539. doi:10.1007/BF01949873
Akmaz, S., Iscan, O., Gurkaynak, M. A., and Yasar, M. (2011). The Structural Characterization of Saturate, Aromatic, Resin, and Asphaltene Fractions of Batiraman Crude Oil. Pet. Sci. Technol. 29, 160–171. doi:10.1080/10916460903330361
Ali, H. R., El-Gendy, N. S., Moustafa, Y. M., Roushdy, M. I., and Hashem, A. I. (2012). Degradation of Asphaltenic Fraction by Locally Isolated Halotolerant Bacterial Strains. ISRN Soil Sci. 2012, 435485. doi:10.5402/2012/435485
Asadollahi, L., Salehizadeh, H., and Yan, N. (2016). Investigation of Biosurfactant Activity and Asphaltene Biodegradation by Bacillus Cereus. J. Polym. Environ. 24, 119–128. doi:10.1007/s10924-016-0754-y
Bertrand, J. C., Rambeloarisoa, E., Rontani, J. F., Giusti, G., and Mattei, G. (1983). Microbial Degradation of Crude Oil in Sea Water in Continuous Culture. Biotechnol. Lett. 5, 567–572. doi:10.1007/BF01184950
Brunner, C., Wolf, M., and Bachofen, R. (1987). Enrichment of Bitumen-Degrading Microorganisms. FEMS Microbiol. Lett. 43, 337–344. doi:10.1111/j.1574-6968.1987.tb02169.x
Chen, B., Huang, J., Yuan, K., Lin, L., Wang, X., Yang, L., et al. (2016). Direct Evidences on Bacterial Growth Pattern Regulating Pyrene Degradation Pathway and Genotypic Dioxygenase Expression. Mar. Pollut. Bull. 105, 73–80. doi:10.1016/j.marpolbul.2016.02.054
Chen, B., Ye, X., Zhang, B., Jing, L., and Lee, K. (2019). “Marine Oil Spills-Preparedness and Countermeasures,” in World Seas: An Environmental Evaluation (Second Edition) (London, UK: Academic Press), 407–426. doi:10.1016/B978-0-12-805052-1.00025-5
Davoodi, S. M., Miri, S., Taheran, M., Brar, S. K., Galvez-Cloutier, R., and Martel, R. (2020). Bioremediation of Unconventional Oil Contaminated Ecosystems under Natural and Assisted Conditions: A Review. Environ. Sci. Technol. 54, 2054–2067. doi:10.1021/acs.est.9b00906
de la Huz, R., Lastra, M., and López, J. (2011). “Oil Spills,” in Encyclopedia of Environmental Health. Editor Nriagu, J. O. B. (Burlington: Elsevier), 251–255. doi:10.1016/B978-0-444-52272-6.00568-7
Debruyn, J. M., Chewning, C. S., and Sayler, G. S. (2007). Comparative Quantitative Prevalence of Mycobacteria and Functionally Abundant nidA, nahAc, and nagAc Dioxygenase Genes in Coal Tar Contaminated Sediments. Environ. Sci. Technol. 41, 5426–5432. Available at: http://www.ncbi.nlm.nih.gov/pubmed/17822112. doi:10.1021/es070406c
DeBruyn, J. M., Mead, T. J., and Sayler, G. S. (2012). Horizontal Transfer of PAH Catabolism Genes in Mycobacterium: Evidence from Comparative Genomics and Isolated Pyrene-Degrading Bacteria. Environ. Sci. Technol. 46, 99–106. doi:10.1021/es201607y
Dellagnezze, B. M., de Sousa, G. V., Martins, L. L., Domingos, D. F., Limache, E. E. G., de Vasconcellos, S. P., et al. (2014). Bioremediation Potential of Microorganisms Derived from Petroleum Reservoirs. Mar. Pollut. Bull. 89, 191–200. doi:10.1016/J.MARPOLBUL.2014.10.003
Dhar, K., Subashchandrabose, S. R., Venkateswarlu, K., Krishnan, K., and Megharaj, M. (2020). Anaerobic Microbial Degradation of Polycyclic Aromatic Hydrocarbons: A Comprehensive Review. Rev. Environ. Contam. Toxicol. 251, 25–108. doi:10.1007/398_2019_29
Drent, W. (1972). Effect of Microorganims on Bituminous Materials. Literature Review. European company for the Chemical Processing of Irradatiated Fuels. Available at: https://inis.iaea.org/collection/NCLCollectionStore/_Public/04/043/4043942.pdf (Accessed November 11, 2021). doi:10.2175/106143007x156646
Etoumi, A., El Musrati, I., El Gammoudi, B., and El Behlil, M. (2008). The Reduction of Wax Precipitation in Waxy Crude Oils by Pseudomonas Species. J. Ind. Microbiol. Biotechnol. 35, 1241–1245. doi:10.1007/s10295-008-0420-z
Fakher, S., Ahdaya, M., Elturki, M., and Imqam, A. (2020). Critical Review of Asphaltene Properties and Factors Impacting its Stability in Crude Oil. J. Petrol. Explor Prod. Technol. 10, 1183–1200. doi:10.1007/s13202-019-00811-5
Fakhru’l-Razi, A., Pendashteh, A., Abdullah, L. C., Biak, D. R. A., Madaeni, S. S., and Abidin, Z. Z. (2009). Review of Technologies for Oil and Gas Produced Water Treatment. J. Hazard. Mater. 170, 530–551. doi:10.1016/j.jhazmat.2009.05.044
Farhadian, M., Vachelard, C., Duchez, D., and Larroche, C. (2008). In Situ bioremediation of Monoaromatic Pollutants in Groundwater: A Review. Bioresour. Technol. 99, 5296–5308. doi:10.1016/j.biortech.2007.10.025
Fathepure, B. Z. (2014). Recent Studies in Microbial Degradation of Petroleum Hydrocarbons in Hypersaline Environments. Front. Microbiol. 5, 173. doi:10.3389/fmicb.2014.00173
Gao, H., Zhang, J., Lai, H., and Xue, Q. (2017). Degradation of Asphaltenes by Two Pseudomonas A Strains and Their Effects on Physicochemical Properties of Crude Oil. Int. Biodeterioration Biodegradation 122, 12–22. doi:10.1016/j.ibiod.2017.04.010
Groenzin, H., and Mullins, O. C. (2000). Molecular Size and Structure of Asphaltenes from Various Sources. Energy Fuels 14, 677–684. doi:10.1021/ef990225z
Hao, R., and Lu, A. (2009). Biodegradation of Heavy Oils by Halophilic Bacterium. Prog. Nat. Sci. 19, 997–1001. doi:10.1016/j.pnsc.2008.11.010
Hao, R., Lu, A., and Zeng, Y. (2004). Effect on Crude Oil by Thermophilic Bacterium. J. Pet. Sci. Eng. 43, 247–258. doi:10.1016/j.petrol.2004.02.017
Haritash, A. K., and Kaushik, C. (2016). Degradation of Low Molecular Weight Polycyclic Aromatic Hydrocarbons by Microorganisms Isolated from Contaminated Soil. Int. J. Environ. Sci. 6, 472–782. doi:10.6088/ijes.6053
Haritash, A. K., and Kaushik, C. P. (2009). Biodegradation Aspects of Polycyclic Aromatic Hydrocarbons (PAHs): A Review. J. Hazard. Mater. 169, 1–15. doi:10.1016/j.jhazmat.2009.03.137
Iraji, S., and Ayatollahi, S. (2019). Experimental Investigation on Asphaltene Biodegradability Using Microorganism: Cell Surface Properties' Approach. J. Petrol. Explor Prod. Technol. 9, 1413–1422. doi:10.1007/s13202-018-0537-1
Jahromi, H., Fazaelipoor, M. H., Ayatollahi, S., and Niazi, A. (2014). Asphaltenes Biodegradation under Shaking and Static Conditions. Fuel 117, 230–235. doi:10.1016/j.fuel.2013.09.085
Janbandhu, A., and Fulekar, M. H. (2011). Biodegradation of Phenanthrene Using Adapted Microbial Consortium Isolated from Petrochemical Contaminated Environment. J. Hazard. Mater. 187, 333–340. doi:10.1016/j.jhazmat.2011.01.034
Kiel, M., and Engesser, K.-H. (2015). The Biodegradation vs. Biotransformation of Fluorosubstituted Aromatics. Appl. Microbiol. Biotechnol. 99, 7433–7464. doi:10.1007/s00253-015-6817-5
Kim, Y. M., Ahn, C. K., Woo, S. H., Jung, G. Y., and Park, J. M. (2009). Synergic Degradation of Phenanthrene by Consortia of Newly Isolated Bacterial Strains. J. Biotechnol. 144, 293–298. doi:10.1016/j.jbiotec.2009.09.021
Koshlaf, E., Ball, A. S., and S Ball, A. (2017). Soil Bioremediation Approaches for Petroleum Hydrocarbon Polluted Environments. AIMS Microbiol. 3, 25–49. doi:10.3934/microbiol.2017.1.25
Kuznicki, T., Masliyah, J. H., and Bhattacharjee, S. (2008). Molecular Dynamics Study of Model Molecules Resembling Asphaltene-Like Structures in Aqueous Organic Solvent Systems. Energy Fuels 22, 2379–2389. doi:10.1021/ef800057n
Lapham, L., Proctor, L., and Chanton, J. (1999). Using Respiration Rates and Stable Carbon Isotopes to Monitor the Biodegradation of Orimulsion by Marine Benthic Bacteria. Environ. Sci. Technol. 33, 2035–2039. doi:10.1021/es981158a
Lavania, M., Cheema, S., Sarma, P. M., Mandal, A. K., and Lal, B. (2011). Biodegradation of Asphalt by Garciaella Petrolearia TERIG02 for Viscosity Reduction of Heavy Oil. Biodegradation 23, 15–24. doi:10.1007/s10532-011-9482-0
León, O., Rogel, E., Espidel, J., and Torres, G. (2000). Asphaltenes: Structural Characterization, Self-Association, and Stability Behavior. Energy Fuels 14, 6–10. doi:10.1021/ef9901037
Mafiana, M. O., Bashiru, M. D., Erhunmwunsee, F., Dirisu, C. G., and Li, S.-W. (2021). An Insight into the Current Oil Spills and On-Site Bioremediation Approaches to Contaminated Sites in Nigeria. Environ. Sci. Pollut. Res. 28, 4073–4094. doi:10.1007/s11356-020-11533-1
Martins, L. F., and Peixoto, R. S. (2012). Biodegradation of Petroleum Hydrocarbons in Hypersaline Environments. Braz. J. Microbiol. 43, 865–872. doi:10.1590/S1517-83822012000300003
Meckenstock, R. U., and Mouttaki, H. (2011). Anaerobic Degradation of Non-substituted Aromatic Hydrocarbons. Curr. Opin. Biotechnol. 22, 406–414. doi:10.1016/j.copbio.2011.02.009
Meckenstock, R. U., Safinowski, M., and Griebler, C. (2004). Anaerobic Degradation of Polycyclic Aromatic Hydrocarbons. FEMS Microbiol. Ecol. 49, 27–36. doi:10.1016/j.femsec.2004.02.019
Merlin Christy, P., Gopinath, L. R., and Divya, D. (2014). A Review on Anaerobic Decomposition and Enhancement of Biogas Production through Enzymes and Microorganisms. Renew. Sustain. Energ. Rev. 34, 167–173. doi:10.1016/j.rser.2014.03.010
Minai-Tehrani, D., Rohanifar, P., and Azami, S. (2015). Assessment of Bioremediation of Aliphatic, Aromatic, Resin, and Asphaltene Fractions of Oil-Sludge-Contaminated Soil. Int. J. Environ. Sci. Technol. 12, 1253–1260. doi:10.1007/s13762-014-0720-y
Mukherjee, A. K., Bhagowati, P., Biswa, B. B., Chanda, A., and Kalita, B. (2017). A Comparative Intracellular Proteomic Profiling of Pseudomonas A Strain ASP-53 Grown on Pyrene or Glucose as Sole Source of Carbon and Identification of Some Key Enzymes of Pyrene Biodegradation Pathway. J. Proteomics 167, 25–35. doi:10.1016/j.jprot.2017.07.020
Ngara, T. R., and Zhang, H. (2018). Recent Advances in Function-Based Metagenomic Screening. Genomics, Proteomics & Bioinformatics 16, 405–415. doi:10.1016/j.gpb.2018.01.002
Nikolova, C., and Gutierrez, T. (2020). Use of Microorganisms in the Recovery of Oil from Recalcitrant Oil Reservoirs: Current State of Knowledge, Technological Advances and Future Perspectives. Front. Microbiol. 10, 2996. Available at: https://www.frontiersin.org/article/10.3389/fmicb.2019.02996. doi:10.3389/fmicb.2019.02996
Niu, J., Liu, Q., Lv, J., and Peng, B. (2020). Review on Microbial Enhanced Oil Recovery: Mechanisms, Modeling and Field Trials. J. Pet. Sci. Eng. 192, 107350. doi:10.1016/j.petrol.2020.107350
Nzila, A. (2018a). Biodegradation of High-Molecular-Weight Polycyclic Aromatic Hydrocarbons under Anaerobic Conditions: Overview of Studies, Proposed Pathways and Future Perspectives. Environ. Pollut. 239, 788–802. doi:10.1016/j.envpol.2018.04.074
Nzila, A. (2018b). Current Status of the Degradation of Aliphatic and Aromatic Petroleum Hydrocarbons by Thermophilic Microbes and Future Perspectives. Int. J. Environ. Res. Public Health 15, 2782. doi:10.3390/ijerph15122782
Nzila, A. (2017). Mini Review: Update on Bioaugmentation in Anaerobic Processes for Biogas Production. Anaerobe 46, 3–12. doi:10.1016/j.anaerobe.2016.11.007
Nzila, A., and Musa, M. M. (2020). Current Status of and Future Perspectives in Bacterial Degradation of Benzo[a]pyrene. Int. J. Environ. Res. Public Health 18, 262. doi:10.3390/ijerph18010262
Nzila, A., Ramirez, C. O., Musa, M. M., Sankara, S., Basheer, C., and Li, Q. X. (2018). Pyrene Biodegradation and Proteomic Analysis in Achromobacter Xylosoxidans, PY4 Strain. Int. Biodeterioration Biodegradation 130, 40–47. doi:10.1016/j.ibiod.2018.03.014
Nzila, A. (2013). Update on the Cometabolism of Organic Pollutants by Bacteria. Environ. Pollut. 178, 474–482. doi:10.1016/j.envpol.2013.03.042
Olabemiwo, O. M., Adediran, G. O., Adekola, F. A., Adelowo, O. O., and Alajjire, A. A. (2014). Biodegradation of Hydrocarbon Compounds in Agbabu Natural Bitumen. Afr. J. Biotechnol. 13, 1257–1264. doi:10.5897/AJB2013.12974
Olabemiwo, O. M., Adediran, G. O., Adekola, F. A., Adelowo, O. O., and Olajire, A. A. (2011). Preliminary Study on Biodegradation of Nigerian Natural Bitumen. Microbiol. J. 1, 139–148. doi:10.3923/mj.2011.139.148
Othumpangat, S., and Castranova, V. (2014). “Oil Spills,” in Encyclopedia of Toxicology (Third Edition). Editor P. B. Wexler (Oxford: Academic Press), 677–681. doi:10.1016/B978-0-12-386454-3.00359-6
Pendrys, J. P. (1989). Biodegradation of Asphalt Cement-20 by Aerobic Bacteria. Appl. Environ. Microbiol. 55, 1357–1362. Available at: http://aem.asm.org/content/55/6/1357.abstract. doi:10.1128/aem.55.6.1357-1362.1989
Pineda-Flores, G., and Mesta-Howard, A. M. (2001). Petroleum Asphaltenes: Generated Problematic and Possible Biodegradation Mechanisms. Rev. Latinoam. Microbiol. 43, 143–150. Available at: http://www.ncbi.nlm.nih.gov/pubmed/17061501.
Pineda-Flores, G., Boll-Argüello, G., Lira-Galeana, C., and Mesta-Howard, A. M. (2004). A Microbial Consortium Isolated from a Crude Oil Sample that Uses Asphaltenes as a Carbon and Energy Source. Biodegradation 15, 145–151. doi:10.1023/B:BIOD.0000026476.03744.bb
Porter, A. W., and Young, L. Y. (2014). Benzoyl-CoA, a Universal Biomarker for Anaerobic Degradation of Aromatic Compounds. Adv. Appl. Microbiol. 88, 167–203. doi:10.1016/B978-0-12-800260-5
Porter, A. W., and Young, L. Y. (2013). The bamA Gene for Anaerobic Ring Fission Is Widely Distributed in the Environment. Front. Microbiol. 4, 302. doi:10.3389/fmicb.2013.00302
Potter, T. L., and Duval, B. (2001). Cerro Negro Bitumen Degradation by a Consortium of marine Benthic Microorganisms. Environ. Sci. Technol. 35, 76–83. Available at: http://www.ncbi.nlm.nih.gov/pubmed/11352029. doi:10.1021/es001296b
Redelius, P. (2009). Asphaltenes in Bitumen, what They Are and what They Are Not. Road Mater. Pavement Des. 10, 25–43. doi:10.1080/14680629.2009.9690234
Rojas-Avelizapa, N. G., Cervantes-Gonzalez, E., Cruz-Camarillo, R., and Rojas-Avelizapa, L. I. (2002). Degradation of Aromatic and Asphaltenic Fractions by Serratia Liquefasciens and Bacillus Sp. Bull. Environ. Contam. Toxicol. 69, 835–842. doi:10.1007/s00128-002-0135-1
Rojo, F. (2009). Degradation of Alkanes by Bacteria. Environ. Microbiol. 11, 2477–2490. doi:10.1111/j.1462-2920.2009.01948.x
Rontani, J. F., Bosser-Joulak, F., Rambeloarisoa, E., Bertrand, J. C., Giusti, G., and Faure, R. (1985). Analytical Study of Asthart Crude Oil Asphaltenes Biodegradation. Chemosphere 14, 1413–1422. doi:10.1016/0045-6535(85)90161-4
Róźalska, S., and Iwanicka-Nowicka, R. (2016). “Organic Pollutants Degradation by Microorganisms: Genomics, Metagenomics and Metatranstriptomics Backgrounds,” in Microbial Biodegradation: From Omics to Function and Application. Editor J. Długoński (Norfolk, UK: Caister Academic Press), 1–12. doi:10.21775/9781910190456.01
Ruan, M.-Y., Liang, B., Mbadinga, S. M., Zhou, L., Wang, L.-Y., Liu, J.-F., et al. (2016). Molecular Diversity of Bacterial bamA Gene Involved in Anaerobic Degradation of Aromatic Hydrocarbons in Mesophilic Petroleum Reservoirs. Int. Biodeterioration Biodegradation 114, 122–128. doi:10.1016/j.ibiod.2016.06.005
Safdel, M., Anbaz, M. A., Daryasafar, A., and Jamialahmadi, M. (2017). Microbial Enhanced Oil Recovery, a Critical Review on Worldwide Implemented Field Trials in Different Countries. Renew. Sustain. Energ. Rev. 74, 159–172. doi:10.1016/j.rser.2017.02.045
Sayed, K., Baloo, L., and Sharma, N. K. (2021). Bioremediation of Total Petroleum Hydrocarbons (TPH) by Bioaugmentation and Biostimulation in Water with Floating Oil Spill Containment Booms as Bioreactor Basin. Ijerph 18, 2226. doi:10.3390/ijerph18052226
Scoma, A., Yakimov, M. M., and Boon, N. (2016). Challenging Oil Bioremediation at Deep-Sea Hydrostatic Pressure. Front. Microbiol. 7, 1203. doi:10.3389/fmicb.2016.01203
Seo, J.-S., Keum, Y.-S., and Li, Q. (2009). Bacterial Degradation of Aromatic Compounds. Int. J. Environ. Res. Public Health 6, 278–309. doi:10.3390/ijerph6010278
Shahebrahimi, Y., Fazlali, A., Motamedi, H., Kord, S., and Mohammadi, A. H. (2020). Effect of Various Isolated Microbial Consortiums on the Biodegradation Process of Precipitated Asphaltenes from Crude Oil. ACS Omega 5, 3131–3143. doi:10.1021/acsomega.9b02056
Speight, J. G. (2004). Petroleum Asphaltenes - Part 1: Asphaltenes, Resins and the Structure of Petroleum. Oil Gas Sci. Technol. - Rev. IFP 59 (5), 467–477. doi:10.2516/ogst:2004032
Szewczyk, R., and Kowalski, K. (2016). “Metabolomics and Crucial Enzymes in Microbial Degradation of Contaminants,” in Microbial Biodegradation: From Omics to Function and Application. Editor J. Długoński (Norfolk, UK: Caister Academic Press), 43–66. doi:10.21775/9781910190456.04
Tavassoli, T., Mousavi, S. M., Shojaosadati, S. A., and Salehizadeh, H. (2012). Asphaltene Biodegradation Using Microorganisms Isolated from Oil Samples. Fuel 93, 142–148. doi:10.1016/j.fuel.2011.10.021
Vasconcellos, S. P. D., Angolini, C. F. F., García, I. N. S., Martins Dellagnezze, B., Silva, C. C. D., Marsaioli, A. J., et al. (2010). Screening for Hydrocarbon Biodegraders in a Metagenomic Clone Library Derived from Brazilian Petroleum Reservoirs. Org. Geochem. 41, 675–681. doi:10.1016/J.ORGGEOCHEM.2010.03.014
Vasconcellos, S. P., Sierra-Garcia, I. N., Dellagnezze, B. M., Vicentini, R., Midgley, D., Silva, C. C., et al. (2017). Functional and Genetic Characterization of Hydrocarbon Biodegrader and Exopolymer-Producing Clones from a Petroleum Reservoir Metagenomic Library. Environ. Technol. 38, 1139–1150. doi:10.1080/09593330.2016.1218940
Venkateswaran, K., Hoaki, T., Kato, M., and Maruyama, T. (1995). Microbial Degradation of Resins Fractionated from Arabian Light Crude Oil. Can. J. Microbiol. 41, 418–424. doi:10.1139/m95-055
Wolf, M., and Bachofen, R. (1991a). Microbial Degradation of Bitumen Matrix Used in Nuclear Waste Repositories. Naturwissenschaften 78, 414–417. doi:10.1007/BF01133415
Wolf, M., and Bachofen, R. (1991b). Microbial Legradation of Bitumen. Experientia 47, 542–548. doi:10.1007/BF01949875
World Health Organization (2004). Asphalt (Bitumen). Concise International Chemical Assessment Document 59. Geneva. Available at: https://www.who.int/ipcs/publications/cicad/cicad59_rev_1.pdf (Accessed November 11, 2021).
Yap, H. S., Zakaria, N. N., Zulkharnain, A., Sabri, S., Gomez-Fuentes, C., and Ahmad, S. A. (2021). Bibliometric Analysis of Hydrocarbon Bioremediation in Cold Regions and a Review on Enhanced Soil Bioremediation. Biology 10, 354. doi:10.3390/biology10050354
Zhang, J., Gao, H., and Xue, Q. (2020). Potential Applications of Microbial Enhanced Oil Recovery to Heavy Oil. Crit. Rev. Biotechnol. 40, 459–474. doi:10.1080/07388551.2020.1739618
Keywords: asphaltenes, resins, asphalt, bitumen, biodegradation, bacteria, pollution, petroleum products
Citation: Nzila A and Musa MM (2021) Current Knowledge and Future Challenges on Bacterial Degradation of the Highly Complex Petroleum Products Asphaltenes and Resins. Front. Environ. Sci. 9:779644. doi: 10.3389/fenvs.2021.779644
Received: 19 September 2021; Accepted: 02 November 2021;
Published: 19 November 2021.
Edited by:
Erick Bandala, Desert Research Institute (DRI), United StatesReviewed by:
Emad K. Radwan, National Research Centre, EgyptDeborah Xanat Flores-Cervantes, University of the Americas Puebla, Mexico
Copyright © 2021 Nzila and Musa. This is an open-access article distributed under the terms of the Creative Commons Attribution License (CC BY). The use, distribution or reproduction in other forums is permitted, provided the original author(s) and the copyright owner(s) are credited and that the original publication in this journal is cited, in accordance with accepted academic practice. No use, distribution or reproduction is permitted which does not comply with these terms.
*Correspondence: Alexis Nzila, alexisnzila@kfupm.edu.sa