- 1Mammal Research Institute, Department of Zoology and Entomology, University of Pretoria, Pretoria, South Africa
- 2Department of Economics, Universidad de Alcalá, Alcalá de Henares, Spain
South Africa is one of the most diverse countries in the world but the increase in agricultural, industrial and technological development to meet the needs of the growing human population has led to increased amounts of potentially toxic elements (PTEs) and other chemicals in the environment. As regional and global environmental processes influence local conditions to differing degrees, all organisms within a specific environment are exposed to highly complex, ill-defined PTE and chemical mixtures. Differences in feeding strategies within and between vertebrate trophic levels are likely to influence the degree to which individuals may be exposed to and affected by PTE presence. Using vertebrate faeces as a biological matrix, we investigate and compare quantitative differences in PTE concentrations in herbivorous, omnivorous and carnivorous terrestrial vertebrates from two protected areas in South African savannah. Of the eleven PTEs assessed [aluminium (Al), arsenic (As), barium (Ba), cadmium (Cd), chromium (Cr), mercury (Hg), lead (Pb), antimony (Sb), tin (Sn), strontium (Sr), and vanadium (V)], the highest concentrations of Al, As, Cr, Pb, Sn, and V were found in carnivores. General patterns were evident between groups at each site for specific elements, but absolute values for the same elements were site-specific. This is the first study to non-invasively examine and compare PTE concentrations in a variety of free-ranging mammalian wildlife occupying different trophic levels within South African protected areas. Our results confirm that all individuals across trophic levels within these sites are exposed to multiple and varied PTE mixtures on a continuous basis. Whether PTEs at these concentrations cause synergistic or antagonistic disruption of physiological and biological systems alone or in combination in free-ranging African wildlife species is still unclear and requires further investigation.
Introduction
Potentially toxic elements (PTEs) have no established biological or physiological function (Järup, 2003). Even when concentrations are too low to be individually effective, the combined action of multiple PTEs at low concentrations alone and in combination with other organic and synthetic chemicals can result in adverse effects (Rhind, 2009; Martin et al., 2021). Repeated exposures, particularly when the interval between exposures is brief, may result in cumulative storage of a substance and its subsequent increase in body burden until a permanent state of storage has been reached (Baynes et al., 2012). The sequential and simultaneous exposure of wildlife to mixtures of synthetic compounds and PTEs from direct, indirect, past or contemporary exposures that interact in additive or synergistic ways, can disrupt various physiological systems resulting in subsequent negative cascading effects (Kortenkamp and Faust, 2018; Martin et al., 2021).
The Global Assessment of Soil Pollution Report (FAO and UNEP, 2021) highlights that contamination of soils degrades soil structure and organic carbon stores, reduces terrestrial ecosystem resilience and affects the ability to protect and secure water quality. Trace elements originating from geogenic processes are naturally occurring constituents within the environment (Jaishankar et al., 2014). However, increasing anthropogenic activities have distinctly altered the geochemical cycles and the biochemical balance of PTEs within the environment (Rzymski et al., 2015). The differences in geochemical heterogeneity, coupled with gradients in precipitation, vegetation and climatic conditions result in the availability of trace elements at different spatial intensities at a local, regional and global scale (McNaughton and Georgiadis, 1986; Shorrocks and Bates, 2015). In addition, the chemical form and abundance at which an element is present in the environment influences its availability for uptake by an organism (Kabata-Pendias, 2011; Olaniran et al., 2013).
Protected areas are considered the cornerstone of biodiversity and conservation. As regional and global environmental processes influence local conditions to differing degrees, specific environments contain unique combinations of PTEs and other chemicals resulting from geogenic processes and surrounding activities (Webster et al., 2021a). It is reasonable to assume that all organisms within a specific environment are exposed to highly complex, ill-defined mixtures of PTEs and other synthetic compounds (Thrupp et al., 2018). Individual animal behaviour and species-specific physiology contribute substantially to potential exposure or bioaccumulation, particularly when lipophilic substances are involved (Clotfelter et al., 2004; Gall et al., 2015). Food web magnifications occur with each predator-prey interaction and can result in PTE trophic increases of 10,000 to 100,000 fold (Gobas, 2008). PTEs that accumulate in the bones (Pb) or adipose tissue (As, Cd, Hg, and Pb) can be remobilized during periods when the body’s demand for calcium increases (e.g., pregnancy and lactation). Early exposure to PTEs and other chemical contaminants during critical periods of development can manifest in adverse effects later in life (World Health Organisation/International Programme on Chemical Safety, 2002; World Health Organisation/United Nations Environment Programme, 2012; Chen et al., 2014). In terrestrial carnivores, successful hunts are often interspersed with periods of nutritional stress (Gulson et al., 2003; Hayward and Kerley, 2008). When lipid reserves are remobilised for energy, circulating PTEs can result in damage to organs and systems (World Health Organisation/International Programme on Chemical Safety, 2002).
The varied ecosystems, species richness and endemism of the biomes make South Africa one of the most diverse countries in the world (Driver et al., 2011). Pollution has however become a key threat to protected areas and resident vertebrates may be at risk of associated effects through the consumption of contaminated vegetation, prey items and drinking water (Reglero et al., 2008). Traditional methods used for toxicological risk assessment in wildlife are typically invasive, involving the sacrifice of live animals in laboratory studies (Egorova and Ananikov, 2017; Kenston et al., 2018), the chemical immobilization and handling of live animals during routine management, translocation or assessment procedures (Facimire et al., 1995; Naidoo et al., 2017) or the opportunistic collection of various matrices from carcasses after mass mortality or debilitation events (Richards et al., 2014; Ogada et al., 2016). Soft tissue is generally considered optimal for accurate identification of specific toxin(s)/pollutant(s), however, environmental factors such as temperature and humidity may cause desiccation of soft tissue, while insect or scavenger activity may hasten the decomposition of pollutant residues and the samples themselves. In this regard, the window for recovery, detection and identification of toxins can be extremely narrow (Richards et al., 2014). Historical assessments have also primarily focused on the assessment of single elements, aspects related to that element (e.g., lipophilicity) or the impact on a specific species or trophic level within the food web (Streit, 1992). More recent approaches have, however demonstrated that low dose exposures to contaminant mixtures are synergistic (Cobbina et al., 2015; Thrupp et al., 2018; Martin et al., 2021). Interactions between numerous PTEs and/or steroidal pharmaceuticals can therefore result in higher toxicities and greater effects than single element exposures at either high or low concentrations (Rhind, 2009; Kortenkamp et al., 2019).
Complex intrinsic and extrinsic dynamics including seasonal variations in PTE contamination within available resources, sex, age, life stage, and exposure to environmental stressors, can influence an individual’s ability to acquire and assimilate food resources and associated PTEs (Warne, 2014). Dietary intake is closely related to faecal concentrations, which in turn reflects actual utilization of resources (Böswald et al., 2018) making faeces a practical, non-invasive matrix for assessment. Faecal analysis has previously been used to assess stress-related and reproductive hormone concentrations (Kersey and Dehnhard, 2014; Webster et al., 2018; Palme, 2019), measure nutrient stress (Grant et al., 2000) and to quantify exposure to toxic substances (Gupta and Bakre, 2013; Richards et al., 2014; Celis et al., 2015; Sach et al., 2020) in various species of captive and free-ranging wildlife. As a consequence of distinct morphological and physiological differences between species, the partitioning of food sources within herbivore, omnivore and carnivore groups that facilitate the sharing of community resources within a specific protected area (Du Toit and Cumming, 1999) and the degrees to which PTE mixtures may adversely affect different trophic groups are still largely unknown.
Given the typically invasive measures associated with sample collection, management plans of South African wildlife reserves do not include consistent monitoring and assessment of the environment or biota for the presence or persistence of synthetic or trace element contaminants (Mpumalanga Tourism and Parks Agency, 2015; Spies, 2018). As a result, information related to PTE presence within specific protected areas and the various wildlife species that occur there has been restricted to pollution events and mass die offs (Ferreira and Pienaar, 2011; Woodborne et al., 2012). A broad assessment of environmental matrices within the two protected areas of interest in this study (Webster et al., 2021a) identify unique geological signatures within each reserve, but also highlight some PTE concentrations above acceptable limits outside of geological influence. Given these previously established concerns in environmental matrices, the need for further assessment in biological components of these systems became evident (Webster et al., 2021b). The current study aimed to investigate and compare quantitative differences of eleven PTEs (Al, As, Ba, Cd, Cr, Hg, Pb, Sb, Sn, Sr, and V) across trophic levels of terrestrial African vertebrates using faeces as a non-invasive analytical matrix.
Materials and Methods
Study Sites
Tswalu Kalahari Reserve (TKR), consisting of the Korannaberg (±101,000 ha) and Lekgaba (±20,000 ha) sections, is situated at S 27°29′61″ and E 22°39′43″ in the arid Northern Cape Province of South Africa (Figure 1A). Prior to the establishment of the reserve in 1998, historical land-use practices on these owner-managed properties were restricted to domestic livestock farming and hunting. Anthropogenic activity in surrounding areas includes manganese mining, thermal and solar power generation and livestock farming which may influence atmospheric deposition of certain elements. Seasonal pans (depressions that fill with rainwater in the wet season) are present on the property but the majority of water is supplied from artificial boreholes. The fenced perimeter prevents migration to higher resource areas and unpredictable rainfall patterns influence vegetation biomass. Supplementary salt and mineral blocks are however provided at water sources to somewhat mitigate nutrient stress. Mean temperatures range from 5 to 24°C in the dry winter months and 22–37°C in the wet summer months with a mean annual rainfall of ∼325 mm (World Weather Online, 2020b). Mountain streams provide seasonal access to water but major rivers do not drain the reserve (van Rooyen and van Rooyen, 2017).
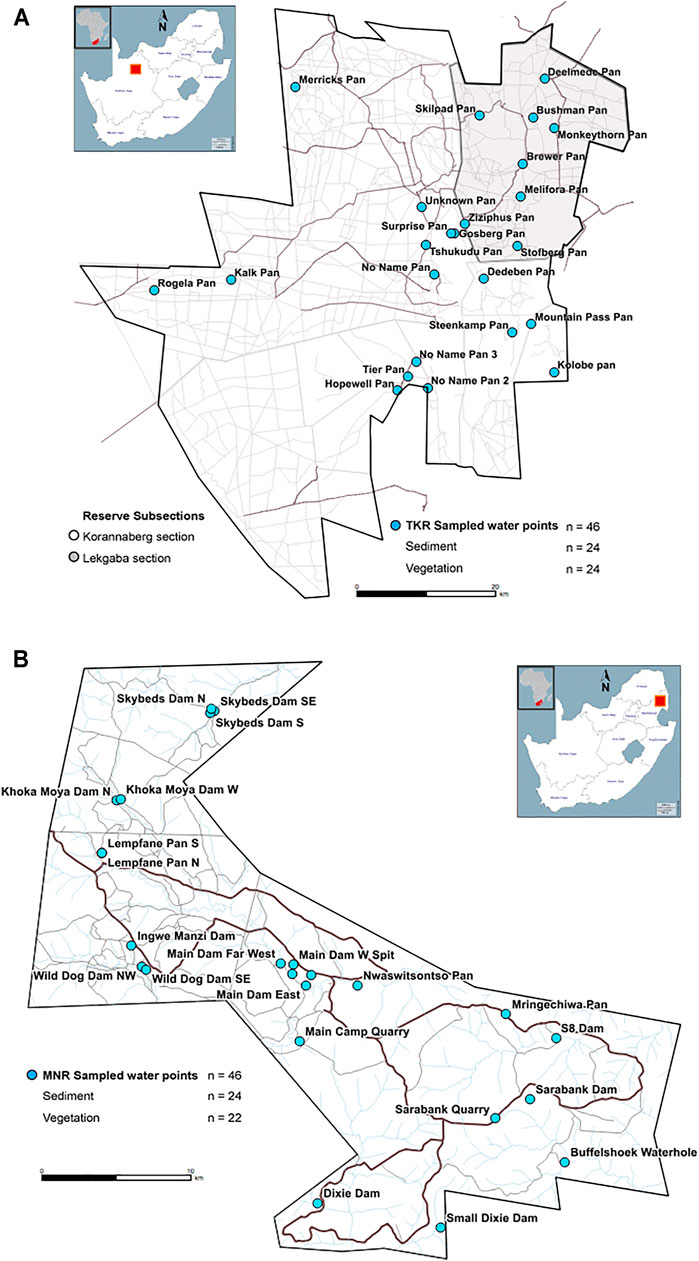
FIGURE 1. Tswalu Kalahari Reserve (1A) within the arid Northern Cape Province South Africa, showing fenced boundaries and the Korannaberg (white: ±101,000 ha) and Lekgaba sections (grey: ±20,000 ha). Manyeleti Nature Reserve (1B) within the mesic Mpumalanga Lowveld of South Africa with unfenced boundaries to the northwest (Associated Private Nature Reserves, east (Kruger National Park) and South (Sabi Sand Game Reserve).
The ±22,497 ha Manyeleti Nature Reserve (MNR), established in 1963 from land previously dedicated to pastoral activities by local indigenous populations, is situated at S 24°64′80″ and E 31°52′63″ in the Mpumalanga Lowveld of South Africa (Figure 1B). The MNR shares unfenced boundaries with the Kruger National Park to the east, the Sabi Sand Game Reserve to the south and the Associated Private Nature Reserves to the northwest (Mpumalanga Tourism and Parks Agency, 2015). Given animals can migrate freely to the north, east and south, provision of artificial water and nutrient supplements are not used as management tools on this property (Personal communication A Webster—M Bourne). Mean temperatures range from 7 to 22°C in the dry winter months and 18–40°C in the wet summer months with annual rainfall between 500 and 700 mm (World Weather Online, 2020a). The Nwaswitsontso and Mthlowa Rivers drain the northern and central regions, filling the main catchment dams in the centre of the reserve. The Phungwe, Thorndale, Mhluwati and Tswayini Rivers drain the southern regions (Cronje et al., 2005). The reserve forms part of the Kruger National Park western boundary, which abuts a number of growing rural communities that rely mostly on pit latrine ablutions and government-controlled spraying of chemicals for vector control. Anthropogenic activities in surrounding areas include subsistence agricultural and livestock activities and small businesses associated with motor repair, leather tanning and other industries.
Sample Collection, Preparation and Digestion for ICP-MS Analysis
Fresh faecal matter from 12 herbivorous (n = 194), 2 omnivorous (n = 50), and 7 carnivorous (n = 137) mammalian species was collected from Tswalu Kalahari Reserve (TKR), Northern Cape Province (April-June 2019), and Manyeleti Nature Reserve (MNR), Mpumalanga Province, (July to September 2019) (Supplementary Table S1). It is unclear whether specific analytes are excreted at the same rate within a faecal deposit. To limit this possible influence on trace element concentrations being measured, faecal deposits were homogenised in situ before collection of sub-samples from the centre of multiple faecal boli or pellets from monogastric and ruminant herbivores respectively (Ganswindt et al., 2002). All faecal samples (∼20 g) were collected from observed defecation events to ensure samples were not contaminated with urine. The majority of fresh carnivore faecal samples were collected opportunistically from observed defecation events around kills, near dens or latrines. However, tracks and other sign around overnight faecal deposits and at latrines left by nocturnal species were used to locate and identify faecal samples belonging to specific species and collected before 07h00 each morning to minimize UV degradation of samples (Liebenberg, 1990a; Liebenberg, 1990b). In the event faecal deposits could not be assigned to a specific species (through observation or track interpretation), samples were not collected. Steenbok (Raphicerus campestris) faecal samples were uncovered after burial and lightly brushed with a paintbrush to remove excess soil. Additionally, to avoid contamination from metal-containing implements, all faecal samples were collected using gloves, subsequently placed into individual metal-free screw-top plastic sampling containers, labelled and stored at –20°C to prevent microbial or chemical degradation of samples post-defaecation.
Faecal samples were lyophilized at –50°C for 5–8 days. Dried faecal samples from all species were subsequently pulverized using a ceramic grater to separate any undigested material and sieved through a plastic-mesh (37 µm) strainer to remove large particles of bone, sinew and vegetation. The use of metal equipment was avoided to prevent contamination of samples during all phases of preparation. Individual herbivore and carnivore ∼0.3 g dry mass (DM) samples were microwave digested (MARS® −5) in 6.5 ml (65%) HNO3: 0.5 ml (30%) HCl. Post-digestion, de-ionized water was added to make 50 ml final volume of each sample (United States Environmental Protection Agency, 2007). All faecal concentrations are given as microgram per kilogram (µg/kg) faecal dry mass (DM).
Trace Element Analysis and Quality Control
Trace element analyses was performed on an Agilent 7900 quadrupole Inductively Coupled Plasma-Mass Spectrometer (ICP-MS) equipped with a High Matrix Introduction system and Agilent Mass Hunter software (version 4.4) for instrument control and data processing (Agilent Technologies, 2009). Detailed parameters for method quantification, adjustments and validation are outlined in Webster et al. (2021c), in Webster et al. (2021a) for analysis of environmental samples and in Webster et al. (2021b) for analysis of essential elements 11B, 55Mn, 56Fe, 59Co, 60Ni, 63Cu, 66Zn, 95Mo, Se. In brief, before sample introduction into the plasmas, argon as the dilution gas was added. Helium collision cell mode using the 4th generation Octopole Reaction System was used for analysis of element isotopes 11B, 27Al, 51V, 52Cr, 55Mn, 56Fe, 59Co, 60Ni, 63Cu, 66Zn, 75As, 88Sr, 95Mo, 111Cd, 118Sn, 121Sb, 137Ba, 202Hg, and 208Pb except Se. Inter-element corrections were made for possible isobaric interferences of high 115Sn on 115In used as an internal standard. Optimization for sensitivity and low oxide ratios (CeO/Ce < 0.3%) were performed daily.
Respective sets of digestions included dual quality controls of a blank acid mixture and a matrix-suitable Certified Reference Material obtained from the National Institute for Science and Technology, Gaithersburg, United States. NIST 1573a tomato leaf ∼0.3 g (dm) and NIST 1577c bovine liver ∼0.2 g (dm) were used as controls for herbivore and carnivore faecal sample digestions respectively. In addition, every third set of carnivore digestions contained ∼0.3 g (dm) of NIST 1573a tomato leaf CRM control to ensure consistency throughout the digestion sequence of carnivore faecal samples. Accuracy (% recovery) and precision (% RSD) of replicate measurements for certified reference material controls fell within 20% of expected value at lower concentrations and 15% at higher concentrations for all elements except Al (United Nations Office on Drugs and Crime, 2009).
Data Analysis
All statistical analyses were conducted using the R software: v 3.6.1 (R Core Team, 2019). In total, 381 faecal samples were collected from 21 different terrestrial mammal species belonging to three trophic groups (herbivore, omnivore and carnivore) at two geographically different sites (MNR and TKR) (Supplementary Table S1). To balance the comparison between “sites” and “groups”, species present at both study sites were selected for evaluation. In the event a given species was present at only one site (e.g., Meerkat/Brown hyaena) a similar surrogate species (e.g., Dwarf mongoose/Spotted hyaena) representing the same trophic group was selected at the other site. Faecal concentrations are given as microgram per kilogram (µg/kg) faecal dry mass (DM). Descriptive statistics for all measured potentially toxic elements (PTEs) were determined using all samples from each species and evaluated at each site. To exclusively facilitate a visual comparison, the scales of measure for each PTE were homogenized (mean = 1), dividing each element’s concentration by its mean across sites and species. Subsequently, plots (Figures 2, 3) were created to compare relative concentrations of elements within animal groups and at each site (ggplot2 R package). Consequently, 24 outliers that produced extensive compression of scale for a specific element, thus obscuring specific differences between species’ boxplots within animal groups were identified and removed, one by one, before further analyses.
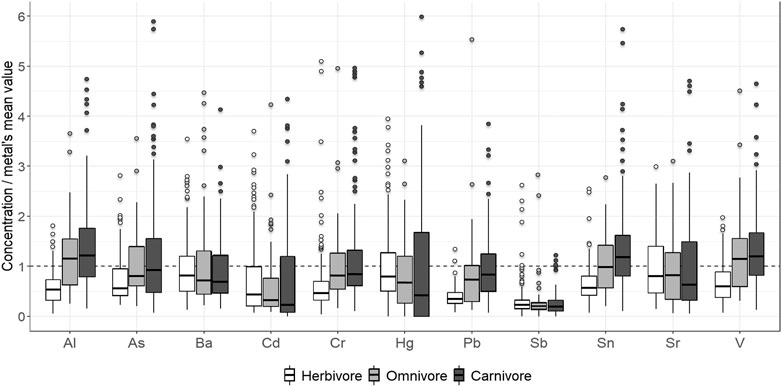
FIGURE 2. Overall profile of potentially toxic element concentration measured in herbivorous (white), omnivorous (light grey), and carnivorous (dark grey) mammals relative to the mean for both sites (dashed line). Dark lines within boxes represent median values; the box represents the upper (3rd) and lower (1st) quartiles. Whiskers represent minimum and maximum values excluding outliers while points represent outliers. Concentrations are relativized and given as microgram per kilogram (µg/kg) faecal dry mass (DM).
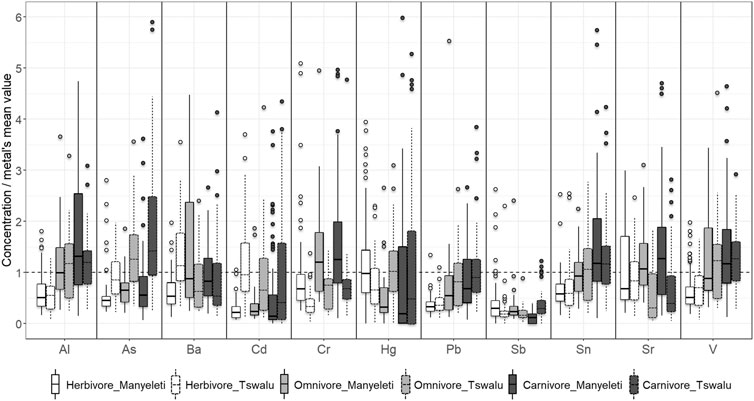
FIGURE 3. Differences in measured potentially toxic element concentrations between sites (MNR: solid borders, TKR: dashed borders) in herbivorous (white), omnivorous (light grey), and carnivorous (dark grey) mammals relative to the mean for both sites (dashed line). Dark lines within boxes represent median values; the box represents the upper (3rd) and lower (1st) quartiles. Whiskers above and below the box represent minimum and maximum values excluding outliers. Points represent outliers. Concentrations are relativized and given as microgram per kilogram (µg/kg) faecal dry mass (DM).
Before testing for differences between trophic groups and/or study sites, individual effects (with the potential to affect element means) were considered in the estimation model. To therefore account for the variability of measured element concentrations between species, numbered blocks (“block” variable in Supplementary Table S1) were assigned to each species (or comparable species) unique to each study site. Following this a 3-way ANOVA randomized block design was used that considered “sites” and “groups” as respective fixed effect factors and “blocks” as a random effect factor. Subsequently, a linear mixed-effects model assuming heterogeneous variances and including an interaction effect between “sites” and “groups” was built for each PTE and estimated by maximum likelihood (nmle and car R-packages). Using these estimated models (summary of fitted models Supplementary Table S2), marginal and conditional means as well as standard errors (SE) were determined for “groups” and “sites” using the predicted marginal means method (emmeans R-package). Mean differences between “groups” or “sites” were investigated by applying Tukey’s pairwise post-hoc test (multcomp R-package). Basic results are reported as mean ± SE and statistical significance was determined at the p < 0.05 α level (Table 1). Associations between PTEs were investigated using their corresponding Pearson’s r correlation coefficients and p-values (Tables 2, 3).
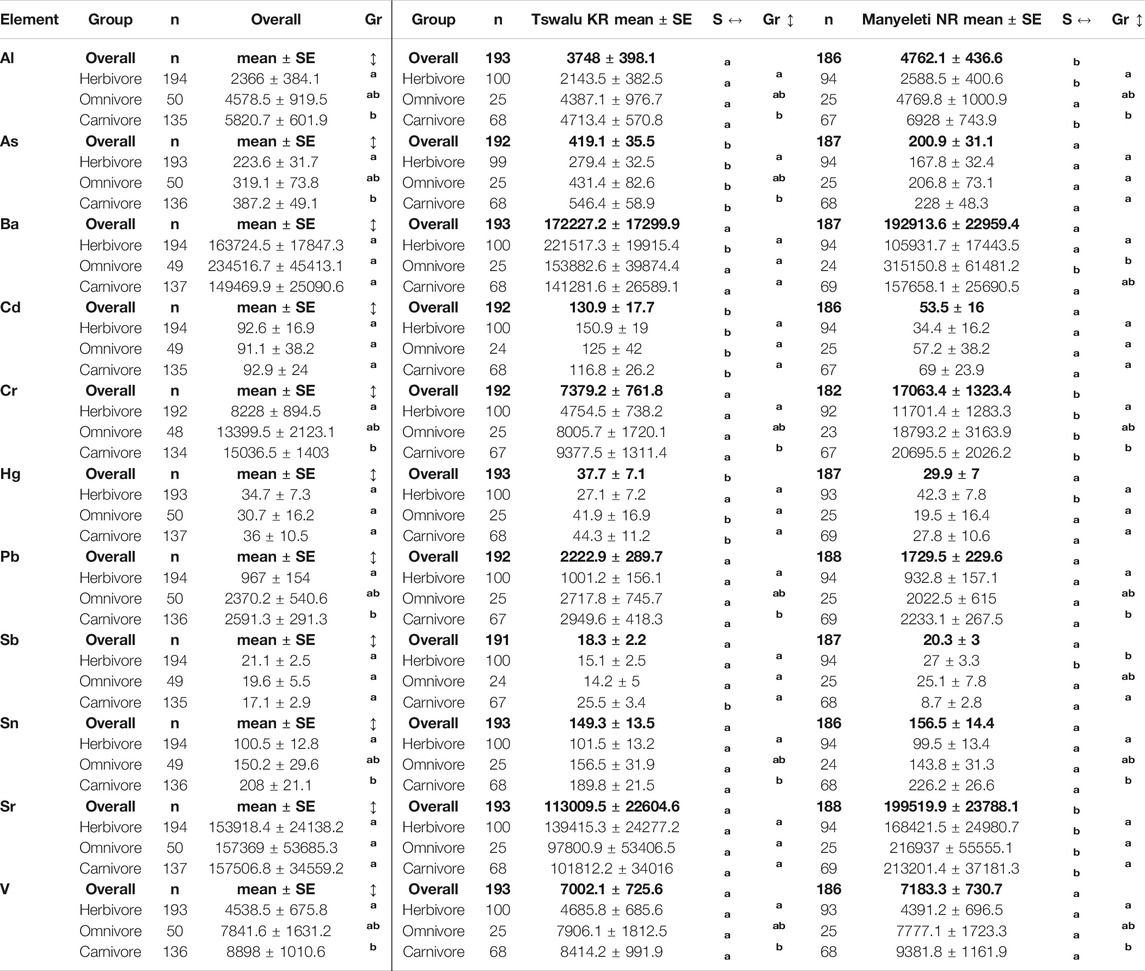
TABLE 1. Estimates for potentially toxic element concentrations between animal groups overall (left) and between sites for any group (right). Note: Overall differences between groups are shown on the left of the table. Comparisons between sites (overall and in any group) and between groups (within each site) are shown on the right. Within row letter subscripts a, b indicate a significant difference between sites (p < 0.05) and within column letter superscripts a, b, c indicates significant differences between groups (p < 0.05). Faecal concentrations are given as microgram per kilogram (µg/kg) faecal dry mass (DM).
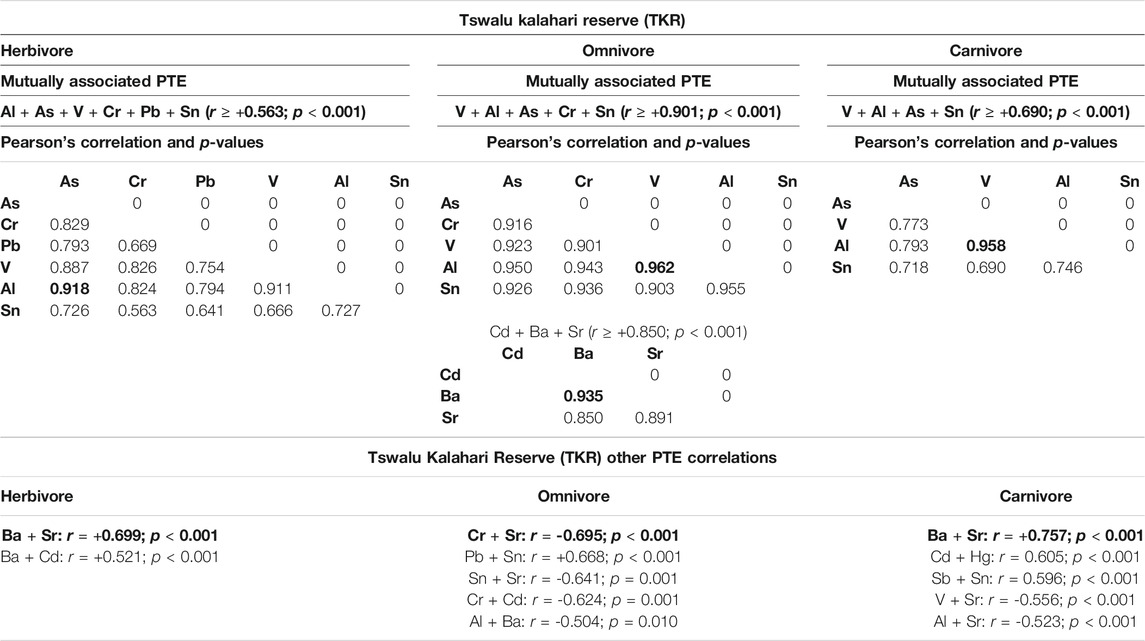
TABLE 2. Mutually associated PTEs and other PTE correlations at the Tswalu Kalahari Reserve (TKR) in different trophic groups. Grey highlighted blocks with bold lettering show highest mutually associated PTE for respective trophic groups. Bold text represents the highest mutually correlated elements and other correlated PTEs for specific trophic groups. Faecal concentrations are given as microgram per kilogram (µg/kg) faecal dry mass (DM).
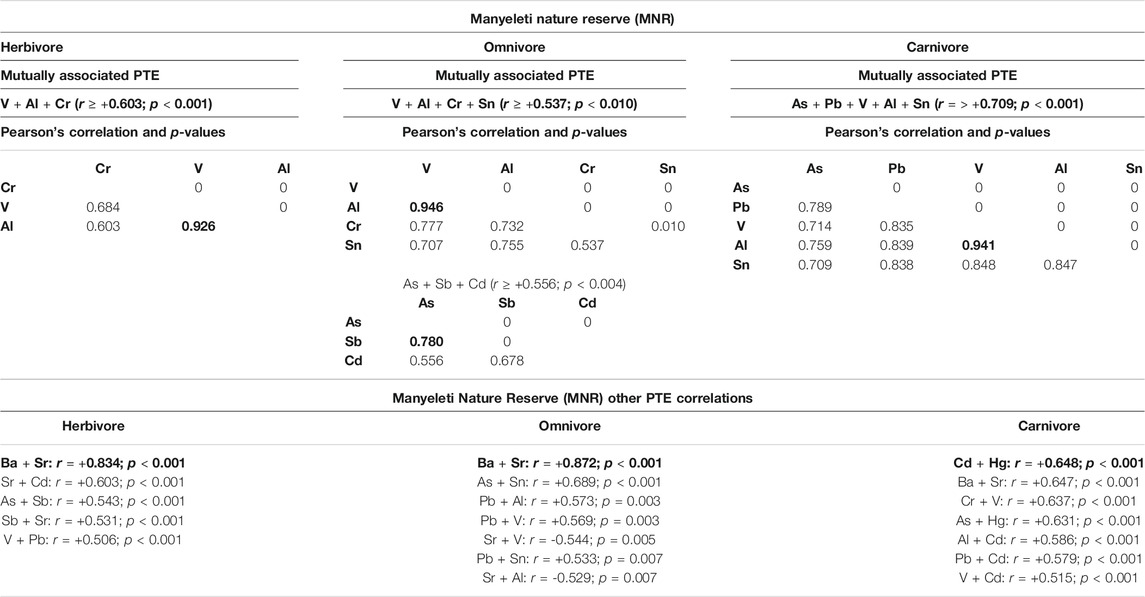
TABLE 3. Mutually associated PTEs and other PTE correlations at the Manyeleti Nature Reserve (MNR) in different trophic groups. Grey highlighted blocks with bold lettering show highest mutually associated PTE for respective trophic groups. Bold text represents the highest mutually correlated elements and other correlated PTEs for specific trophic groups. Faecal concentrations are given as microgram per kilogram (µg/kg) faecal dry mass (DM). Tswalu Kalahari Reserve (TKR) other PTE correlations" Herbivores - "Ba + Sr: r = +0.699 ; p < 0.001.
Results
Overall Comparison of PTEs Between Trophic Groups
Overall PTE concentrations varied between individuals and between animal groups (Figure 2). Measured mean concentrations for respective PTEs were four to six times higher than their respective means in some individuals. When measured concentrations from animal groups from both sites were compared, Al, As, Cd, Pb, Sn, and V were significantly higher in carnivores compared to herbivores. Measured mean element concentrations in omnivores did not differ significantly from those in either herbivores or carnivores. Measured mean concentrations of Ba, Cd, Hg, Sb and Sr did not differ significantly overall between trophic groups (Table 1: left Gr column).
Comparison of PTEs in Trophic Groups Within Sites
Differences between mean measured PTE concentrations in trophic groups within and between sites are shown in Figure 3. Within the TKR site, concentrations were significantly higher for Al, As, Cr, Pb, Sn, and V in carnivores compared to herbivores (Table 1: right Gr column). Concentrations in omnivores did not differ significantly to those measured in either herbivore or carnivore groups. There was no significant difference in concentration between groups for Ba, Cd, Hg, Sb, or Sr at the TKR site. Within the MNR site, concentrations were significantly higher for Al, Cr, Pb, Sn and V in carnivores compared to herbivores, but concentrations in omnivores did not differ significantly from either herbivores or carnivores (Table 1: right Gr column). In omnivores, Ba concentrations were significantly higher than those in herbivores, but concentrations in carnivores were not significantly different to those measured in other groups. In herbivores, Sb concentrations were significantly higher to those measured in carnivores but did not differ significantly in omnivores compared to other groups. There was no significant difference in concentration between groups for As, Cd, Hg, or Sr at the MNR site.
Comparison of PTEs in Trophic Groups Between Sites
When mean measured PTE concentrations in trophic groups were compared between sites (Table 1: right S column), animal groups at the TKR site showed significantly higher concentrations of As, Cd, and Hg while those in the MNR site had significantly higher concentrations of Al, Cr, and Sr. High As, Cd, and Hg concentrations were mirrored in carnivores and omnivores from the TKR site. Sb was also significantly high in carnivores. Herbivores at the TKR site had significantly higher concentrations of As, Ba, and Cd than those measured in their MNR counterparts. At the MNR site, Al, Cr, and Sr in carnivores, Ba, Cr, and Sr in omnivores and Al, Cr, Hg, Sb, and Sr in herbivores were significantly higher than those measured in the same animal groups in the TKR. There was no significant difference in concentrations for Pb, Sn, and V in any group between sites. Clear patterns emerge between groups for certain elements within sites but absolute values for the same elements differ between sites. Detailed results and summary of fitted models for each PTE are presented in Table 1 and Supplementary Table S2 of supplementary material.
Comparison of PTE Associations in Trophic Groups Between Sites
Mutually associated (considerable correlation for any PTE pair) and correlated (between elements) PTEs for each trophic group at the TKR site can be seen in Table 2. In herbivores at the TKR site a mutual association between Al + As + V + Cr + Pb + Sn (minimum correlation: r = +0.563) was evident. The highest correlation within mutually associated PTEs was between Al + As (r = +0.918; p < 0.001), while the highest correlation for other PTEs was between Ba + Sr (r = +0.0.699; p < 0.001). In TKR omnivores, V + Al + As + Cr + Sn (minimum correlation; r = +0.901) were mutually associated with Al + V (r = +0.962; p < 0.0001) being the highest. In addition, Cd + Ba + Sr (minimum correlation: r = +0.850) were also mutually associated in omnivores, with Ba + Cd (r = +0.935; p < 0.001) being the highest. The highest significant correlations for other PTE in omnivores was between Cr + Sr (r = 0.695; p < 0.001). In carnivores at the TKR site, mutual association between PTE’s V + Al + As + Sn (with a minimum correlation; r = +0.690) was evident. Within mutually associated PTE in carnivores, the correlation between Al + V (r = +0.958; p < 0.001) was the highest, while the highest other correlated PTEs for carnivores at the TKR site were Ba + Sr (r = 0.757; p < 0.001).
Mutually associated and correlated PTEs for each trophic group at the MNR site can be seen in Table 3. At the MNR site, mutual association between PTEs V + Al + Cr (minimum correlation r = +0.603) was evident in herbivores with Al + V (r = 0.926; p < 0.001) being the highest. The highest other PTE correlations in herbivores were Ba + Sr (r = +0.834; p < 0.001). In MNR omnivores mutual associations were evident between PTE As + Sb + Cd (minimum correlation r = +0.556) with the strongest mutual association being between Sb + As (r = +0.780; p < 0.004). In addition, mutual associations were found between V + Al + Cr + Sn (minimum correlation r = +0.537) with Al + V (r = +0.946; p < 0.010) being the highest. Ba + Sr (r = +0.872; p < 0.001) were other highly correlated PTEs for omnivores at this site. In MNR carnivores, a mutual association between PTEs As + Pb + V + Al + Sn (minimum correlation; r = +0.709) was evident with Al + V (r = +0.941; p < 0.001) being the highest. Cd + Hg (r = +0.648; p < 0.001) were other highly correlated PTEs in MNR carnivores.
Discussion and Conclusion
“Heavy metal” pollution rated highest of 22 themes related to emerging chemical management issues in developing countries that are not covered by international treaties (STAP, 2012). Compared to marine and aquatic environments, information related to the presence and effects of PTEs and other pollutants in terrestrial systems is limited (Rodríguez-Jorquera et al., 2017). Although valuable information has been gained from previously used invasive sampling approaches, much of what has been learnt has occurred as a result of opportunistic sampling of fresh carcasses at mass die offs and debilitation events (Ferreira and Pienaar, 2011). Faecal material is a readily produced waste product and is an effective matrix for trace element assessment in free-ranging wildlife species. With the exception of Hg, which can undergo chemical change in the liver, rumen and digestive tract, most elements may be stored within the body after ingestion but are not chemically changed through metabolism in the liver or kidney. In this regard, many trace elements are detectable in their original form within excreta (Das et al., 2019). This study presents the first quantitative assessment and comparison of multiple PTEs in herbivorous, omnivorous and carnivorous terrestrial mammals in a mesic and arid protected area of South Africa, using faeces as a non-invasive matrix.
When measured concentrations from animal groups at both sites were compared, Al, Cd, Pb, Sn, and V as well as As at the TKR site were significantly higher in carnivores than in other animal groups. Site-specific geology contributes to natural background levels of metals and metalloids within the environment (FAO and UNEP, 2021), however anthropogenic activities can increase bioavailability and bio-accessibility of PTEs. The physical interaction between water, geologic substrate and soils (Knoop and Walker, 1985) additionally influences vegetation structure and PTE concentrations in woody and C4 plant species, making soils a key component of terrestrial ecosystems (Herselman, 2007; Vaughn et al., 2015). Relatively high levels of PTEs As, Cd, Cr, Pb, and Sb are associated with the banded iron ores of the Kalahari region surrounding the TKR site (Varentsova and Kuleshovb, 2019). In contrast, Archaean gneiss and prominent intrusions of Timbavati gabbroic rock underlie much of the MNR site, particularly in the west (Walraven, 1986). The gabbro intrusions closest to the MNR are associated with relatively high levels of Cr and V as well as elements Co, Fe and Zn (Walraven, 1986), which are considered essential for biological function (Webster et al., 2021b). In addition to site-specific geochemical signatures that may in part account for high concentrations of specific PTE particularly in carnivores, results indicate dietary-niche peculiarities and possible biomagnification of environmental concentrations at both sites.
When trophic groups within sites were compared, Al, As, Cr, Pb, Sn, and V were highest in carnivores at the TKR site. At the MNR site, Al, Cr, Pb, Sn, and V were highest in carnivores, Ba was higher in omnivores and Sb was highest in herbivores than in any other trophic groups at that site. Aluminium is an abundant element in the Earth’s crust (Kabata-Pendias, 2011) and its occurrence in carnivores at both sites may in part be attributed to its presence in underlying geological signatures, but may also be attributed to one or a combination of behavioural factors. These include the ingestion of Al-containing soil during consumption of kills, the grooming habits of social meso- and apex predators or consumption of ground-dwelling invertebrates and arthropods by insectivorous mesocarnivores. High levels of Al in carnivores may also be indicative of trophic biomagnification from ingestion of herbivorous/omnivorous prey species with high body burden (Ali and Khan, 2019). Al is not generally available for participation in biogeochemical reactions given its insoluble nature (Driscoll and Schecher, 1990), however environmental sources of exposure to this element remain speculative. Whether concentrations at the levels measured here have deleterious effects alone or in combination with co-occurring PTE in wildlife is uncertain.
The presence of arsenic (As) and many of its water-soluble compounds (Agency for Toxic Substances and Disease Registry (ATSDR), 2007a) in the geological signature of the TKR, translated to significantly high measured concentrations across animal groups and a clear pattern of trophic accumulation. Drinking from contaminated water sources is a major route of exposure for wildlife species. Between 70 and 90% of inorganic As is absorbed through the gastrointestinal tract and distributed primarily to the liver, kidneys, bladder and lungs and secondarily to muscle and nerve tissue (Palma-Lara et al., 2020). Herbivores must access water regularly to meet the physiological demands of thermoregulation and rumination (Boyers et al., 2019). Species such as buffalo (Syncerus caffer) and impala (Aepyceros melampus) are water dependant and must access water daily (Redfern et al., 2003). Gemsbok (Oryx gazella), springbok (Antodorcas marsupialis), and steenbok (Raphicerus campestris) are adapted to higher temperatures and limited surface water availability and are able to concentrate excretions to minimise water loss (Eloff, 1973; Knight, 2013; Boyers et al., 2019). As the mechanistic adaptations of this trophic group may translate into As-accumulation in the kidneys, liver and bladder, herbivores are at high risk of repeated and continual exposure through consumption of As-contaminated water. Carnivores, particularly those living in arid environments, access surface water when available, obtaining the majority of their moisture through the prey items they consume (Eloff, 1973). Because of their high nutrient content, blood rich organs such as the liver and kidneys are often consumed first (Kohl et al., 2015). Toxins within these organs then add to the body burden of carnivorous predators. The assessment of long-term exposure to inorganic arsenic is complicated by the duration of exposure, pathway of ingestion, physicochemical properties of the compound and symptoms that differ between individuals, species and geographical areas (World Health Organisation, 2019; Palma-Lara et al., 2020). Long-term As exposure in animals has been linked to pregnancy-related complications in females including miscarriage, low foetal birth weight and foetal deformity (Agency for Toxic Substances and Disease Registry (ATSDR), 2007a) all of which affect survival and reproductive ability of carnivore populations.
Chromium (Cr) the most abundant element measured in environmental matrices (Webster et al., 2021a) at the MNR site was reflected across animal groups. In part, concentrations could be attributed to the geochemical signature of the region however, in mesic environments, interactions between sulphate and iron carriers in plants facilitate high rates of Cr uptake in vegetation (Shankar et al., 2005). Herbivores and omnivores feeding on this vegetation daily (Reglero et al., 2008) and social species that exhibit allo-grooming behaviour are likely to accumulate concentrations of this element to a greater extent than solitary species. Damage to the reproductive system in male animals and increased complications during parturition have been documented in females (World Health Organisation/International Agency for Research on Cancer, 1990; Agency for Toxic Substances and Disease Registry (ATSDR), 2012a). Biomagnification in end-consumers would therefore have negative effects on reproductive success.
Significantly high levels of lead (Pb) were measured in carnivores at both sites. In biological systems, Pb and calcium act in a similar manner, facilitating Pb-storage within bone (Gulson et al., 2003) and remobilisation of Pb into the blood stream during periods of physiological or nutritional stress. Consumption of bones by scavenging and obligate carnivores may contribute to Pb concentrations within carnivores and other species such as vultures (Ogada et al., 2012; Naidoo et al., 2017). Foraging opportunities for suitable prey species are unpredictable, so carnivores are particularly vulnerable to the effects of Pb remobilisation during periods of nutrient stress. Lead is a systemic toxicant and there is growing evidence to suggest that low-level environmental exposure from various sources impairs renal function and neurological development in juveniles, which can result in life-long neurological deficits, endocrine disruption and reproductive failure (Jadhav et al., 2007; Assi et al., 2016; Agency for Toxic Substances and Disease Registry (ATSDR), 2019b).
Carnivores within both sites also had significantly high measured concentrations of Tin (Sn) and vanadium (V). Sn is found in fungicides, insecticides and pesticides and given the increased conversion of land for agricultural purposes, has become a threat to the environment. Although Sn has generally low solubility in soil, in response to a decrease in soil pH, uptake in plants increases (Nakamaru and Uchida 2008). Whether V is an essential element is still being debated however, it accumulates in plant roots and is a known insulin mimic (Agency for Toxic Substances and Disease Registry (ATSDR), 2012b). Although site-specific geochemistry may be linked to Sn and V concentrations at both sites, Sn presence at the TKR site may be related to historical legacy of livestock farming while at the MNR site, increasing subsistence agricultural activity in communities surrounding the reserve may be influencing concentrations. Whether Sn and V alone, in combination with other elements or combined with exogenous toxins cause deleterious effects in different free-ranging wildlife species is unclear.
Significantly high concentrations of antimony (Sb) were measured in herbivores at the MNR site and are most likely related to geological signature and soil to plant transfer (Agency for Toxic Substances and Disease Registry (ATSDR), 2019a). Ba concentrations were significantly higher in omnivores at this site than in other groups at either site while herbivores at the TKR site had higher concentrations of Ba than other groups. Although these differences may be attributed to feeding ecology between species at the different sites, Ba compounds are mostly insoluble in water so persist in the environment. Although barium-carbonate is insoluble in water, it is soluble in the acid environment of the GI tract resulting in toxic effects. Ba compounds are often associated with waste sites, which contaminate surrounding soil, plants and ground water through leaching (Agency for Toxic Substances and Disease Registry (ATSDR), 2007b). Historical land use and waste disposal methods are unknown for the TKR site but previous disposal of waste in landfills may play a role in Ba concentrations in herbivores utilizing rehabilitated sites on this property. In contrast, omnivores at the MNR site frequent the landfill site situated in close proximity to the riverine stretches along the main catchment area during daily foraging forays. Established vegetation and sources of fruit, berries and other food items may be contributing to concentrations in this trophic group. Further research is however required to determine site-specific sources of this element and the negative effects they may have (if any) in the species with high concentrations.
Mercury is an agricultural, industrial, mining and waste-related pollutant and is classified as one of the top 10 contaminants of health concern (FAO and UNEP, 2021). Hg was found in concentrations above the TKR mean in environmental matrices (Webster et al., 2021a) and in carnivores (this study) and may be related to anthropogenic activities associated with the landing strip. Although contamination at the TKR site was localised, highest levels of Hg at the MNR site were measured in herbivores and may result from water-transport of mercury-containing fertilizers and anti-parasitic veterinary drugs used in substance farming by communities in close proximity to the reserve. Additionally, direct runoff from contaminated livestock manure into surface water may be a contributing factor to contamination of grazing areas in close proximity to water catchment areas (Richards et al., 2014; Webster et al., 2021a). Cadmium is used in multiple industries and agricultural fertilizers and when bound to fine particles, can be transported atmospherically (Burger, 2008). Cd concentrations were significant in environmental matrices at the TKR site, which is reflected across animal groups (Webster et al., 2021a). Plants have the ability to accumulate Cd in roots and shoots at levels that are toxic to most other organisms. Although this mechanism possibly developed as a defence against herbivory (Godinho et al., 2018), species consuming Cd-containing forage are likely to accumulate Cd in the liver and kidneys. Toxicity is exacerbated by low rates of excretion, accumulation in the proximal renal tubules and high rate of reabsorption through the kidneys (Azeh Engwa et al., 2019).
Although strontium (Sr) is a substantial component of igneous rock (Kabata-Pendias, 2011), electronic waste sites containing broken visual display units and television screens and the improper disposal of mechanical greases contribute to its presence in the environment. All stable isotopes behave in a similar chemical manner to each other, but also behave in a chemically similar way to Ba and calcium (Pathak and Gupta, 2020). Prolonged Sr exposure in plants causes reduced phytoestrogen content resulting in decreased antioxidant capacity and impaired uptake of other micronutrients (Dresler et al., 2018). When Sr-containing plants are consumed by herbivorous or omnivorous species, Sr presence can affect bone growth, the ability of bone marrow to produce blood cells and compromised clotting (Agency for Toxic Substances and Disease Registry (ATSDR), 2004).
The highest mutually associated PTEs were Al and V in all trophic groups at both sites, and are likely indicative of their abundance in the environment. However, site-specific patterns in mutually associated PTE were in contrast between sites for other correlated PTEs (Tables 2, 3). At the TKR site mutual associations between these elements were highest in omnivores > carnivores > herbivores. In addition, a mutual association between Ba and Cd in omnivores was also high at this site. Ba and Sr were other highly correlated PTEs in omnivores and herbivores at this site, while Ba and Sr followed by Cd and Hg were other highly correlated elements in TKR carnivores. At the MNR site, a mutual association between Al and V was highest in carnivores > herbivores > omnivores. Sb and As were also mutually correlated in omnivores at this site. Ba and Sr were other highly correlated PTEs in omnivores and herbivores, followed by Cr and V. Cd and Hg as well as Ba and Cr were highly correlated in MNR carnivores. In biological terms, PTE associations at each site are likely influenced to some degree by localized geochemistry but clear patterns also emerge related to feeding ecology, PTE transfer via trophic interactions and biomagnification in carnivores and soil-plant transfer in herbivores and omnivores. Additionally, interactions between essential and potentially toxic elements are well known for some elements including (Fe and Cd; Akesson et al., 2002, Ca and Pb; Gulson et al., 2003, Fe, Cu, and Zn; Hooser, 2018), but require more specific investigation in free-ranging wildlife species.
Previous studies have been conducted in heavily polluted protected areas (Gupta and Bakre, 2013), but this is the first assessment of PTE in African savannahs that considers differences between trophic groups in relatively “pristine” protected areas. Our understanding of the mechanisms by which PTEs affect biological systems has increased with new technology and the development of environmental assessment frameworks. Much of what we know is related to established mechanisms associated with essential and toxic elements but there is still much to learn about how these elements interact with one another in wildlife species of different body size, age, sex, and life stage living in habitats with site-specific contaminant signatures. Additionally, more detailed analyses related to the effects different diets and consumption of various food items may have on PTE accumulation in different trophic groups (and species) are required. Addressing these gaps in our knowledge will require a One-Health approach and the sharing of information between veterinary, medical and ecological professionals as well as application of invasive methods simultaneous to the use of a non-invasive approach. Faecal material is one of numerous matrices to consider for non-lethal environmental monitoring but it is important to remember that all methods have limitations. We know faecal material closely reflects actual intake; we also know that chemically intact elemental compounds can be measured in faecal material. Although some physiological mechanisms related to elimination of toxins from the body are known what we do not yet know without lethal investigation is how much of the actual intake is being retained as body burden in the organs of different species occupying different dietary niches. Additionally, although faecal material is a suitable metric for the measurement of essential and potentially toxic trace elements in wildlife, it may not be an effective tool for the monitoring of other pollutants not excreted in the faeces or for those that are chemically altered through metabolism in the body.
Given trace element concentrations within protected areas are influenced by background geochemical signatures, PTE pollution is site, species-as well as diet-specific. Although faecal analysis cannot tell the full story related to the presence and/or persistence of hazardous pollutants and their effects on ecosystem integrity, it can help to identify species at high risk and hot spots for more intensive environmental monitoring within protected areas, acting as an early warning system for potentially devastating pollution events. In addition to mammalian species, faecal samples can practically be collected in a non-invasive manner from birds, insects, bats and reptiles in conjunction with environmental matrices such as water, soil/sediment and vegetation samples to provide a more complete assessment of ecosystem integrity. Information gathered in this way would allow us to draw general conclusions related to animal exposure that are relevant to species biodiversity assessments, wildlife management and species conservation. Further research is required at both plant and animal species-specific levels to provide greater insight into how metabolism changes pollutant compounds for more accurate determination of different toxins using faecal material, the possible sources of pollutants within specific protected areas and the routes of exposure that result in wildlife exposure to toxins in protected areas.
Data Availability Statement
The datasets presented in this study can be found in online repositories. The names of the repository/repositories and accession number(s) can be found below: Doi:10.25403/UPresearchdata.14675388.
Ethics Statement
The animal study was reviewed and approved by the University of Pretoria Research and Animal Use and Care Committee (Reference EC043-18 and EC043-18-A1) and the South African Department of Agriculture, Forestry and Fisheries (DAFF-18/02/2019). The MNR site lies within the “infected zone” for the control of Foot and Mouth Disease (FMD) in South Africa. As a result, faecal subsamples from all samples collected were transported under Red Cross Veterinary Permit to the Agricultural Research Council, Trans-boundary Animal Diseases facility at the Onderstepoort Veterinary Research Campus, South Africa, for testing prior to removal from the MNR site. Upon receipt of negative FMD results and termination of fieldwork, frozen faecal samples were transported under Red Cross Veterinary Permit to the University of Pretoria’s Endocrine Research Laboratory for preparation and subsequent diagnostic testing.
Author Contributions
ABW: Principal investigator, Conceptualisation, Methodology, Data collection and analysis, Visualisation, Project administration, Data curation and Writing–Original draft. JFC: Formal analysis, Data curation, Writing–Review and editing. NCB: Conceptualisation, Academic supervision, Funding, Writing–Review and editing. AG: Conceptualisation, Academic Supervision, Writing–Review and editing.
Funding
This research was supported by the Department of Science and Technology and National Research foundation SARChI chair of Mammalian Behavioural Ecology and Physiology, South Africa (GUN number 64756), The University of Pretoria Post-graduate Scholarship Programme and The Tswalu Foundation, South Africa. The National Institute for Science and Technology, Gaithersburg, United States of America is acknowledged for donation of domestic sludge and tomato leaf Certified Reference Materials.
Conflict of Interest
The authors declare that the research was conducted in the absence of any commercial or financial relationships that could be construed as a potential conflict of interest.
Publisher’s Note
All claims expressed in this article are solely those of the authors and do not necessarily represent those of their affiliated organizations, or those of the publisher, the editors and the reviewers. Any product that may be evaluated in this article, or claim that may be made by its manufacturer, is not guaranteed or endorsed by the publisher.
Acknowledgments
Tswalu Kalahari Reserve and Mpumalanga Tourism and Parks Agency, Manyeleti Game Reserve are thanked for facilitating this research. Central Analytical Facilities, ICP-MS Laboratory, University of Stellenbosch is thanked for sample analysis. The University of Pretoria Endocrine Research laboratory and CSIR Stellenbosch are thanked for assistance with sample preparation.
Supplementary Material
The Supplementary Material for this article can be found online at: https://www.frontiersin.org/articles/10.3389/fenvs.2021.794487/full#supplementary-material
References
Agency for Toxic Substances and Disease Registry (ATSDR) (2004). Toxicological Profile for Strontium. Atlanta, GA: U.S. Department of Health and Human Services, Public Health Service. Available at: https://www.atsdr.cdc.gov/substances/toxsubstance.asp?toxid=120 (Accessed April 12, 2020).
Agency for Toxic Substances and Disease Registry (ATSDR) (2007a). Toxicological Profile for Arsenic. Atlanta, GA: U.S. Department of Health and Human Services, Public Health Service. Available at: https://www.atsdr.cdc.gov/substances/toxsubstance.asp?toxid=3 (Accessed April 12, 2020).
Agency for Toxic Substances and Disease Registry (ATSDR) (2007b). Toxicological Profile for Barium. Atlanta, GA: U.S. Department of Health and Human Services, Public Health Service. Available at: https://www.atsdr.cdc.gov/substances/toxsubstance.asp?toxid=57 (Accessed April 12, 2020).
Agency for Toxic Substances and Disease Registry (ATSDR) (2012a). Toxicological Profile for Chromium. Atlanta, GA: U.S. Department of Health and Human Services, Public Health Service. Available at: https://www.atsdr.cdc.gov/toxprofiles/tp.asp?id=62&tid=17 (Accessed July 12, 2020).
Agency for Toxic Substances and Disease Registry (ATSDR) (2012b). Toxicological Profile for Vanadium. Atlanta, GA: U.S. Department of Health and Human Services, Public Health Service. Available at: https://www.atsdr.cdc.gov/substances/toxsubstance.asp?toxid=50 (Accessed April 12, 2020).
Agency for Toxic Substances and Disease Registry (ATSDR) (2019a). Toxicological Profile for Antimony. Atlanta, GA: U.S. Department of Health and Human Services, Public Health Service. Available at: https://www.atsdr.cdc.gov/substances/toxsubstance.asp?toxid=58 (Accessed April 12, 2020).
Agency for Toxic Substances and Disease Registry (ATSDR) (2019b). Toxicological Profile for Lead. (Draft for Public Comment). Atlanta, GA: U.S. Department of Health and Human Services, Public Health Service. Available at: https://www.atsdr.cdc.gov/substances/toxsubstance.asp?toxid=22 (Accessed April 12, 2020).
Agilent Technologies (2009). Comparing Collision/Reaction Cell Modes for the Measurement of Interfered Analytes in Complex Matrices Using the Agilent 7700 Series ICP-MS: Technical Overview. Available at: http://hpst.cz/sites/default/files/attachments/5990-3236en-comparing-collision-reaction-cell-modes-measurement-interfered-analytes-complex-matrices.pdf (Accessed May 6, 2020).
Akesson, A., Berglund, M., Schütz, A., Bjellerup, P., Bremme, K., and Vahter, M. (2002). Cadmium Exposure in Pregnancy and Lactation in Relation to Iron Status. Am. J. Public Health 92 (2), 284–287. doi:10.2105/ajph.92.2.284
Ali, H., and Khan, E. (2019). Trophic Transfer, Bioaccumulation, and Biomagnification of Non-essential Hazardous Heavy Metals and Metalloids in Food Chains/webs-Concepts and Implications for Wildlife and Human Health. Hum. Ecol. Risk Assess. Int. J. 25 (6), 1353–1376. doi:10.1080/10807039.2018.1469398
Assi, M. A., Hezmee, M. N. M., Haron, A. W., Sabri, M. Y., and Rajion, M. A. (2016). The Detrimental Effects of Lead on Human and Animal Health. Vet. World 9 (6), 660–671. doi:10.14202/vetworld.2016.660-671
Azeh Engwa, G., Udoka Ferdinand, P., Nweke Nwalo, F., and Unachukwu, M. N. (2019). “Mechanism and Health Effects of Heavy Metal Toxicity in Humans,” in Poisoning in the Modern World - New Tricks for an Old Dog. Editors O. Karcioglu, and B. Arslan (London: IntechOpen). Available at: https://www.intechopen.com/books/poisoning-in-the-modern-world-new-tricks-for-an-old-dog-/mechanism-and-health-effects-of-heavy-metal-toxicity-in-humans (Accessed February 14, 2018).
Baynes, R. E., Dix, K. J., Riviere, J. E., and Riviere, J. E. (2012). “Distribution and Pharmacokinetics Models,” in Pesticide Biotransformation and Disposition. Editor E. Hodgeson (San Diego: Elsevier, Academic Press), 117–147. doi:10.1016/b978-0-12-385481-0.00006-x
Böswald, L. F., Dobenecker, B., Clauss, M., and Kienzle, E. (2018). A Comparative Meta-Analysis on the Relationship of Faecal Calcium and Phosphorus Excretion in Mammals. J. Anim. Physiol. Anim. Nutr. 102 (2), 370–379. doi:10.1111/jpn.12844
Boyers, M., Parrini, F., Owen-Smith, N., Erasmus, B. F. N., and Hetem, R. S. (2019). How Free-Ranging Ungulates with Differing Water Dependencies Cope with Seasonal Variation in Temperature and Aridity. Conservation Physiol. 7 (1), coz064. doi:10.1093/conphys/coz064
Burger, J. (2008). Assessment and Management of Risk to Wildlife from Cadmium. Sci. Total Environ. 389, 37–45. doi:10.1016/j.scitotenv.2007.08.037
Celis, J. E., Espejo, W., Barra, R., Gonzalez-Acuña, D., Gonzalez, F., and Jara, S. (2015). Assessment of Trace Metals in Droppings of Adélie Penguins (Pygoscelis Adeliae) from Different Locations of the Antarctic Peninsula Area. Adv. Polar Sci. 26 (1), 1–7. doi:10.13679/j.advps.2015.1.00001
Chen, Z., Myers, R., Wei, T., Bind, E., Kassim, P., Wang, G., et al. (2014). Placental Transfer and Concentrations of Cadmium, Mercury, lead, and Selenium in Mothers, Newborns, and Young Children. J. Expo. Sci. Environ. Epidemiol. 24 (5), 537–544. doi:10.1038/jes.2014.26
Clotfelter, E. D., Bell, A. M., and Levering, K. R. (2004). The Role of Animal Behaviour in the Study of Endocrine-Disrupting Chemicals. Anim. Behav. 68, 665–676. doi:10.1016/j.anbehav.2004.05.004
Cobbina, S. J., Chen, Y., Zhou, Z., Wu, X., Zhao, T., Zhang, Z., et al. (2015). Toxicity Assessment Due to Sub-chronic Exposure to Individual and Mixtures of Four Toxic Heavy Metals. J. Hazard. Mater. 294, 109–120. doi:10.1016/j.jhazmat.2015.03.057
Cronje, H. P., Cronje, I., and Botha, A. J. (2005). The Distribution and Seasonal Availability of Surface Water on the Manyeleti Game Reserve, Limpopo Province, South Africa. Koedoe 48 (2), 11–21. doi:10.4102/koedoe.v48i2.93
Das, K. K., Honnutagi, R., Mullur, L., Reddy, R. C., Das, S., Majid, D. S. A., et al. (2019). “Heavy Metals and Low-Oxygen Microenvironment-Its Impact on Liver Metabolism and Dietary Supplementation,” in Dietary Interventions in Liver Disease : Foods, Nutrients, and Dietary Supplements. Editors R. R. Watson, and V. R. Preedy (London, San Diego, Cambridge, Oxford: Academic Press), 315–332. doi:10.1016/b978-0-12-814466-4.00026-4
Dresler, S., Wójciak-Kosior, M., Sowa, I., Strzemski, M., Sawicki, J., Kováčik, J., et al. (2018). Effect of Long-Term Strontium Exposure on the Content of Phytoestrogens and Allantoin in Soybean. Int. J. Mol. Sci. 19 (12), 3864. doi:10.3390/ijms19123864
Driscoll, C. T., and Schecher, W. D. (1990). The Chemistry of Aluminum in the Environment. Environ. Geochem. Health 12, 28–49. doi:10.1007/BF01734046
Driver, A., Sink, K. J., Nel, J. L., Holness, S., van Niekerk, L., Daniels, F., et al. (2011). National Biodiversity Assessment 2011: An Assessment of South Africa's Biodiversity and Ecosystems. Synthesis Report. Pretoria: South African National Biodiversity Institute and Department of Environmental Affairs. Available at: http://biodiversityadvisor.sanbi.org/wp-content/uploads/2016/07/NBA-2011-Synthesis-Report-low-resolution.pdf (Accessed March 11, 2018).
Du Toit, J. T., and Cumming, D. H. M. (1999). Functional Significance of Ungulate Diversity in African Savannas and the Ecological Implications of the Spread of Pastoralism. Biodivers. Conserv. 8, 1643–1661. doi:10.1023/A:1008959721342
Egorova, K. S., and Ananikov, V. P. (2017). Toxicity of Metal Compounds: Knowledge and Myths. Organometallics 36, 4071–4090. doi:10.1021/acs.organomet.7b00605
Eloff, F. C. (1973). Water Use by the Kalahari Lion (Panthera Leo Vernayi). Koedoe 16 (1), 149–154. doi:10.4102/koedoe.v16i1.891
Facemire, C. F., Gross, T. S., and Guillette, L. J. (1995). Reproductive Impairment in the Florida Panther: Nature or Nurture? Environ. Health Perspect. 103 (4), 79–86. doi:10.1289/ehp.103-1519283
FAOUNEP (2021). Global Assessment of Soil Pollution - Summary for Policy Makers. Rome: FAO. doi:10.4060/cb4827en
Ferreira, S. M., and Pienaar, D. (20112011). Degradation of the Crocodile Population in the Olifants River Gorge of Kruger National Park, South Africa. Aquat. Conserv. Mar. Freshw. Ecosyst. 21, 155–164. doi:10.1002/aqc.1175
Gall, J. E., Boyd, R. S., and Rajakaruna, N. (2015). Transfer of Heavy Metals through Terrestrial Food Webs: A Review. Environ. Monit. Assess. 187 (4), 201. doi:10.1007/s10661-015-4436-3
Ganswindt, A., Heistermann, M., Borragan, S., and Hodges, J. K. (2002). Assessment of Testicular Endocrine Function in Captive African Elephants by Measurement of Urinary and Fecal Androgens. Zoo Biol. 21, 27–36. doi:10.1002/zoo.10034
Gobas, F. A. P. C. (2008). “Food-Web Bioaccumulation Models,” in Encyclopedia of Ecology. Editors S. E. Jørgensen, and B. D. Fath (Canada: Elsevier Science), 1643–1652. doi:10.1016/b978-008045405-4.00398-0
Godinho, D. P., Serrano, H. C., Da Silva, A. B., Branquinho, C., and Magalhães, S. (2018). Effect of Cadmium Accumulation on the Performance of Plants and of Herbivores that Cope Differently with Organic Defenses. Front. Plant Sci. 9, 1723. doi:10.3389/fpls.2018.01723
Grant, C. C., Peel, M. J. S., Zambatis, N., and van Ryssen, J. B. J. (2000). Nitrogen and Phosphorus Concentration in Faeces: An Indicator of Range Quality as a Practical Adjunct to Existing Range Evaluation Methods. Afr. J. Range Forage Sci. 17 (12&3), 81–92. doi:10.2989/10220110009485743
Gulson, B. L., Mizon, K. J., Korsch, M. J., Palmer, J. M., and Donnelly, J. B. (2003). Mobilization of lead from Human Bone Tissue during Pregnancy and Lactation-Aa Summary of Long-Term Research. Sci. Total Environ. 303 (1-2), 79–104. doi:10.1016/s0048-9697(02)00355-8
Gupta, V., and Bakre, P. (2013). Metal Contamination in Mammalian Fauna of Sariska Tiger Reserve, Alwar, India. J. Ecophysiol. Occup. Health 12 (1-2), 43–48. doi:10.18311/jeoh/2012/1744
Hayward, M. W., and Kerley, G. I. H. (2008). Prey Preferences and Dietary Overlap Amongst Africa's Large Predators. South Afr. J. Wildl. Res. 38 (2), 93–108. doi:10.3957/0379-4369-38.2.93
Herselman, J. E. (2007). The Concentration of Selected Trace Metals in South African Soils. PhD Thesis. South Africa: University of Stellenbosch.
Hooser, S. B. (2018). “Iron,” in Veterinary Toxicology Basic and Clinical Principles. Editor R. C. Gupta. Third edition (London, San Diego, Cambridge, Oxford: Academic Press), 433–437. doi:10.1016/b978-0-12-811410-0.00028-3
Järup, L. (2003). Hazards of Heavy Metal Contamination. Br. Med. Bull. 68, 167–182. doi:10.1093/bmb/ldg032
Jadhav, S. H., Sarkar, S. N., Patil, R. D., and Tripathi, H. C. (2007). Effects of Subchronic Exposure via Drinking Water to a Mixture of Eight Water-Contaminating Metals: A Biochemical and Histopathological Study in Male Rats. Arch. Environ. Contam. Toxicol. 53 (4), 667–677. doi:10.1007/s00244-007-0031-0
Jaishankar, M., Tseten, T., Anbalagan, N., Mathew, B. B., and Beeregowda, K. N. (2014). Toxicity, Mechanism and Health Effects of Some Heavy Metals. Interdiscip. Toxicol. 7 (2), 60–72. doi:10.2478/intox-2014-0009
Kenston, S. S. F., Su, H., Li, Z., Kong, L., Wang, Y., Song, X., et al. (2018). The Systemic Toxicity of Heavy Metal Mixtures in Rats. Toxicol. Res. 7, 396–407. doi:10.1039/c7tx00260b
Kersey, D. C., and Dehnhard, M. (2014). The Use of Noninvasive and Minimally Invasive Methods in Endocrinology for Threatened Mammalian Species Conservation. Gen. Comp. Endocrinol. 203, 296–306. doi:10.1016/j.ygcen.2014.04.022
Knight, M. H. (2013). “Oryx gazella Gemsbok (Southern oryx),” in Mammals of Africa: Pigs, Hippopotamuses, Chevrotain, Giraffes, Deer and Bovids Vol VI. Editors J. Kingdon, and M. Hoffmann (London: Bloomsbury Publishing), 572–575.
Knoop, W. T., and Walker, B. H. (1985). Interactions of Woody and Herbaceous Vegetation in a Southern African Savanna. J. Ecol. 73, 235–253. doi:10.2307/2259780
Kohl, K. D., Coogan, S. C. P., and Raubenheimer, D. (2015). Do wild Carnivores Forage for Prey or for Nutrients? Bioessays 37 (6), 701–709. doi:10.1002/bies.201400171
Kortenkamp, A., and Faust, M. (2018). Regulate to Reduce Chemical Mixture Risk. Science 361 (6399), 224–226. doi:10.1126/science.aat9219
Kortenkamp, A., Faust, M., Backhaus, T., Altenburger, R., Scholze, M., Müller, C., et al. (2019). Mixture Risks Threaten Water Quality: the European Collaborative Project SOLUTIONS Recommends Changes to the WFD and Better Coordination across All Pieces of European Chemicals Legislation to Improve protection from Exposure of the Aquatic Environment to Multiple Pollutants. Environ. Sci. Eur. 31, 69. doi:10.1186/s12302-019-0245-6
Liebenberg, L. (1990a). The Art of Tracking: The Origin of Science. Cape Town, South Africa: David Philip Publishers and Creda Press.
Liebenberg, L. (1990b). The Field Guide to the Animal Tracks of Southern Africa. Cape Town and Johannesburg, South Africa: David Philip Publishers.
Martin, O., Scholze, M., Ermler, S., McPhie, J., Bopp, S. K., Kienzler, A., et al. (2021). Ten Years of Research on Synergisms and Antagonisms in Chemical Mixtures: A Systematic Review and Quantitative Reappraisal of Mixture Studies. Environ. Int. 146, 106206. doi:10.1016/j.envint.2020.106206
McNaughton, S. J., and Georgiadis, N. J. (1986). Ecology of African Grazing and Browsing Mammals. Annu. Rev. Ecol. Syst. 17, 39–66. doi:10.1146/annurev.es.17.110186.000351
Mpumalanga Tourism and Parks Agency (2015). Integrated Management Plan: Manyeleti Nature Reserve. South Africa: Mpumalanga Tourism and Parks Agency facilitated by NuLeaf and Environmental.
Nakamaru, Y., and Uchida, S. (2008). Agricultural Soils and the Factors Affection tin Sorption Behaviour. J. Environ. Radioactiv. 99 (6), 1003–1010. doi:10.1016/j.jenvrad.2007.11.012
Naidoo, V., Wolter, K., and Botha, C. J. (2017). Lead Ingestion as a Potential Contributing Factor to the Decline in Vulture Populations in Southern Africa. Environ. Res. 152, 150–156. doi:10.1016/j.envres.2016.10.013
Ogada, D., Botha, A., and Shaw, P. (2016). Ivory Poachers and Poison: Drivers of Africa's Declining Vulture Populations. Oryx 50 (4), 593–596. doi:10.1017/S0030605315001209
Ogada, D. L., Keesing, F., and Virani, M. Z. (2012). Dropping Dead: Causes and Consequences of Vulture Population Declines Worldwide. Ann. New York Acad. Sci. 1249, 57–71. doi:10.1111/j.1749-6632.2011.06293.x
Olaniran, A., Balgobind, A., and Pillay, B. (2013). Bioavailability of Heavy Metals in Soil: Impact on Microbial Biodegradation of Organic Compounds and Possible Improvement Strategies. Int. J. Mol. Sci. 14, 10197–10228. doi:10.3390/ijms140510197
Palma-Lara, I., Martínez-Castillo, M., Quintana-Pérez, J. C., Arellano-Mendoza, M. G., Tamay-Cach, F., Valenzuela-Limón, O. L., et al. (2020). Arsenic Exposure: A Public Health Problem Leading to Several Cancers. Regul. Toxicol. Pharmacol. 110, 104539. doi:10.1016/j.yrtph.2019.104539
Palme, R. (2019). Non-invasive Measurement of Glucocorticoids: Advances and Problems. Physiol. Behav. 199, 229–243. doi:10.1016/j.physbeh.2018.11.021
Pathak, P., and Gupta, D. K. (2020). “Strontium Contamination in the Environment,” in The Handbook of Environmental Chemistry. Editors P. Pathak, and D. K. Gupta (Switzerland: Springer International Publishing).
R Core Team (2019). R: A Language and Environment for Statistical Computing. Vienna, Austria: R Foundation for Statistical Computing. Available at: https://www.R-project.org/(Accessed May 11, 2020).
Redfern, J. V., Grant, R., Biggs, H., and Getz, W. M. (2003). Surface-Water Constraints on Herbivore Foraging in the Kruger National Park, South Africa. Ecology 84, 2092–2107. doi:10.1890/01-0625
Reglero, M. M., Monsalve-González, L., Taggart, M. A., and Mateo, R. (2008). Transfer of Metals to Plants and Red Deer in an Old Lead Mining Area in Spain. Sci. Total Environ. 406 (1-2), 287–297. doi:10.1016/j.scitotenv.2008.06.001
Rhind, S. M. (2009). Anthropogenic Pollutants: a Threat to Ecosystem Sustainability? Phil. Trans. R. Soc. B 364 (1534), 3391–3401. doi:10.1098/rstb.2009.0122
Richards, N. L., Hall, S. W., Harrison, N. M., Gautam, L., Scott, K. S., Dowling, G., et al. (2014). Merging Wildlife and Environmental Monitoring Approaches with Forensic Principles: Application of Unconventional and Non-invasive Sampling in Eco- Pharmacovigilance. J. Forensic Res. 05 (3), 1000228. doi:10.4172/2157-7145.1000228
Rodríguez-Jorquera, I. A., Vitale, N., Garner, L., Perez-Venegas, D. J., Galbán-Malagón, C. J., Duque-Wilckens, N., et al. (2017). Contamination of the Upper Class: Occurrence and Effects of Chemical Pollutants in Terrestrial Top Predators. Curr. Pollut. Rep. 3, 206–219. doi:10.1007/s40726-017-0061-9
Rzymski, P., Tomczyk, K., Rzymski, P., Poniedziałek, B., Opala, T., and Wilczak, M. (2015). Impact of Heavy Metals on the Female Reproductive System. Ann. Agric. Environ. Med. 22 (2), 259–264. doi:10.5604/12321966.1152077
Sach, F., Yon, L., Henley, M. D., Bedetti, A., Buss, P., de Boer, W. F., et al. (2020). Spatial Geochemistry Influences the Home Range of Elephants. Sci. Total Environ. 729, 139066. doi:10.1016/j.scitotenv.2020.139066
Shanker, A., Cervantes, C., Lozatavera, H., and Avudainayagam, S. (2005). Chromium Toxicity in Plants. Environ. Int. 31, 739–753. doi:10.1016/j.envint.2005.02.003
Shorrocks, B., and Bates, W. (2015). The Biology of African Savannahs, Biology of Habitats. Illustrated and Revised. edn. Oxford, London: Oxford University Press.
Spies, A. (2018). Kruger National Park, Park Management Plan 2018-2028. Available at: https://www.sanparks.org/assets/docs/conservation/park_man/knp/knp-approved-plan.pdf (Accessed October 12, 2019).
STAP (2012). GEF Guidance on Emerging Chemicals Management Issues in Developing Countries and Countries with Economies in Transition. A STAP Advisory Document. Washington DC: The Scientific and Technical Advisory Panel of the Global Environment Facility.
Streit, B. (1992). Bioaccumulation Processes in Ecosystems. Experientia 48, 955–970. doi:10.1007/BF01919142
Thrupp, T. J., Runnalls, T. J., Scholze, M., Kugathas, S., Kortenkamp, A., and Sumpter, J. P. (2018). The Consequences of Exposure to Mixtures of Chemicals: Something from 'nothing' and 'a Lot from a Little' when Fish Are Exposed to Steroid Hormones. Sci. Total Environ. 619-620, 1482–1492. doi:10.1016/j.scitotenv.2017.11.081
United Nations Office on Drugs and Crime (2009). Guidance for theValidation of Analytical Methodology and Calibration of Equipment Used for Testing of Illicit Drugs in Seized Materials and Biological Specimens. Vienna ,United Nations ,New York: Laboratory and Scientific Section UNODC.
United States Environmental Protection Agency (2007). Method 3051a (SW-846): Microwave Assisted Acid Digestion of Sediments, Sludges, Soils, and Oils, Part of Test Methods for Evaluating Solid Waste, Physical/Chemical Methods. Revision 1. Washington DC. Available at: https://www.epa.gov/esam/us-epa-method-3051a-microwave-assisted-acid-digestion-sediments-sludges-and-oils (Accessed April 24, 2020).
van Rooyen, N., and van Rooyen, G. (2017). Ecological Evaluation of Tswalu Kalahari Reserve. An Ecological Report compiled by Ecotrust CC.
Varentsov, I. M., and Kuleshov, V. N. (2019). Rare Elements-Markers of the Formation Setting of Manganese and Iron Ores in the Kalahari and Postmasburg Manganese Fields (South Africa): Communication 2. Postmasburg Iron and Manganese Field. Lithol. Miner. Resour. 54 (5), 412–428. doi:10.1134/s0024490219050067
Vaughn, N. R., Asner, G. P., Smit, I. P. J., and Riddel, E. S. (2015). Multiple Scales of Control on the Structure and Spatial Distribution of Woody Vegetation in African Savanna Watersheds. PLoS One 10 (12), e0145192. doi:10.1371/journal.pone.0145192
Walraven, F. (1986). The Timbavati Gabbro of the Kruger National Park. Koedoe 29 (1), a521. doi:10.4102/koedoe.v29i1.521
Warne, R. W. (2014). The Micro and Macro of Nutrients across Biological Scales. Integr. Comp. Biol. 54 (5), 864–872. doi:10.1093/icb/icu071
Webster, A. B., Burroughs, R. E., Laver, P., and Ganswindt, A. (2018). Non-invasive Assessment of Adrenocortical Activity as a Measure of Stress in Leopards Panthera pardus. Afr. Zoolog. 53 (2), 53–60. doi:10.1080/15627020.2018.1467280
Webster, A. B., Rossouw, R., Callealta, F. J., Bennett, N. C., and Ganswindt, A. (2021a). Assessment of Trace Element Concentrations in Sediment and Vegetation of Mesic and Arid African Savannahs as Indicators of Ecosystem Health. Sci. Total Environ. 760, 143358. doi:10.1016/j.scitotenv.2020.143358
Webster, A. B., Callealta, F. J., Ganswindt, A., and Bennett, N. C. (2021b). A Non-invasive Assessment of Essential Trace Element Utilization at Different Trophic Levels in African Wildlife. J. Environ. Manage. 293, 112820. doi:10.1016/j.jenvman.2021.112820
Webster, A. B., Ganswindt, A., Small, C., Rossouw, R., Ganswindt, A., and Bennett, N. C. (2021c). Optimised ICP-MS Quantification Method for Using Animal Faeces as a Measure of Protected Area Ecosystem Health. MethodsX 8, 101441. doi:10.1016/j.mex.2021.101441
Woodborne, S., Huchzermeyer, K. D. A., Govender, D., Pienaar, D. J., Hall, G., Myburgh, J. G., et al. (2012). Ecosystem Change and the Olifants River Crocodile Mass Mortality Events. Ecosphere 3 (10), art87–17. doi:10.1890/ES12-00170.1
World Health Organisation (2019). Arsenic. Available at: https://www.who.int/news-room/fact-sheets/detail/arsenic (Accessed October 19, 2020).
World Health Organisation/International Programme on Chemical Safety (2002). Global Assessment of the State-Of-The-Science-Of Endocrine Disruptors: An Assessment Prepared by an Expert Group on Behalf of the World Health Organisation, the International Labour Organisation, and the United Nations Environment Programme. Editors T. Damstra, S. Barlow, A. Bergman, R. Kavlock, and G. Van Der Kraak, Geneva, Switzerland. Available at: https://www.who.int/ipcs/publications/new_issues/endocrine_disruptors/en/ (Accessed December 11, 2017).
World Health Organisation/International Agency for Research on Cancer (1990). Chromium and Chromium Compounds. IARC Monographs on the Evaluation of Carcinogenic Risks to Humans, 49. Lyon, France: IARC Working Group on the Evaluation of Carcinogenic Risks to Human, 49–256. Available at: https://monographs.iarc.fr/wp-content/uploads/2018/06/mono49.pdf (Accessed May 23, 2018).
World Health Organisation/United Nations Environment Programme (2012). State of the Science of Endocrine Disrupting Chemicals: An Assessment of the State of the Science of Endocrine Disruptors Prepared by a Group of Experts for the United Nations Environment Programme and World Health Organisation. Editors A. Bergman, J. J. Heindel, S. Jobling, K. A. Kidd, and R. T. Zoeller (Geneva, Switzerland: WHO). Available at: https://www.who.int/ceh/publications/endocrine/en/(Accessed December 14, 2018).
World Weather Online (2020a). World Weather Online Skukuza, Kruger National Park, Mpumalanga Province. Available at: https://www.worldweatheronline.com/lang/en-in/skukuza-weather-averages/mpumalanga/za.aspx (Accessed July 30, 2020).
World Weather Online (2020b). World Weather Online Van Zylsrus, Northern Cape Province. Available at: https://www.worldweatheronline.com/van-zylsrus-weather-averages/northern-cape/za.aspx (Accessed July 30, 2020).
Keywords: environmental pollution, potentially toxic elements (PTEs), non-invasive risk assessment, animal faeces, non-lethal risk assessment, protected areas (PA), African savannah
Citation: Webster AB, Callealta JF, Bennett NC and Ganswindt A (2022) Non-Lethal Assessment of Potentially Toxic Elements Across Mammalian Trophic Levels in African Savannahs. Front. Environ. Sci. 9:794487. doi: 10.3389/fenvs.2021.794487
Received: 13 October 2021; Accepted: 27 December 2021;
Published: 27 January 2022.
Edited by:
Denina Bobbie Dawn Simmons, Ontario Tech University, CanadaReviewed by:
Jean Remy Davee Guimaraes, Federal University of Rio de Janeiro, BrazilAndrew Hursthouse, University of the West of Scotland, United Kingdom
Copyright © 2022 Webster, Callealta, Bennett and Ganswindt. This is an open-access article distributed under the terms of the Creative Commons Attribution License (CC BY). The use, distribution or reproduction in other forums is permitted, provided the original author(s) and the copyright owner(s) are credited and that the original publication in this journal is cited, in accordance with accepted academic practice. No use, distribution or reproduction is permitted which does not comply with these terms.
*Correspondence: Andrea B. Webster, YW5kcmVhLndlYnN0ZXJAdHVrcy5jby56YQ==