- Laboratory of Environmental Ecology, Department of Bioscience and Biotechnology, Chungnam National University, Daejeon, South Korea
Trait-based functional studies are widely used to elucidate the relationships between ecological indicators and environmental parameters as well as to predict functional change in aquatic biota in response to various types of human disturbance. Clarifying how functional traits of aquatic organisms depend on environmental conditions can facilitate aquatic conservation and management, but determining the importance of these traits to ecological river health requires further investigation. As fish play a key role in the assessment of ecological conditions, we examined the relevance of the functional diversity of lotic fish to the river health assessment using multi-metric models of water pollution (mWPI) and fish-based biological integrity (mIBI). Twelve fish traits related to food acquisition, environmental stability, and mobility were used for the functional analyses. Chemical river health was highly sensitive to downstream organic matter and nutrient pollution according to mWPI. Based on the present gradient of chemical health and water chemical variables, we identified three water quality groups (G-I, G-II, and G-III). G-I, G-II, and G-III showed low, intermediate, and high levels of water quality degradation, respectively. Spatially significant differences among these groups were observed for both the taxonomic and functional structures of lotic fish as well as ecological river health based on mIBI. The dominance of sensitive species was high in G-I, whereas tolerant and exotic species contributed strongly to the species compositions of G-II and G-III. Functional richness and dispersal were significantly reduced in G-III, and their decreases correlated with ecological health and the loss of species that are insectivorous, rheophilic, and sensitive to water pollution. Regarding redundancy analyses, both the models of functional trait metrics (F = 8.06, p < 0.001) and mIBI metrics (F = 4.88, p < 0.01) indicated good performance in terms of the variation in water quality and chemical river health parameters. Overall, the functional trait-based diversity of lotic fish is significant to the assessment of ecological river health and reflects water chemical quality. This association arises because niche occupation in functional space by all species, along with their abundance distribution, is highly responsive to the loss of species with sensitive traits due to water pollution.
1 Introduction
A number of types of human-induced disturbance are rapidly intensifying, endangering the balance of interactions between biotic and abiotic components of lotic ecosystems (Dudgeon et al., 2006; Strayer and Dudgeon, 2010). In recent years, the functional trait approach has been used in a wide range of ecological studies to describe and predict the responses of aquatic communities to anthropogenic disturbances, such as water pollution (Péru and Dolédec, 2010; Pallottini et al., 2017; Paz et al., 2022), fragmentation (Pool et al., 2010; Oliveira et al., 2018; Wang et al., 2022), flow regime modification (Kelley et al., 2018; Belmar et al., 2019; Wu et al., 2019), invasion by exotic species (Matsuzaki et al., 2016; Toussaint et al., 2018; Milardi et al., 2019), and climate change (Buisson et al., 2013; Boucek and Rehage, 2014; Galego de Oliveira et al., 2019). This method is adopted because the functional diversity of biotic communities relies heavily on trait-specific interactions between species and the environment (McGill et al., 2006; Frimpong and Angermeier, 2010; Péru and Dolédec, 2010; Kelley et al., 2018).
Trait-based studies have covered various aquatic taxa, such as phytoplankton (Pálffy et al., 2013; Du et al., 2022), periphyton (Bichoff et al., 2018; Wang et al., 2022), zooplankton (Kuczyńska-Kippen et al., 2020; Shen et al., 2022), macroinvertebrates (Pallottini et al., 2017; Belmar et al., 2019), and fish (Brind’Amour et al., 2011; Toussaint et al., 2016). They have also examined diverse geographic regions, including North America (Boucek and Rehage, 2014; Sinclair et al., 2021), Europe (Belmar et al., 2019; Wu et al., 2019), Australia (Galego de Oliveira et al., 2019; Lam-Gordillo et al., 2020), and Asia (Matsuzaki et al., 2016; Wang et al., 2022). Functional diversity is generally governed by two deterministic processes underlying community assembly (Mouchet et al., 2010). Competitive exclusion limits the similarity of co-occurring species, resulting in stable coexistence of functionally dissimilar species within communities (MacArthur and Levins, 1967; Mason et al., 2007). Environmental filtering for traits, by contrast, strongly governs the functional diversity of communities (Cornwell et al., 2006; Mouillot et al., 2013; Kraft et al., 2015). According to this process, each human-induced disturbance is expected to filter for species with a certain suite of traits that support adaptation to the altered habitat and exclude species with unsuitable traits (McGill et al., 2006; Sinclair et al., 2021). Loss of functional diversity and functional homogenization can be observed in the affected aquatic communities (Toussaint et al., 2016; Villéger et al., 2017). Therefore, the use of trait-based models and functional indices can facilitate the assessment of ecological river health. However, studies of the relationship between ecological river health and the trait-based diversity of aquatic fauna remain insufficient.
Diagnosing ecological river health is essential to maintaining the structure and function of ecosystems and the well-being of human society (Hering et al., 2006). The river health is generally evaluated based on physicochemical (flow regime, habitat characteristics, and water quality) and biological components of lotic habitats through comparison to reference conditions (Hering et al., 2006; Choi et al., 2011). Water chemical quality, which is strongly related to various anthropogenic pollutants (Sabater et al., 2018; Stets et al., 2020), is a significant factor to consider when selecting reference sites for the assessment of ecological health (DEHP, 2009). Multi-metric models of biological indicators, such as diatoms, macroinvertebrates, and fish, are widely used to evaluate the ecological consequences of human activity in lotic systems (Karr, 1981; Ruaro et al., 2020). Because they evaluate the integrated responses of taxonomic richness, abundance, trophic guilds, and tolerance guilds to change in water physicochemical properties, indices of biological integrity (mIBI) can support accurate evaluation of ecological river health (Hering et al., 2006; Karr et al., 2022). However, this approach must be continually improved by linking with the finding of more responsible candidates for measuring environmental change. The selection of metrics is essential for ecological interpretations of mIBI (Ruaro et al., 2020). Furthermore, functional diversity indices may enhance the performance of mIBIs by providing more detailed insights into species-environment relationships. Functional changes in community structure is commonly assessed by several complementary indices, which are functional richness (F-Rich), functional evenness (F-Eve), and functional divergence (F-Div) (Villéger et al., 2008). Also, functional mean pairwise distance (F-Mpd) and functional dispersal (F-Dis) can indicate the community variations due to abiotic conditions (Weiher et al., 1998; Laliberté and Legendre, 2010).
Fish assemblages in lotic systems can provide reliable information about changes in biological structure and function associated with environmental factors and human-induced disturbances (Schinegger et al., 2016; Simon and Evans, 2017). The diversity and abundance of fish are generally influenced by the availability of resources and the heterogeneity of habitats along downstream gradients (Matthews, 1998; Muneepeerakul et al., 2008). Functional changes in fish communities also correspond to the continuous gradient of physical characteristics from upstream to downstream locations (Vannote et al., 1980; Aarts and Nienhuis, 2003; Pease et al., 2012). However, the taxonomic and functional compositions of fish assemblages are highly vulnerable to human-induced disturbances, which act as environmental filters on biological communities (Kruk, 2007; Hermoso et al., 2011; Keck et al., 2014). Water pollution causes major disturbances to the physicochemical environment, affecting its suitability for the health of lotic fish (Dudgeon et al., 2006; Whitney et al., 2019). Elevated concentrations and loads of water chemical parameters arising from point and non-point sources, in particular nutrients and organic matter, can greatly affect the functional composition (through tolerance and trophic guilds) and diversity of fish, thereby reducing ecological river health (Chalar et al., 2013; Gao et al., 2015; HaRa et al., 2019). Other anthropogenic disturbances, including fragmentation, flow regime change, and invasive species, co-occur in most lotic systems affected by water pollution (Dudgeon et al., 2006; Whitney et al., 2019; Atique et al., 2020). Accordingly, the responses of fish assemblage structures to water quality parameters can provide fundamental information about co-occurring human impacts on rivers. From this perspective, functional trait analyses (functional diversity indices, trait-based model) of lotic fish may play an important role in the assessment of ecological river health, reflecting water chemical quality. Particularly, as river health decreases due to water pollution, the functional diversity indices are likely to decline caused by losses of specialist fish (or functionally distinct) distribution within lotic systems.
Using a continuous data set of fish and water quality data collected from 2011 to 2016, we examined the use of functional diversity components (functional traits and indices) for evaluating ecological river health and compared multi-metric models of biological integrity (mIBI) and water pollution (mWPI). We hypothesized that the composition of functional traits is strongly correlated with change in water chemical quality, a loss of functional diversity in polluted sites results in a decrease in species with traits that are sensitive to water pollution, functional indices and mIBI are complementary and effective measures for assessing change in water chemical quality and chemical river health, and integrating different methods of assessing river health is useful for river conservation and management. The studied river provided a suitable background for addressing these hypotheses. Several sources of water pollution co-occur in the studied watershed, including intense agricultural activity and weirs, urban-industrial runoff, and the release of sewage water. These activities have led to elevated organic matter and nutrient levels and loads in the river, resulting in water quality degradation.
2 Materials and methods
2.1 Study area
The Mangyeong River flows through the northern part of the Honam Plain, located in the western lowland region of South Korea. It has a length of 80.86 km and a watershed area of 1,602 km2 and has been widely used for irrigation and transportation since ancient times. A total of eight sites were selected for this study (Figure 1), of which four are located in the main stem of the river (M1, M2, M3, and M4) and four are in its tributaries (T1, T2, T3, and T4). Stream order classification was based on the method of Strahler (1957).
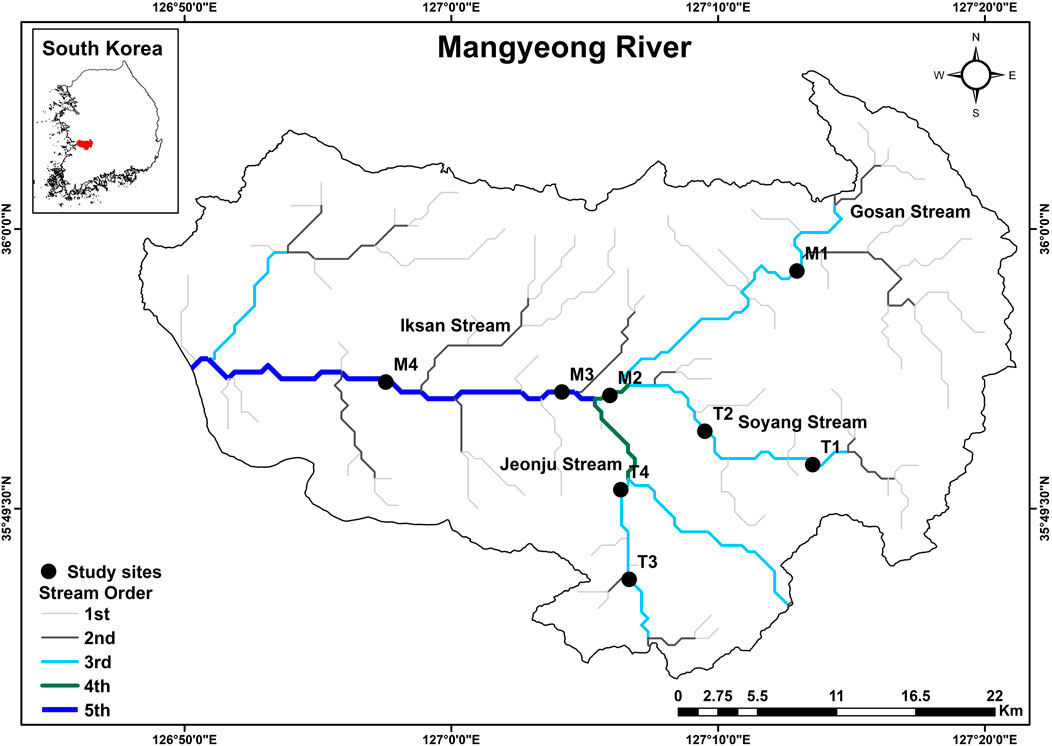
FIGURE 1. Mangyeong River watershed and the locations of the eight study sites, showing stream order.
M1 is the uppermost site in our study and in a third-order stream, which arises from the merging of mountainous streams. T1 and T2 are in a third-order stream; these two tributary sites are located in Soyang Stream. The streambed at M1 and T1 is mainly composed of large and small stones and gravel, and the natural stream shape is well preserved. Forest inputs from surrounding areas are predominant at these sites, and rapids are present in both the upstream and downstream sections. M2 is a fourth-order stream located upstream of the merge with Jeonju Stream, where water quality deteriorates because of civil sewage and industrial wastewater from Jeonju City. At this station, nearby agricultural land is considered the main source of pollution. Two sites (T3 and T4) along an urban-industrial tributary are both in a third-order stream, with the site T4 located in an urban-industrial area. M3 is located downstream of the Jeonju Stream inflow and is a fifth-order stream. The riverbed consists mainly of gravel and sand and is well developed. Water quality is likely impacted by inflow from an urban-industrial stream. M4, the most downstream location studied, is along a fifth-order river. The site is located downstream of Iksan Stream, where effluent from a livestock manure treatment plant has deteriorated water quality. The deterioration of water quality at this site is a concern because of the infiltration of water from livestock manure treatment as well as large inputs from cropland surrounding upstream tributaries. The land use changes within the river watershed were shown in Supplementary Figure S1.
2.2 Fish sampling
Fish were sampled twice at each of the eight study sites during two seasons (spring: May–June and fall: September–October) from 2011 to 2016, when river flow was relatively stable. In Korea, the water level and velocity in rivers rise sharply during summer (July–August), which is the monsoon period, when around 60% of total annual precipitation occurs. Previous studies of aquatic ecosystems in Korea have demonstrated that heavy precipitation during the monsoon season leads to highly altered chemical conditions, habitat disturbances, changes in the diversity of aquatic organisms, and a shift in aquatic population dynamics. For this reason, fish sampling was not conducted during the summer monsoon period. A modified wading method (MOE/NIER, 2008) based on the US. EPA method (Barbour, 1999) was the basic approach used for sampling. Sampling gear included cast nets (mesh size: 4 × 4 mm) and hand nets (mesh size: 2 × 2 mm). Fish sampling and handling techniques were based on catch per unit effort methods (Klemm, 1993), with a sampling period of 50 min at each location. All sites selected for this study corresponded to national long-term chemical monitoring sites.
2.3 Water quality data
Monthly data for 11 chemical variables observed from 2011 to 2016 were used to assess water quality. Water sampling and analyses were conducted twice per month by the National Institute of Environmental Research (Korea). Water samples were collected in 5 L polyethylene bottles for chemical analysis and kept in a dark icebox during transport. Water temperature (WT) and electrical conductivity (EC) were measured in situ with multi-parameter water quality sensors (YSI Sonde Model 6600; YSI Incorporated, Yellow Springs, OH, United States). Total nitrogen (TN) was determined through ultraviolet spectrophotometry followed by potassium sulfate digestion. Phosphorus contents were determined using unfiltered (for total phosphorus [TP]) and filtered (for total dissolved phosphorus [TDP]) water digested with ascorbic acid after persulfate oxidation. Other measured parameters included 5-day biological oxygen demand (BOD), chemical oxygen demand (COD), total suspended solids (TSS), and sestonic chlorophyll-a (Chl-a) assessed using standard methods (APHA, 2005). Nutrient analyses were performed in triplicate, and BOD, COD, and TSS were measured in duplicate.
2.4 Biodegradability index and river trophic state
The biodegradability index (BI), a measure of the impacts of wastewater, is the ratio between BOD and COD. According to this index (Martín et al., 2006), biodegradability is categorized as high (>0.4), moderate (0.2–0.4), or low (<0.2). In lotic systems, trophic state is generally characterized using TN, TP, and Chl-a. Because of the relatively high concentrations of TN in Korean rivers, trophic state classification in this study was based on TP and Chl-a according to Dodds et al. (1998). Based on TP, the boundaries of oligotrophic-mesotrophic and mesotrophic-eutrophic states are 25 and 75 μg/L, respectively. Also, the trophic state boundaries are determined as Chl-a concentration at 10 μg/L (oligotrophic-mesotrophic) and 30 μg/L (mesotrophic-eutrophic).
2.5 Integrated assessment of river health based on mWPI and mIBI
The chemical health was evaluated using the multi-metric model of water pollution (mWPI) according to our previous research (Kim and An, 2015). The mWPI included seven metrics related to four chemical health indicators. Each metric was assigned a score of 5, 3, or 1 based on measured concentrations (Supplementary Table S1). The metrics were M1: TN (mg/L), M2: TP (µg/L), M3: TN:TP ratio, M4: BOD (mg/L), M5: TSS (mg/L), M6: EC (µS/cm), and M7: Chl-a (µg/L). The overall chemical health of the river was assessed as the sum of the scores for all metrics and categorized as excellent (31–35), good (25–29), fair (19–23), poor (13–17), or very poor (7–11).
The multi-metric IBI model (mIBI) was used to determine ecological river health, which was developed by An et al. (2006). The last metric of the original mIBI (percent of individuals with anomalies) was modified to reflect the percent of exotic carnivore species due to the following reasons. First, the information on individuals with anomalies was not properly available for study sites. Second, the previous study showed that the percentage of exotic carnivore species can be a good metric for river health assessment (Atique et al., 2019). Accordingly, the mIBI included eight metrics representing three fish indicators (Supplementary Table S2). The metrics were M1: total number of native fish species, M2: number of riffle-benthic species, M3: number of sensitive species, M4: percentage of individuals that are tolerant species, M5: percentage of individuals that are omnivorous species, M6: percentage of individuals that are native insectivorous species, M7: total number of native individuals, and M8: percentage of individuals that are exotic carnivorous species. Each metric was assigned a value of 5, 3, or 1 reflecting low, moderate, or high anthropogenic impact on the fish community in a lotic system, respectively (Simon and Evans, 2017). The expected values of M1–M3 and M7 vary by stream order. Based on the total mIBI score, the ecological health was categorized as excellent (36–40), good (28–34), fair (20–26), poor (14–18), or very poor (8–13).
2.6 Functional traits and functional diversity analysis
Twelve metrics of five fish traits, including traits related to food acquisition, environmental stability, and mobility, were identified for each species and used to calculate functional diversity (Table 1). The selection of fish traits was based on their likelihood to respond to disturbance associated with eutrophication and organic pollution. Trait values and metrics were extracted from a regional identification guide (Kim and Park, 2002) and a global fish database (http://www.fishbase.org). Details of the functional trait metrics for each species are available in Supplementary Table S3.
Five functional diversity indices of fish were estimated from the trait-based distance matrix and relative abundance (RA) of species, namely, functional richness (F-Ric), functional evenness (F-Eve), functional divergence (F-Div), functional dispersal (F-Dis), and functional mean pairwise distance (F-Mpd). Trait-based dissimilarities among fish species were based on the Gower distance, as categorical traits were selected for analyses (Gower, 1971). Principal coordinate analysis (PCoA) was performed to illustrate the multidimensional functional space filled by all species in a community, and the highest quality functional space was determined with the index of mean absolute deviation (MAD) that better reflects the inaccuracy of species distances in PCoA coordinates. And so a low value on the index indicates a high-quality functional space or PCoA coordinates (Magneville et al., 2022). Accordingly, the best functional space was a three-dimensional PCoA in this study (Supplementary Table S4). We calculated the functional indices using the functional space.
F-Ric is the volume of a convex hull (or functional space) formed by species positions along trait-based PCoA coordinates (Cornwell et al., 2006). F-Eve represents the regularity of species positions and abundance along the minimum spanning tree in functional space (Villéger et al., 2008). The minimum spanning tree links the positions of all species in functional space. F-Div is the deviation in the proportion of total abundance supported by the species with the most extreme traits (i.e., the species farthest from the center of gravity in functional space; Villéger et al., 2008). F-Dis is the average abundance-weighted species distance from the centre of gravity in functional space (Laliberté and Legendre, 2010). F-Mpd is the abundance-weighted mean distance between all pairs of species in functional space (Weiher et al., 1998).
2.7 Statistical analysis
Principal component analysis (PCA) was performed on water chemical indicators, trophic state, and river chemical health to detect spatial cluster groups for water quality. Group detection was based on the scores of study sites for the first two components (with 95% confidence intervals for each defined group) that explained the most variation in water chemical condition among sites. All analyses (Gower distance, PCoA, MAD) and calculations of functional indices were performed with the mFD package in the R program. This package was recently developed to provide a broad set of functions including all steps necessary for functional distance-based analysis (Magneville et al., 2022). Redundancy analysis (RDA) was used to indicate the responses of fish functional indices, functional trait composition, and the metrics of the fish-based mIBI to water quality variables and chemical river health. To examine correlations among functional indices, mIBI, and mWPI, Pearson analyses were performed on their seasonal values at study sites. All variance analyses in this study used Tukey’s test, which is a single-step multiple comparison procedure.
3 Results
3.1 River chemistry and pollution
3.1.1 River chemical indicators and health
Variation in water chemical variables showed a clear longitudinal gradient from upstream to downstream along the axes of both the main river and its tributaries. Using boxplots (Figure 2, Supplementary Figure S2), we identified the distribution of each chemical indicator for each site and determined statistical differences among sites (p < 0.05).
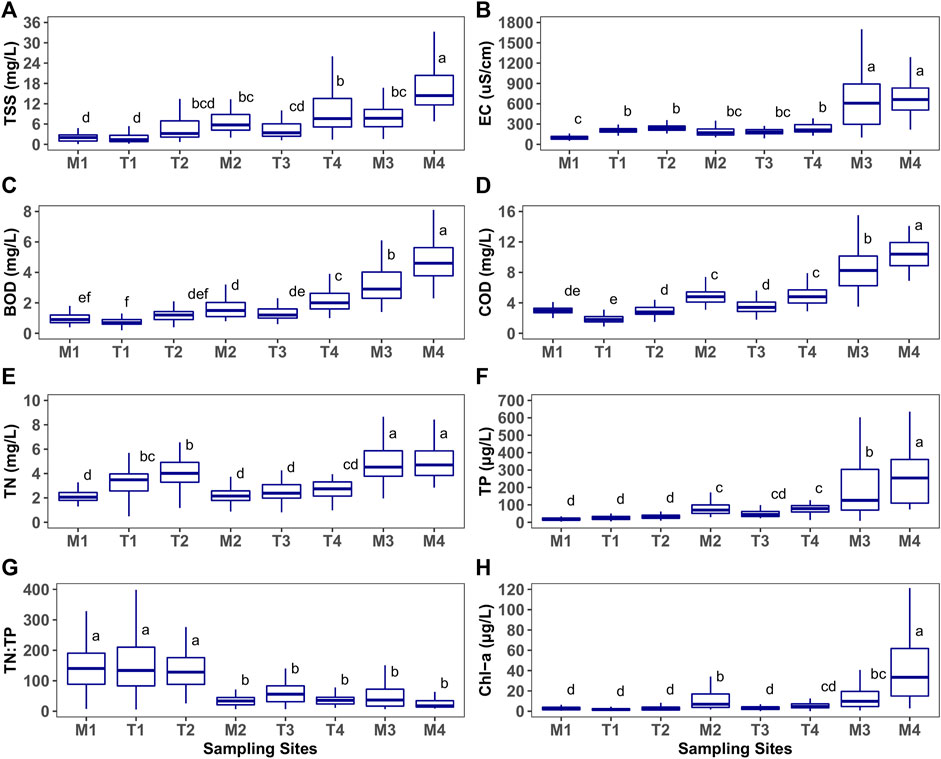
FIGURE 2. Spatial variations of water chemical indicators (TSS, total suspended solids; EC, electrical conductivity; BOD, biological oxygen demand; COD, chemical oxygen demand; TN, total nitrogen; TP, total phosphorus; TN:TP ratio; Chl-a, chlorophyll-a). For each variable, mean values labeled with the same letter do not differ significantly.
TSS was significantly lower at M1 and T1, but higher at M4 (Figure 2A), than at other sites. It varied relatively little among other study sites. The ionic content indicator (EC) showed significantly higher levels at M3 and M4 than at other sites (Figure 2B). The organic matter indicators (BOD, COD, and TOC) had relatively low levels at T1 and increased significantly downstream, in particular at M2, T4, M3, and M4 (Figures 2C,D). The nutrient indicators were significantly higher at the two downstream sites (M3 and M4) in the main river (Figures 2E,F). By contrast, the nutrient ratios (TN:TP and TDN:TDP) were relatively high at M1, T1, and T2 and dropped downstream of those sites. Excluding M1, the primary productivity indicator (Chl-a) tended to be higher at main stem sites (M2–M4) than in tributaries (T1–T4). And the large increase of Chl-a observed at the last site (Figure 2H).
The trophic state based on TP and Chl-a increased greatly in the downstream direction. This pattern was clear along the axes of both the main river and its tributaries (Figures 3A,B). Also, the river generally had high biodegradability according to the ratio of BOD to COD or the BI (Figure 3C). The chemical health of the river, measured using mWPI, was highly sensitive to organic matter and nutrient pollution in the downstream area (Figure 3D). Finally, the spatial differences of chemical indicators, trophic state and chemical health supported the possibility of grouping the sites based on water quality.
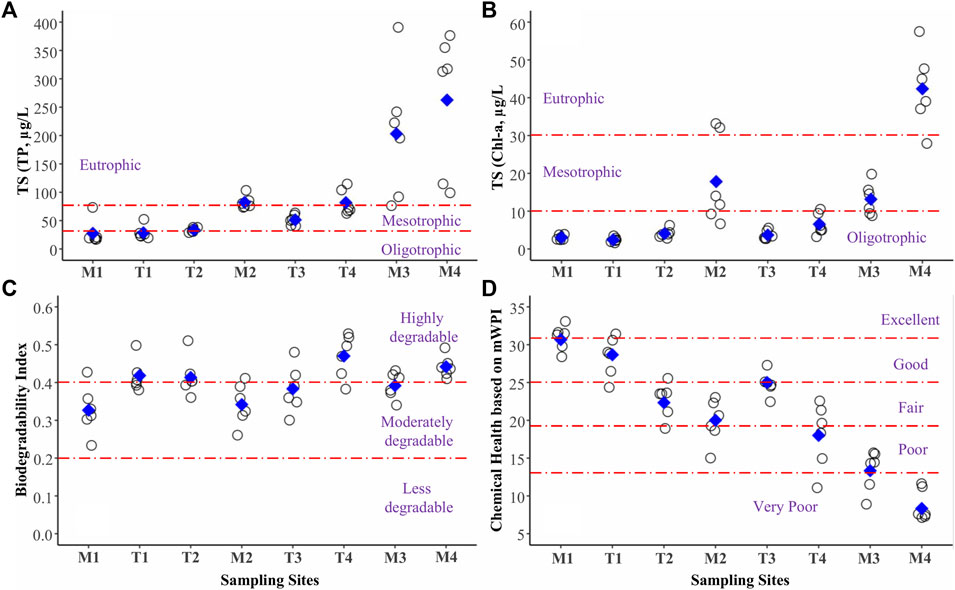
FIGURE 3. Spatial variations of river trophic state based on TP (A) and Chl-a (B), biodegradability (C), and chemical health using mWPI (D).
3.1.2 Spatial water quality groups
We identified three spatial water quality groups based on the scores of study sites along the first axis (or component) of the PCA (Figure 4). Biplots depicting the first two significant components (PC 1 and PC 2) were used to illustrate the findings. PC 1 explained 64.8% of the variance in the parameters among all sites. PC 2 explained a further 14.4% of the variance, and the cumulative explained variance was 79.2%. Along PC 1, all variables showed strong loadings, either negative or positive. The variables with negative loadings, which were TN:TP, TDN:TDP, and mWPI, were strongly correlated with Group–I (G - I), which supported our reference group for water chemical quality. G - I included sites M1, T1, T2, and T3. By contrast, the variables with strong positive loadings, including TSS, EC, nutrients, organic matter, and Chl-a, were positively correlated with Group–III (G - III). This indicates that the chemical water quality for this group had depreciated observably due to disturbances associated with pollution in the river. G - III included sites M3 and M4. Group–II (G - II) contained sites of M2 and T4 with moderate chemical water quality and did not have an explanation of variables (Figure 4).
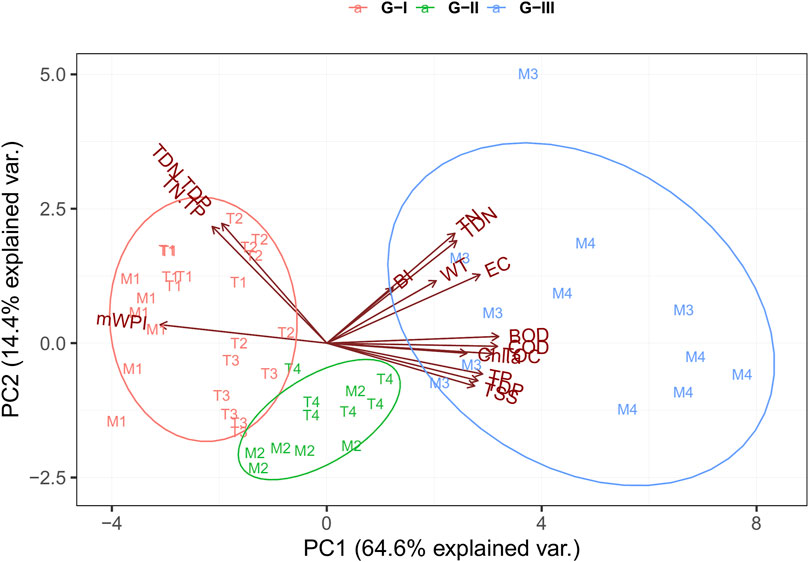
FIGURE 4. Spatial water quality groups based on trophic state, chemical health, and variations of chemical variables through PCA.
3.2 Analysis of fish communities and ecological river health
A total of 8.084 individuals representing 54 species were sampled from 2011 to 2016. The riverine fish community was dominated by Zacco platypus (36.4%), Zacco koreanus (6.7%), and Pseudogobio esocinus (6.5%) based on the total number of individuals (NI) collected throughout the investigation. A spatial-seasonal summary of fish composition and functional diversity indices, as well as ecological health, is provided in Supplementary Table S5. The following fish community analyses were conducted with the water quality groups identified through PCA.
3.2.1 Fish composition and ecological health
Observable differences in fish diversity, abundance, and species composition were found among the water quality groups (Figure 5). The total number of species (TNS) was 49, 29, and 25 in G - I, G - II, and G - III, respectively. The NI was high in G - I (1,407 individuals), moderate in G - II (778 individuals), and low in G - III (457 individuals) when averaged among sites within each group. Unlike TNS and NI, species evenness, determined using Pielou’s index, increased from G - I (J = 0.27) to G - II (J = 0.30) and further to G - III (J = 0.50).
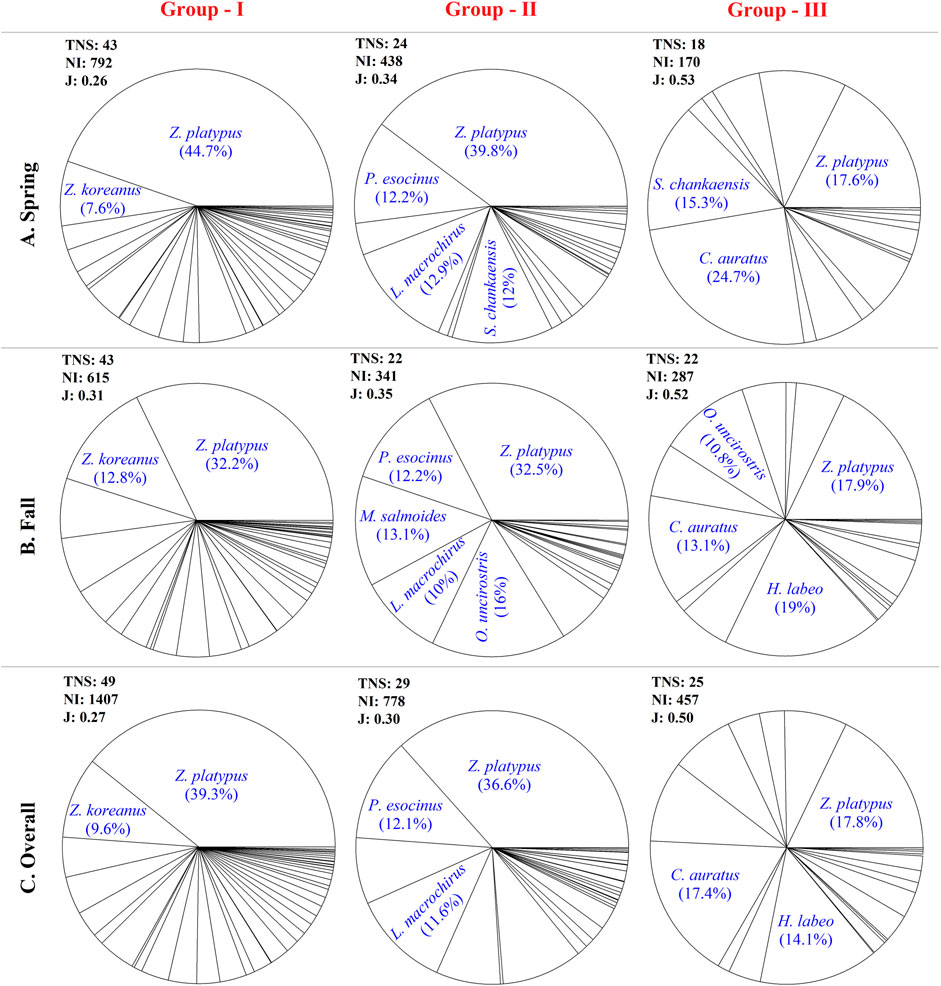
FIGURE 5. Seasonal and overall differences of fish communities among the spatial water quality groups that determined by PCA.
The spatial shifts in the composition of fish community were measureable, whereas seasonal variability was low in all groups (Figure 5). Z. platypus and Z. koreanus dominated the fish community of G - I overall and seasonally. These two dominant species accounted for 48.9% of total relative abundance (TRA), whereas the remaining 47 species contributed 51.1%. By contrast, several tolerant and exotic species (Opsariichthys uncirostris, Lepomis macrochirus and Micropterus salmoides) was highly abundant in the fish community of G - II seasonally, followed by Z. platypus. Overall, 60.3% of TRA was accounted for by the three dominant species (Z. platypus, P. esocinus, and L. macrochirus). In G - III, the RA of species was more even than in other groups, likely related to low species richness (Rich). The dominant species in G - III were Z. platypus (17.8%), Carassius auratus (17.4%), and Hemibarbus labeo (14.1%).
mIBI showed that ecological river health decreased from upstream to downstream sites (Supplementary Table S6). At each site, seasonal variation was relatively low. A similar finding was revealed among the spatial water quality groups (Figure 6). According to total IBI scores, G - I showed good ecological health, ranging from fair to excellent. G - II was considered poor to fair, whereas G - III was very poor to poor. All details of the ecological health evaluation at study sites was shown in Supplementary Table S6.
3.2.2 Functional diversity and trait composition of fishes
Functional changes, measured using functional indices and species trait metrics, were found among the chemical water quality groups. Fish traits used to calculate functional indices exhibited a significant effect (p < 0.001) on at least one axis of a three-dimensional functional space (Table 2; Supplementary Figure S3). The metrics, which are insectivore, sensitive, rheophilic and riffle-benthic, were negatively correlated with the first axis (PC 1). In contrast, the metrics omnivore, tolerant, and stagnophilic were positively correlated with PC 1. Increased trophic level and carnivore metric were negatively correlated with the second axis (PC 2), whereas intermediate tolerance guild were positively correlated. For the third axis (PC 3), significant effects of trophic guild, tolerance guild, and vertical position were found.
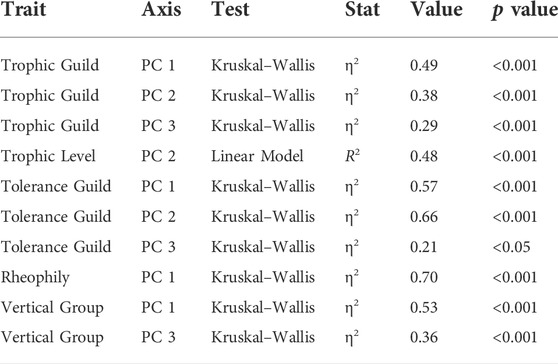
TABLE 2. Traits with significant effect on axes of the best functional space in PCoA (three-dimensional).
Spatial-seasonal variation in the functional metrics was determined based on the abundance of species (Figure 7). The abundance of insectivorous species decreased from G - I to G - II and further to G - III, whereas a similar pattern of increases was observed for carnivorous and omnivorous species. However, the RA of trophic guilds differed seasonally among groups. The RA of sensitive species showed a clear trend, decreasing from G - I to G - II and further to G - III. By contrast, the abundance of tolerant species increased in that order among the groups. Intermediate species showed a lower abundance in G - III than in G - I and G - II (Figure 7). The RA for stagnophilic species increased with group number (G - I to G - III), whereas the RA of rheophilic species decreased. Benthic species were most abundant in G - II, whereas the RA of riffle-benthic species decreased from G - I to G - II and further to G - III.
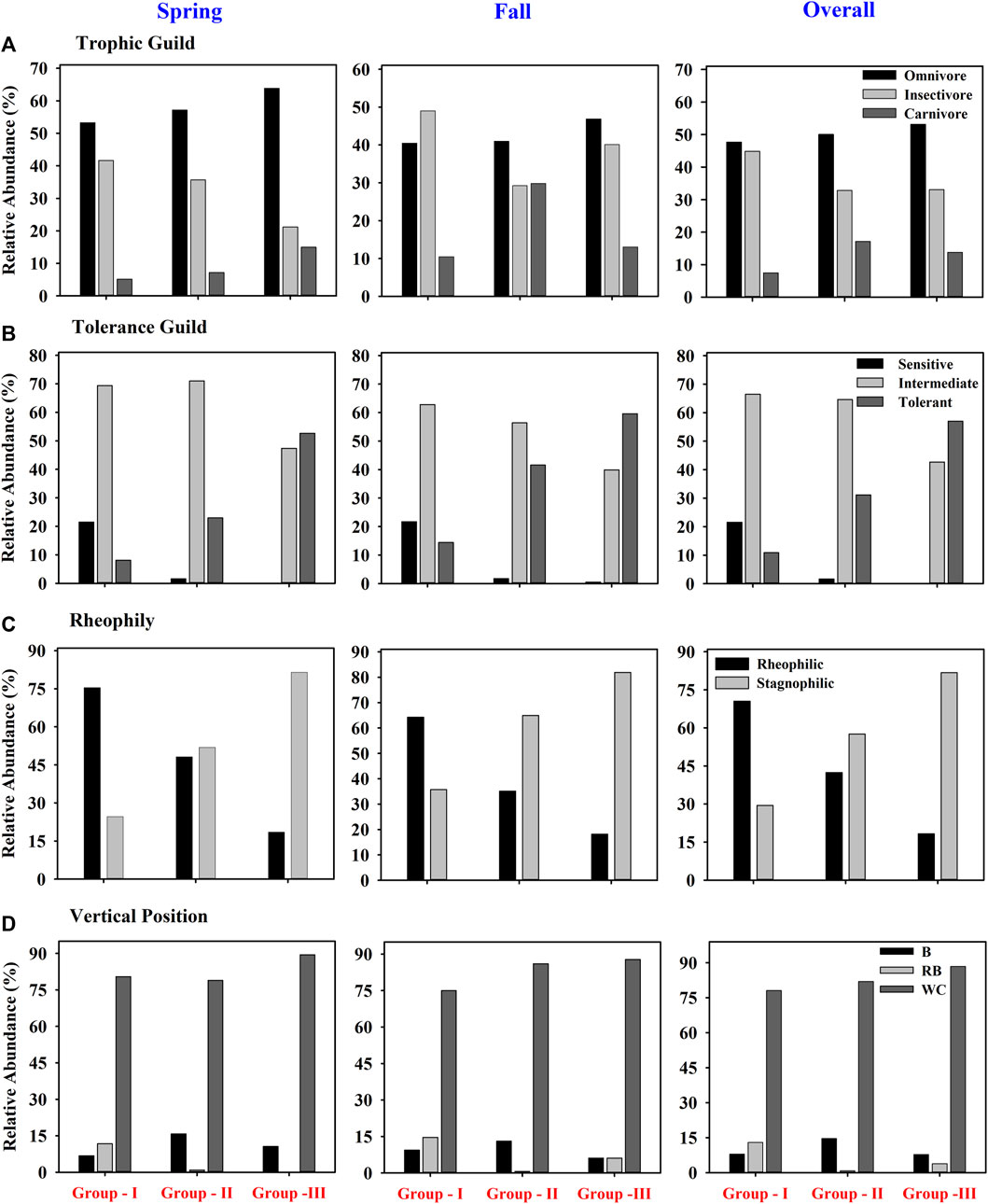
FIGURE 7. Relative abundance of functional metrics of trophic (A) and tolerance guilds (B), rheophily (C) and vertical position preference (D) in three spatial water quality (B-benthic; RB, riffle-benthic; WC, water column species).
Besides, F-Ric decreased from G - I (0.98) to G - II (0.82) and further to G - III (0.34), whereas increases in that order were observed for F-Eve and F-Div (Figure 8). F-Dis showed similar values for G - II and G - III (0.64 and 0.65, respectively) but was lower in G - I (0.57) (Figure 8). Because above functional values were not possible to indicate statistical differences between groups, analysis of variance with Tukey’s test was performed using seasonal calculation of these indices for sites within each group (Table 3). The seasonal values of functional indices at each study site were provided in Supplementary Table S5. The findings indicated significant differences among groups for all indices except F-Eve. F-Ric differed significantly in all pairwise comparisons (p < 0.001). F-Div showed no significant difference between G - I and G - II but was significantly higher in G - III than in the other groups. By contrast, F-Dis and F-Mpd were significantly lower in G - III than in G - I and G - II (Table 3).
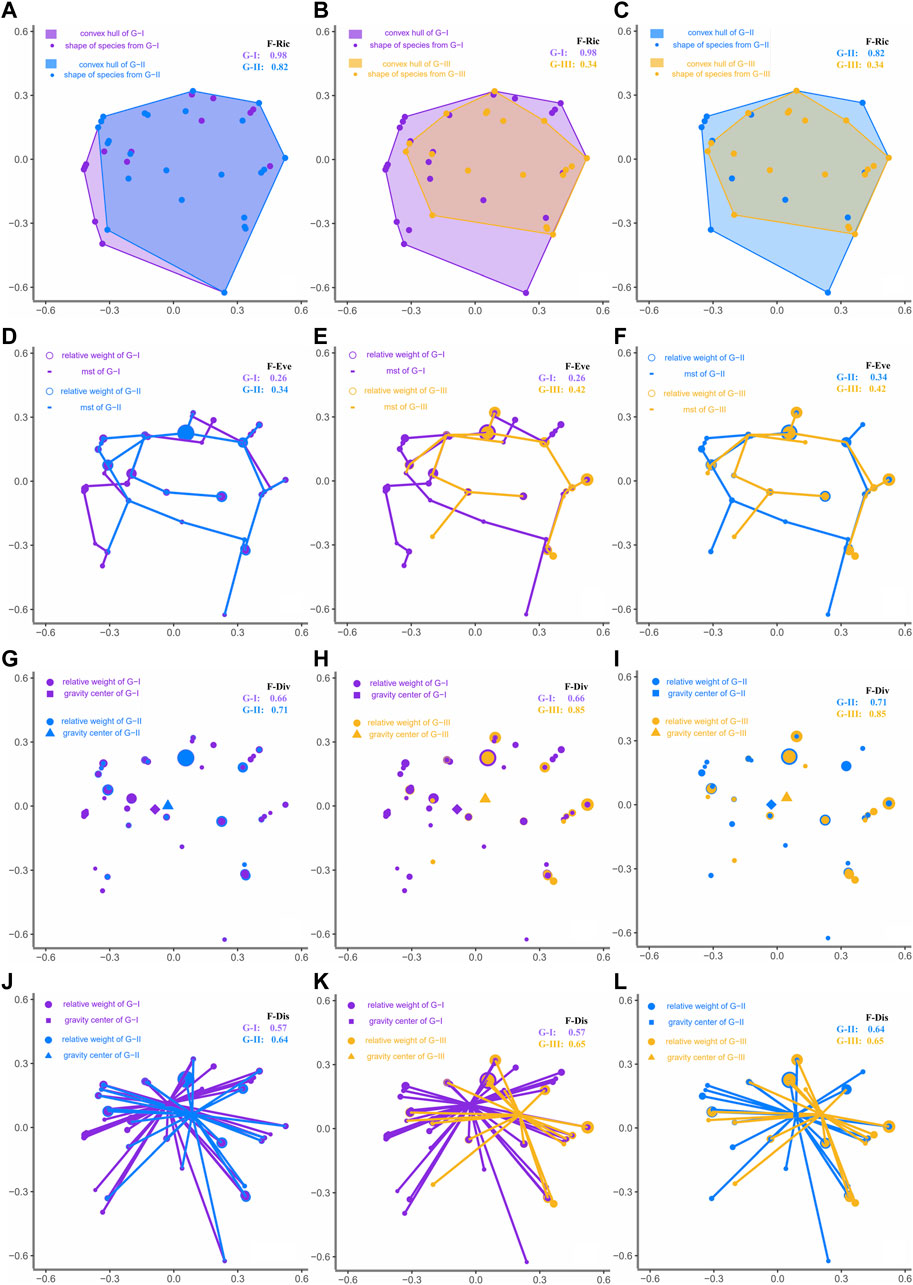
FIGURE 8. Calculation of the functional indices in the multidimensional functional space for fish species of each spatial water quality group (G-I, G-II, and G-III) (A–C) for F-Ric - the volume of a convex hull formed by species positions along trait-based PCoA coordinates, (D–F) for F-Eve - the regularity of species positions and abundance along the minimum spanning tree (mst) in functional space (G–I) for F-Div - the deviation in the proportion of total abundance supported by the species with the most extreme traits, (J,K) for F-Dis - the average abundance-weighted species distance from the centre of gravity in functional space. (L) Functional indices compared between the groups (G-I and G-II, G-I and G-III, G-II and G-III).
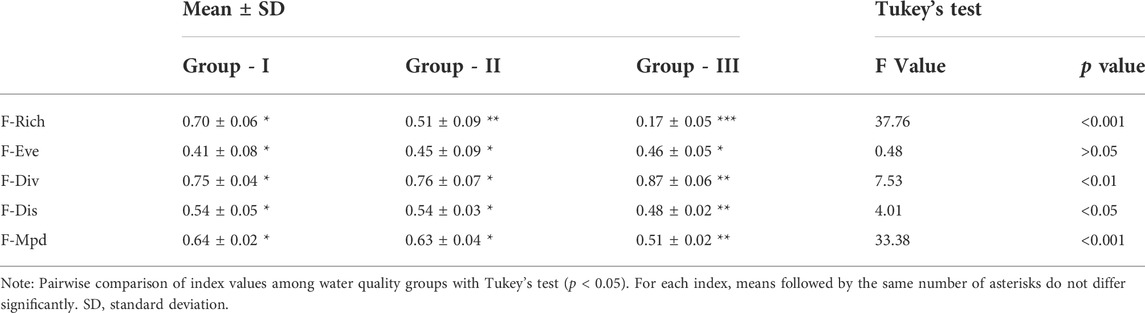
TABLE 3. Mean of functional diversity indices for the water quality groups based on their seasonal calculation at each study site.
3.3 Correlations among functional diversity metrics, river health, and water quality indicators
RDA indicated that changes in fish functional diversity, functional metrics, and mIBI were associated with gradients of water chemical variables and chemical river health. The permutation test (n = 999) revealed that three RDA models were statistically significant (p < 0.05), with the first model for responses of functional indices and mIBI (Figure 9A), the second model for responses of functional traits (Figure 9B), and the third model for responses of the metrics included in mIBI (Figure 9C).
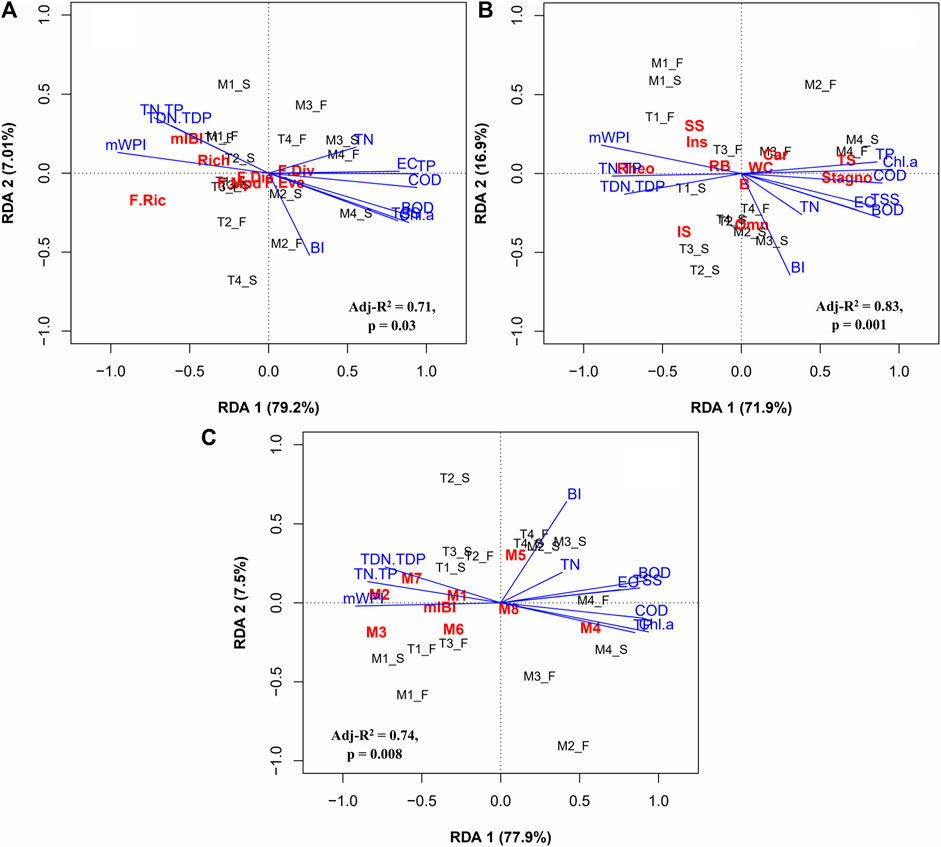
FIGURE 9. Redundancy analysis (RDA) plots on (A) responses of functional diversity indices (B) responses of functional trait metrics, (C) responses of the mIBI metrics to water quality variation and chemical health. The abbreviations of the response and explanatory variables were available in Materials and Methods. The black letters (M1_S, etc.) indicate the study site and season (S-spring and F-fall).
The first axis (RDA 1) in Figure 9A accounted for 79.2% of the total variance in the variables. The second axis (RDA 2) contributed only a small portion of the variance (7.6%), and therefore RDA 1 was the most useful. F-Ric and mIBI were sensitive to chemical variation along RDA 1. The ratios of TN:TP and TDN:TDP, as well as mWPI (or chemical health), were positively correlated with F-Ric and mIBI, whereas variables with positive loadings (EC, TSS, BOD, COD, TP, and Chl-a) were negatively correlated with those parameters (Figure 9A). As shown in Figure 9B, the first axis (RDA 1) explained 71.9% of the total variance, and the second axis (RDA 2) explained 16.9%. The metrics tolerant and stagnophilic were positively correlated with physicochemical variables that had positive loadings along RDA 1 (TSS, EC, BOD, COD, TP, and Chl-a). Sensitive, insectivore, and rheophilic metrics responded negatively to those variables and correlated positively with TN:TP, TDN:TDP, and chemical health. The second axis correlated positively with the metric omnivore and negatively with BI. Regarding Figure 9C, RDA 1 accounted for 78% of the total variance in the variables, whereas RDA 2 made a small contribution to the total variance. Along RDA 1, the metrics of mIBI (M2, M3, and M7) were highly sensitive to variables with positive loadings (TSS, EC, COD, TP, Chl-a, and BI). By contrast, the metrics were positively correlated with TN:TP, TDN:TDP, and chemical health (Figure 9C). Only the metric M4 was positively correlated with TSS, EC, COD, TP, Chl-a, and BI.
Finally, correlation analyses revealed that the relationships among Rich, functional indices (except for F-Eve), and ecological health were statistically significant (p < 0.05; Table 4). Positive correlations were observed between Rich and F-Ric, F-Dis, F-Mpd, mIBI, and mWPI. Rich was negatively correlated with F-Div. In particular, Rich, F-Ric and F-Mpd were strongly correlated with river health, represented by mIBI and mWPI (p < 0.001).
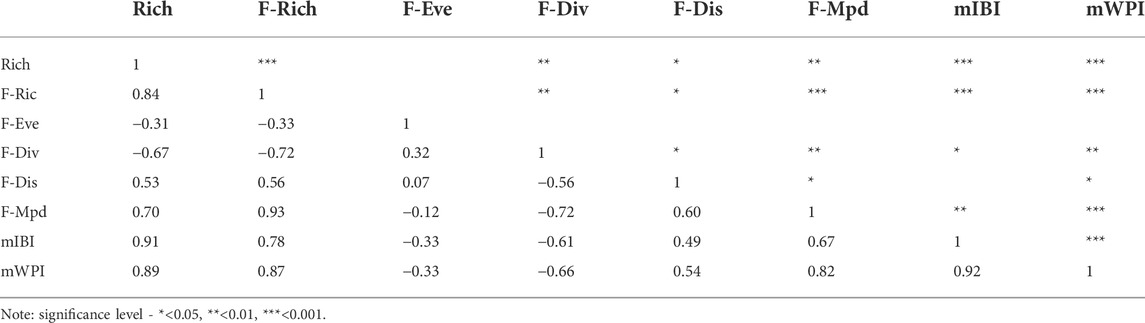
TABLE 4. Correlations among species richness (Rich), functional indices, and river health assessment based mWPI and mIBI.
4 Discussion
The functional diversity of aquatic organisms has recently been recognized as an effective tool for defining ecosystem function and structure and assessing ecological conditions in lotic systems (Kraft et al., 2015; Villéger et al., 2017). Fish play an imperative role in assessments of ecological water quality (Schinegger et al., 2016; Simon and Evans, 2017), and the findings from this study demonstrate the relevance of the functional trait-based diversity of lotic fish to ecological river health. mWPI and mIBI form the basis of the assessment of river health (An et al., 2006; Choi et al., 2011; Kim and An, 2015). Also, we found that water pollution (eutrophication and organic matter) had significant effects on the ecological river health and fish community structures at our sites based on both functional and taxonomic information.
Eutrophication (nutrient enrichment) and organic pollutants contributed to the degradation of water quality from upstream to downstream reaches. Associated increases in suspended solids and ionic contents were revealed through analyses of water chemical quality. Such water quality problems are clearly linked to land-use changes along the river (Supplementary Figure S1). Environmental conditions of each site supported this finding (see section 2.1 of Materials and Methods). The upstream sites (M1, T1, T3) in the study area predominantly receive forest inputs from upstream areas. However, there is much more agricultural (cropland and livestock farming) and urban-industrial activity at the midstream and downstream sites. Accordingly, multiple sources of pollution have led to large increases in nutrients, organic matter, and primary productivity, along with low ratios of TN:TP and TDN:TDP. Chemical river health, estimated as mWPI, was highly sensitive to water quality changes that likely associated with intensifying land-use. Similar impacts on chemical health conditions in lotic systems have been identified in previous studies (Mamun and An, 2018; Hara et al., 2019; Atique et al., 2020). The primary problems associated with eutrophication in lotic systems include reduced ecological integrity, harmful algal blooms, hypoxic conditions, and an inability to use the stream for human purposes (Chalar et al., 2013; McDowell et al., 2020; Stets et al., 2020).
The PCA on water quality variables and chemical health identified three water quality groups that were spatially significant for the structure of fish communities. G - I, which was strongly correlated with TN:TP, TDN:TDP, and mWPI, was used as our reference group for water quality. Conversely, G - III was strongly associated with TSS, EC, nutrients, organic matter, and primary productivity. These associations indicate that chemical condition of this group was observably depreciated because of river pollution. In addition, G - II exhibited moderate chemical water quality, falling between the other two groups.
Large differences in fish diversity, abundance, and composition were found among the three spatial groups. Rich and the abundance of fish generally increase from upstream to downstream reaches because of habitat diversification (Matthews, 1998; Muneepeerakul et al., 2008). However, the patterns observed in this study differed, with a clear decrease in fish abundance and richness from G - I to G - II followed by a further decrease to G - III. Furthermore, fish composition varied by group in terms of the dominant species. The dominance of sensitive species, such as Zacco koreanus and Zacco temminskii, was high in G - I. However, several tolerant (Opsariichthys uncistris, Carassius auratus, and Hemibarbus labeo) exotic species (Micropterus salmoides and L. macrochirus) contributed significantly to the species composition of G - II and G - III. These findings suggest that water quality degradation caused by agricultural and urban-industrial pollutants can significantly affect local fish communities (Chalar et al., 2013; Gao et al., 2015; Whitney et al., 2019) and support the proliferation of invasive exotic species (Kennard et al., 2005; Hermoso et al., 2011; Kim et al., 2021). Besides, mIBI showed that ecological river health decreased from upstream to downstream reaches. Evidence was found of differences in ecological health among the spatial water quality groups (G - I: good, G - II: poor to fair, G - III: poor), which demonstrates the ecological consequences of water physicochemical change (chemical health degradation) due to pollution sources along the length of a river.
The spatial water quality differences played a key role in functional changes of the river fish community measured by functional diversity indices. F-Ric was sensitive to spatial differences of chemical variables among the groups. F-Ric increased significantly from G - III to G - II and further to G - I. F-Dis and F-Mpd were significantly lower in G - III, but F-Div was higher, relative to other groups. The decreases in functional indices in G - III were closely related with the loss of species in the functional spaces, particularly species with a more specific diet (insectivores) and those sensitive to water pollution. The findings indicated several loss of specialist species and their abundance distribution can lead to low niche occupation in functional space as water chemical health decreases. The most abundant fishes in G–I and G–II were near to the center of gravity in functional spaces despite high F - Rich, By contrast, functionally distinct species in G–III were highly abundant and more evenly distributed, supporting higher F–Div in G–III that the two other groups (Villéger et al., 2008). Most abundant species in G–III were characterized by the combination of traits which were tolerant to water pollution, stagnophilic, and omnivores. High functional diversity can indicate better ecosystem functioning due to more efficient resource allocation (Mason et al., 2005; Mouillot et al., 2013). As shown by F - Ric, F - Dis, and F - Mpd, the resource availability among species was apparently linked to water chemical health (or physicochemical quality), which differed among the groups. Thus, if a local environment has suitable chemical health, lotic fish can utilize its resources effectively.
Differences in functional indices were directly correlated with the positions of fish species in local functional space formed based on functional trait metrics and abundance. The trophic and tolerance guilds showed large variation among the water quality groups. Insectivorous and sensitive species decreased from G - I to G - II and further to G - III, whereas omnivorous and tolerant species increased. Several studies have suggested that such functional variation in fish is strongly related to water quality degradation driven by agricultural and urban-industrial pollutants in lotic systems (Gao et al., 2015; Schinegger et al., 2016; Mamun and An, 2020). Also, clear longitudinal variation was revealed for the abundance of both rheophilic and stagnophilic species from upstream to downstream sites. The abundance of rheophilic species decreased in the downstream direction, whereas the abundance of stagnophilic species increased. The decreases of F-Ric, F-Mpd, and F-Dis from G - I to G - II and further to G - III were highly sensitive to the loss of species with insectivore, sensitive, and rheophilic characteristics. Also, these functional indices were significantly correlated with the river health as determined with mIBI and mWPI. These results suggest that water quality degradation along longitudinal river zones can act as an environmental filter on fish functional traits (Aarts and Nienhuis, 2003; Cornwell et al., 2006; Pease et al., 2012; Keck et al., 2014) and thus is connected to the structuring of local fish communities in the river.
Moreover, RDA indicated significant responses of the functional and taxonomical structures of river fish to water quality variables and chemical river health. The analyses were based on spatial-seasonal average values for chemical variables and fish information (functional indices, functional trait metrics, mIBI and its metrics; n = 16). Rich, F-Ric, and mIBI were sensitive to increases in chemical indicators, including TSS, EC, organic matter, nutrients, and Chl-a. Chemical health (measured as mWPI) was positively correlated with the ratios of TN:TP and TDN:TDP. Models based on both functional traits and metrics of mIBI showed good performance based on variation in water chemical variables and chemical health. The metrics, which are rheophilic/stagnophilic and tolerant/sensitive, were significantly explained by the chemical indicators. The metrics rheophilic, sensitive, and insectivore responded negatively to TSS, EC, organic matter, nutrients, and Chl-a, whereas stagnophilic and tolerant metrics showed positive responses. The fish metrics of mIBI were highly responsive to variation in water quality variables and chemical health, which suggests that mIBI is applicable to ecological river assessment, reflecting water pollution in lotic systems (Choi et al., 2011; Ruaro et al., 2020; Karr et al., 2022). A significant and positive correlation was found between F-Ric, F-Dis, and F-Mpd and mIBI and mWPI, indicating similar responses to water quality degradation. However, correlation coefficients were generally not very high (0.4–0.7) between F-Dis, F-Mpd, and mIBI, suggesting low redundancy. Accordingly, mIBI and the functional indices, except for F-Ric, were complementary to evaluate river health because they may respond differently to anthropogenic disturbances and natural factors that affect water quality. Additionally, although mIBI had good performance, the inclusion of these functional indices as a metric may further enhance mIBI’s performance on the river health diagnosis.
Overall, the functional trait-based diversity of lotic fish is significant to the assessment of ecological river health and reflects water chemical quality. This association arises because niche occupation in functional space by all species, along with their abundance distribution, is highly responsive to the loss of species with unsuitable traits due to water pollution. Our findings supported that functional diversity metrics of aquatic communities is feasibility responsive to disturbances associated with river health degradation, such as fragmentation and flow regime change (Kelley et al., 2018; Oliveira et al., 2018), and land-use intensification (Pool et al., 2010; Larentis et al., 2021). It is pertinent to note that functional indices (F-Dis and F-Mpd) and mIBI are complementary and effective measures for assessing chemical water quality and river health. Combining these diagnostic methods for river health can therefore be useful for aquatic conservation and management. Also, managing water quality effectively requires collaborating with anthropogenic pollutant sources to preserve and maintain the functional diversity of lotic fish communities and ecological health.
Data availability statement
The raw data supporting the conclusions of this article will be made available by the authors, without undue reservation.
Author contributions
NJ: Conceptualization; formal analysis; data curation; methodology; software; visualization; writing—original draft, review and editing. MM: Formal analysis; Software; visualization. C-YC: Formal analysis; data curation. K-GA: Visualization, validation; supervision; project administration; funding acquisition.
Funding
This work was supported by “Korea Environment Industry & Technology Institute (KEITI)” through the “Exotic Invasive Fish Species Management Project”, funded by the Ministry of Environment, Korea (2018002270003, RE201807019).
Acknowledgments
This paper acknowledges the Korea Environment Industry and Technology Institute (KEITI), and also acknowledges Daejeon Green Environmental Centre (Yr. 2016) for the data support.
Conflict of interest
The authors declare that the research was conducted in the absence of any commercial or financial relationships that could be construed as a potential conflict of interest.
Publisher’s note
All claims expressed in this article are solely those of the authors and do not necessarily represent those of their affiliated organizations, or those of the publisher, the editors and the reviewers. Any product that may be evaluated in this article, or claim that may be made by its manufacturer, is not guaranteed or endorsed by the publisher.
Supplementary material
The Supplementary Material for this article can be found online at: https://www.frontiersin.org/articles/10.3389/fenvs.2022.1012420/full#supplementary-material
References
Aarts, B. G., and Nienhuis, P. H. (2003). Fish zonations and guilds as the basis for assessment of ecological [2pt] integrity of large rivers. Hydrobiologia 500 (1), 157–178. doi:10.1023/A:1024638726162
An, K. G., Lee, J. Y., Bae, D. Y., Kim, J. H., Hwang, S. J., Won, D. H., et al. (2006). Ecological assessments of aquatic environment using multi-metric model in major nationwide stream watersheds. J. Korean Soc. Water Environ. 22 (5), 796–804.
APHA (2005). Standard methods for the examination of water and wastewater. 21st ed. New York, NY, USA: American Public Health Association.
Atique, U., Kwon, S., and An, K. G. (2020). Linking weir imprints with riverine water chemistry, microhabitat alterations, fish assemblages, chlorophyll-nutrient dynamics, and ecological health assessments. Ecol. Indic. 117, 106652. doi:10.1016/j.ecolind.2020.106652
Atique, U., Lim, B., Yoon, J., and An, K. G . (2019). Biological health assessments of lotic waters by biotic integrity indices and their relations to water chemistry. Water 11 (3), 436. doi:10.3390/w11030436
Barbour, M. T. (1999). Rapid bioassessment protocols for use in wadeable streams and rivers: Periphyton, benthic macroinvertebrates and fish. Washington, DC: US Environmental Protection Agency (US EPA), Office of Water.
Belmar, O., Bruno, D., Guareschi, S., Mellado-Díaz, A., Millán, A., and Velasco, J. (2019). Functional responses of aquatic macroinvertebrates to flow regulation are shaped by natural flow intermittence in Mediterranean streams. Freshw. Biol. 64 (5), 1064–1077. doi:10.1111/fwb.13289
Bichoff, A., Osorio, N. C., Ruwer, D. T., Dunck, B., and Rodrigues, L. (2018). Trait structure and functional diversity of periphytic algae in a floodplain conservation area. Braz. J. Bot. 41 (3), 601–610. doi:10.1007/s40415–018–0467–7
Boucek, R. E., and Rehage, J. S. (2014). Climate extremes drive changes in functional community structure. Glob. Chang. Biol. 20 (6), 1821–1831. doi:10.1111/gcb.12574
Brind’Amour, A., Boisclair, D., Dray, S., and Legendre, P. (2011). Relationships between species feeding traits and environmental conditions in fish communities: A three-matrix approach. Ecol. Appl. 21 (2), 363–377. doi:10.1890/09–2178.1
Buisson, L., Grenouillet, G., Villéger, S., Canal, J., and Laffaille, P. (2013). Toward a loss of functional diversity in stream fish assemblages under climate change. Glob. Chang. Biol. 19 (2), 387–400. doi:10.1111/gcb.12056
Chalar, G., Delbene, L., González-Bergonzoni, I., and Arocena, R. (2013). Fish assemblage changes along a trophic gradient induced by agricultural activities (Santa Lucía, Uruguay). Ecol. Indic. 24, 582–588. doi:10.1016/j.ecolind.2012.08.010
Choi, J. W., Kumar, H. K., Han, J. H., and An, K. G. (2011). The development of a regional multimetric fish model based on biological integrity in lotic ecosystems and some factors influencing the stream health. Water Air Soil Pollut. 217 (1), 3–24. doi:10.1007/s11270–010–0563–1
Cornwell, W. K., Schwilk, D. W., and Ackerly, D. D. (2006). A trait-based test for habitat filtering: Convex hull volume. Ecology 87 (6), 1465–1471. doi:10.1890/0012–9658(2006)87[1465:ATTFHF]2.0.CO;2
DEHP (2009). Queensland water quality guidelines, version 3. Queensland Government, Brisbane: Department of Environment and heritage Protection. ISBN 978-o-9806986–0–2. Available from: https://www.des.qld.gov.au/water/pdf/water-quality-guidelines.pdf.
Dodds, W. K., Jones, J. R., and Welch, E. B. (1998). Suggested classification of stream trophic state: Distributions of temperate stream types by chlorophyll, total nitrogen, and phosphorus. Water Res. 32 (5), 1455–1462. doi:10.1016/S0043–1354(97)00370–9
Du, X., Song, D., Ming, K., Yang, J., Jin, X., Wang, H., et al. (2022). Functional responses of phytoplankton assemblages to watershed land use and environmental gradients. Front. Ecol. Evol. 9, 819252. doi:10.3389/fevo.2021.819252
Dudgeon, D., Arthington, A. H., Gessner, M. O., Kawabata, Z. I., Knowler, D. J., Lévêque, C., et al. (2006). Freshwater biodiversity: Importance, threats, status and conservation challenges. Biol. Rev. 81 (2), 163–182. doi:10.1017/S1464793105006950
Frimpong, E. A., and Angermeier, P. L. (2010). “Trait-based approaches in the analysis of stream fish communities,” in Community ecology of stream fishes: Concepts, approaches, and techniques (Bethesda, Maryland, United States: American Fisheries Society), 73, 109–136. Symposium.
Galego de Oliveira, A., Bailly, D., Cassemiro, F. A., Couto, E. V. D., Bond, N., Gilligan, D., et al. (2019). Coupling environment and physiology to predict effects of climate change on the taxonomic and functional diversity of fish assemblages in the Murray-Darling Basin, Australia. PLoS One 14 (11), e0225128. doi:10.1371/journal.pone.0225128
Gao, X., Zhang, Y., Ding, S., Zhao, R., and Meng, W. (2015). Response of fish communities to environmental changes in an agriculturally dominated watershed (Liao River Basin) in northeastern China. Ecol. Eng. 76, 130–141. doi:10.1016/j.ecoleng.2014.04.019
Gower, J. C. (1971). A general coefficient of similarity and some of its properties. Biometrics 27, 857–871. doi:10.2307/2528823
HaRa, J., Mamun, M., and An, K. G. (2019). Ecological river health assessments using chemical parameter model and the index of biological integrity model. Water 11 (8), 1729. doi:10.3390/w11081729
Hering, D., Feld, C. K., Moog, O., and Ofenböck, T. (2006). “Cook book for the development of a Multimetric Index for biological condition of aquatic ecosystems: Experiences from the European AQEM and STAR projects and related initiatives,” in The ecological status of European rivers: Evaluation and intercalibration of assessment methods (Dordrecht: Springer), 311–324. doi:10.1007/978–1–4020–5493–8_22
Hermoso, V., Clavero, M., Blanco-Garrido, F., and Prenda, J. (2011). Invasive species and habitat degradation in Iberian streams: An analysis of their role in freshwater fish diversity loss. Ecol. Appl. 21 (1), 175–188. doi:10.1890/09–2011.1
Karr, J. R. (1981). Assessment of biotic integrity using fish communities. Fisheries 6 (6), 21–27. doi:10.1577/1548-8446(1981)006<0021:aobiuf>2.0.co;2
Karr, J. R., Larson, E. R., and Chu, E. W. (2022). Ecological integrity is both real and valuable. Conserv. Sci. Pract. 4 (2), e583. doi:10.1111/csp2.583
Keck, B. P., Marion, Z. H., Martin, D. J., Kaufman, J. C., Harden, C. P., Schwartz, J. S., et al. (2014). Fish functional traits correlated with environmental variables in a temperate biodiversity hotspot. PLoS One 9 (3), e93237. doi:10.1371/journal.pone.0093237
Kelley, J. L., Grierson, P. F., Collin, S. P., and Davies, P. M. (2018). Habitat disruption and the identification and management of functional trait changes. Fish. Fish. (Oxf). 19 (4), 716–728. doi:10.1111/faf.12284
Kennard, M. J., Arthington, A. H., Pusey, B. J., and Harch, B. D. (2005). Are alien fish a reliable indicator of river health? Freshw. Biol. 50 (1), 174–193. doi:10.1111/j.1365–2427.2004.01293.x
Kim, I. S., and Park, J. Y. (2002). Freshwater fish of Korea. Seoul, Korea: Kyohak Publishing. (in Korean).
Kim, J. Y., and An, K. G. (2015). Integrated ecological river health assessments, based on water chemistry, physical habitat quality and biological integrity. Water 7 (11), 6378–6403. doi:10.3390/w7116378
Kim, J. Y., Atique, U., and An, K. G. (2021). Relative abundance and invasion dynamics of alien fish species linked to chemical conditions, ecosystem health, native fish assemblage, and stream order. Water 13 (2), 158. doi:10.3390/w13020158
Klemm, D. J. (1993). “Fish field and laboratory methods for evaluating the biological integrity of surface waters,” in Environmental monitoring systems laboratory, office of modeling, monitoring systems, and quality assurance, office of research and development, US environmental protection agency (US EPA). Washington, DC: Environmental Monitoring Systems Laboratory, Office of Modeling, Monitoring Systems, Office of Research and Development, US Environmental Proctection Agency.
Kraft, N. J., Adler, P. B., Godoy, O., James, E. C., Fuller, S., and Levine, J. M. (2015). Community assembly, coexistence and the environmental filtering metaphor. Funct. Ecol. 29 (5), 592–599. doi:10.1111/1365–2435.12345
Kruk, A. (2007). Role of habitat degradation in determining fish distribution and abundance along the lowland Warta River, Poland. J. Appl. Ichthyol. 23 (1), 9–18. doi:10.1111/j.1439–0426.2006.00784.x
Kuczyńska-Kippen, N., Špoljar, M., Zhang, C., and Pronin, M. (2020). Zooplankton functional traits as a tool to assess latitudinal variation in the northern-southern temperate European regions during spring and autumn seasons. Ecol. Indic. 117, 106629. doi:10.1016/j.ecolind.2020.106629
Laliberté, E., and Legendre, P. (2010). A distance-based framework for measuring functional diversity from multiple traits. Ecology 91 (1), 299–305. doi:10.1890/08–2244.1
Lam-Gordillo, O., Baring, R., and Dittmann, S. (2020). Establishing the South Australian macrobenthic traits (SAMT) database: A trait classification for functional assessments. Ecol. Evol. 10 (24), 14372–14387. doi:10.1002/ece3.7040
Larentis, C., Pavanelli, C. S., and Delariva, R. L. (2021). Do environmental conditions modulated by land use drive fish functional diversity in streams? Hydrobiologia, 1–19. doi:10.1007/s10750–021–04756-x
MacArthur, R., and Levins, R. (1967). The limiting similarity, convergence, and divergence of coexisting species. Am. Nat. 101 (921), 377–385. https://www.jstor.org/stable/2459090. doi:10.1086/282505
Magneville, C., Loiseau, N., Albouy, C., Casajus, N., Claverie, T., Escalas, A., et al. (2022). mFD: An R package to compute and illustrate the multiple facets of functional diversity. Ecography 2022 (1), e05904. doi:10.1111/ecog.05904
Mamun, M., and An, K. G. (2018). Ecological health assessments of 72 streams and rivers in relation to water chemistry and land-use patterns in South Korea. Turkish J. Fish. Aquatic Sci. 18 (7), 871–880. doi:10.4194/1303-2712-v18_7_05
Mamun, M., and An, K. G. (2020). Stream health assessment using chemical and biological multi-metric models and their relationships with fish trophic and tolerance indicators. Ecol. Indic. 111, 106055. doi:10.1016/j.ecolind.2019.106055
Martín, I., Betancourt, J., Salas, J. J., Peñate, B., Pidre, J. R., and Sandron, N. (2006). Guía sobre tratamientos de aguas residuales urbanas para pequeños núcleos de población: Mejora de la calidad de los efluentes. 1st ed. Las Palmas, Spain: Daute Diseño, S.L.
Mason, N. W., Mouillot, D., Lee, W. G., and Wilson, J. B. (2005). Functional richness, functional evenness and functional divergence: The primary components of functional diversity. Oikos 111 (1), 112–118. doi:10.1111/j.0030-1299.2005.13886.x
Mason, N. W., Lanoiselée, C., Mouillot, D., Irz, P., and Argillier, C. (2007). Functional characters combined with null models reveal inconsistency in mechanisms of species turnover in lacustrine fish communities. Oecologia 153 (2), 441–452. doi:10.1007/s00442–007–0727-x
Matsuzaki, S. I. S., Sasaki, T., and Akasaka, M. (2016). Invasion of exotic piscivores causes losses of functional diversity and functionally unique species in Japanese lakes. Freshw. Biol. 61 (7), 1128–1142. doi:10.1111/fwb.12774
Matthews, W. J. (1998). Patterns in freshwater fish ecology. New York: Springer Science & Business Media.
McDowell, R. W., Noble, A., Pletnyakov, P., Haggard, B. E., and Mosley, L. M. (2020). Global mapping of freshwater nutrient enrichment and periphyton growth potential. Sci. Rep. 10 (1), 3568. doi:10.1038/s41598–020–60279-w
McGill, B. J., Enquist, B. J., Weiher, E., and Westoby, M. (2006). Rebuilding community ecology from functional traits. Trends Ecol. Evol. 21 (4), 178–185. doi:10.1016/j.tree.2006.02.002
Milardi, M., Gavioli, A., Soininen, J., and Castaldelli, G. (2019). Exotic species invasions undermine regional functional diversity of freshwater fish. Sci. Rep. 9 (1), 17921. doi:10.1038/s41598–019–54210–1
MOE/NIER (2008). Survey and evaluation of aquatic ecosystem health in Korea. Inchon, Korea: MOE/NIER (Ministry of Environment/National Institute of Environmental Research). (in Korean with English summary).
Mouchet, M. A., Villéger, S., Mason, N. W., and Mouillot, D. (2010). Functional diversity measures: An overview of their redundancy and their ability to discriminate community assembly rules. Funct. Ecol. 24 (4), 867–876. doi:10.1111/j.1365–2435.2010.01695.x
Mouillot, D., Graham, N. A., Villéger, S., Mason, N. W., and Bellwood, D. R. (2013). A functional approach reveals community responses to disturbances. Trends Ecol. Evol. 28 (3), 167–177. doi:10.1016/j.tree.2012.10.004
Muneepeerakul, R., Bertuzzo, E., Lynch, H. J., Fagan, W. F., Rinaldo, A., and Rodriguez-Iturbe, I. (2008). Neutral metacommunity models predict fish diversity patterns in Mississippi–Missouri basin. Nature 453 (7192), 220–222. doi:10.1038/nature06813
Oliveira, A. G., Baumgartner, M. T., Gomes, L. C., Dias, R. M., and Agostinho, A. A. (2018). Long-term effects of flow regulation by dams simplify fish functional diversity. Freshw. Biol. 63 (3), 293–305. doi:10.1111/fwb.13064
Pálffy, K., Présing, M., and Vörös, L. (2013). Diversity patterns of trait-based phytoplankton functional groups in two basins of a large, shallow lake (Lake Balaton, Hungary) with different trophic state. Aquat. Ecol. 47 (2), 195–210. doi:10.1007/s10452–013–9434–3
Pallottini, M., Cappelletti, D., Fabrizi, A., Gaino, E., Goretti, E., Selvaggi, R., et al. (2017). Macroinvertebrate functional trait responses to chemical pollution in agricultural–industrial landscapes. River Res. Appl. 33 (4), 505–513. doi:10.1002/rra.3101
Paz, L. E., Rodriguez, M., Gullo, B., and Capítulo, A. R. (2022). Impacts of urban and industrial pollution on functional traits of benthic macroinvertebrates: Are some traits advantageous for survival? Sci. Total Environ. 807, 150650. doi:10.1016/j.scitotenv.2021.150650
Pease, A. A., González-Díaz, A. A., Rodiles-Hernández, R., and Winemiller, K. O. (2012). Functional diversity and trait–environment relationships of stream fish assemblages in a large tropical catchment. Freshw. Biol. 57 (5), 1060–1075. doi:10.1111/j.1365–2427.2012.02768.x
Péru, N., and Dolédec, S. (2010). From compositional to functional biodiversity metrics in bioassessment: A case study using stream macroinvertebrate communities. Ecol. Indic. 10 (5), 1025–1036. doi:10.1016/j.ecolind.2010.02.011
Pool, T. K., Olden, J. D., Whittier, J. B., and Paukert, C. P. (2010). Environmental drivers of fish functional diversity and composition in the Lower Colorado River Basin. Can. J. Fish. Aquat. Sci. 67 (11), 1791–1807. doi:10.1139/F10–095
Ruaro, R., Gubiani, E. A., Hughes, R. M., and Mormul, R. P. (2020). Global trends and challenges in multimetric indices of biological condition. Ecol. Indic. 110, 105862. doi:10.1016/j.ecolind.2019.105862
Sabater, S., Bregoli, F., Acuña, V., Barceló, D., Elosegi, A., Ginebreda, A., et al. (2018). Effects of human-driven water stress on river ecosystems: A meta-analysis. Sci. Rep. 8 (1), 11462. doi:10.1038/s41598–018–29807–7
Schinegger, R., Palt, M., Segurado, P., and Schmutz, S. (2016). Untangling the effects of multiple human stressors and their impacts on fish assemblages in European running waters. Sci. Total Environ. 573, 1079–1088. doi:10.1016/j.scitotenv.2016.08.143
Shen, J., Qin, G., Gu, X., Liu, Y., An, S., Liu, R., et al. (2022). Effects of seasonal hydrological regulation of cascade dams on the functional diversity of zooplankton: Implications for the management of massive reservoirs and dams. J. Hydrology 610, 127825. doi:10.1016/j.jhydrol.2022.127825
Simon, T. P., and Evans, N. T. (2017). “Environmental quality assessment using stream fishes,” in Methods in stream ecology: Vol. 1 ecosystem structure. Editors F. R. Hauer., and G. Lamberti (Cambridge, MA: Academic Press), 319–334.
Sinclair, J. S., Fraker, M. E., Hood, J. M., Frank, K. T., DuFour, M. R., Gorman, A. M., et al. (2021). Functional traits reveal the dominant drivers of long-term community change across a North American Great Lake. Glob. Chang. Biol. 27 (23), 6232–6251. doi:10.1111/gcb.15902
Stets, E. G., Sprague, L. A., Oelsner, G. P., Johnson, H. M., Murphy, J. C., Ryberg, K., et al. (2020). Landscape drivers of dynamic change in water quality of US rivers. Environ. Sci. Technol. 54 (7), 4336–4343. doi:10.1021/acs.est.9b05344
Strahler, A. N. (1957). Quantitative analysis of watershed geomorphology. Trans. AGU. 38 (6), 913–920. doi:10.1029/TR038i006p00913
Strayer, D. L., and Dudgeon, D. (2010). Freshwater biodiversity conservation: Recent progress and future challenges. J. North Am. Benthol. Soc. 29 (1), 344–358. doi:10.1899/08–171.1
Toussaint, A., Charpin, N., Beauchard, O., Grenouillet, G., Oberdorff, T., Tedesco, P. A., et al. (2018). Non-native species led to marked shifts in functional diversity of the world freshwater fish faunas. Ecol. Lett. 21 (11), 1649–1659. doi:10.1111/ele.13141
Toussaint, A., Charpin, N., Brosse, S., and Villéger, S. (2016). Global functional diversity of freshwater fish is concentrated in the Neotropics while functional vulnerability is widespread. Sci. Rep. 6 (1), 22125–22129. doi:10.1038/srep22125
Vannote, R. L., Minshall, G. W., Cummins, K. W., Sedell, J. R., and Cushing, C. E. (1980). The river continuum concept. Can. J. Fish. Aquat. Sci. 37 (1), 130–137. doi:10.1139/f80–017
Villéger, S., Brosse, S., Mouchet, M., Mouillot, D., and Vanni, M. J. (2017). Functional ecology of fish: Current approaches and future challenges. Aquat. Sci. 79 (4), 783–801. doi:10.1007/s00027–017–0546-z
Villéger, S., Mason, N. W., and Mouillot, D. (2008). New multidimensional functional diversity indices for a multifaceted framework in functional ecology. Ecology 89 (8), 2290–2301. doi:10.1890/07–1206.1
Wang, Y., Wu, N., Tang, T., Wang, Y., and Cai, Q. (2022). Small run-of-river hydropower dams and associated water regulation filter benthic diatom traits and affect functional diversity. Sci. Total Environ. 813, 152566. doi:10.1016/j.scitotenv.2021.152566
Weiher, E., Clarke, G. P., and Keddy, P. A. (1998). Community assembly rules, morphological dispersion, and the coexistence of plant species. Oikos 81 (2), 309–322. doi:10.2307/3547051
Whitney, J. E., Holloway, J. A., Scholes, D. T., and King, A. D. (2019). Long-term change of fish communities in a polluted watershed: Does cleaner water “act” on fishes? Trans. Am. Fish. Soc. 148 (1), 191–206. doi:10.1002/tafs.10130
Wu, N., Thodsen, H., Andersen, H. E., Tornbjerg, H., Baattrup-Pedersen, A., and Riis, T. (2019). Flow regimes filter species traits of benthic diatom communities and modify the functional features of lowland streams-a nationwide scale study. Sci. Total Environ. 651, 357–366. doi:10.1016/j.scitotenv.2018.09.210
Keywords: river, ecological integrity, eutrophication, fish, species traits, functional diversity, mIBI, water chemistry
Citation: Jargal N, Mamun M, Choi C-Y and An K-G (2022) Combining functional diversity of lotic fish communities with river health assessment based on multi-metric chemical pollution and biological integrity index models. Front. Environ. Sci. 10:1012420. doi: 10.3389/fenvs.2022.1012420
Received: 05 August 2022; Accepted: 11 October 2022;
Published: 20 October 2022.
Edited by:
Teresa Ferreira, University of Lisbon, PortugalReviewed by:
Rui Manuel Vitor Cortes, University of Trás-os-Montes and Alto Douro, PortugalCarla Ferreira Rezende, Federal University of Ceara, Brazil
Copyright © 2022 Jargal, Mamun, Choi and An. This is an open-access article distributed under the terms of the Creative Commons Attribution License (CC BY). The use, distribution or reproduction in other forums is permitted, provided the original author(s) and the copyright owner(s) are credited and that the original publication in this journal is cited, in accordance with accepted academic practice. No use, distribution or reproduction is permitted which does not comply with these terms.
*Correspondence: Kwang-Guk An, a2dhbkBjbnUuYWMua3I=