- 1Institute of Soil Research, University of Natural Resources and Life Sciences, Vienna, Austria
- 2Ministry of Agriculture and Livestock, Galápagos, Ecuador
- 3Central University of Ecuador, Galápagos, Ecuador
- 4Galápagos National Park Directorate, Galápagos, Ecuador
While the extended absence of human influence has led to matchless natural conditions on the Galápagos archipelago, agricultural activities on the inhabited islands are increasingly affecting soil health and functioning. However, a systematic assessment of the agricultural soils on the Galápagos Islands is still absent. Plate tectonics and hotspot volcanism cause an eastward drift of the archipelago and result in a west-to-east soil age gradient from approx. 1 to 1,000 ka. In addition, precipitation regimes change from arid to humid with elevation on the higher-standing islands. The objective of this study was to investigate differences in soil fertility parameters and Mehlich (III)-extractable nutrient levels along these gradients in order to provide baseline information for sustainable agricultural management. Topsoil samples (0–20 cm) from 125 farms of the islands Isabela, Santa Cruz and San Cristóbal were analyzed. Gravel and sand content, pH, electrical conductivity, cation exchange capacity, base saturation, soil organic C and total N content tended to decrease with increasing island age, while clay content, soil macroaggregate stability, plant-available water and bulk density increased. Mehlich (III)-extractable base cations Ca, K, Mg and Na as well as P, Fe and Zn showed a decreasing tendency from the youngest to the oldest island, while Mn increased with island age. Mehlich (III)-extractable Cu and Na reached their highest levels on the most intensively farmed, intermediate-aged island Santa Cruz, likely related to anthropogenic inputs and irrigation with brackish water, respectively. Changes along the altitudinal climate gradient within the studied islands were most significant for soil pH, base saturation, and Mehlich (III)-extractable Ca and Mn. Our results highlight the importance of site-specific agricultural management to account for the strong heterogeneity in soil parameters among and within the Galápagos archipelago. The data provided herein shall serve as a baseline for targeted future management strategies to avoid soil degradation, restore and maintain soil functioning and, hence, sustain the soils’ provision of ecosystem services in this unique archipelago.
Introduction
Soils play a vital role in terrestrial ecosystems, providing the basis for food and biomass production, regulating and purifying water flows as well as significantly contributing to biodiversity, among other services (Adhikari and Hartemink, 2016). Land degradation processes driven by anthropogenic impacts result in impaired soil functions and increasingly compromise ecosystem services. Owing to its remote geographical location, the Galápagos archipelago had remained untouched by human influence for a long period of time, enabling the evolution of numerous endemic species under matchless biological conditions (Tye et al., 2002). However, several of the islands are now inhabited, and agricultural activities have intensified in recent decades in efforts to meet the increasing demand for agricultural products (Watson et al., 2010). Considering the growing pressure on this unique ecosystem and the islands’ local food security, a better understanding of the island’s soil resources is a prerequisite for sustainable agricultural management and also represents an important contribution to the environmental research agenda for Galápagos (Izurieta et al., 2018).
The remote island chain is an extraordinary “natural laboratory,” not only for studying the evolution of plant and animal species but also to investigate how soils have formed in response to different environmental factors. Plate tectonics in combination with ongoing volcanic activity in the western part of Galápagos cause an eastward drift of the archipelago and result in a pronounced gradient of rock ages between the islands (Zehetner et al., 2020). In addition, climate and especially precipitation regimes driven by the southeast trade winds change from arid lowlands to humid highlands on the windward side of the higher-standing islands (Trueman and D’Ozouville, 2010).
Systematic information on (top)soil properties in Galápagos is still scarce, and most of the available baseline information is drawn from a geo-pedological mission in 1962 (Stoops, 2013; Taboada et al., 2016; Rial et al., 2017) with very few additional later studies (e.g., Adelinet et al., 2008). In 2016 and 2017, three soil scientific expeditions laid the foundation for further, more detailed investigations, and the present study forms part of these ongoing research efforts carried out by the University of Natural Resources and Life Sciences Vienna in cooperation with the Galápagos National Park Directorate and other partner institutions. From these recent activities, several new papers on weathering and soil formation have already been published (e.g., Candra et al., 2019; Zehetner et al., 2020), and also the agricultural soils of the archipelago have been investigated (e.g., Gerzabek et al., 2019; Dinter et al., 2020, Dinter et al., 2021). Gerzabek et al. (2019) studied the effects of agricultural land management on soil quality parameters on two of the islands (Santa Cruz and San Cristóbal) by comparing arable soils to soils under forest in adjacent Galápagos National Park areas. They found signs of soil fertility decline on both investigated arable sites such as decreased SOC and total N stocks, diminished M3-extractable nutrient levels as well as reduced microbial biomass. The publications of Dinter et al. (2020), Dinter et al. (2021) are of special interest for the present study, because they are based on the same sample set as the present study, including three islands of differing ages (Isabela, Santa Cruz and San Cristóbal) and covering elevation/climate zones ranging from dry lowlands to humid highlands. Dinter et al. (2020) characterized the basic properties and nutrient reserves of agricultural soils in relation to climate and island age. They found that non-crystalline constituents and andic properties were mainly present in the young soils of Isabela Island, while the highly weathered, older soils of San Cristóbal Island were dominated by crystalline clays and iron oxides and had already lost andic properties. Furthermore, aqua regia-extractable base cations tended to decrease with increasing island age and elevation/moisture, while Al and Fe accumulated along the same gradients (Dinter et al., 2020). In Dinter et al. (2021), the focus was on trace elements, and total contents of Cd, Co, Cr, Cu, Ni and Zn were above threshold levels in many of the studied soils. The results of that study also suggest that elevated soil concentrations of Cd, Zn and Cu may be attributable to the use of agrochemicals (Dinter et al., 2021).
The present study looks more specifically into fertility parameters of arable topsoils and the resulting agricultural implications for the respective islands, considering physical and chemical indicators of soil fertility as well as Mehlich (III)-extractable (M3-extractable) nutrient levels. While Dinter et al. (2020), Dinter et al. (2021) reported on aqua regia-extractable nutrient reserves of the studied agricultural soils, our study focusses on rather bioavailable fractions of macro- and micronutrients (P, K, Ca, Mg, Na, Fe, Mn, Zn, Cu). We analyzed topsoil samples from 125 farms across three of the Galápagos islands (Isabela, Santa Cruz and San Cristóbal), which corresponds to approximately 16.5% of the agricultural production units on the archipelago (CGREG et al., 2014). The selected sites include various soil types that have formed over different durations and under different climatic regimes. Our main objectives were 1) to assess the soils fertility status in relation to soil age across the three studied islands, 2) to assess soil fertility differences between the different elevation zones within the agricultural areas of the youngest and oldest studied island, and 3) to provide baseline data for sustainable agricultural management practices on the different Galápagos islands against the background of changing environmental conditions.
Study Area
Natural Setting and Environmental Gradients
The Galápagos archipelago is situated at the equator approx. 1,000 km west of the coast of Ecuador (Figure 1). The islands were formed by hotspot-induced volcanism under the Nazca tectonic plate. The estimated center of the volcanic hotspot is located southwest of Fernandina Island at the western margin of the archipelago (Hooft et al., 2003; Figure 1), and the Nazca plate has been moving eastward at a speed of approx. 51 km/Ma (Argus et al., 2011). This results in a pronounced age gradient of the volcanic parent materials from west to east. The western island Isabela has large areas of geologically young land surfaces (<5 ka; Reynolds et al., 1995), while the oldest dated lavas on the eastern island San Cristóbal are 2.35 Ma old (White et al., 1993). The parent materials of the studied soils cover an age range from approx. 1.5 to ≥1,070 ka and include alkali basalts and tholeiites. For more details on the geologic setting, s. Zehetner et al. (2020).
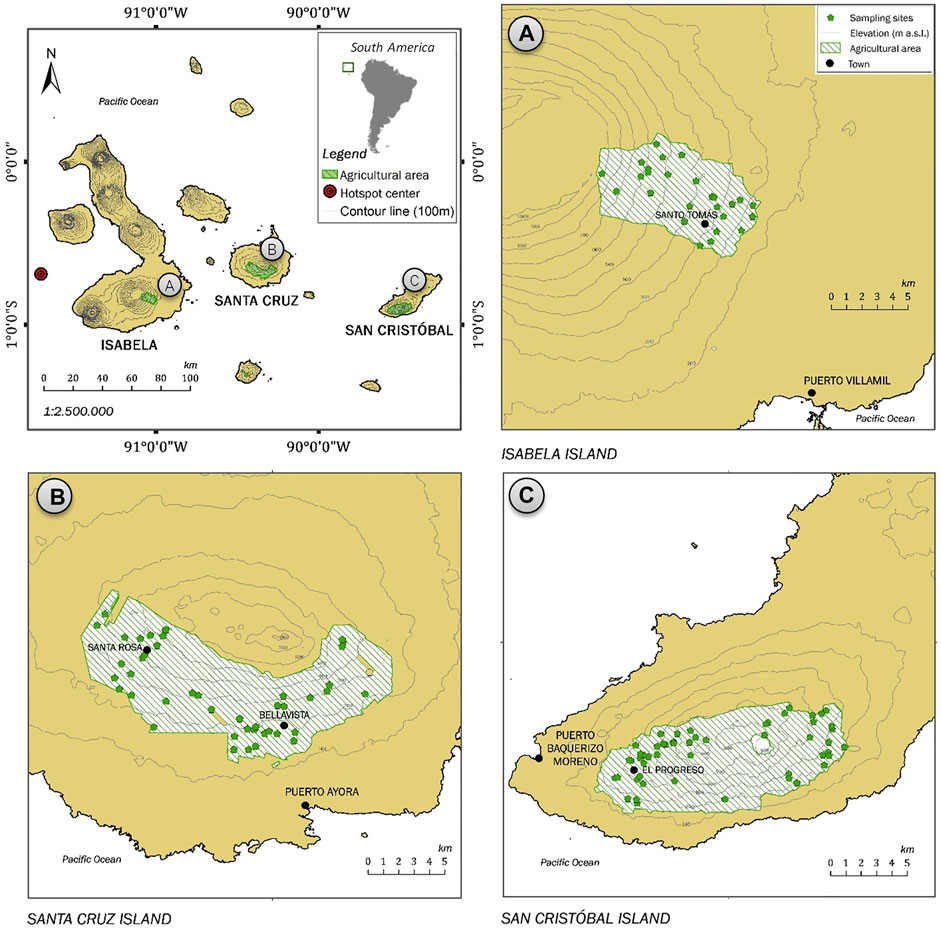
FIGURE 1. Map of the sampling locations within the agricultural areas of the three studied islands Isabela, Santa Cruz and San Cristóbal. The center of the volcanic hotspot was estimated by Hooft et al. (2003); m a.s.l. = meters above sea level.
The climate of the Galápagos Islands is rather cool (mean annual temperature: 18–21°C in the highlands, where most of the agricultural area is located) and dry compared to other equatorial regions (Lasso et al., 2018), which is due to cool ocean currents and the prevailing southeast trade winds. The latter convey moisture to the windward, southeast side of the higher-standing islands, which results in a pronounced climatic gradient from arid lowlands to humid highlands, while the leeward side remains rather dry throughout (Itow, 2003). For Santa Cruz Island, median annual rainfall of 277 and 813 mm has been recorded by meteorological stations in the arid coastal zone (2 m a.s.l.) and in the transition zone (194 m a.s.l.), respectively, while mean annual precipitation in the humid highlands has been estimated up to 1,600 mm (Trueman and D’Ozouville, 2010). For more details on the climatic conditions, s. Zehetner et al. (2020). The agricultural areas under study are located on the windward side of the three islands covering an elevation range from approx. 100–900 m a.s.l. (Figure 1). In analogy to Dinter et al. (2020), the sites of this study were grouped into three elevation zones reflecting different moisture regimes: arid, dry and transition zone (<250 m a.s.l.), humid zone (250–500 m a.s.l.) and very humid zone (>500 m a.s.l.). This classification is based on the bioclimatic zonation outlined by Rial et al. (2017), vegetation zones described by Ingala-Pronareg-Orstom. (1987) and isohyets for total seasonal rainfall developed by Trueman and D’Ozouville. (2010).
Agriculture on Galápagos
Agriculture on the archipelago was initiated by the first colonizers during the 1800’s (Perry, 1984) and has expanded over time. In 1959, the Galápagos National Park was created and 97% of the land area was declared protected national park area, with only 3% remaining for agriculture and human settlements (Guézou et al., 2010). In recent decades, agricultural land use within these 3% of land has intensified in order to meet the increasing demand for food of a growing resident population and flourishing tourism industry. Along with this, the use of mineral fertilizers and agrochemicals has increased (CGREG et al., 2014). A major challenge for agriculture on Galápagos is the limitation of freshwater during extended periods of drought, which may be further exacerbated in the future, as precipitation events governed by the El Niño phenomenon become increasingly irregular in response to changing global climatic patterns (Snell and Rea, 1999). Presently, a great variety of agricultural products can be encountered on the archipelago, including short cycle crops (grains and vegetables) and long cycle crops (fruits and sugarcane), along with several areas dedicated to grazing (Villa and Segarra, 2010). Most of the products are cultivated by hand without the use of heavy machinery and sold locally or grown for private consumption, aside from little exports of coffee (O’Connor Robinson et al., 2018).
Materials and Methods
Soil Sampling
The 125 topsoil samples under investigation were collected in 2016 from areas designated for agricultural use (Figure 1) on the islands Isabela (26), Santa Cruz (46) and San Cristóbal (53). Bulk soil samples were drawn from a homogenized mixture of 15–20 subsamples per site, which were collected on a 2,500 m2 plot from a depth of 0–20 cm. The bulk samples were air-dried and sieved to 2 mm. Additionally, undisturbed core samples were extracted in 250-cm3 steel cylinders at approx. 10 cm soil depth at each site. The samples cover an elevation range of 146–920 m a.s.l. on Isabela, 130–572 m a.s.l. on Santa Cruz, and 176–604 m a.s.l. on San Cristóbal.
Soil Physical Parameters
Volumetric gravel content (>2 mm) of soils with predominantly lapilli of <30 mm equivalent diameter was measured in graduated cylinders after separating the gravel from the fine earth fraction (<2 mm) by sieving. For soils with coarse fragments >30 mm, the gravel content was visually estimated in profile pits using field comparison charts. For bulk density (BD) determination, the material from the 250-cm3 cylinders was weighed after oven-drying at 105°C. Soil macroaggregate stability (250–2000 µm) was tested by ultrasonic dispersion at a specific ultrasonic energy of 1500 J ml−1 applied to a suspension containing 5 g of dried soil aggregates (1,000–2000 µm) in 200 ml of water. A commercial ultrasonic device (Bandelin Sonoplus HD 2200 with cylindrical ultrasonic probe V70) was used, and the degree of ultrasonic aggregate stability (USAS) was calculated according to Mentler et al. (2004) from the stable macroaggregates remaining. Water retention at 30 and 1,500 kPa tension, respectively, was measured with a pressure membrane apparatus (Klute, 1986). Plant-available water (PAW) was calculated as the difference between the amount of water held at 30 kPa (field capacity) and 1,500 kPa (permanent wilting point), taking into account the sampling depth of 20 cm and the volumetric percentage of coarse fragments. Particle size distribution was determined using a combined sieve and pipette method as described by Soil Survey Staff. (2014). The unique nature of volcanic soils required various steps of pretreatments and dispersion techniques, which are detailed in Dinter et al. (2020).
Soil Chemical Parameters
Electrical conductivity (EC) was measured in water extracts at a soil-to-solution ratio of 1:10, and soil pH was determined in H2O at a ratio of 1:1 and in 1 M sodium fluoride (NaF) at 1:50 (Soil Survey Staff, 2014). Total C and N was determined by dry combustion (Tabatabai and Bremner, 1991) and soil organic carbon (SOC) was calculated as the difference between total C and carbonate C determined gas-volumetrically according to Soil Survey Staff (2014). Phosphate retention was analyzed using the method of Blakemore et al. (1987). Iron contained in crystalline and non-crystalline hydrous Fe oxides was extracted using citrate-bicarbonate-dithionite (Fed; Mehra and Jackson, 1958). Iron and aluminum associated with non-crystalline constituents was extracted using acid ammonium oxalate at pH 3 (Feo, Alo; Schwertmann, 1964). Selected macro- and micronutrients (P, K, Ca, Mg, Na, Fe, Mn, Zn, Cu) were extracted with the Mehlich (III) method (Mehlich, 1984), and the extracted element concentrations were determined by inductively coupled plasma-optical emission spectroscopy (ICP-OES). It should be noted that the M3-extractable elements in the present study cannot be directly considered as plant-available due to the lack of on-site calibration with field experiments to determine the actual plant uptake. Nevertheless, the Mehlich (III) multiple-element extractant has proven its ability to produce relatively good correlations between extracted nutrients and plant response over a wide pH range (Zbíral, 2016). Cation exchange capacity (CEC) was estimated by summation of M3-extractable base cations plus exchangeable acidity (Ngewoh et al., 1989). Base saturation was calculated as the percentage of CEC occupied by base cations Ca2+, Mg2+, K+ and Na+ (Havlin, 2005). Exchangeable sodium percentage (ESP) was calculated by dividing exchangeable Na+ by the sum of exchangeable cations and multiplying the result by 100 (Levy and Shainberg, 2005).
Statistical Analyses
Statistical analyses were performed with R Studio software for Windows (version 3.4.3), except for the correlation graphs, which were elaborated in Microsoft Excel (Office 365). Descriptive statistics was displayed as boxplots showing arithmetic mean, median, upper and lower quartiles, minimum and maximum values, as well as outliers for each island separately. Principal component analysis (PCA) was carried out using the R package FactoMineR, where values were standardized prior to analysis to ensure standard deviation between 0 and 1. The factor scores of the individual islands were grouped into three elevation zones (<250, 250–500 and >500 m a.s.l.), as mentioned above. Many of the variables displayed a non-normal distribution and the skewness values ranged from −0.5 up to 5.3 in extreme cases, largely attributable to outliers. As outliers can convey important information about the data, we decided to keep them in the dataset and include them in all the calculations. Therefore, we used Spearman’s rank correlation coefficient, which is not unduly influenced by skewness. The corresponding correlation tables are enclosed in Supplementary Tables S3–S5.
The hotspot distances of the respective sampling sites were calculated by spatial distance measurements in ArcMap 10.6.1 based on the location of the hotspot center as estimated by Hooft et al. (2003) (s. Figure 1). Maps displaying the spatial distribution of the analyzed soil parameters on each island are shown in Supplementary Figures S1–S24. These maps were elaborated using ArcMap 10.6.1 for Windows. All data displayed in the maps were classified using natural breaks.
Results
Basic Soil Physical and Chemical Properties
The results of the physical and chemical analyses are shown in Table 1 and Figure 2, with maps showing their spatial distribution on the studied islands enclosed in Supplementary Figures S1–S15. Descriptive statistics and Spearman’s rank correlation coefficients are enclosed in Supplementary Tables S1, S3–S5, respectively. The soils became finer-textured with increasing island age. On the youngest studied island, Isabela, the soils had high gravel and sand contents and prevailing silt loam texture, while on the intermediate-aged and oldest studied islands, Santa Cruz and San Cristóbal, respectively, gravel contents were much lower and the soils more clayey. Nevertheless, the existence of outliers, reflecting local heterogeneity within the islands should be noted. Soil BD increased with increasing island age from a mean of 0.51 g cm−3 on the youngest island, to means of 0.83 and 0.91 g cm−3 on the intermediate-aged and oldest island, respectively. Moreover, BD was negatively correlated with elevation on the youngest island, Isabela (rs −0.46, p < 0.05; Supplementary Table S4). Soil pH and EC decreased with increasing island age from 6.5 to 5.6 and from 393 to 221 μS cm−1 (mean values), respectively. SOC varied between 5 and 30% (mean: 16%) on the youngest island, while on the oldest island, SOC was <5% for most of the tested samples. On the intermediate-aged island, Santa Cruz, we found SOC levels between 4 and 12%. Similarly, total N strongly decreased with increasing hotspot distance (rs -0.77, p < 0.001, Supplementary Table S3), showing island means of 14.5 g kg−1 on Isabela, 5.7 g kg−1 on Santa Cruz and 3.3 g kg−1 on San Cristóbal. PAW content of the studied topsoils (0–20 cm) increased only slightly with island age, while soil macroaggregate stability (USAS) increased strongly with hotspot distance (rs 0.79, p < 0.001, Supplementary Table S3), from an island mean of 9.6% on Isabela to 81.2% on San Cristóbal. CEC was similar for islands Isabela (mean: 348 mmolc kg−1) and Santa Cruz (332 mmolc kg−1) but significantly lower on the oldest studied island, San Cristóbal (165 mmolc kg−1). Base saturation tended to decrease with increasing island age, although the wide interquartile range on San Cristóbal indicates a high level of heterogeneity. The andic indicator Alo+0.5Feo decreased with increasing island age, showing mean values above the andic threshold level of ≥2% (IUSS Working Group WRB, 2015) on the youngest and intermediate-aged islands, while for the oldest studied island, San Cristóbal, Alo+0.5Feo was clearly below this level (mean: 0.66%). Conversely, iron contained in hydrous iron oxides (Fed) increased with island age, reaching a maximum of 121.6 g kg−1 (mean: 62.9 g kg−1) on San Cristóbal.
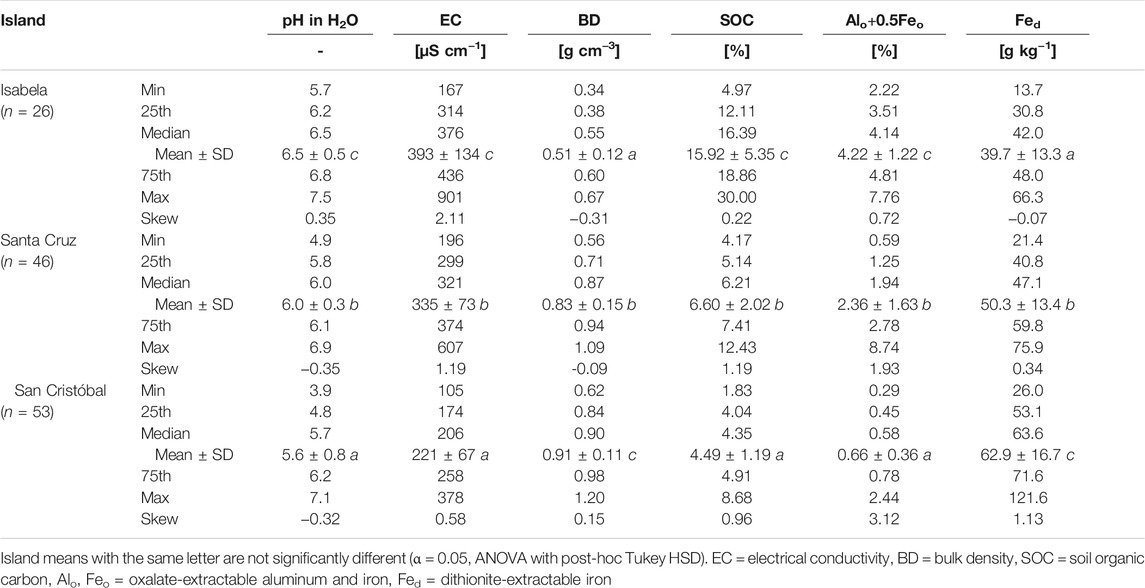
TABLE 1. Descriptive statistics (minimum, 25th percentile, median, mean ± standard deviation, 75th percentile, maximum, skewness) of key soil properties on the three studied islands.
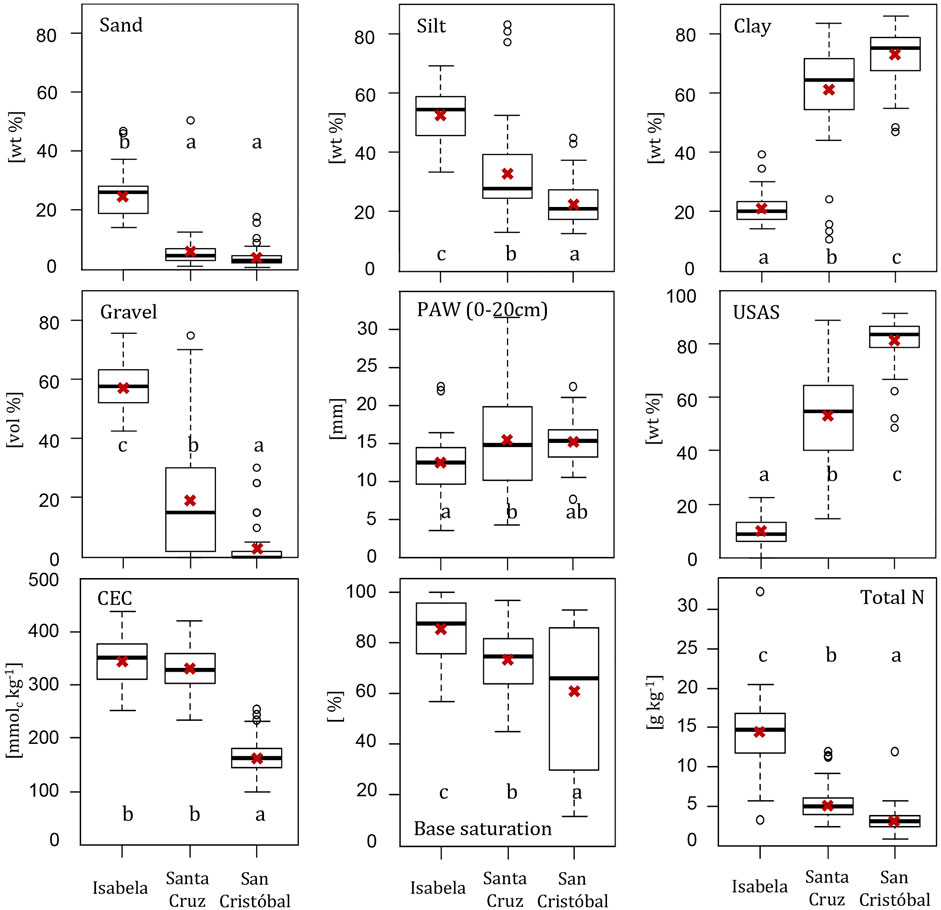
FIGURE 2. Selected soil physical and chemical properties across the islands Isabela, Santa Cruz and San Cristóbal. Mean values are indicated by the symbol x, dots indicate outliers. Horizontal bars represent minimum, 25th percentile, median, 75th percentile and maximum. PAW = plant-available water, USAS = ultrasonic aggregate stability, CEC = cation exchange capacity.
Mehlich(III)-Extractable Elements
The M3-extractable concentrations of macro- and micronutrients are presented in Figure 3, and their spatial distribution on the studied islands is shown in Supplementary Figures S16–S24. Descriptive statistics and Spearman’s rank correlation coefficients are enclosed in Supplementary Tables S2, S3–S5, respectively. The island means of K and Mg were significantly higher on Isabela and Santa Cruz compared to San Cristóbal. M3-extractable Ca, Fe and Zn showed a decreasing trend with increasing island age (correlations with hotspot distance: Ca: rs −0.74, Fe: rs −0.64, Zn: rs −0.63; p < 0.001; Supplementary Table S3), although the difference of Fe mean values for Santa Cruz and San Cristóbal was not significant (Figure 3). The means of M3-extractable P on the three studied islands were not significantly different from one another, and extreme outliers were detected especially on Santa Cruz Island. However, we found decreasing P median values from the youngest to the oldest studied island and a generally decreasing trend with increasing hotspot distance (P: rs −0.27, p < 0.01; Supplementary Table S3). Although M3-extractable Na concentrations decreased between the youngest (median: 35.0 mg kg−1) and oldest (median: 22.0 mg kg−1) island, we detected highest concentrations on the intermediate-aged island, Santa Cruz (median: 53.5 mg kg−1). Likewise, M3-extractable Cu was elevated on Santa Cruz Island (median: 5.2 mg kg−1, compared to 3.0 mg kg−1 and 2.5 mg kg−1 on the islands Isabela and San Cristóbal, respectively). By contrast, M3-extractable Mn was much lower (median: 8.5 mg kg−1) on the youngest studied island, compared to the intermediate-aged (81.5 mg kg−1) and oldest studied island (68.0 mg kg−1).
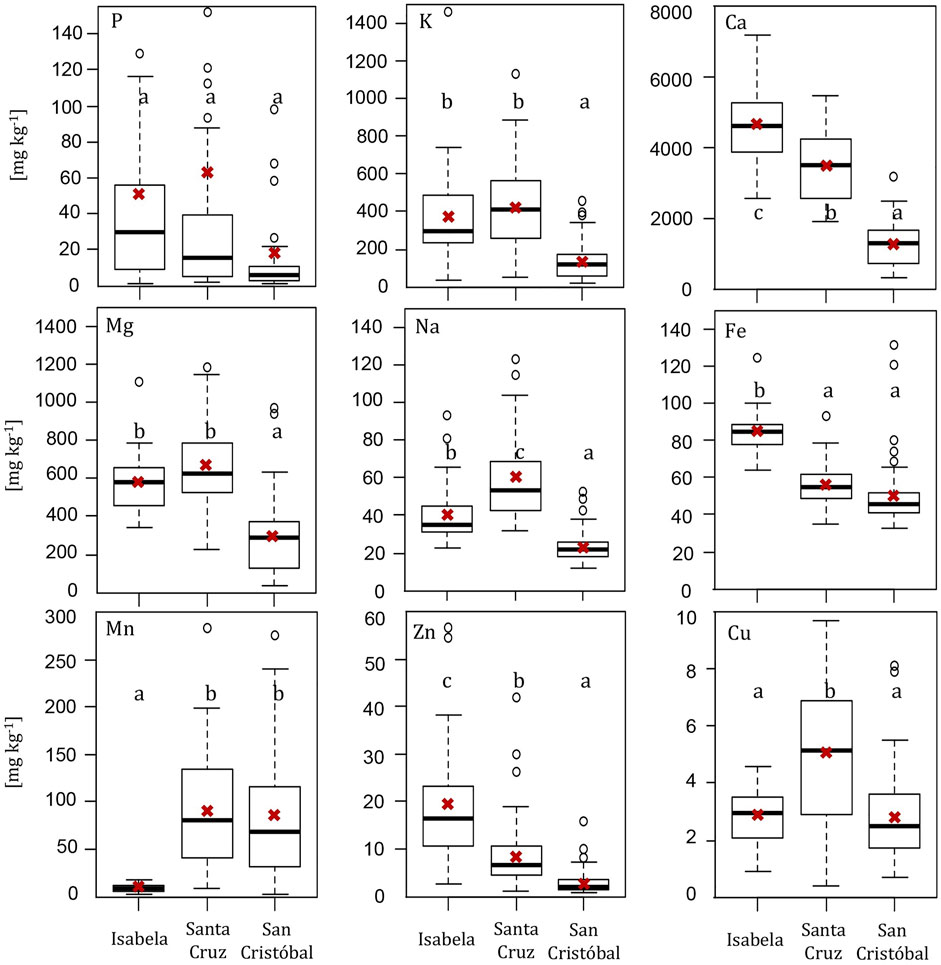
FIGURE 3. Mehlich (III)-extractable element concentrations across the islands Isabela, Santa Cruz and San Cristóbal. Mean values are indicated by the symbol x, dots indicate outliers. Horizontal bars represent minimum, 25th percentile, median, 75th percentile and maximum. For P, five extreme outliers are not displayed in the boxplot: 423 mg kg-1 on Isabela, 573 and 1,036 mg kg-1 on Santa Cruz, and 213 and 222 mg kg-1 on San Cristóbal.
The altitudinal trends of M3-extractable Ca and Mn on the youngest and oldest studied islands are displayed along with soil pH in Figure 4. Correlations of all studied M3-extractable elements with elevation are shown in Supplementary Tables S4, S5. M3-extractable Ca followed the altitudinal trends of soil pH and decreased with increasing elevation for both islands (Isabela: rs −0.70, San Cristóbal: rs −0.57, p < 0.001; Supplementary Tables S4, S5). Conversely, M3-extractable Mn increased with increasing elevation on the youngest island, Isabela, while it decreased on the oldest island, San Cristóbal. M3-extractable Cu decreased only slightly with increasing elevation on Isabela (rs −0.46, p < 0.05; Supplementary Table S4), but more strongly on San Cristóbal (rs −0.69, p < 0.001; Supplementary Table S5).
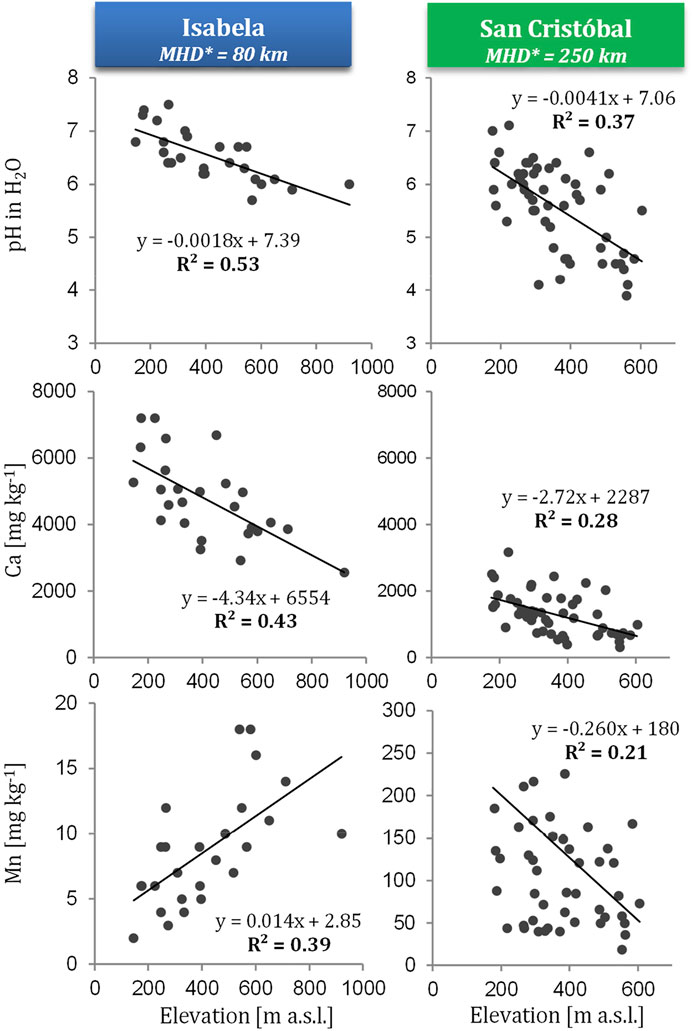
FIGURE 4. Altitudinal trends in soil pH and Mehlich (III)-extractable Ca and Mn for the islands Isabela and San Cristóbal. MHD = mean hotspot distance (horizontal distance of sampling points from the hotspot center as estimated by Hooft et al., 2003); m a.s.l. = meters above sea level.
Principal Component Analysis
The results of principal component analyses (PCAs) performed with the M3-extractable element data are shown in Figure 5. Differences between the studied islands were investigated in a PCA based on element data from all islands (Figure 5, top), while trends within the youngest island, Isabela (Figure 5, middle), and the oldest island, San Cristóbal (Figure 5, bottom), were investigated in separate PCAs based on element data of the respective islands and grouping into three elevation zones. Hotspot distance, elevation and additional soil properties were included as supplementary variables to help identify potential drivers of the observed patterns.
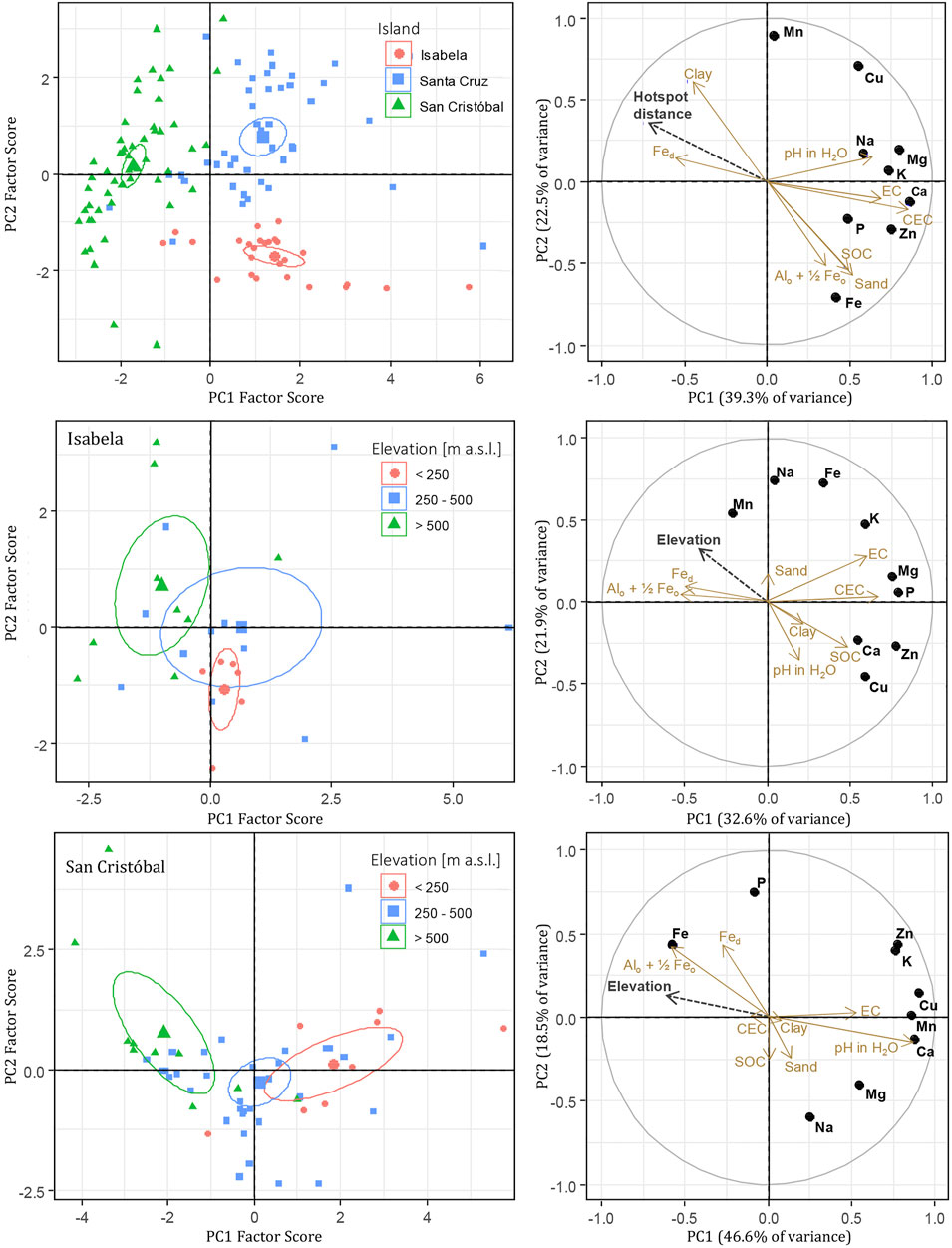
FIGURE 5. Principal component analyses based on Mehlich (III)-extractable element concentrations for all studied islands (top), Isabela (middle) and San Cristóbal (bottom). Enlarged symbols and ellipses in the factor scores plots (left side) indicate means and 95% confidence intervals, respectively. The right side displays the loadings of the Mehlich (III)-extractable element concentrations (dots) and relationships with other parameters (arrows). EC = electrical conductivity, CEC = cation exchange capacity, SOC = soil organic carbon, Fed = dithionite-extractable iron, Alo and Feo = oxalate-extractable aluminum and iron; m a.s.l. = meters above sea level.
The all-island PCA showed a segregation of the oldest island, San Cristóbal (negative scores), from the other two islands (positive scores) along PC1, and a segregation of the youngest island, Isabela (negative scores), from the other two islands (dominantly neutral to positive scores) along PC2 (Figure 5, top left). PC1 (39.3% of variance) was directly related to soil pH, EC and CEC, and inversely related to hotspot distance and Fed. It showed positive loadings for all studied M3-extractable elements, with particularly high loadings for Ca, Mg, K and Zn. PC2 (22.5% of variance) was directly related to clay content and inversely related to sand content, SOC and Alo+0.5Feo. It displayed the highest positive loadings for micronutrients Mn and Cu and a high negative loading for Fe.
In the PCAs of the youngest and oldest studied islands, Isabela and San Cristóbal, respectively, the three elevation zones were segregated with some overlap of their 95% confidence intervals, and their arrangement in the scores plots broadly corresponded to the direction of the factor elevation shown in the loadings plots (Figure 5, middle and bottom). For Isabela, PC1 (32.6% of variance) was directly related to EC and CEC, and inversely related to elevation, Alo+0.5Feo and Fed. It showed positive loadings for most of the M3-extractable elements except for Mn. For San Cristóbal, PC1 (46.6% of variance) was directly related to soil pH and EC, and inversely related to elevation and Alo+0.5Feo. It showed high positive loadings for Ca, K, Mn, Cu and Zn, and a negative loading for Fe. PC2 (21.9% of variance for Isabela, 18.5% for San Cristóbal) was little related to any of the tested supplementary variables and showed an inconsistent picture in the factor loadings for the two islands, e.g. Na had a high positive loading for Isabela and the opposite loading for San Cristóbal.
Discussion
Trends With Island Age
Our results indicate marked pedogenic development along the studied island chain, resulting in strong differences in fertility parameters between the volcanic topsoils of our study. Soil texture and gravel content exhibited very pronounced changes, with particle sizes sharply decreasing with increasing distance from the volcanic hotspot (Figure 2). Soils formed on basaltic parent materials often show high concentrations of volcanic glass in the early stage and rapid neoformation of clay-sized, poorly crystalline materials in the subsequent weathering stage (e.g., Chorover et al., 2004; Mikutta et al., 2009). Similar trends of rapidly increasing clay contents with soil age have been reported by several studies on the development of volcanic soils under different climatic conditions, including Lowe. (1986), Jahn et al. (1987), Delvaux et al. (1989) and Zehetner and Miller. (2006). Soil BD changed from very low values (mean: 0.51 g cm−3) on the youngest studied island, Isabela, to higher values on the intermediate and oldest island (means: 0.83 and 0.91 g cm−3, respectively; Table 1). Our findings are broadly comparable to those reported for Hawaii. For example, Chadwick et al. (2003) mainly observed low BD values (<0.5 g cm−3) in the upper layers of soils developed on young lava flows, while higher BD values (mean: 1.25 g cm−3) have been reported for older, kaolinitic soils in Hawaii (Santo and Tsuji, 1977).
SOC and total N contents were extraordinarily high on the youngest island, Isabela (means: 15.9% and 14.5 g kg−1, respectively; Figure 2 and Table 1) which is characteristic of soils formed on young volcanic parent materials (Shoji et al., 1993; Nanzyo, 2002), and subsequently decreased with increasing soil age. The pronounced accumulation of soil organic matter (SOM) on Isabela Island is probably due to protective effects by non-crystalline constituents (mean Alo+0.5Feo: 4.22%; Table 1), as pointed out by Torn et al. (1997) for Hawaiian soils. The slight increase in PAW with island age may be related to increases in clay content and Fe oxides (Figure 2; Table 1). Nevertheless, comparatively high PAW was also found in the young soils of Isabela Island (mean: 12.5 mm in 0–20 cm depth) despite relatively low clay contents (mean: 21.4%) compared to Santa Cruz (60.7%) and San Cristóbal (72.8%). Possible explanations for this could be the aforementioned accumulation of SOM and abundance of non-crystalline materials as well as the high porosity in the young Andosols, properties that have been reported beneficial for water retention (Delmelle et al., 2015). Also, Jahn and Stahr (1996) reported higher PAW contents (20 vol%) in approx. 6-ka-old Andosols on Lanzarote Island compared to older polygenetic soils (40 ka; 10–20 vol%).
The pronounced increase in macroaggregate stability (USAS) observed in our study paralleled increasing clay and Fe oxide (Fed) contents with island age, but opposed the trends observed for SOC and non-crystalline constituents (Alo+0.5Feo) across the three studied islands (Figure 2; Table 1). SOM and non-crystalline materials have been found beneficial for soil micro- and macroaggregate stability in many cases (e.g., Tisdall and Oades, 1982; Shoji et al., 1993). However, in our study, the stabilizing effects of these soil constituents could not be verified for macroaggregates (250–2000 µm) subjected to high ultrasonic energy levels (1500 J ml−1). Our results rather suggest that macroaggregate stability in the studied topsoils is enhanced by bonding among and between crystalline Fe oxides and clay particles (Six et al., 2000). Indeed, we observed the formation of a pseudo-sand microstructure in soils of the oldest studied island, San Cristóbal, which is a typical feature in highly weathered, oxide-rich soils (IUSS Working Group WRB, 2015).
The significantly lower CEC levels in the soils of San Cristóbal, compared to the younger islands (Figure 2) could be attributable to changes in clay mineralogy from non-crystalline constituents such as allophanes to low-activity clays, notably kaolinite (Candra et al., 2021) and increasing crystallinity of Fe oxides (Dinter et al., 2020) providing less surface area (Sollins et al., 1988), in addition to decreasing pH and declining SOM contents with increasing soil age. Similarly, high CEC levels in young Andosols on Lanzarote Island have been linked to the formation of non-crystalline weathering products and the accumulation of humic substances in the early stage of soil formation (Jahn and Stahr, 1996). In our study, the soils’ base saturation dropped in conjunction with pH and EC (Figure 2; Table 1), which reflects the progressive leaching of base cations with soil age and increasing hotspot distance, as also shown along component 1 in the PCA (Figure 5, top).
Concentrations of M3-extractable Ca decreased significantly with increasing island age (Figure 3), likely due to the element’s high mobility and the continuous exposure to leaching processes. Rapid dissolution of non-hydrolyzing cations (e.g., Ca, Mg) in the early weathering stages of volcanic parent materials and subsequent leaching was also observed in a chronosequence in Hawaii (Chorover et al., 2004). Even though the base cations Mg, K and Na showed a decreasing tendency between the youngest and oldest island, the highest mean values were observed for the intermediate-aged island, Santa Cruz, which was most strongly pronounced (and statistically significant) for M3-extractable Na. This circumstance may be attributable to irrigation with brackish water containing high levels of dissolved salts, which is a wide-spread practice on that island. Also, the application of fertilizers and the common use of agrochemicals may be reflected in the distribution of M3-extractable Mg, K, P, and especially Cu along and within the islands, showing highest mean values and considerable outliers on Santa Cruz Island. A recent census report points out that Santa Cruz is the most intensively farmed island of Galápagos, with almost twice as much annual expenditures on fertilizers and pesticides as on Isabela and San Cristóbal Island combined (CGREG et al., 2014). M3-extractable Zn showed a sharp decrease with increasing island age similar to Ca, which is probably related to the relatively high mobility and progressive leaching of Zn associated with lower soil pH values (Adriano, 2001), especially on the oldest studied island. Also M3-extractable Fe progressively decreased with increasing island age, while dithionite-extractable Fe sharply increased (Figure 3; Table 1). This is because the M3 reactant extracts only a fraction of Fe that corresponds to available and soluble Fe (Marcos et al., 1998) but does not extract crystalline Fe forms. The comparatively low concentrations of M3-extractable P on the oldest studied island, San Cristóbal (Figure 3), are probably related to its increasing occlusion in Al and Fe oxides (Walker and Syers, 1976). In addition, Rechberger et al. (2021) recently showed that increasing acidification enhanced phosphate retention in highly weathered Galápagos topsoils (≥165 ka). In contrast to the other M3-extractable elements, the levels of Mn were very low on the youngest studied island, Isabela, probably due to the soils early weathering stage with limited release of Mn from primary minerals, while this element became more available on the older islands (Figure 3).
Results from PCA based on M3-extractable element data from all islands (Figure 5, top) further illustrate the observed trends with soil age. Notably, the factor loadings of the mobile base cations Ca, Mg, K, Na and additionally Zn were located opposite of the factor hotspot distance on PC1. Furthermore, the PCA results suggest that the observed segregation between San Cristóbal and the two younger islands on PC1 may be influenced by substantial differences in key soil properties on the oldest studied island. In particular, decreased pH and CEC levels and the transition from amorphous to crystalline Fe forms as a consequence of progressing pedogenic development may have contributed to the reduced overall nutrient availability on San Cristóbal.
Trends With Elevation/Climate
While soil physical characteristics did not show any clear trends with elevation in the agricultural zones of the three studied islands (data not shown), soil pH (and, hence, base saturation; data not shown) and several of the M3-extractable nutrients varied significantly with altitude (Figure 4, Supplementary Tables S4, S5), which is likely an effect of altitudinal variations in precipitation. For all three of the studied Galápagos islands, soil pH decreased with increasing elevation, which is likely attributable to enhanced leaching of base cations and, in case of the older islands, also to the solubilization of Al, driven by the humid moisture regime in the highlands (Vitousek and Chadwick, 2013). Furthermore, enhanced soil weathering with elevation is also indicated by increasing Fe oxide content, as Dinter et al. (2020) found dithionite-extractable Fe (Fed) in correspondence with the highest elevation group (>500 m a.s.l.) factor scores in their PCA of all three islands.
The elemental assemblage of a particular soil depends on the degree of soil development, the respective mobility of the elements and the specific environmental conditions (Martínez Cortizas et al., 2003), hence, a complex interplay of several factors. This was also shown in the present study when comparing the trends of M3-extractable Ca and Mn along the altitudinal gradients of the youngest and oldest studied islands, Isabela and San Cristóbal, respectively (Figure 4, Supplementary Tables S4, S5). M3-extractable Ca decreased with increasing elevation on both islands, which is likely reflective of its enhanced leaching owing to rising precipitation levels with altitude. Similar findings on the depletion of mobile base cations have been reported for climate gradients in New Zealand (Dixon et al., 2016) and Hawaii (Vitousek and Chadwick, 2013). By contrast, M3-extractable Mn showed very distinct patterns for Isabela and San Cristóbal. On the youngest studied island, M3-extractable Mn was at a very low level (mean: 8.7 mg kg−1) but became increasingly available with elevation and increasing precipitation likely due to weathering-induced release from primary minerals. On the oldest studied island, where primary minerals have largely been weathered, M3-extractable Mn levels were much higher (mean: 85.8 mg kg−1) but decreased with increasing elevation, likely due to long-term leaching losses under acidic conditions. On the oldest studied island, San Cristóbal, most of the M3-extractable element concentrations (K, Ca, Mg, Na, Mn, Zn and Cu) decreased significantly with increasing elevation, while M3-extractable Fe increased significantly (rs 0.68, p < 0.001; Supplementary Table S5). This is probably related to the low pH values on San Cristóbal (25th percentile of soil pH in H2O: 4.8), particularly pronounced at higher elevations (Figure 4). The acidic conditions in conjunction with the humid environment likely enhance the availability of (M3-extractable) Fe. Significant negative correlation between M3-extractable Fe and soil pH was also found in a series of very heterogeneous soils (pH 2.7–8.4) of northwest Spain (Marcos et al., 1998). Results from PCA based on M3-extractable element data of the individual islands (Figure 5, middle and bottom) highlight the different mobility of the elements across the studied elevation/climate zones. In the case of Ca, the factor loadings were opposed to the factor elevation for both islands, while the factor loadings of Mn were opposed to the factor elevation only for San Cristóbal but closely related to elevation for Isabela Island.
When investigating the effects of climate on soil formation and soil (fertility) parameters, past climate variations need to be considered, especially for the older islands Santa Cruz and San Cristóbal. Vitousek and Chadwick (2013) suggested paleoclimates to be potentially influential on observed differences in soil geochemistry on the Hawaiian archipelago. Findings from pollen analysis in sediments of El Junco crater lake on San Cristóbal suggest that the soils of the Galápagos archipelago have been subjected to long dry periods broadly corresponding to glaciation in the northern hemisphere, interrupted by moister periods during interglacial times (Colinvaux, 1972). However, in our study, differences in soil parameters related to island age clearly outweigh climate-driven altitudinal variations within the agricultural zones.
Implications for Soil Functioning and Sustainable Agricultural Management
In the preceding sections, we reported about pronounced soil differences depending on island age and climate zone, which affect soil functioning and entail distinct implications for agricultural management. Moreover, climate change is predicted to lead to moister conditions on the archipelago (Rial et al., 2017), probably causing a downward expansion of the humid zone into currently drier areas. Increased precipitation levels may lead to enhanced nutrient leaching, and more extreme weather events could increase the risk of drought and soil erosion. Profound knowledge of the soil properties on the different Galápagos islands is therefore essential not only to ensure the sustainability of current land management and prevent soil degradation, but also to better adapt to changing conditions in the future.
In the following sections, implications for soil functioning and sustainable agricultural management are derived from the studied soil parameters for the three islands under investigation. For M3-extractable elements, different classifications of critical concentrations of macro- and micronutrients have been proposed (e.g., Zhang et al., 2014; Zbíral, 2016). Application of such critical concentrations to the agricultural soils of Galápagos must be conducted with caution, because they may vary according to soil type, climate and crop to be grown (Zhang et al., 2014) and should ideally be calibrated with field experiments to assess plant responses. Nevertheless, they may still be useful for relative comparisons between the islands and to put the results from Galápagos into a broader context.
Isabela Island (Young Soils)
The agricultural soils of Isabela show features commonly found in young volcanic soils and generally favorable for crop production (Wada, 1985; Shoji et al., 1993). These include high SOC contents (mean: 15.9%), silt loam texture, low BD (mean: 0.51 g cm−3) and, hence, high porosity. Topsoil PAW on Isabela Island (mean: 12.5 mm in 0–20 cm depth) was similar as for the older studied islands. However, less solum thickness (20–50 cm) compared to the other islands (Zehetner et al., 2020) may represent restrictions for water availability and agricultural production in some areas of Isabela. Nevertheless, in many cases, plant roots can reach the frequently encountered underlying paleosols for additional water and nutrient supply on this island (Zehetner et al., 2020). The high porosity of Isabela’s soils implies the risk of leaching losses of nutrients as well as agrochemical residues and other contaminants. High gravel contents consisting mainly of scoriaceous lapilli (mean: 57.5%) further contribute to high hydraulic conductivity but may also reduce the risk of soil compaction (Rücknagel et al., 2013). As a consequence of the comparatively low macroaggregate stability, the agricultural soils of Isabela Island are likely more prone to erosion (by water and wind) compared to the other studied islands. This is especially important in the face of climate change with increasing magnitudes of extreme events, and calls for adaptation measures to prevent soil erosion.
Total N (mean: 14.5 g kg−1) was significantly higher on Isabela than on the other islands, probably related to the high SOC contents. However, the mineralization of organic matter and subsequent release of available N is probably limited by strong SOM stabilization due to high amounts of non-crystalline constituents in the young Andosols (Torn et al., 1997). The M3-extractable base cations Ca, Mg and K are rated high for the great majority of samples from Isabela Island according to Zhang et al. (2014). The relatively high pH (mean: 6.5 in H2O) and base saturation (mean: 84.9%) in conjunction with high CEC (mean: 348 mmolc kg−1) indicate high acid buffering and nutrient retention capacity. A decrease of soil pH along with (leaching) losses of M3-extractable Ca was observed for higher elevations on Isabela Island, while Mn became more readily available with elevation (Figure 4). Nevertheless, M3-extractable Mn is rated low in all soils of Isabela according to Zbíral (2016), while Cu levels are, on average, rated medium and Zn levels high. Interestingly, M3-extractable Fe concentrations are rated medium for all analyzed samples from Isabela (mean: 85.1 mg kg−1), but are, on average, rated low for the older studied islands. We assume that the higher availability of Fe on Isabela, in spite of comparatively high soil pH, is related to the fact that Fe occurs predominantly in amorphous oxides and organic compounds in the young Andosols of this island, while crystalline Fe oxides prevail on the older islands (Candra et al., 2021). Although strong P sorption is reported to be one of the major factors limiting plant growth in young volcanic soils (Dahlgren et al., 2004), M3-extractable P levels in the agricultural soils of Isabela are rated low for 42% of the studied soils but medium to high for the majority of the soils (Zhang et al., 2014). This is likely attributable to the relatively high pH values of these soils. Indeed, many studies reported P availability to peak at pH 6.5 (Penn and Camberato, 2019). Nevertheless, fertilization recommendations should account for potentially strong sorption of phosphate to non-crystalline materials in young volcanic soils, as recently shown by Rechberger et al. (2021) also for topsoils of Isabela Island.
Santa Cruz Island (Intermediate-Aged Soils)
Many of the investigated soil parameters displayed relatively high variability for Santa Cruz, reflecting the island’s intermediate position with volcanic deposits ranging from approx. 25 to 200 ka on the southern flank (White et al., 1993; Schwartz, 2014), where the agricultural zone is located. We found very heterogeneous soil texture, rather low BD (mean: 0.83 g cm−3), medium to high macroaggregate stability, high CEC (mean: 332 mmolc kg−1) and, on average, high levels of most of the studied M3-extractable nutrients (K, Ca, Mg, Zn, Cu). Topsoil PAW (mean: 15.8 mm in 0–20 cm depth) showed marked spatial variation, as displayed in Supplementary Figure S7, which is likely associated with the strong heterogeneity of the soil parent materials (i.e. a mosaic of different lava flows and scoria deposits of varying ages) and, hence, large variations in gravel content and soil texture on this island (Figure 2). Also, the soils macroaggregate stability varied widely, but was considerably higher than on the youngest island, indicating no imminent risk of erosion for most of the studied soils, although the steep slopes of some highland farms near Cerro Crocker (Adelinet et al., 2008) may benefit from measures to stabilize the soil surface against erosion. SOC and total N contents (means: 6.6% and 5.7 g kg−1, respectively) as well as soil pH (mean: 6.0 in H2O) and base saturation (mean: 73.1%) were significantly lower than on Isabela Island, but still relatively high and conducive to productive agriculture.
As Santa Cruz is the agriculturally most intensively used island of the archipelago (CGREG et al., 2014), extreme outliers of M3-extractable P and Zn as well as the observed peak in M3-extractable Cu among the three islands (mean: 5.1 mg kg−1) may be related to agrochemical inputs, especially to the application of phosphate fertilizers and fungicides (Oorts, 2013; Cabral Pinto et al., 2015; Dinter et al., 2021). Elevated levels of M3-extractable Na, Mg and K, compared to the other studied islands, were observed in soils irrigated with brackish water on Santa Cruz. The highest EC values measured in the soils of Santa Cruz (max: 607 μS cm−1 in 1:10 water extracts; Table 1) indicate a beginning threat of salinization, as EC threshold levels (in saturated soil extracts) range from 1,000 to 2,500 μS cm−1 for the majority of vegetables (Almeida Machado and Serralheiro, 2017). On the other hand, the exchangeable sodium percentages in these soils (ESP max: 3.82%; Supplementary Table S1) do not indicate pronounced sodicity, as ESP values of >15% would be required to classify soils as sodic (USSL Staff, 1954). M3-extractable concentrations of Fe (mean: 55.7 mg kg−1, 75th percentile: 61.3 mg kg−1) were significantly lower compared to Isabela Island and are, on average, classified as low according to Zbíral (2016), which is probably related to progressing pedogenesis and crystallization of Fe oxides with island age. Moreover, levels of M3-extractable P (median: 15.5 mg kg−1) are rated low for the majority of the studied soils on Santa Cruz (Zhang et al., 2014). Hence, nutrient management strategies should especially target Fe and P in these soils. Besides their addition via fertilizers and/or manure, also mobilization strategies should be considered. Mobilization of P from inorganic compounds could be achieved, for instance, by increasing the ion competition on the sorption complex (exchangeable P) through the application of silicic acid (Schaller et al., 2020), and by cover-cropping P-capturing plants (e.g., buckwheat, lupine; Hallama et al., 2019), while biofertilization (e.g., with Bacillus megaterium phosphaticum) has been reported to facilitate microbial P solubilization from organic compounds (Alori et al., 2017). In addition, organic anions from dissolved organic matter can compete with phosphate ions for sorption sites and thereby increase plant-availability of P (Iyamuremye et al., 1996). Manipulating the rhizosphere (e.g. via water regulation, root fertilization) and crop management strategies such as intercropping and enhanced crop varieties may be promising measures to address Fe deficiencies in the respective soils (Zuo and Zhang, 2011).
San Cristóbal Island (Old Soils)
The studied soils of San Cristóbal showed typical features of highly weathered soils, including clayey texture (mean clay content: 72.8%) with little gravel remaining, high macroaggregate stability, rather low pH (in H2O; mean: 5.6, 25th percentile: 4.8) and base saturation (mean: 59.3%, 25th percentile: 30.1%), as well as lower SOC and total N contents (means: 4.5% and 3.3 g kg−1, respectively) compared to the younger and intermediate-aged islands. Soil BD was higher compared to the islands Isabela and Santa Cruz, but still predominantly <1 g cm−3 and, hence, relatively low and indicative of high porosity. This, and the high macroaggregate stability (Figure 2) related to the commonly found pseudo-sand structure in these soils (IUSS Working Group WRB, 2015) are likely to contribute to rapid water infiltration and percolation in spite of the very high clay contents, which reduces the risk of surface runoff and soil erosion. The clayey soils of San Cristóbal Island are inherently more prone to compaction through heavy machinery or intensive grazing than the soils of the younger and intermediate-aged islands. However, such management practices are currently not common on the Galápagos Islands. The soils CEC (mean: 165 mmolc kg−1) was significantly lower than on the other studied islands, which entails reduced nutrient retention and acid buffering capacity. Aluminum toxicity in soil may occur at pH < 5.5 (Evans and Kamprath, 1970), which was found for many soils of San Cristóbal, in particular in the humid highlands of this island.
The advanced weathering stage of San Cristóbal’s soils has significant implications for their nutrient status. Our results show that (M3-extractable) nutrient availability has been lowered compared to the younger studied islands, probably through progressive leaching of mobile cations in combination with low soil pH, especially in the higher-elevation zones (Dinter et al., 2021), as well as through strong bonding and occlusion of anionic nutrients like P commonly found in acidic soils rich in Al and Fe oxides (Cross and Schlesinger, 1995; Sanchez et al., 2003). Indeed, the M3-extractable P levels in the studied soils of San Cristóbal are predominantly rated low (Zhang et al., 2014), with a 75th percentile value of 11 mg kg−1 (Supplementary Table S2). The levels of the M3-extractable base cations Ca, K and Mg are, on average, rated high according to Zhang et al. (2014), but approx. 25% of the studied samples are rated medium for Ca and low for K (25th percentile for Ca: 740 mg kg−1, and for K: 57 mg kg−1). For micronutrients, medium levels of M3-extractable Zn, Mn and Cu and low levels of Fe were, on average, found according to Zbíral (2016). Nevertheless, the M3-extractable concentrations of Zn and Cu are rated low for approx. 25% of the studied San Cristóbal soils (25th percentile for Zn: 1.3 mg kg−1, and for Cu: 1.7 mg kg−1). Many of the agricultural soils on San Cristóbal Island would benefit from measures to replenish nutrient stocks and increase SOM levels, such as the application of chicken dung (Nakamaru et al., 2000) and green manure, as well as the incorporation of crop residues and compost (Valarini et al., 2009). Liming of the low-pH soils would help to alleviate Al toxicity, to mobilize P by deprotonating variable charges on oxide surfaces and stimulating mineralization of soil organic P (Haynes, 1982), and also to restore the soils buffer-filter function.
Summary and Conclusion
Our results show that the age of the individual Galápagos islands has strong bearings on the soils natural fertility. We found increasing trends of clay content and Fe oxides, BD and macroaggregate stability with soil age, and decreasing trends of SOM, pH, CEC and base saturation. PAW contents (in 0–20 cm) were rather similar among the islands. Decreasing levels of M3-extractable base cations Ca, Mg, K and Na as well as P, Zn and Fe were observed from the youngest to the oldest studied island, while Mn concentrations increased with island age. M3-extractable Cu and Na peaked on the intermediate-aged island Santa Cruz, probably attributable to intensified agricultural activities, such as the application of agrochemicals and irrigation with brackish water, respectively. Trends along the elevation gradient within the agricultural areas of each island highlight the impact of varying precipitation regimes on soil weathering and nutrient leaching. While soil physical parameters were less influenced, pronounced changes with elevation were observed for pH, base saturation and M3-extractable nutrients.
Our study points out that the high degree of diversity in the tested soil properties and nutrient levels between and within the different Galápagos islands necessitates island-specific and climate-adapted soil management strategies to maintain soil functioning and, as a consequence, the sustainable provision of ecosystem services. A thorough account of the soils’ respective pedogenic stage and associated implications for their fertility status will be decisive for future agricultural management on the inhabited islands, considering food security and the unique ecological value of the archipelago.
Data Availability Statement
The raw data supporting the conclusions of this article will be made available by the authors, without undue reservation.
Author Contributions
FZ and MG contributed to conception and design of the study. MS, EV and NR contributed to laboratory analyses and data processing, and FZ validated the analytical results. MS performed the statistical analyses, visualized the results including GIS maps, and wrote the original draft of the manuscript. All authors contributed to manuscript revision, read, and approved the submitted version. FZ acquired the funding and supervised the project.
Funding
This study was conducted under research permit no. PC-60–16 of the Galápagos National Park Directorate (GNPD) and supported by the Prometeo Project of Ecuador’s Secretariat of Higher Education, Science, Technology and Innovation (SENESCYT).
Conflict of Interest
The authors declare that the research was conducted in the absence of any commercial or financial relationships that could be construed as a potential conflict of interest.
Publisher’s Note
All claims expressed in this article are solely those of the authors and do not necessarily represent those of their affiliated organizations, or those of the publisher, the editors and the reviewers. Any product that may be evaluated in this article, or claim that may be made by its manufacturer, is not guaranteed or endorsed by the publisher.
Acknowledgments
We wish to thank the GNPD for their help, especially Jorge Carrión, the former director, for supporting our research in Galápagos. Thank you to the Ecuadorian Ministry of Agriculture and Livestock (MAG) staff members Sandra García, René Ramírez, Ángeles Prado, José Antonio Rueda, Bárbara Ordoñez, César Vinueza, Romny Rodríguez, Hernán Simbaña, Tania Guamanquishpe and Francisco Cervantes for collecting the soil samples and completing the bulk density analysis. Thank you to the laboratory staff at BOKU University’s Institute of Soil Research for assistance with the laboratory analyses.
Supplementary Material
The Supplementary Material for this article can be found online at: https://www.frontiersin.org/articles/10.3389/fenvs.2021.788082/full#supplementary-material
References
Adelinet, M., Fortin, J., d’Ozouville, N., and Violette, S. (2008). The Relationship between Hydrodynamic Properties and Weathering of Soils Derived from Volcanic Rocks, Galapagos Islands (Ecuador). Environ. Geol. 56, 45–58. doi:10.1007/s00254-007-1138-3
Adhikari, K., and Hartemink, A. E. (2016). Linking Soils to Ecosystem Services - A Global Review. Geoderma 262, 101–111. doi:10.1016/j.geoderma.2015.08.009
Adriano, D. C. (2001). Trace Elements in Terrestrial Environments: Biogeochemistry, Bioavailability and Risks of Metals. 2nd Edition. New York: Springer, 867. doi:10.1007/978-0-387-21510-5
Alori, E. T., Glick, B. R., and Babalola, O. O. (2017). Microbial Phosphorus Solubilization and its Potential for Use in Sustainable Agriculture. Front. Microbiol. 8, 971. doi:10.3389/fmicb.2017.00971
Argus, D. F., Gordon, R. G., and DeMets, C. (2011). Geologically Current Motion of 56 Plates Relative to the No-Net-Rotation Reference Frame. Geochem. Geophys. Geosyst. 12, Q11001. doi:10.1029/2011GC003751
Blakemore, L. C., Searle, P. L., and Daly, B. K. (1987). Methods for Chemical Analysis of Soils, N.Z. Soil Bureau Scientific Report 80. New Zealand: N.Z. Soil Bureau, Lower Hutt.
Cabral Pinto, M. M. S., Ferreira da Silva, E., Silva, M. M. V. G., and Melo-Gonçalves, P. (2015). Heavy Metals of Santiago Island (Cape Verde) Top Soils: Estimated Background Value Maps and Environmental Risk Assessment. J. Afr. Earth Sci. 101, 162–176. doi:10.1016/j.jafrearsci.2014.09.011
Candra, I. N., Gerzabek, M. H., Ottner, F., Tintner, J., Wriessnig, K., and Zehetner, F. (2019). Weathering and Soil Formation in Rhyolitic Tephra along a Moisture Gradient on Alcedo Volcano, Galápagos. Geoderma 343, 215–225. doi:10.1016/j.geoderma.2019.01.051
Candra, I. N., Gerzabek, M. H., Ottner, F., Wriessnig, K., Tintner, J., Schmidt, G., et al. (2021). Soil Development and Mineral Transformations along a One‐million‐year Chronosequence on the Galápagos Islands. Soil Sci. Soc. Am. J. 85, 2077–2099. doi:10.1002/saj2.20317
Chadwick, O. A., Gavenda, R. T., Kelly, E. F., Ziegler, K., Olson, C. G., Elliott, W. C., et al. (2003). The Impact of Climate on the Biogeochemical Functioning of Volcanic Soils. Chem. Geology. 202, 195–223. doi:10.1016/j.chemgeo.2002.09.001
Chorover, J., Amistadi, M. K., and Chadwick, O. A. (2004). Surface Charge Evolution of Mineral-Organic Complexes During Pedogenesis in Hawaiian Basalt. Geochimica et Cosmochimica Acta 68, 4859–4876. doi:10.1016/j.gca.2004.06.005
Cross, A. F., and Schlesinger, W. H. (1995). A Literature Review and Evaluation of the Hedley Fractionation: Applications to the Biogeochemical Cycle of Soil Phosphorus in Natural Ecosystems. Geoderma 64 (3), 197–214. doi:10.1016/0016-7061(94)00023-4
Dahlgren, R. A., Saigusa, M., and Ugolini, F. C. (2004). The Nature, Properties and Management of Volcanic Soils. Adv. Agron. 82, 113–182. doi:10.1016/S0065-2113(03)82003-5
Delmelle, P., Opfergelt, S., Cornelis, J.-T., and Ping, C.-L. (2015). “Volcanic Soils,” in The Encyclopedia of Volcanoes. Editor H. Sigurdssonet al. (Cambridge, Massachusetts: Academic Press), 1253–1264. doi:10.1016/B978-0-12-385938-9.00072-9
Delvaux, B., Herbillon, A. J., and Vielvoye, L. (1989). Characterization of a Weathering Sequence of Soils Derived from Volcanic Ash in Cameroon: Taxonomic, Mineralogical and Agronomic Implications. Geoderma 45, 375–388. doi:10.1016/0016-7061(89)90017-7
Dinter, T. C., Gerzabek, M. H., Puschenreiter, M., Strobel, B. W., Couenberg, P. M., and Zehetner, F. (2021). Heavy Metal Contents, Mobility and Origin in Agricultural Topsoils of the Galápagos Islands. Chemosphere 272, 129821. doi:10.1016/j.chemosphere.2021.129821
Dinter, T. C., Gerzabek, M. H., Puschenreiter, M., Strobel, B. W., Strahlhofer, M., Couenberg, P. M., et al. (2020). Changes in Topsoil Characteristics with Climate and Island Age in the Agricultural Zones of the Galápagos. Geoderma 376, 114534. doi:10.1016/j.geoderma.2020.114534
Dixon, J. L., Chadwick, O. A., and Vitousek, P. M. (2016). Climate‐driven Thresholds for Chemical Weathering in Postglacial Soils of New Zealand. J. Geophys. Res. Earth Surf. 121, 1619–1634. doi:10.1002/2016JF003864
Evans, C. E., and Kamprath, E. J. (1970). Lime Response as Related to Percent Al Saturation, Solution Al, and Organic Matter Content. Soil Sci. Soc. Am. J. 34, 893–896. doi:10.2136/sssaj1970.03615995003400060023x
Geist, D. J., McBirney, A. R., and Duncan, R. A. (1986). Geology and Petrogenesis of Lavas from San Cristobal Island, Galapagos Archipelago. Geol. Soc. Am. Bull. 97, 555. doi:10.1130/0016-7606(1986)97<555:GAPOLF>2.0.CO;2
Gerzabek, M. H., Bajraktarevic, A., Keiblinger, K., Mentler, A., Rechberger, M., Tintner, J., et al. (2019). Agriculture Changes Soil Properties on the Galápagos Islands - Two Case Studies. Soil Res. 57, 201. doi:10.1071/SR18331
Guézou, A., Trueman, M., Buddenhagen, C. E., Chamorro, S., Guerrero, A. M., Pozo, P., et al. (2010). An Extensive Alien Plant Inventory from the Inhabited Areas of Galapagos. PLOS ONE 5, e10276. doi:10.1371/journal.pone.0010276
Hallama, M., Pekrun, C., Lambers, H., and Kandeler, E. (2019). Hidden Miners - the Roles of Cover Crops and Soil Microorganisms in Phosphorus Cycling through Agroecosystems. Plant Soil 434, 7–45. doi:10.1007/s11104-018-3810-7
Havlin, J. L. (2005). “Fertility,” in Encyclopedia of Soils in the Environment. Editor D. Hillel (Amsterdam: Academic Press), 2, 10–20.
Haynes, R. J. (1982). Effects of Liming on Phosphate Availability in Acid Soils. Plant Soil 68, 289–308. doi:10.1007/BF02197935
Hooft, E. E. E., Toomey, D. R., and Solomon, S. C. (2003). Anomalously Thin Transition Zone beneath the Galápagos Hotspot. Earth Planet. Sci. Lett. 216, 55–64. doi:10.1016/S0012-821X(03)00517-X
INGALA-PRONAREG-ORSTOM (1987). Islas Galápagos: Mapa de Formaciones Vegetales. Instituto Geográfico Militar, Quito, Ecuador.
Itow, S. (2003). Zonation Pattern, Succession Process and Invasion by Aliens in Species-Poor Insular Vegetation of the Galápagos Islands. Glob. Environ. Res. 7, 39–58.
IUSS Working Group WRB (2015). “International Soil Classification System for Naming Soils and Creating Legends for Soil Maps,” in World Soil Resources Reports, 106. Rome, Italy: FAO.
Iyamuremye, F., Dick, R. P., and Baham, J. (1996). Organic Amendments and Phosphorus Dynamics: III. Phosphorus Speciation. Soil Sci. 161, 444–451. doi:10.1097/00010694-199607000-00004
Izurieta, A., Delgado, B., Moity, N., Calvopiña, M., Cedeño, I., Banda-Cruz, G., et al. (2018). A Collaboratively Derived Environmental Research Agenda for Galápagos. Pac. Conserv. Biol. 24, 168. doi:10.1071/PC17053
Jahn, R., and Stahr, K. (1996). Development of Soils and Site Qualities on Basic Volcanoclastics with Special Reference to the Semiarid Environment of Lanzarote, Canary Islands, Spain. Revista Mexicana de Ciencias Geológicas 13, 104–112.
Jahn, R., Zarei, M., and Stahr, K. (1987). Formation of Clay Minerals in Soils Developed from Basic Volcanic Rocks under Semiarid Climatic Conditions in Lanzarote, Spain. Catena 14, 359–368. doi:10.1016/0341-8162(87)90027-0
Klute, A. (1986). “Water Retention: Laboratory Methods,” in Methods of Soil Analysis. Part 1. Physical and Mineralogical Methods. Editor A. Klute. 2nd ed. (Madison, Wisconsin: ASA and SSSA), 635–660.
Lasso, L., Espinosa, J., Espinosa, J., Moreno, J., and Bernal, G. (2018). “Soils from the Galapagos Islands,” in The Soils of Ecuador Editor J. Espinosa et al. World Soils Book Series (Cham, Switzerland: Springer International Publishing), 139–150.
Levy, G. J., and Shainberg, I. (2005). “Sodic Soils,” in Encyclopedia of Soils in the Environment. Editor D. Hillel (Amsterdam: Academic Press), Vol. 3, 504–513. doi:10.1016/B0-12-348530-4/00218-6
Lowe, D. J. (1986). “Controls on the Rates of Weathering and Clay Mineral Genesis in Airfall Tephras: A Review and New Zealand Case Study,” in Rates of Chemical Weathering of Rocks and Minerals. Editors S. M. Colman, and D. P. Dethier (Orlando, FL: Academic Press), 265–330.
Machado, R., and Serralheiro, R. (2017). Soil Salinity: Effect on Vegetable Crop Growth. Management Practices to Prevent and Mitigate Soil Salinization. Horticulturae 3, 30. doi:10.3390/horticulturae3020030
Marcos, M. L. F., Alvarez, E., and Monterroso, C. (1998). Aluminum and Iron Estimated by Mehlich‐3 Extractant in Mine Soils in Galicia, Northwest Spain. Commun. Soil Sci. Plant Anal. 29, 599–612. doi:10.1080/00103629809369970
Martínez Cortizas, A., Garcia-Rodeja Gayoso, E., Nóvoa Muñoz, J. C., Pontevedra Pombal, X., Buurman, P., and Terribile, F. (2003). Distribution of Some Selected Major and Trace Elements in Four Italian Soils Developed from the Deposits of the Gauro and Vico Volcanoes. Geoderma 117, 215–224. doi:10.1016/S0016-7061(03)00124-1
Mehlich, A. (1984). Mehlich 3 Soil Test Extractant: A Modification of Mehlich 2 Extractant. Commun. Soil Sci. Plant Anal. 15, 1409–1416. doi:10.1080/00103628409367568
Mehra, O. P., and Jackson, M. L. (1958). Iron Oxide Removal from Soils and Clays by a Dithionite-Citrate System Buffered with Sodium Bicarbonate. Clays and Clay Minerals 7, 317–327. doi:10.1346/ccmn.1958.0070122
Mentler, A., Mayer, H., Straus, P., and Blum, W. E. H. (2004). Characterisation of Soil Aggregate Stability by Ultrasonic Dispersion. Int. Agrophysics 18, 39–45.
Mikutta, R., Schaumann, G. E., Gildemeister, D., Bonneville, S., Kramer, M. G., Chorover, J., et al. (2009). Biogeochemistry of Mineral-Organic Associations across a Long-Term Mineralogical Soil Gradient (0.3-4100 kyr), Hawaiian Islands. Geochimica et Cosmochimica Acta 73, 2034–2060. doi:10.1016/j.gca.2008.12.028
Nakamaru, Y., Nanzyo, M., and Yamasaki, S.-i. (2000). Utilization of Apatite in Fresh Volcanic Ash by Pigeonpea and Chickpea. Soil Sci. Plant Nutr. 46, 591–600. doi:10.1080/00380768.2000.10409124
Ngewoh, Z. S., Taylor, R. W., and Shuford, J. W. (1989). Exchangeable Cations and CEC Determinations of Some Highly Weathered Soils. Commun. Soil Sci. Plant Anal. 20, 1833–1855. doi:10.1080/00103628909368187
O’Connor Robinson, M., Selfa, T., and Hirsch, P. (2018). Navigating the Complex Trade-Offs of Pesticide Use on Santa Cruz Island, Galapagos. Soc. Nat. Resour. 31, 232–245. doi:10.1080/08941920.2017.1382625
Oorts, K. (2013). “Copper,” in Heavy Metals in Soils, Environmental Pollution. Editor B. J. Alloway (Dordrecht: Springer Netherlands), 367–394. doi:10.1007/978-94-007-4470-7_13
Penn, C., and Camberato, J. (2019). A Critical Review on Soil Chemical Processes that Control How Soil pH Affects Phosphorus Availability to Plants. Agriculture 9, 120. doi:10.3390/agriculture9060120
Perry, R. (1984). “Chapter 1: The Islands and Their History,” in Galapagos, Key Environments. Editor R. Perry. 1st ed. (Oxford, New York: Pergamon Press in collaboration with the International Union for Conservation of Nature and Natural Resources), 1–14.
Soil Survey Staff (2014). “Kellogg Soil Survey Laboratory Methods Manual. Soil Survey Investigations Report No. 42, ver. 5.0. National Soil Survey Center, Natural Resources Conservation Service, U.S. Department of Agriculture, Lincoln, NE.
Rechberger, M. V., Zehetner, F., and Gerzabek, M. H. (2021). Phosphate Sorption‐Desorption Properties in Volcanic Topsoils along a Chronosequence and a Climatic Gradient on the Galápagos Islands. J. Plant Nutr. Soil Sci. 184, 479–491. doi:10.1002/jpln.202000488
Reynolds, R. W., Geist, D., and Kurz, M. D. (1995). Physical Volcanology and Structural Development of Sierra Negra Volcano, Isabela Island, Galápagos Archipelago. Geol. Soc. Am. Bull. 107, 1398–1410. doi:10.1130/0016-7606(1995)107<1398:pvasdo>2.3.co;2
Rial, M., Martínez Cortizas, A., Taboada, T., and Rodríguez-Lado, L. (2017). Soil Organic Carbon Stocks in Santa Cruz Island, Galapagos, under Different Climate Change Scenarios. Catena 156, 74–81. doi:10.1016/j.catena.2017.03.020
Rücknagel, J., Götze, P., Hofmann, B., Christen, O., and Marschall, K. (2013). The Influence of Soil Gravel Content on Compaction Behaviour and Pre-compression Stress. Geoderma 209-210, 226–232. doi:10.1016/j.geoderma.2013.05.030
Sanchez, P. A., Palm, C. A., and Buol, S. W. (2003). Fertility Capability Soil Classification: A Tool to Help Assess Soil Quality in the Tropics. Geoderma 114, 157–185. doi:10.1016/S0016-7061(03)00040-5
Santo, L. T., and Tsuji, G. Y. (1977). Soil Bulk Density and Water Content Measurements by Gamma-Ray Attenuation Techniques. Tech. Bull. 98, 1–20.
Schaller, J., Frei, S., Rohn, L., and Gilfedder, B. S. (2020). Amorphous Silica Controls Water Storage Capacity and Phosphorus Mobility in Soils. Front. Environ. Sci. 8, 94. doi:10.3389/fenvs.2020.00094
Schwartz, D. (2014). Volcanic, Structural, and Morphological History of Santa Cruz Island, Galápagos Archipelago. Moscow, ID: Master's Thesis, University of Idaho.
Schwertmann, U. (1964). Differenzierung der Eisenoxide des Bodens durch Extraktion mit Ammoniumoxalat-Lösung. Z. Pflanzenernaehr. Dueng. Bodenk. 105, 194–202. doi:10.1002/jpln.3591050303
Shen, J., Yuan, L., Zhang, J., Li, H., Bai, Z., Chen, X., et al. (2011). Phosphorus Dynamics: From Soil to Plant. Plant Physiol. 156, 997–1005. doi:10.1104/pp.111.175232
Shoji, S., Nanzyo, M., and Dahlgren, R. (1993). Ash Soils: Genesis, Properties, and utilization. Developments in Soil Science. New York, Amsterdam: Elsevier.
Six, J., Elliott, E. T., and Paustian, K. (2000). Soil Structure and Soil Organic Matter: II. A Normalized Stability Index and the Effect of Mineralogy. Soil Sci. Soc. Am. J. 64, 1042–1049. doi:10.2136/sssaj2000.6431042x
Snell, H., and Rea, S. (1999). The 1997–98 El Niño in Galápagos: Can 34 Years of Data Estimate 120 Years of Pattern? Noticias de Galápagos 60, 11–20.
Sollins, P., Robertson, G. P., and Uehara, G. (1988). Nutrient Mobility in Variable- and Permanent-Charge Soils. Biogeochemistry 6, 181–199. doi:10.1007/bf02182995
Stoops, G. (2013). A Micromorphological Evaluation of Pedogenesis on Isla Santa Cruz (Galápagos). Span. J. Soil Sci. 3, 14–37. doi:10.3232/SJSS.2013.V3.N2.02
Tabatabai, M. A., and Bremner, J. M. (1991). “Automated Instruments for Determination of Total Carbon, Nitrogen, and Sulfur in Soils by Combustion Techniques,” in Soil Analysis. Editor K. A. Smith (New York: Marcel Dekker), 261–286.
Taboada, T., Rodríguez-Lado, L., Ferro-Vázquez, C., Stoops, G., and Martínez Cortizas, A. (2016). Chemical Weathering in the Volcanic Soils of Isla Santa Cruz (Galápagos Islands, Ecuador). Geoderma 261, 160–168. doi:10.1016/j.geoderma.2015.07.019
Tisdall, J. M., and Oades, J. M. (1982). Organic Matter and Water-Stable Aggregates in Soils. J. Soil Sci. 33, 141–163. doi:10.1111/j.1365-2389.1982.tb01755.x
Torn, M. S., Trumbore, S. E., Chadwick, O. A., Vitousek, P. M., and Hendricks, D. M. (1997). Mineral Control of Soil Organic Carbon Storage and Turnover. Nature 389, 170–173. doi:10.1038/38260
Trueman, M., and D’Ozouville, N. (2010). Characterizing the Galapagos Terrestrial Climate in the Face of Global Climate Change. Galapagos Res. 67, 26–37.
Tye, A., Snell, H. L., Peck, S. B., and Andersen, H. (2002). “Outstanding Terrestrial Features of the Galapagos Archipelago,” in A Biodiversity Vision for the Galapagos Islands. Editor R. Bensted-Smith (Puerto Ayora, Galápagos: Charles Darwin Foundation and World Wildlife Fund).
USSL Staff (1954). Diagnosis and Improvement of Saline and Alkali Soils. Washington DC, USA: USDA Handbook No 60, 160.
Valarini, P. J., Curaqueo, G., Seguel, A., Manzano, K., Rubio, R., Cornejo, P., et al. (2009). Effect of Compost Application on Some Properties of a Volcanic Soil from Central South Chile. Chilean J. Agric. Res. 69. doi:10.4067/S0718-58392009000300015
Villa, Á., and Segarra, P. (2010). “Changes in Land Use and Vegetative Cover in the Rural Areas of Santa Cruz and San Cristobal,” in Galapagos Report 2009-2010. (Puerto Ayora, Galápagos, Ecuador. Puerto Ayora: Charles Darwin Foundation and Galápagos National Park and Governing Council of Galápagos), 85–91.
Vitousek, P. M., and Chadwick, O. A. (2013). Pedogenic Thresholds and Soil Process Domains in Basalt-Derived Soils. Ecosystems 16, 1379–1395. doi:10.1007/s10021-013-9690-z
Vitousek, P. M. (1994). Potential Nitrogen Fixation during Primary Succession in Hawai`i Volcanoes National Park. Biotropica 26, 234–240. doi:10.2307/2388844
Wada, K. (1985). “The Distinctive Properties of Andosols,” in Soil Restoration. Editors R. Lal, and B. A. Stewart (New York: Springer), 173–229. doi:10.1007/978-1-4612-5088-3_4
Walker, T. W., and Syers, J. K. (1976). The Fate of Phosphorus during Pedogenesis. Geoderma 15, 1–19. doi:10.1016/0016-7061(76)90066-5
Watson, J., Trueman, M., Tufet, M., Henderson, S., and Atkinson, R. (2010). Mapping Terrestrial Anthropogenic Degradation on the Inhabited Islands of the Galapagos Archipelago. Oryx 44, 79–82. doi:10.1017/S0030605309990226
White, W. M., McBirney, A. R., and Duncan, R. A. (1993). Petrology and Geochemistry of the Galápagos Islands: Portrait of a Pathological Mantle Plume. J. Geophys. Res. 98, 19533–19563. doi:10.1029/93jb02018
Zaman, M., Shahid, S. A., and Heng, L. (2018). “Irrigation Water Quality,” in Guideline for Salinity Assessment, Mitigation and Adaptation Using Nuclear and Related Techniques (Cham, Switzerland): Springer International Publishing), 113–131. doi:10.1007/978-3-319-96190-3_5
Zbíral, J. (2016). Determination of Plant-Available Micronutrients by the Mehlich 3 Soil Extractant - a Proposal of Critical Values. Plant Soil Environ. 62, 527–531. doi:10.17221/564/2016-PSE
Zehetner, F., Gerzabek, M. H., Shellnutt, J. G., Ottner, F., Lüthgens, C., Miggins, D. P., et al. (2020). Linking Rock Age and Soil Cover across Four Islands on the Galápagos Archipelago. J. South Am. Earth Sci. 99, 102500. doi:10.1016/j.jsames.2020.102500
Zehetner, F., and Miller, W. P. (2006). “Toward Sustainable Crop Production in Cotacachi: An Assessment of the Soils' Nutrient Status,” in Development with Identity: Community, Culture and Sustainability in the Andes. Editor R. E. Rhoades (Wallingford, UK and Cambridge, MA: CABI Publishing), 177–196. doi:10.1079/9780851999494.0177
Zhang, H., Hardy, D. H., Mylavarapu, R., and Wang, J. J. (2014). “Mehlich-3,” in Soil Test Methods from the Southeastern United States. Southern Cooperative Series Bulletin. Editor F. J. Sikora, 419, 101–110.
Keywords: volcanic ash soils, pedogenesis, Mehlich(III), soil structure, soil and water management, soil functions
Citation: Strahlhofer M, Gerzabek MH, Rampazzo N, Couenberg PM, Vera E, Salazar Valenzuela X and Zehetner F (2021) Soil Fertility Changes With Climate and Island Age in Galápagos: New Baseline Data for Sustainable Agricultural Management. Front. Environ. Sci. 9:788082. doi: 10.3389/fenvs.2021.788082
Received: 01 October 2021; Accepted: 12 November 2021;
Published: 07 December 2021.
Edited by:
Thomas Keller, Swedish University of Agricultural Sciences, SwedenReviewed by:
Jean-Thomas Cornelis, University of British Columbia, CanadaCalogero Schillaci, Joint Research Centre (Italy), Italy
Copyright © 2021 Strahlhofer, Gerzabek, Rampazzo, Couenberg, Vera, Salazar Valenzuela and Zehetner. This is an open-access article distributed under the terms of the Creative Commons Attribution License (CC BY). The use, distribution or reproduction in other forums is permitted, provided the original author(s) and the copyright owner(s) are credited and that the original publication in this journal is cited, in accordance with accepted academic practice. No use, distribution or reproduction is permitted which does not comply with these terms.
*Correspondence: Franz Zehetner, ZnJhbnouemVoZXRuZXJAYm9rdS5hYy5hdA==