- 1Laboratory of Molecular Biology, Institute of crop and Ecology, Hangzhou Academy of Agricultural Sciences, Hangzhou, China
- 2Laboratory of Plant Molecular Biology and Proteomics, Institute of biotechnology; Hangzhou Academy of Agricultural Sciences, Hangzhou, China
- 3Institute of Vegetable, Hangzhou Academy of Agricultural Sciences, Hangzhou, China
Heavy metal-containing atmospheric particulate matter (PM) and acid rain (AR) trigger molecular alteration in plants, perturbing metabolites and damaging plant growth. However, the molecular mechanisms of plants under AR along with Cd-containing atmospheric fine particulate matter (PM2.5-Cd) stress remain unknown. In this study, integrated transcriptomics and metabolomics analyses of pak choi (Brassica pekinensis (Lour.) Rupr) exposed to AR (pH3.5) and PM2.5-Cd (500 μg·m−3) stress were performed. Metabolomics analyses revealed that AR-Cd stress mainly affected 42 metabolic pathways, including 451 differentially expressed metabolites (DEMs). RNA-seq identified 735 common differentially expressed genes (DEGs: 557 upregulated and 178 downregulated). Further analysis found several important DEGs (transcription factors, metabolic pathways genes, and signal transduction genes), including WRKY11, WRKY53, WRKY41, MYB73, NAC062, NAC046, HSFA4A, ABCC3, CAXs, GSTs, AZFs, PODs, PME41, CYP707A2, and CDPK32 implying that Cd chelate sequestration into the vacuoles, the antioxidant system, cell wall biosynthesis pathway, and calcium signaling play a critical role in AR damage and Cd detoxification. Conjoint revealed phenylpropanoid and flavonoid biosynthesis pathways with different metabolism patterns, including the key DEM, chlorogenic acids. The results obtained using multiple approaches provide a molecular-scale perspective on plant response to AR-Cd stress.
Introduction
With the development of industries, urbanization, and agricultural activities, toxic atmospheric contaminant pollution has become a worldwide environmental issue (Shahid et al., 2017). Heavy metals (e.g., cadmium), which form the main inorganic components of atmospheric fine particulate matter (PM2.5), are highly accumulative and toxic in the environment (Wang et al., 2017). It has been reported that 25%–40% of the heavy metals in plants originate from the atmospheric particulate matter deposition in polluted areas (Schreck et al., 2012). As a non-essential element, Cd can produce serious toxic effects on plants physiologically and can detriment plants’ morphological, physiological, and molecular characteristics (He et al., 2017; Rasafi et al., 2020). Numerous academic studies found that Cd can inhibit plant growth and development by affecting multiple metabolic pathways such as plant photosynthesis, carbohydrate metabolism, nitrogen metabolism, phytohormone signaling, and ion transporter genes and transcription factors (TFs) (Chen et al., 2020; Coakley et al., 2021; Hussain et al., 2021). In recent years, a large number of genes involved in Cd regulation have been detected using transcriptomics (Peng et al., 2015), which provide an understanding of the molecular mechanisms of Cd uptake, transport, and detoxification. In addition, high-throughput sequencing strategies offer new insights into unknown transcriptional states under specific stresses. Transcriptomics, which links genetic information to biological function, plays an important role in identifying genes associated with growth and development, stress adaptation, and evolution (Pandey and Somssich, 2009). Currently, transcriptomics has been successfully applied to different response mechanisms of various plants to abiotic stress including Cd stress (e.g., rice under Cd stress (Tan et al., 2017; Cheng et al., 2018), broad bean (Rui et al., 2018), maize under drought stress (Jia et al., 2020), and peas under low-temperature stress (Min et al., 2020)). However, research on the molecular mechanism of the response induced by Cd-containing PM2.5 (PM2.5-Cd) stress in pak choi (Chinese cabbage; Brassica pekinensis (Lour.) Rupr) is scarce.
Acid rain (AR) is one of the well-known environmental problems that can negatively impact plant growth and health (Liu et al., 2016; Ma et al., 2021). It can affect the structure of plant leaves, destroy the cuticle, cause acidic components to penetrate the cells through stomata or epidermal diffusion, alter the cytoplasmic acid–base balance of plant stems and leaves, increase the permeability, cause leaves to lose a large amount of nutrients (e.g., potassium, calcium, and magnesium), and affect the expression levels of proteins involved in basic metabolism, cell building, photosynthesis, and transcription (Hu et al., 2019; Liu et al., 2019). However, a previous study on the effects of AR on plants have mainly focused on the physiological and biochemical aspects, and the gene expression patterns under AR stress have only been reported for Arabidopsis (Lee et al., 2006; Liu et al., 2019).
As an important edible part of leafy vegetables, the leaf blade is also one of the more sensitive plant parts to environmental stresses (Xiong et al., 2016; Yang et al., 2018; Liu et al., 2019; Gao et al., 2020). Leafy vegetables growing in the open air are affected by both AR and PM2.5-Cd deposition. When acid rain reacts with fine particulate matter deposited on the surface of leafy vegetables, the coordinated effect of both may significantly enhance the toxicity of the mixed compounds. In our previous study, AR (pH3.5) and PM2.5-Cd (500 μg·m−3) combined stress was found to cause growth retardation and leaf yellowing in pak choi (Zha et al., 2022), indicating that growth inhibition of leaves is one of the most obvious symptoms of Cd and AR toxicity. However, the molecular mechanisms of detoxification and tolerance of pak choi in response to AR and PM2.5-Cd stress are unknown. Based on transcriptome and metabolome analyses, we hypothesized that key resistance genes and metabolites would be significantly upregulated under the combined stresses of AR and PM2.5-Cd. In the present study, an integrated analysis combined with transcriptomics and metabolomics analyses was performed to investigate the regulatory network of pak choi under the combined stress of AR and PM2.5-Cd. The objectives of this study are to 1) identify pivotal Cd-responsive differentially expressed genes (DEGs)/metabolites (DEMs) and the essential pathways involved and 2) unveil the molecular mechanisms of detoxification and tolerance of pak choi in response to AR and PM2.5-Cd stress. The results obtained could help to further elucidate the detoxification and tolerance mechanism of pak choi exposed to AR and PM2.5-Cd stress and identify some important responsive candidate genes for phytoremediation and provide a theoretical basis for exploring the molecular mechanisms of the response of pak choi to the combined effects of AR and heavy metal-containing PM2.5. These results will provide novel insights into the combined effects of PM2.5-Cd and acid rain on leafy vegetables and might also serve as a guide to evaluate agricultural restoration and biological safety in areas with PM2.5 and acid rain pollution.
Materials and methods
Plant materials, growth conditions, and experimental design
Healthy-looking and uniformly sized seeds of pak choi (Degao508, Henan seed co, Ltd., China) were surface-sterilized with 1% sodium hypochlorite solution for 10 min, followed by repeatedly washing with double distilled water (DDW). Then, the seeds were sown in 16 soil-filled plastic pots (diameter = 12 cm, height = 10 cm) filled with the 2.0 kg air-dried clean soil (P: 0.20 g·kg−1, N: 0.46 g·kg−1, and organic matter: 11.37 g·kg−1). The yellow soil was collected from the arable layer (0–20 cm) of an agriculture field, Green Yuan family farms, Linan district, Zhejiang province, China. The soil was clay loam containing 47% sand, 28% silt, and 25% clay contents. The soil had pH and EC of 7.67 and 1.82 dS/m, respectively, and the organic matter content of 0.92%, it contained 474 mg·kg−1 of rapid available P and 528 mg·kg−1 of slowly available K; the total N was 0.145%, the total P was 794 mg·kg−1, total K was 1.42 × 104 mg·kg−1, no Cd detected in soil. The miniature greenhouse controlled conditions: 25 ± 5°C (day/night); 60%/70% relative humidity (RH, day/night) and 16 h photoperiod. The pots were divided into two groups (control and treatment), with each group comprising eight replicates. The pak choi seeds were allowed to grow up to the third true leaf stage and treated with deionized water (pH 7.0; control group) or 15-mm AR (pH 3.5; treatment group) once every 5 days. Furthermore, the treatment group was subjected to simultaneous PM2.5-Cd aerosol (produced by an aerosol generator) treatment for 480 s. The eight replicates were established in 1 day, and simulations were performed every 72 h. The plants in both the groups were harvested at the 21-day growth stage, and the leaves were removed, rapidly frozen in liquid nitrogen, and stored in a −80°C freezer.
RNA isolation and transcriptome sequencing
The collected 21-day pak choi leaf samples from both treatment and control groups were rinsed thrice with ultrapure water, immediately frozen in liquid nitrogen, and powdered. Then, 0.1 g of the leaves’ powder was extracted for the isolation of total RNA using an RNAprep Pure Kit (Tiangen, DP411, Beijing, China). The quantity and quality of the total RNA were assessed using a NanoDrop 2000 (Thermo Scientific) and Agilent Bioanalyzer 2100 system (Agilent Technologies, Santa Clara, CA, United States), respectively. The integrity of the RNA was tested using a biochip analysis system (Agilent 2100 Bioanalyzer, United States), and RNA samples with an integrity value of >7.0 were used to construct a sequencing library. The libraries were paired-end sequenced using an Illumina HiSeq Sequencer 4000 Platform at Lianchaun Personal Biotechnology Co., Ltd. A total of three biological replicates per sampling site were used for sequencing. To obtain high-quality clean reads, the raw reads were trimmed and quality-controlled by Seqprep (https://github.com/jstjohn/SeqPrep) software with default parameters. The expression level of each transcript was calculated by RSEM based on TPM (transcript per million reads). Differential expression analysis was performed using DESeq2 with a Q value ≤ 0.05, and the up- or downregulated levels were considered significantly different when the Q value was ≥ 2-fold (p < 0.05). The Gene Ontology (GO) and Kyoto Encyclopedia of Genes and Genomes (KEGG) enrichment analyses of DEGs were performed to determine the biological functions of the DEGs and the metabolic pathways predominantly enriched with DEGs.
Non-targeted metabonomics profiling analysis
The collected 21-day pak choi leaf samples from both treatment and control groups were rinsed thrice with ultrapure water, immediately frozen in liquid nitrogen, powdered, and analyzed at Hangzhou Lianchuan Biotechnology Co. A total of 100 mg of the sample powder were dissolved in a 50% methanol-buffer solution and extracted overnight at −20°C. Subsequently, the samples were centrifuged at 4000 ×g for 20 min, the supernatant was collected, and the samples were filtered through a 0.22-μm microporous filter membrane and stored at −80°C prior to liquid chromatography–mass spectrometry (LC–MS/MS) analysis. The pooled QC samples were prepared by combining 10 μl of each extraction mixture. A total of six biological replicates were set up for each sample.
All the samples were acquired by the ultra-high liquid chromatography (UPLC) system (SCIEX, United Kingdom). The chromatographic conditions are as follows: chromatographic column: waters ACQUITY UPLCHSST3 C18 (100 mm*2.1 mm, 1.8 μm). Column temperature: 35°C, flow rate: 0.4 ml/min, and sample size: 4 μl; mobile phase A is ultra-pure water (with 0.04% acetic acid), and mobile phase B is acetonitrile (with 0.04% acetic acid). The gradient elution conditions were set as follows: 00.5 min, 5% B; 0.5–7 min, 5%–100% B; 7–8 min, 100% B; 8–8.1 min, 100%–5% B; and 8.1–10 min, 5% B.
Integrated metabolome and transcriptome analyses
After combining the TPM of DEGs and DEMs in each sample, KEGG pathway annotation of significantly expressed DEGs and DEMs was performed to determine genes and metabolites that show considerable changes in the same biological process, target the metabolic pathways, and resolve the regulatory mechanisms in combination with molecular biology.
Statistical analysis
The Gene Ontology (GO) and Kyoto Encyclopedia of Genes and Genomes (KEGG) analyses of differentially expressed genes (DEGs) were performed (http://ncbi.nlm.nih.gov, http://www.geeont ology.org and http://www.kegg.jp), and the physiological data were examined with DPS 11.0. The differences among the treatments or pak choi lines were determined by the least significant difference (LSD) test at the 0.05 level.
Results
Metabolic changes in pak choi leaves exposed to combined stress of acid rain and PM2.5-Cd
Non-targeted metabolomics techniques (LC–MS/MS) were used to identify different metabolites associated with AR and PM2.5-Cd complex stresses. First, multivariate analyses [principal component analysis (PCA) and partial least squares-discriminant analysis (PLS-DA)] were conducted to visualize the clustering information profile between the control and treatment groups. Both PCA and PLS-DA score plots showed obvious distinction between the control and treatment groups (Figures 1A,B), indicating that the metabolite composition in the pak choi leaves of the control and treated groups was significantly different. A total of 4086 metabolites were screened in the two groups, including 451 common differentially expressed metabolites (DEMs, VIP ≥1 and p ≤ 0.05) (Figure1C). To better observe the expression pattern of the 451 common DEMs, hierarchical clustering analysis was implemented based on the similarity of DEM abundance profile. The analyzed DEMs could be mainly classified into phenylpropanoids and polyketides (6%), lipids (3.8%), nucleotides (3.8%), flavonoids (2.7%), organic acids and derivatives (0.8%), and others (Supplementary Table S1).
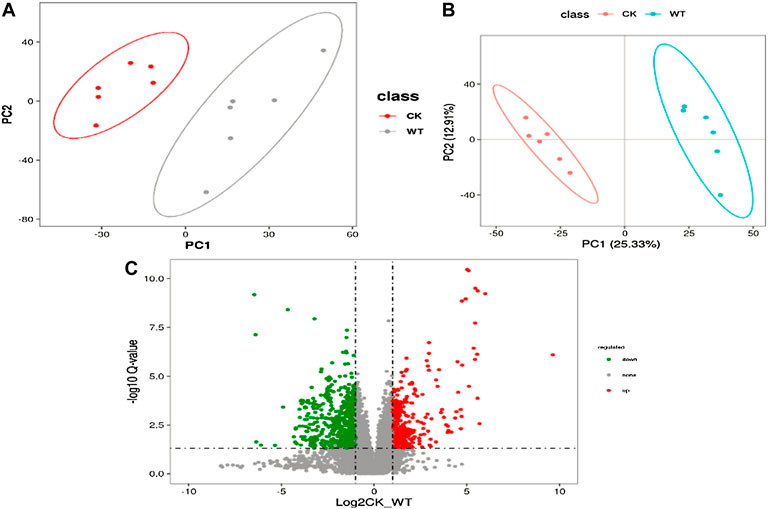
FIGURE 1. PCA (A) and PLS-DA (B) score plots of all detected metabolites in the AR-Cd treatment group. (C) Vocalno plot of differentially accumulated metabolites in CK vs. WT leaves. Red dots are metabolites accumulated more in AR-Cd treatment, and green dots are metabolites accumulated more in control treatment.
The DEMs with high VIP values were mainly prenol lipids, organooxygen compounds, and isoflavonoids, such as costunolide, geranylhydroquinone, 2-hydroxy-3-oxopropanoate, glycitein, and 13(s)-hOTrE (Supplementary Table S1). Fold change and VIP value revealed that the accumulation of most of the metabolites (cyclopamine, (3s)-9-hydroxy-3-(methoxycarbonyl)-2-methylenenonanoic acid, costunolide, geranylhydroquinone, etc.), was lower, while that of 4,4'-(9 h-fluorene-9,9-diyl)bis (2-fluoroaniline), 3-vinyltoluene, and 6-deoxy-2,3-di-o-methyl-d-allopyranose was higher after AR-Cd treatment (Table 1). Figure 2 shows the hierarchical clustering analysis of DEMs between the control and treatment groups, which indicated that these metabolites might play a crucial role in the response of pak choi leaves to combined stress of AR and PM2.5-Cd.
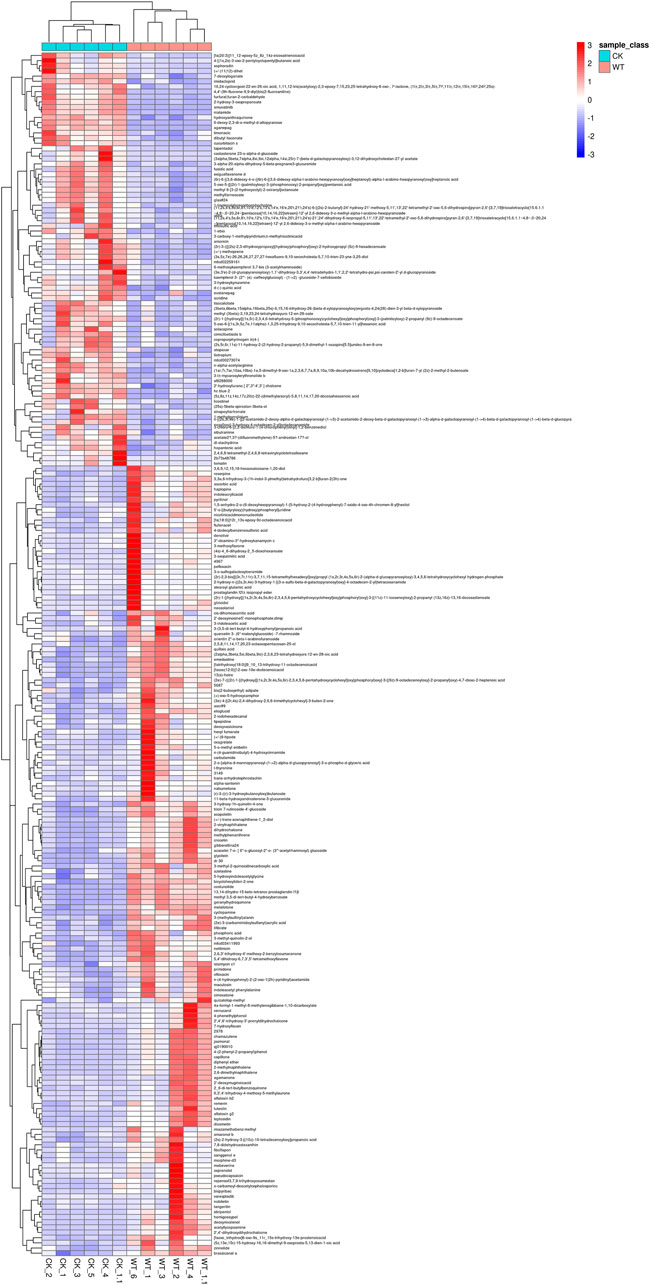
FIGURE 2. Hierarchical clustering analysis of differentially expressed metabolites between the control and AR-Cd treatment. (CK-WT heatmap-metabolite)
Pathway analysis of differentially expressed metabolites
KEGG enrichment pathway analysis further identified key metabolic pathways associated with the response of pak choi leaves to the combined stress of AR and PM2.5-Cd.As shown in Figure 3, 42 significant metabolic pathways (Q≤0.05) were identified by searching the KEGG database for differentially expressed metabolites in plants under combined AR and PM2.5-Cd stresses. Secondary metabolite biosynthesis-, phenylpropanoid biosynthesis-, and flavonoid biosynthesis-related pathways presented better significance and rich factor. A total of seven DEMs were mapped to flavonoid biosynthesis (ko00941), four were mapped to isoflavonoid biosynthesis (ko00943), and five metabolites were mapped to phenylpropanoid biosynthesis (ko00940). In addition, DEMs involved in tryptophan metabolism, flavone and flavonol biosynthesis, ascorbate and aldarate metabolism, ABC transporters, photosynthesis, phenylalanine metabolism, oxidative phosphorylation, glutathione metabolism, plant hormone signal transduction as well as arginine and proline metabolism were also significantly enriched.
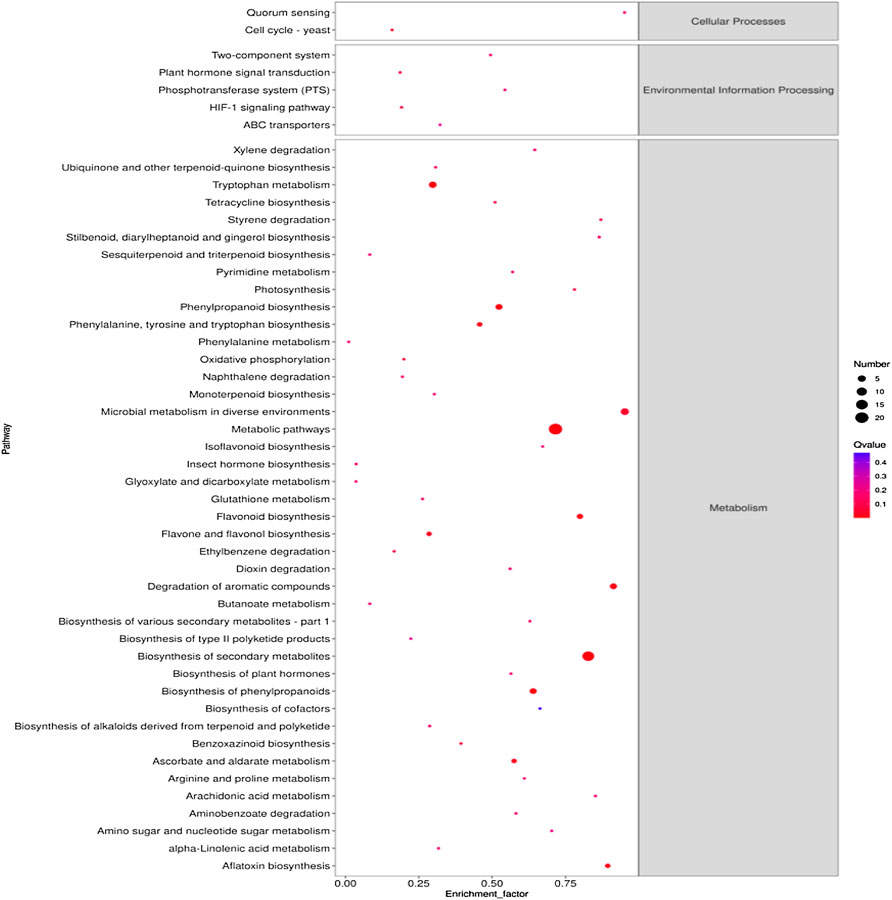
FIGURE 3. KEGG metabolic pathway enrichment analysis of the differential metabolites in different AR-Cd treatment groups. The most significant catalogs with lowest corrected p values are shown.
Transcriptome sequencing and differentially expressed gene identification
To further understand the molecular mechanisms of the response of pak choi to AR and PM2.5-Cd stresses, transcriptome sequencing studies on AR and PM2.5 resistance of pak choi were conducted using the HiSeq.2500 platform (Illumina). A total of 47,107 unigenes were detected (Supplementary Table S2), and the correlation coefficient between the control and treatment groups revealed > 92.8% correlation among the different biological replicates, indicating high correlation between the samples and good biological repeatability, and that the data can be used for subsequent analysis (Figure 4A). The results of PCA showed that the expression of gene clusters in control and treatment groups varied (Figure 4B). Hence, the two groups were compared to identify DEGs. As shown in Figure 4C and Supplementary Table S3, 735 (557 upregulated and 178 downregulated) DEGs were identified by transcriptome sequencing analysis between the control and treatment groups, implying that pak choi leaves activated numerous gene expression to cope with AR and PM2.5-Cd toxicity.
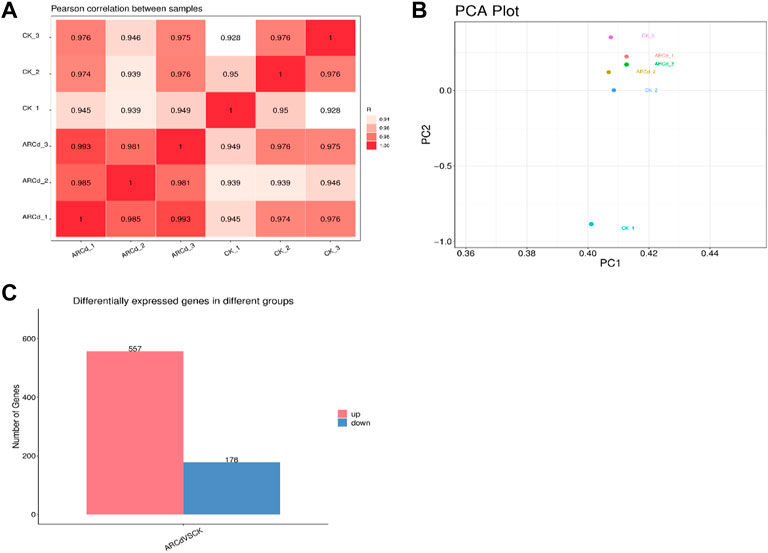
FIGURE 4. Correlation analysis of patterns (A) of gene expression in control and AR-Cd-treated groups. Principal component analysis (PCA) (B) of FPKM profiles in control and AR-Cd treated groups. (C) Number of differentially express genes (DEGs) in AR-Cd treated groups.
Gene Ontology and Kyoto Encyclopedia of Genes and Genomes pathway analysis of differentially expressed genesThe GO enrichment analysis was performed to elucidate the specific biological functions of the DEGs in the two groups. The results revealed that the DEGs were mainly enriched in response to jasmonic acid, TF activity, oxidative stress, abscisic acid, Ca2+ ion binding, carbohydrate metabolic process, metal ion binding, cadmium ion, calcium-mediated signaling pathway, etc. (Figure 5A).
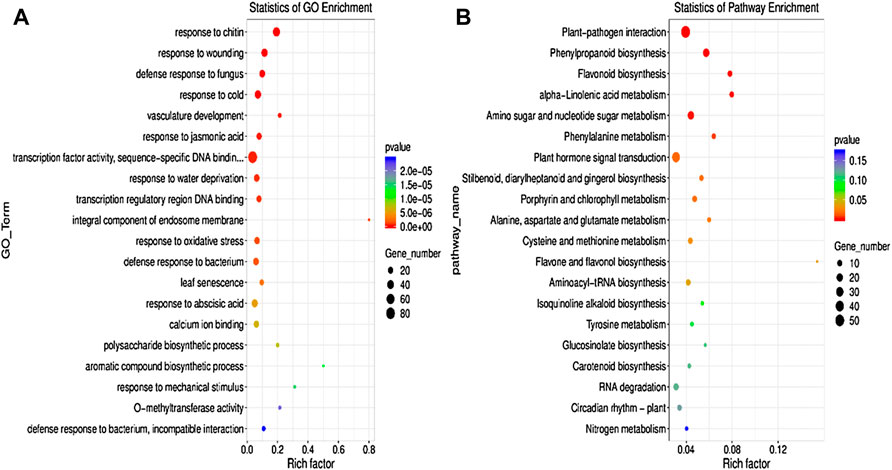
FIGURE 5. Gene Ontology (GO) (A) pathway and KEGG (B) pathway enrichment analyses of DEGs between the control and AR-Cd treated groups. The rich factor indicates the degree of enrichment of the GO items and KEGG pathways.
To confirm the biological pathways induced by AR-Cd stress, all the DEGs were assigned to the KEGG database for KEGG pathway enrichment analysis. The findings revealed significant enrichment of numerous DEGs in metabolic pathways and secondary metabolite biosynthesis. In particular, the DEMs of KEGG enrichment analysis, DEGs are mainly enriched in phenylpropanoid biosynthesis, flavonoid biosynthesis, plant hormone signal transduction, nitrogen metabolism, oxidative phosphorylation, ABC transporters, porphyrin and chlorophyll metabolism, carbon fixation in photosynthetic organisms, and glutathione metabolism (Figure 5B). These results indicated that AR-Cd stress significantly disturbed plant physiological processes.
Integrated analysis of metabolites and transcripts in the phenylpropanoid and flavonoid biosynthesis pathways
Integrated analysis indicated that the metabolism and expression patterns of metabolites and transcripts involved in 22 KEGG reference pathways, including flavonoid and phenylpropanoid biosynthesis, varied between the control and treatment groups. In the treatment group, three DEGs, predicted as PAL, CCR, and COMT, involved in the phenylpropanoid biosynthesis pathway were upregulated, and 5-hydroxyferulic acid accumulation increased. In addition, the expressions of two DEGs, predicted as CCoAOMT and HCT, in the flavonoid biosynthesis pathway were also upregulated, and the chlorogenic acid content remarkably increased in the treatment group. Figures 6, 7 show the phenylpropanoid and flavonoid biosynthesis pathways and the connection network, respectively.
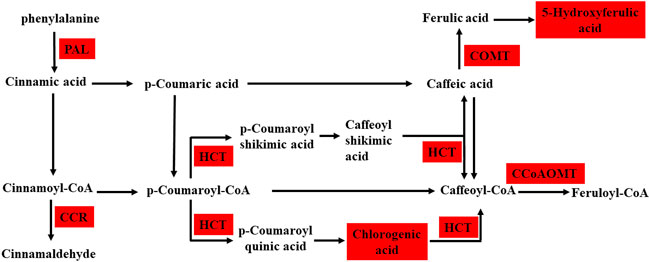
FIGURE 6. Schematic diagram shows phenylpropanoid and flavonoid biosynthesis between the control and AR-Cd treated groups, the red box indicates the common upregulated DEGs and DEMs. This pathway is constructed based on the KEGG pathway. Genes that are differently expressed include phenylalanine ammonia-lyase (PAL), cinnamoyl-CoA reductase (CCR), shikimate O-hydroxycinnamoyltransferase (HCT), caffeoyl-CoA O-methyltransferase (CCoAOMT), and caffeic acid 3-O-methyltransferase (COMT).
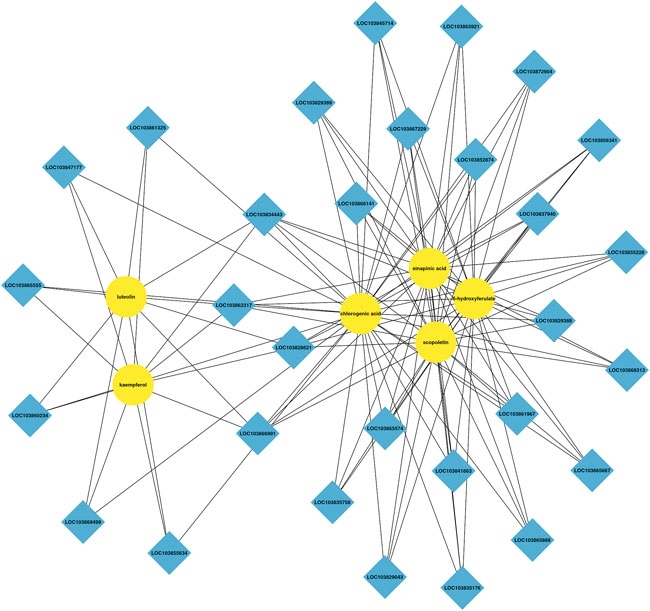
FIGURE 7. Connection network between differentially accumulated metabolites and related genes between the control and AR-Cd treatment.
Differentially expressed genes related to transcription factors and signal transduction under AR-Cd stress
TFs are the key regulators of the level of gene expression and are mainly involved in the initial stages of RNA transcription. In the present study, a large number of TFs responsive to the combined AR-Cd stress in pak choi were identified. A total of 45 TFs corresponding to seven families of TFs, including WRKY, MYB, bHLH, NAC, zinc finger protein, Trihelix, and heat stress TFs (HSF TFs), were detected among the DEGs between the control and treatment groups. In particular, the expression levels of many WRKY, MYB, NAC, and zinc finger protein TFs, including WRKY11, WRKY53, WRKY41, MYB73, NAC046, NAC062, and HSFA4A, were higher in the treatment group (Figure 8A).
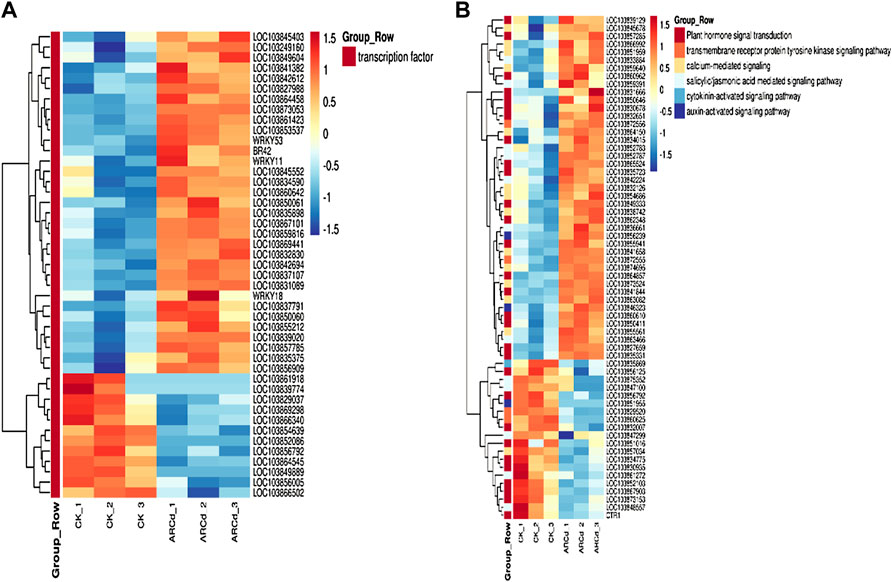
FIGURE 8. (A) Heat maps represented the log2-fold change in the expression of DEGs (red is upregulated and blue is downregulated) related to transcription factors between the control and AR-Cd treated groups. (B) Heat maps represented the log2-fold change in the expression of DEGs (red is upregulated and blue is downregulated) related to hormone signal transduction and calcium-mediated signaling between the control and AR-Cd treated groups.
Furthermore, genes associated with hormone signal transduction including three abscisic acid signal transduction DEGs (LOC103839129, LOC103830935, and LOC103854686), four IAA signal transduction DEGs (LOC103865524, LOC103841844, LOC103832007, and LOC103846323), and two gibberellin signal transduction DEGs (LOC103850646 and LOC103856125), which mainly encode abscisic acid 8′-hydroxylase 3, auxin-responsive protein SAUR22-like protein, auxin efflux carrier component 5, gibberellin 20 oxidase 3-like protein, and gibberellin-regulated protein 4, were differentially expressed between the control and treatment groups. A total of 14 genes related to calcium signal pathways, including five calmodulin (CaM)-related proteins (LOC103873524, LOC103857034, LOC103874695, LOC103863082, and LOC103854686), seven calcium-binding proteins (LOC103832126, LOC103864150, LO C103866992, LOC103841658, LOC103838742, LOC103851959, and LOC103859640), and two calcineurin proteins (LOC103855561 and LOC103845678), were significantly upregulated in the treatment group (Figure 8B).
Noticeable differentially expressed genes related to metabolic pathways under AR-Cd stress
Previous studies had indicated that vacuolar sequestration and cell wall retention of Cd might be the main causes of the differences in genetic expression in plants under Cd stress (Min et al., 2020). Transcriptomic analysis had revealed that the expression of a set of genes related to primary metabolisms, including nitrogen, sulfur, amino acid, photosynthesis, and reactive oxygen species (ROS) metabolism, were altered under SiAR (Liu et al., 2019). Consequently, in the present study, the metabolic pathways and DEGs related to ABC transporters, glutathione (GSH), cell wall metabolism, metal ion transporter, cation/H+ exchanger, sulfur and nitrogen metabolism, cell wall metabolism, TCA cycle, nitrogen metabolism, carbohydrate metabolism, photosynthesis metabolism, galactose metabolism, arginine and proline metabolism, response to oxidative stress and antioxidant system were analyzed (Figure 9).
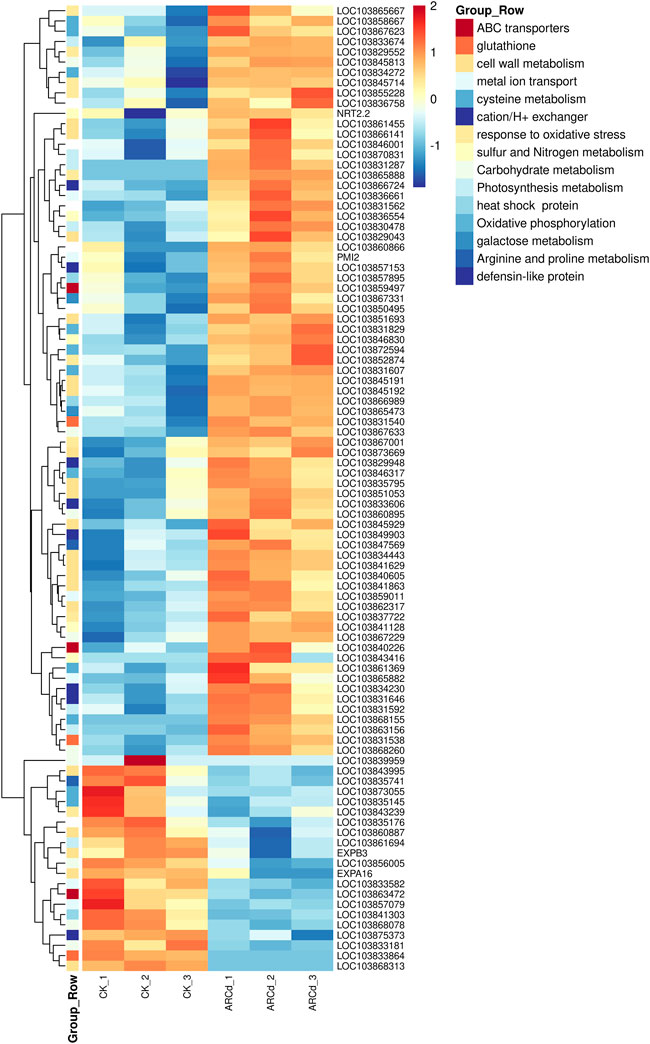
FIGURE 9. Heat maps represented the log2-fold change in the expression of DEGs (red is upregulated and blue is downregulated) related to some important genes between the control and AR-Cd-treated groups.
An investigation of DEGs involved in the vacuolar sequestration of dissociative Cd chelates found three DEGs (ABCG27, ABCG14, and ABCC3) related to ABC transporters. The expression level of LOC103859497 is upregulated, which encodes ABC transporter C family member 3, an ATP-dependent MRP-like ABC transporter that can transport glutathione conjugates, was upregulated in the treatment group. Furthermore, the expressions of two metal ion transport–associated genes (LOC103831562 and LOC103850495) were upregulated in response to Cd ion. In addition, the expression level of five cation/H+ exchanger DEGs (LOC103831646, LOC103829948, LOC103833606, LOC103834230, and LOC103857153) was upregulated (Figure 9), such as LOC103829948 encoding the putative Na+/H+ antiporter family member is involved in the osmoregulation through K(+) fluxes and possible pH modulation of an active endomembrane system, was also upregulated in the treatment group. These results indicated that Cd chelate sequestration into the vacuoles play a key role under AR-Cd stress.
A total of 20 DEGs associated with cell wall metabolism were detected, including expansin, beta-glucosidase, xyloglucan endotransglucosylase/hydrolase protein, cellulose synthase–like protein, and pectin methyl (PEM) were detected in the present study. It must be noted that polysaccharides (cellulose and pectin) are the main components of the cell wall. Interestingly, we noticed that the expression level of BrPME41 (LOC103836758) was upregulated in the treatment group, indicating that AR-Cd stress promoted pectin degradation, which, conversely, might simulate Cd fixation in the cell wall. Furthermore, the expression of three DEGs (LOC103845929, EXPA16, and EXPB3) related to expansin, five (LOC103841863, LOC103841629, LOC103829043, LOC103868313, and LOC103866141) related to beta-glucosidase, three DEGs (LOC103845191, LOC103845192, and LOC103873669) related to xyloglucan endotransglucosylase/hydrolase protein, five peroxidases (LOC103855228, LOC103845714, LOC103852874, LOC103865888, and LOC103865667) related to cellulose synthase, and two DEGs (LOC103861455 and LOC103843995) relative to cellulose synthase were altered in the treatment group (Figure 9), indicating that AR-Cd stress changed the cell wall compositions by modifying pectin, cellulose, and lignin.
Glutathione S-transferases (GSTs) are the important enzymes that participate in GSH metabolism and re-establish the GSH/GSSH ratio to control values during the ROS resistance pathway. They are involved because GST is used in direct excess metal ion quenching as GSH-metal ion compound and sequestration within vacuoles (Lee et al., 2006). In the present study, two DEGs (LOC103831538 and LOC103831540) related to glutathione metabolism were detected, and both of them encode GST and are involved in biosynthesis of GSH and phytochelatin biosynthesis. The expression of GST genes was upregulated in the AR-Cd treatment, indicating AR-Cd stress induced GSH synthesis. In addition, the expressions of five peroxidases (LOC103855228, LOC103845714, LOC103852874, LOC103865888, and LOC103865667), HSPs (LOC103866989, LOC103841303 and LOC103857895), and proline transporters (LOC103867001) were upregulated in the treatment group (Figure 9). These DEGs could be ROS, playing a vital role in alleviating oxidative stress. Thus, these results indicated that the presence of AR-Cd stress causes damage to plant cells by elevating the ROS levels.
Previous studies have reported that the photosynthetic physiology of plants is impaired under AR-Cd stress (Yang et al., 2018; Liu et al., 2019). In the present study, eight DEGs (LOC103841629, LOC103861694, LOC103830478, LOC103870831, LOC103831592, LOC103863156, LOC103831287, and LOC103833674) were found to be related to photosynthesis (Figure 9). In particular, the expression of LOC103861694 encoding protochlorophyllide reductase B was downregulated, which disturbed photosynthesis, indicating that the pak choi leaves were directly damaged via disruption of photosynthesis metabolism under AR-Cd stress.
Discussion
The phenylpropanoid pathway comprises many important plant secondary metabolites including lignin, chlorogenic acids, flavonoids, and phenolic glycosides. Flavonoids belong to polyphenols, and some common flavonoids include anthocyanins, flavonols, flavones, and proanthocyanidins (PAs), many of which have been extensively studied and are generally considered to play a role in defense against biological and abiotic stresses (Ma et al., 2018). Chlorogenic acid is an important intermediate product in the phenylpropane synthesis pathway. In plants, chlorogenic acid as a defense metabolite may be related to the defense signaling network activated by salicylic acid and methyl-jasmonate (Mhlongo et al., 2016; Yin et al., 2021). Hodaei et al. (2018) showed that drought stress could increase the concentration of chlorogenic acid in chrysanthemum. Caffeoyl coenzyme A 3-O-methyltransferase, which is involved in chlorogenic acid metabolism, can convert caffeoyl-CoA to feruloyl-CoA for flavonoid and lignin biosynthesis, and accumulation of chlorogenic acid can promote flavonoid production. In the present study, the accumulation of many DEMs mapped to flavonoid and phenylpropanoid biosynthesis pathways, including chlorogenic acid, increased in the AR-Cd treatment group (Figure 6), suggesting that chlorogenic acid may play a key role under AR-Cd stress.
To further study the molecular adaptation mechanism of pak choi under AR-Cd stress, we analyzed and examined the transcriptome of pak choi under AR-Cd stress. A total of 557 upregulated and 178 downregulated DEGs were identified between the control and treatment groups (Figure 4C), and some crucial DEGs were detected. In response to Ad-Cd stress, WRKY, MYB, NAC and zinc finger protein, and HSF were differentially expressed and many TF DEGs were upregulated (Figure 8A). WRKY11 is involved in abiotic stress responses, and TF WRKY53 is a mutual antagonist in the regulation of the plant stress response. TF WRKY41 is involved in the modulation of defense signal transduction in Arabidopsis thaliana (Higashi et al., 2008). LOC103864458, TF MYB73 is involved in plant defense regulation (Wang et al., 2021), and LOC103855212 encodes NAC046. It has been reported that NAC046 mutants exhibited delayed senescence and increased the chlorophyll content, suggesting the role of NAC046 in the regulation of senescence and chlorophyll degradation (Oda-Yamamizo et al., 2016). LOC103873053 has been found to encode, NAC062 in Brassica rapa, and the heterologous expression of BrNAC062 homologous TF EsNAC1 has been noted to enhance abiotic resistance and inhibit the growth of A. thaliana by regulating the expression of different target genes (Liu et al., 2013). LOC103827988 encodes zinc finger protein AZF1, which has been used for the systematic identification of genes related to plant growth defense tradeoffs under JA signaling in A. rabidopsis (Zhang et al., 2020). BR42 and LOC103837107 encode AZF1 and AZF2, respectively, which can negatively regulate and it has been reported that bidopsis C2H2 zinc finger proteins AZF1 and AZF2 negatively regulate ABA-repressive and auxin-inducible genes under abiotic stress conditions (Kodaira et al., 2011). LOC103839020 encoding zinc finger protein ZAT10 has been observed to respond to abscisic acid and oxidative stress and increase the ascorbate level in A. rabidopsis (Zhou et al., 2009). LOC103856909 encodes a member of Hsf family A-4a (HSFA4A), which acts as a substrate of the (MPK3/MPK6). With regard to TF DEGs down in response to AR-Cd stress (Pérez-Salamó et al., 2014), the expressions of LOC103854639 and LOC103856792 were decreased. LOC103854639 encodes trihelix GTL2 TF, a Ca(2+)-dependent CaM-binding protein, and plant-specific trihelix TF AtGT2L is known to interact with calcium/CaM in response to stress (Xi et al., 2012). LOC103856792 encodes a member of the GATA factor family of zinc finger TFs, which modulates chlorophyll biosynthesis and glutamate synthase (GLU1/Fd-GOGAT) expression, and regulates the nitrogen compound metabolic process (Hudson et al., 2011). Thus, these TFs play important roles in governing transcriptional activation and repression in pak choi under AR-Cd stress.
Furthermore, the expressions of the LOC103839129, LOC1-3841658, and LOC103874695 were upregulated in the AR-Cd treatment group. LOC103839129 encodes abscisic acid 8′-hydroxylase 3(CYP707A2), which plays a major role in the rapid decrease in ABA levels (Kim et al., 2012). LOC103841658 encodes calcium-dependent protein kinase CDPK32, which is involved in plant growth regulation and abiotic stress responses (Sadhukhan et al., 2021), and LOC103874695 encodes CaM-binding protein 60A (Figure 8B), which regulates vacuolar Na+/H+ antiporter cation in a Ca2+- and pH-dependent manner (Yamaguchi et al., 2005). One of the important roles of CaM in plants is to regulate intracellular calcium levels. Calmodulin is also known as a mediator of calcium signal transduction and controls the expression of target genes (Yang and Poovaiab, 2003). CaM is also known to be a mediator of cadmium signal transduction and can control the expression of target genes. It is an important Ca2+ signal receptor in the cells and plays a role in calcium signal transduction under stress, CaM can bind to excess Ca2+ in the cytoplasm to form active Ca2+-CaM, which can regulate the activity of many downstream target proteins such as H2O2, SOD, GR, CAT, and Ca2+ -ATPase. In addition, CaM can also be induced by Ca2+. The results suggested that diversified plant hormone signal transduction and calcium signaling are involved in the response of plants to AR-Cd stress.
The transport of PC (phytochelatinsynthetase)-Cd chelates into the vacuoles is believed to be mediated mainly by ABCC1-3 transporters (Zhao et al., 2009; Park et al., 2012; Brunetti et al., 2015). BnABCC3 may be a central member of the differential vacuolar sequestration of Cd-resistant and Cd-sensitive genotypes of phytochelatinsynthetase-Cd complexes (Zhang et al., 2020). In the present study, the mRNA levels of ABCC3 DEGs were significantly higher than mRNA levels in the AR-Cd stress treatment (Figure 9). LOC103831562 and LOC103850495 encode efflux family protein, which is involved in xenobiotic detoxification by transmembrane export across the plasma membrane and is essential for cation binding and the detoxification of AR-Cd stress (Paul et al., 1996). The cation exchangers (CAXs) are tonoplast-localized, which play a key role in the sequestration of vacuoles and detoxification (Zhao et al., 2009; Sui et al., 2019).
Pectin and cellulose functional groups can bind to metal ions, and the cell wall forms a barrier that prevents heavy metals from entering the root cells (Luo et al., 2018). PMEs control pectin demethylation by regulating the number of negatively charged free carboxyl groups and hydroxyl groups, which significantly affects the ability of the cell wall to capture Cd (Song et al., 2017). An appropriate increase in PME expression and PME activity can improve the degree of pectin demethylation, resulting in more Cd-binding sites in the cell wall (Gutsch et al., 2018). Furthermore, more heavy metals bind to pectin with the increase in the degree of demethylation esterification of pectin (Lozano-Rodriguez et al., 1997). ATPME41 encodes a PME that is sensitive to brassinosteroid regulation and is involved in cell wall modification (Qu et al., 2011). These results indicated that AR-Cd stress could significantly influence the genes involved in cell wall biosynthesis in plants.
Excess ROS formation leads to oxidative damage of cellular components such as lipids, proteins, and nucleic acids, and even cell death (Foreman, 2003; Miller et al., 2010). The antioxidant defense system plays an important role in scavenging ROS in order to protect plant cells against oxidative stress (Bose et al., 2014; Zhang et al., 2016). In the present study, oxidative stress–related TFs, such as LOC103839020 and LOC103856909 were upregulated in pak choi exposed to AR-Cd stress (Figure 7). Peroxidase, as a member of the detoxification system of antioxidant enzymes, is believed to be involved in lignification, cell wall composition cross-linking, and resistance to heavy metal stress (Kim et al., 2010; Gutsch et al., 2018). Some studies had also demonstrated that peroxidase can scavenge ROS and regulate lignin biosynthesis, induce cell wall hardening, and influence heavy metal transport under trace metal stress (Qiao et al., 2019; Zhao et al., 2019; Chen et al., 2021). In the present study, five peroxidases (LOC103855228, LOC103845714, LOC103852874, LOC103865888, and LOC103865667) in pak choi were exposed to AR-Cd stress (Figure 9), revealing that the antioxidant enzymes in pak choi provide an efficient antioxidant defense mechanism against AR-Cd stress.
Photosynthesis is the basic metabolic process in plant growth and development, which is very sensitive to various abiotic stresses (Zheng et al., 2009; Liu et al., 2022). In the present study, the expression level of BrNAC046 was upregulated, while that the expression level of LOC103861694 was downregulated in pak choi exposed to AR-Cd stress (Figure 8), indicating disruption of photosynthesis and suppression of chlorophyll biosynthesis under AR-Cd stress.
Conclusion
In summary, Cd toxicity and AR significantly inhibited plant growth and physiology, and resulted in increased accumulation of some metabolites associated with phenylpropanoid and flavonoid biosynthesis pathways, such as chlorogenic acid. Furthermore, RNA-seq results revealed the upregulation of numerous crucial DEGs, including those involved in the biosynthesis pathway of cell wall components (pectin, lignin, and cellulose), Cd chelates sequestration into the vacuoles, antioxidant system, and calcium signaling were used to alleviate AR-Cd stress. The present study will provide our understanding of the mechanism through which leafy vegetables tolerate PM2.5-Cd and acid rain, and lay the foundation for the remediation of leafy vegetable contamination through molecular breeding methods. In addition, this project is an important basic work to investigate the mechanism of environmental pollution on the safety of agricultural products. Importantly, our research results can provide theoretical basis and technical support for the sustainable development of agroecosystems in regions with severe AR and PM2.5-Cd pollution in the future.
Data availability statement
The original contributions presented in the study are included in the article/Supplementary Material; further inquiries can be directed to the corresponding author.
Author contributions
YZ: conceptualization, writing, and methodology. BZ: data curation. JR: visualization. XL: writing and investigation.
Funding
This work was supported by the National Natural Science Foundation of Zhejiang Province LQ20C030007 and Science and Tech-nology Innovation Fund of Hangzhou Academy of Agricultural Sciences 2022HNCT-13.
Conflict of interest
The authors declare that the research was conducted in the absence of any commercial or financial relationships that could be construed as a potential conflict of interest.
Publisher’s note
All claims expressed in this article are solely those of the authors and do not necessarily represent those of their affiliated organizations, or those of the publisher, the editors, and the reviewers. Any product that may be evaluated in this article, or claim that may be made by its manufacturer, is not guaranteed or endorsed by the publisher.
Supplementary material
The Supplementary Material for this article can be found online at: https://www.frontiersin.org/articles/10.3389/fenvs.2022.1020308/full#supplementary-material
References
Bose, J., Ana, R. M., and Sergey, S. (2014). ROS homeostasis in halophytes in the context of salinity stress tolerance. J. Exp. Bot. 65 (5), 1241–1257. doi:10.1093/jxb/ert430
Brunetti, P., Zanella, L., De Paolis, A., Di Litta, D., Cecchetti, V., Falasca, G., et al. (2015). Cadmium-inducible expression of the ABC-type transporter AtABCC3 increases phytochelatin-mediated cadmium tolerance in Arabidopsis. J. Exp. Bot. 66 (13), 3815–3829. doi:10.1093/jxb/erv185
Chen, H. C., Zhang, S. L., Wu, K. J., Li, R., He, X. R., He, D. N., et al. (2020). The effects of exogenous organic acids on the growth, photosynthesis and cellular ultrastructure of Salix variegata Franch. Under Cd stress. Ecotoxicol. Environ. Saf. 187, 109790. doi:10.1016/j.ecoenv.2019.109790
Chen, X., Wang, J., Hayat, K., Zhang, D., and Zhou, P. (2021). Small structures with big impact: Multi-walled carbon nanotubes enhanced remediation efficiency in hyperaccumulator solanumnigrum L. Under cadmium and arsenic stress. Chemosphere 276, 130130. doi:10.1016/j.chemosphere.2021.130130
Cheng, D., Tan, M., Yu, H., Li, L., Zhu, D., Chen, Y., et al. (2018). Comparative analysis of Cd-responsive maize and rice transcriptomes highlights Cd co-modulated orthologs. BMC Genomics 19, 709. doi:10.1186/s12864-018-5109-8
Coakley, S., Cahill, G., Enright, A-M., O'Rourke, B., and Petti, C. (2021). Enzymatic response to cadmium by impatiens glandulifera: A preliminary investigation. Biochem. Biophys. Rep. 26, 100936. doi:10.1016/j.bbrep.2021.100936
Foreman, J., Demidchik, V., Bothwell, J. H. F., Mylona, P., Miedema, H., Torres, M. A., et al. (2003). Reactive oxygen species produced by NADPH oxidase regulate plant cell growth. Nature 422 (6930), 442–446. doi:10.1038/nature01485
Gao, P. P., Xue, P. Y., Dong, J. W., Zhang, X. M., Sun, H. X., Geng, L. P., et al. (2020). Contribution of PM2.5-Pb in atmospheric fallout to Pb accumulation in Chinese cabbage leaves via stomata. J. Hazard. Mat. 407, 124356. doi:10.1016/j.jhazmat.2020.124356
Gutsch, A., Zouaghi, S., Renaut, J., Cuypers, A., Hausman, J. F., and Sergeant, K. (2018). Changes in the proteome of Medicago sativa leaves in response to long-term cadmium exposure using a cell-wall targeted approach. Int. J. Mol. Sci. 19, 2498. doi:10.3390/ijms19092498
He, S., Yang, X., He, Z., and Baligar, V. C. (2017). Morphological and physiological responses of plants to cadmium toxicity: A review. Pedosphere 27, 421–438. doi:10.1016/s1002-0160(17)60339-4
Higashi, K., Ishiga, Y., Inagaki, Y., Toyoda, K., Shiraishi, T., and Ichinose, Y. (2008). Modulation of defense signal transduction by flagellin-induced WRKY41 transcription factor in Arabidopsis thaliana. Mol. Genet. Genomics 279, 303–312. doi:10.1007/s00438-007-0315-0
Hodaei, M., Rahimmalek, M., Arzani, A., and Talebi, M. (2018). The effect of water stress on phytochemical accumulation, bioactive compounds and expression of key genes involved in flavonoid biosynthesis in Chrysanthemum morifolium L. Ind. Crops Prod. 120, 295–304. doi:10.1016/j.indcrop.2018.04.073
Hu, X. F., Wu, A. Q., Wang, F. C., and Chen, F. S. (2019). The effects of simulated acid rain on internal nutrient cycling and the ratios of Mg, Al, Ca, N, and pin tea plants of a subtropical plantation. Environ. Monit. Assess. 191 (2), 99–113. doi:10.1007/s10661-019-7248-z
Hudson, D., Guevara, D., Mahmoud, W. Y., Hannam, C., Long, N., Clarke, J. D., et al. (2011). GNC and CGA1 modulate chlorophyll biosynthesis and glutamate synthase (GLU1/Fd-GOGAT) expression in Arabidopsis. PLoS One 6 (11), e26765. doi:10.1371/journal.pone.0026765
Hussain, B., Ashraf, M. N., Shafeequr, R., Abbas, A., Li, J., and Farooq, M. (2021). Cadmium stress in paddy fields: Effects of soil conditions and remediation strategies. Sci. Total Environ. 754, 142188. doi:10.1016/j.scitotenv.2020.142188
Jia, S., Li, H., Jiang, Y., Tang, Y., Zhao, G., Zhang, Y., et al. (2020). Transcriptomic analysis of female panicles reveals gene expression responses to drought stress in maize (Zea mays L.). Agronomy 10, 313. doi:10.3390/agronomy10020313
Kim, J., Malladi, A., and van Iersel, M. W. J. (2012). Physiological and molecular responses to drought in petunia: The importance of stress severity. J. Exp. Bot. 63 (18), 6335–6345. doi:10.1093/jxb/ers285
Kim, Y. H., Lee, H. S., and Kwak, S. S. (2010). Differential responses of sweet potato peroxidases to heavy metals. Chemosphere 81 (1), 79–85. doi:10.1016/j.chemosphere.2010.06.063
Kodaira, K. S., Qin, F., Phan Tran, L. S., Maruyama, K., Kidokoro, S., Fujita, T., et al. (2011). Arabidopsis Cys2/His2 zinc-finger proteins AZF1 and AZF2 negatively regulate abscisic acid-repressive and auxin-inducible genes under abiotic stress conditions. Plant Physiol. 157 (2), 742–756. doi:10.1104/pp.111.182683
Lee, Y., Park, J., Im, K., Lee, J., Park, J. A., Lee, T. K., et al. (2006). Arabidopsis leaf necrosis caused by simulated acid rain is related to the salicylic acid signaling pathway. Plant Physiol. Biochem. 44 (1), 38–42. doi:10.1016/j.plaphy.2006.01.003
Liu, T. W., Niu, L., Fu, B., Chen, J., Wu, F. H., Wang, W. H., et al. (2013). A transcriptomic study reveals differentially expressed genes and pathways respond to simulated acid rain in Arabidopsis thaliana. Genome 56 (1), 49–60. doi:10.1139/gen-2012-0090
Liu, L., Zhang, X., Wang, S., Zhang, W., and Lu, X. (2016). Bulk sulfur (S) deposition in China. Atmos. Environ. X. 135, 41–49. doi:10.1016/j.atmosenv.2016.04.003
Liu, H. L., Zhou, J., Li, M., Hu, Y. M., Liu, X., and Zhou, J. (2019). Study of the bioavailability of heavy metals from atmospheric deposition on the soil-pakchoi (Brassica chinensis L.) system. J. Hazard. Mat. 362, 9–16. doi:10.1016/j.jhazmat.2018.09.032
Liu, X., Ma, S. L., Jia, Z. H., Ramzan, M., Meng, M. J., Wang, J. P., et al. (2022). Complex effects of different types of acid rain on root growth of Quercusacutissima and Cunninghamialanceolata saplings. Ecol. Process. 11 (1), 8–14. doi:10.1186/s13717-021-00351-z
Lozano-Rodriguez, E., Hernandez, L. E., Bonay, P., and Carpena-Ruiz, R. O. (1997). Distribution of cadmium in shoot and root tissues1. J. Exp. Bot. 48, 123–128. doi:10.1093/jxb/48.1.123
Luo, J. S., Huang, J., Zeng, D. L., Peng, J. S., Zhang, G. B., Ma, H. L., et al. (2018). A defensin-like protein drives cadmium efflux and allocation in rice. Nat. Commun. 9 (1), 645. doi:10.1038/s41467-018-03088-0
Ma, D. W., Michael, R., Kazuko, Y., Jonathan, G., and Peter, C. C. (2018). Two R2R3-MYB proteins are broad repressors of flavonoidand phenylpropanoid metabolism in poplar. Plant J. 96 (5), 949–965. doi:10.1111/tpj.14081
Ma, S., Liu, X., Jia, Z., Meng, M., Zhang, J., Ren, Q., et al. (2021). Response of QuercusAcutissima foliage to different types of simulated acid rain. Atmos. Pollut. Res. 12, 101112–1011120. doi:10.1016/j.apr.2021.101112
Mhlongo, M. I., Steenkamp, P. A., Piater, L. A., Madala, N. E., and Dubery, I. A. (2016). Profiling of altered metabolomic states in Nicotianatabacum cells induced by priming agents. Front. Plant Sci. 7, 1527. doi:10.3389/fpls.2016.01527
Miller, G., Suzuki, N., Ciftci-Yilmaz, S., and Mittler, R. (2010). Reactive oxygen species homeostasis and signal ling during drought and salinity stresses. Plant Cell Environ. 33 (4), 453–467. doi:10.1111/j.1365-3040.2009.02041.x
Min, X. Y., Liu, Z. P., Wang, Y. R., and Liu, W. X. (2020). Comparative transcriptomic analysis provides insights into the coordinated mechanisms of leaves and roots response to cold stress in Common Vetch. Ind. Crops Prod. 158, 112949. doi:10.1016/j.indcrop.2020.112949
Oda-Yamamizo, C., Mitsuda, N., Sakamoto, S., Ogawa, D., Ohme-Takagi, M., and Ohmiya, A. (2016). The NAC transcription factor ANAC046 is a positive regulator of chlorophyll degradation and senescence in Arabidopsis leaves. Sci. Rep. 6, 23609. doi:10.1038/srep23609
Pandey, S. P., and Somssich, I. E. (2009). The role of WRKY transcription factors in plant immunity. Plant Physiol. 150, 1648–1655. doi:10.1104/pp.109.138990
Park, J., Song, W. Y., Ko, D., Eom, Y., Hansen, T. H., Schiller, M., et al. (2012). The phytochelatin transporters AtABCC1 and AtABCC2 mediate tolerance to cadmium and mercury. Plant J. 69 (2), 278–288. doi:10.1111/j.1365-313X.2011.04789.x
Paul, S., Breuninger, L. M., Tew, K. D., Shen, H., and Kruh, G. D. (1996). ATP-dependent uptake of natural product cytotoxic drugs by membrane vesicles establishes MRP as a broad specificity transporter. Proc. Natl. Acad. Sci. U. S. A. 93 (14), 6929–6934. doi:10.1073/pnas.93.14.6929
Peng, H., He, X., Gao, J., Ma, H., Zhang, Z., Shen, Y., et al. (2015). Transcriptomic changes during maize roots development responsive to Cadmium (Cd) pollution using comparative RNAseq-based approach. Biochem. Biophys. Res. Commun. 464 (4), 1040–1047. doi:10.1016/j.bbrc.2015.07.064
Pérez-Salamó, I., Papdi, C., Rigó, G., Zsigmond, L., Vilela, B., Lumbreras, V., et al. (2014). The heat shock factor A4A confers salt tolerance and is regulated by oxidative stress and the mitogen-activated protein kinases MPK3 and MPK6. Plant Physiol. 165 (1), 319–334. doi:10.1104/pp.114.237891
Qiao, K., Liang, S., Wang, F., Wang, H., Hu, Z., and Chai, T. (2019). Effects of cadmium toxicity on diploid wheat (Triticumurartu) and the molecular mechanism of the cadmium response. J. Hazard. Mat. 374, 1–10. doi:10.1016/j.jhazmat.2019.04.018
Qu, T., Liu, R. F., Wang, W., An, L. Z., Chen, T., Liu, G. X., et al. (2011). Brassinosteroids regulate pectin methylesterase activity and AtPME41 expression in Arabidopsis under chilling stress. Cryobiology 63, 111–117. doi:10.1016/j.cryobiol.2011.07.003
Rasafi, T. E., Oukarroum, A., Haddioui, A., Song, H., Kwon, E. E., Bolan, N., et al. (2020). Cadmium stress in plants: A critical review of the effects, mechanisms, and tolerance strategies. Crit. Rev. Environ. Sci. Technol., 675–726. doi:10.1080/10643389.2020.1835435
Rui, H., Zhang, X., Shinwari, K. I., Zheng, L., and Shen, Z. (2018). Comparative transcriptomic analysis of two Vicia sativa L. varieties with contrasting responses to cadmium stress reveals the important role of metal transporters in cadmium tolerance. Plant Soil 423, 241–255. doi:10.1007/s11104-017-3501-9
Sadhukhan, A., Agrahari, R. K., Wu, L. J., Watanabe, T., Nakano, Y., Panda, S. K., et al. (2021). Expression genome-wide association study identifies that phosphatidylinositol-derived signalling regulates ALUMINIUM SENSITIVE3 expression under aluminium stress in the shoots of Arabidopsis thaliana. Plant Sci. 302, 110711. doi:10.1016/j.plantsci.2020.110711
Schreck, E., Foucault, Y., Sarret, G., Sobanska, S., Cécillon, L., Castrec-Rouelle, M., et al. (2012). Metal and metalloid foliar uptake by various plant species exposed to atmospheric industrial fallout: Mechanisms involved for lead. Sci. Total Environ. 427-428, 253–262. doi:10.1016/j.scitotenv.2012.03.051
Shahid, M., Dumat, C., Khalid, S., Niazi, N. K., and Antunes, P. M. C. (2017). Cadmium bioavailability, uptake, toxicity and detoxification in soil-plant system. Rev. Environ. Contam. Toxicol. 241 (8), 73–137. doi:10.1007/398_2016_8
Song, Y., Jin, L., and Wang, X. (2017). Cadmium absorption and transportation pathways in plants. Int. J. Phytoremediation 19 (2), 133–141. doi:10.1080/15226514.2016.1207598
Sui, F., Zhao, D., Zhu, H., Gong, Y., Tang, Z., Huang, X. Y., et al. (2019). Map-based cloning of a new total loss-of-function allele of OsHMA3 causes high cadmium accumulation in rice grain. J. Exp. Bot. 70 (10), 2857–2871. doi:10.1093/jxb/erz093
Tan, M., Cheng, D., Yang, Y., Zhang, G., Qin, M., Chen, J., et al. (2017). Co-expression network analysis of the transcriptomes of rice roots exposed to various cadmium stresses reveals universal cadmium-responsive genes. BMC Plant Biol. 17 (1), 194. doi:10.1186/s12870-017-1143-y
Wang, B. Q., Liu, J. F., Liu, B. W., Niu, H. H., Chen, R. H., Wang, Z. B., et al. (2017). Personal exposure to PM2.5 associated with heavy metals in four travel modes of Tianjin during the summer season. Environ. Sci. Pollut. Res. 24 (7), 6667–6678. doi:10.1007/s11356-016-8179-7
Wang, L., Qiu, T., Yue, J., Guo, N., He, Y., Han, X., et al. (2021). Arabidopsis ADF1 is regulated by MYB73 and is involved in response to salt stress affecting actin filament organization. Plant Cell Physiol. 62 (9), 1387–1395. doi:10.1093/pcp/pcab081
Xi, J., Qiu, Y. J., Du, L. Q., and Poovaiah, B. W. (2012). Plant-specific trihelix transcription factor AtGT2L interacts with calcium/calmodulin and responds to cold and salt stresses. Plant Sci. 185-186, 274–280. doi:10.1016/j.plantsci.2011.11.013
Xiong, T. T., Austruy, A., Pierart, A., Shahid, M., Schreck, E., Mombo, S., et al. (2016). Kinetic study of phytotoxicity induced by foliar lead uptake for vegetables exposed to fine particles and implications for sustainable urban agriculture. J. Environ. Sci. 46, 16–27. doi:10.1016/j.jes.2015.08.029
Yamaguchi, T., Aharon, G, S., Sottosanto, J. B., and Blumwald, E. (2005). Vacuolar Na+/H+ antiporter cation selectivity is regulated by calmodulin from within the vacuole in a Ca2+- and pH-dependent manner. Proc. Natl. Acad. Sci. U. S. A. 102 (44), 16107–16112. doi:10.1073/pnas.0504437102
Yang, L., Xu, Y. C., Zhang, R., Wang, X. T., and Yang, C. (2018). Comprehensive transcriptome profiling of soybean leaves in response to simulated acid rain. Ecotoxicol. Environ. Saf. 158, 18–27. doi:10.1016/j.ecoenv.2018.04.015
Yang, T., and Poovaiab, B. W. (2003). Calcium/calmodium-mediated signal network in plants. Trend Plant Sci. 8, 505–512. doi:10.1016/.tplants.2003.09.004
Yin, S. Y., Cui, H. R., Zhang, L., Yan, J. L., Qian, L. H., and Ruan, S. L. (2021). Transcriptome and metabolome integrated analysis of two ecotypes of tetrastigmahemsleyanum reveals candidate genes involved in chlorogenic acid accumulation. Plants (Basel) 1288, 1288. doi:10.3390/plants10071288
Zha, Y., Tang, J., and Pang, Y. (2022). The effects of simulated acid rain and cadmium-containing atmospheric fine particulate matter on the pakchoi (Brassica campestris. L.) seedlings growth and physiology. Soil. Sci. Plant Nutr. 68, 826. doi:10.1080/00380768.2021.2023826
Zhang, M., Smith, J. A. C., Harberd, N. P., and Jiang, C. F. (2016). The regulatory roles of ethylene and reactive oxygen species(ROS) in plant salt stress responses. Plant Mol. Biol. 91 (6), 651–659. doi:10.1007/s11103-016-0488-1
Zhang, N. L., Zhao, B., Fan, Z. J., Yang, D. Y., Guo, X. F., Wu, Q. F., et al. (2020). Systematic identification of genes associated with plant growth-defense tradeoffs under JA signaling in Arabidopsis. Planta 251 (2), 43. doi:10.1007/s00425-019-03335-8
Zhao, J., Connorton, J. M., Guo, Y., Li, X., Shigaki, T., Hirschi, K. D., et al. (2009). Functional studies of split Arabidopsis Ca2+/H+ exchangers. J. Biol. Chem. 284 (49), 34075–34083. doi:10.1074/jbc.M109.070235
Zhao, J. M., Xia, B., Meng, Y., Yang, Z. F., Pan, L., Zhou, M., et al. (2019). Transcriptome analysis to shed light on the molecular mechanisms of early responses to cadmium in roots and leaves of king grass (Pennisetumamericanum × P.purpureum). Int. J. Mol. Sci. 20, 2532. doi:10.3390/ijms20102532
Zheng, Y. L., Feng, Y. L., Lei, Y. B., and Yang, C. Y. (2009). Different photosynthetic responses to night chilling among twelve populations of Jatropha curcas. Photosynthetica 47, 559–566. doi:10.1007/s11099-009-0081-9
Keywords: acid rain, cadmium, flavonoid biosynthesis, metabolome, transcriptome
Citation: Zha Y, Zhao B, Qiu J and Li X (2022) Integrated transcriptomic and metabolomic analyses of pak choi [Brassica pekinensis (Lour.) Rupr] response to combined stress of acid rain and cadmium-containing atmospheric fine particulate matter. Front. Environ. Sci. 10:1020308. doi: 10.3389/fenvs.2022.1020308
Received: 16 August 2022; Accepted: 12 September 2022;
Published: 29 September 2022.
Edited by:
Tiana Carla Lopes Moreira, University of São Paulo, BrazilReviewed by:
Abhay Kumar, University of Tuscia, ItalyNasim Ahmad Yasin, University of the Punjab, Pakistan
Copyright © 2022 Zha, Zhao, Qiu and Li. This is an open-access article distributed under the terms of the Creative Commons Attribution License (CC BY). The use, distribution or reproduction in other forums is permitted, provided the original author(s) and the copyright owner(s) are credited and that the original publication in this journal is cited, in accordance with accepted academic practice. No use, distribution or reproduction is permitted which does not comply with these terms.
*Correspondence: Xuqing Li, bGl4cWluZ0Boei5jbg==