- 1São José dos Campos Institute of Science and Technology, São Paulo State University (Unesp), São José dos Campos, Brazil
- 2Laboratory of Sensors and Materials, National Institute for Space Research, São José dos Campos, Brazil
- 3JMHP Consultoria em Materiais e Informática LDTA, Research, Development and Innovation Department, Jacareí, Brazil
Introduction: Water treatment deficit and poor health, hygiene and sanitation infrastructure can contribute to disease transmission by dissemination of contaminants and microorganisms. As an alternative, carbon-based materials coated with antimicrobial molecules have been proposed for water treatment, but few supporting data are available so far. Hence, this study investigates the potential use of PAN-based activated carbon fibers (ACF) decorated with silver nanoparticles in water treatment.
Methods: Silver nanoparticles were incorporated into the material using a cheap and electroless method. Field emission scanning electron microscopy (FEGSEM), Raman spectroscopy and X-ray photoelectron spectroscopy (XPS) characterized the whole material. The textile was mounted on a water filter prototype and had its capacity to remove bacterial (Escherichia coli) and fungal (Candida albicans, Aspergillus niger and Penicillium funiculosum) cells evaluated. Composition and toxicity of the filtered water were determined.
Results: Water filtered by Ag@ACF for 2 and 24 h contained 0.254 mg/L and 0.964 mg/L Ag, respectively. Ag@ACF filtering successfully removed E. coli, C. albicans, and A. niger from the suspensions, but not P. funiculosum. Treated water was non-toxic for Vero cells and Drosophila melanogaster, but toxic for Raphidocelis subcapitata. Ag@ACF showed efficient microbial elimination when applied in water treatment. Silver nanoparticles released in aqueous medium may be responsible for R. subcapitata toxicity. Future studies should be conducted to reduce silver nanoparticles release from the carbon fiber.
1 Introduction
As a result of a combination among population growth, socio-economic development, and changing consumption patterns, the use of water has increased worldwide at a rate of approximately 1% per year since the 1980s (UNESCO, 2019). Access to water in quantity and quality is considered a basic human right and is essential to effective health protection policies (UNESCO, 2017). Despite this, water has a unique ability to transmit diseases and can contain a wide variety of constituents that can be harmful to health. Unfortunately, 30% of the world’s population does not have access to safe drinking water (UNESCO, 2019), in other word water with adequate quality for human use and consumption is becoming increasingly scarce.
Pathogenic microorganisms from infected humans and animals can enter the aquatic environment through waste or feces as a result of inadequate sanitation and can compromise water quality and health (Tran et al., 2015). UNESCO estimates that, on a global scale, more than 80% of wastewater is discharged without proper treatment into the environment, containing several microorganisms and contaminants that can cause diseases and imbalance in the ecosystem (UNESCO, 2017). In addition, 60% of the world’s population does not have access to safely managed sanitation services (UNESCO, 2019). Health, hygiene, and sanitation infrastructure conditions are factors that can influence environmental contamination by different pathogens (Silva et al., 2016) and public health, affecting the quality of life and human rights (WHO, 2019).
The assessment of bacteria indicative of fecal contamination, such as Escherichia coli and Enterococcus spp., in water bodies, is the standard methodology adopted worldwide for monitoring the microbial quality of water (WHO, 2017). The water delivery with these bioindicators can cause major threats to human health (WHO, 2017; Bianco et al., 2020), as problems of food poisoning, gastroenteritis, and urinary tract infections (Ahmed et al., 2019; Pichel et al., 2019).
Fungi can be abundant in water sources, can grow on the surface of materials in water supply systems and produce substances that result in unpleasant tastes and odors in water (WHO, 2017). For this reason, between the 2000s, the occurrence of filamentous fungi in water has been had special attention and are now are accepted as contaminants in drinking water (Hageskal et al., 2009; Oliveira et al., 2013). A study carried out by Oliveira et al. (2013) reported that Penicillium and Aspergillus were the most frequently isolated from surface water. However, to date, they are not considered in international water quality guidelines and are not monitored in water treatment facilities. It is not known how fungi develop in water, as this is not their natural habitat, but preventive measures are important and the presence of fungi in water may require adequate regulations (Hageskal et al., 2009).
Disinfection generally constitutes the final step of drinking water treatment and aims to eliminate pathogenic microorganisms causative of waterborne diseases, using methods such as ultraviolet radiation (UV), filtration, pasteurization, and chemical methods such as chlorination, chloramination, treatment with chlorine dioxide and ozonation. Chlorination is the most frequently used method (Pichel et al., 2019). However, these methods have limitations, such as efficiency, cost, product demand, and toxicity (Pichel et al., 2019). Disinfection effectiveness can also be affected by the presence of pathogens encased in flakes or particles, protecting them from the action of disinfectants (WHO, 2017). Considering that water is an indispensable resource for life, the interest of private and public sectors on these issues is very high, motivating the development of alternative and innovative methods for water treatment (WHO, 2017).
Within this context, the activated carbon fiber (ACF) based on textile polyacrylonitrile polymer (PAN) is a fiber intended for the production of clothing and fabrics in general and represents a low-cost raw material with the potential for transformation into an activated fiber with a high surface area, a high fraction of micropores (Marcuzzo 2016) and high strength (Lee et al., 2014). ACF-PAN textile is a product of varied applicability, with fibers that have a microporous structure, giving it faster and more selective adsorption kinetics to molecular contaminants, standing out in the removal of water pollutants (Marcuzzo, 2016; Lee et al., 2014).
The high surface area and micropore fraction make the material interesting for the adsorptive function, which favors the adhesion of fungi and bacteria. However, microbial proliferation due to adhesion can occur in polymer fiber mesh (Abrigo et al., 2015) and ACF felts (Yoon et al., 2008), which may represent a long-term disadvantage. Thus, antibacterial and antifungal coating agents, such as silver (Park and Jang, 2003), may be advantageous. Silver (Ag) is an effective broad-spectrum antimicrobial agent, capable of causing cell damage and oxidative stress. In addition, it has long-term stability and activity that advantageously assists in the application of coatings for medical devices, bone prostheses, dental compounds and water and air filters (Chaloupka et al., 2010). Studies have shown that detachment of Ag from commercial products to wastewater and the aquatic environment may occur, raising concerns about possible effects of silver on biota (Benn et al., 2010; Lorenz et al., 2012; Kalantzi et al., 2019).
Thus, this study aimed to evaluate the potential application of activated carbon felt produced from PAN and decorated with silver nanoparticles for water treatment, by assessing the microbial retention efficiency and toxicity of the filtered water.
2 Materials and methods
2.1 Synthesis of activated carbon fiber (ACF)
Activated carbon fibers were produced from oxidized PAN textile fiber felts carbonized in an argon atmosphere at 900°C for 20 min and activated in a CO2 plus water vapor atmosphere at 1000°C for 2 h. Electroless plating was performed by submerging part of the ACF in a solution containing silver nitrate (AgNO3) at a ratio of 2 g of metal/L in deionized water (DI) for 24 h, then washed with deionized water and dried in a vacuum oven at 100°C for 4 h for AgNPs incorporation on the felt surface (Ag@ACF) (Rodrigues et al., 2018).
2.2 Characterization of activated carbon fiber (ACF)
Samples underwent chemical and morphological characterization. Field emission scanning electron microscopy (FEG-SEM) was performed using a TESCAN MIRA3; Raman spectroscopy using a Horiba Labram Hr Evolution, with a 514.6 nm laser; and X-ray photoelectron spectroscopy (XPS) using a Kratos Axis Ultra XPS analyzer, with monochromatic Al-Kα X-ray radiation (1486.5 eV). Vacuum was stabilized at 10–7 Pa. Mean diameter of Ag particles present on the Ag@ACF surface was determined by analyzing 30 particles based on Ag@ACF FEG-SEM sample images using Image J software (United States, version 1.53a).
2.3 Filtration unit
Antimicrobial action was analyzed using a PVC filter unit, made according to the schematic design shown in Figure 1. Inner area consisted of a perforated tube coated with an Ag@ACF sample (area 3 cm × 15 cm). The outer side comprised a protective casing connected to a hydraulic pump that allows water recirculation at a flow rate of approximately 3.33 L/min.
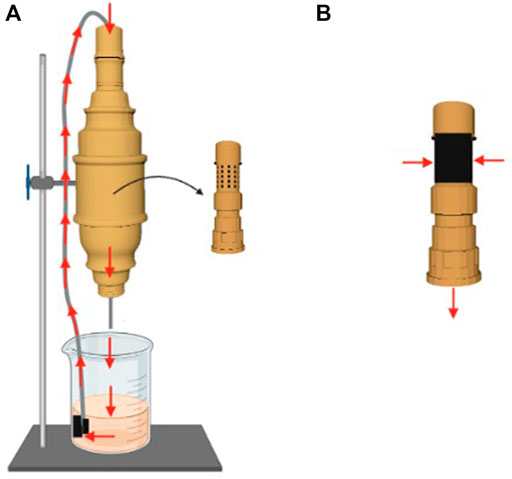
FIGURE 1. Schematic drawing of the filtering unit structure used in the experiments. (A) Illustration of the components and water flow; (B) Ag@ACF-coated inner casing.
2.4 Detection of chemical elements in water sample filtered by Ag@ACF
Water filtered by Ag@ACF for 2 and 24 h was analyzed via ICP Optima 7300 DV Spectrometer to verify and quantify the presence of chemical elements using 188–670 nm wavelengths. 0.5 M nitric acid was added to the treated water for sample conservation. Presence of chemical elements in the solution was compared with the concentration established by the WHO’s Guidelines for Drinking Water Quality (2017) and CONAMA—National Council for the Environment and Resolution, 2005 Distilled water was used as a negative control.
2.5 Evaluation of Ag@ACF microbial retention efficiency
Reference strains of Escherichia coli (ATCC 10799) and Candida albicans fungi (ATCC 18804), Aspergillus niger (CCIBT 2261), and Penicillium funiculosum (ATCC 8725) were used. For E. coli and C. albicans, the strains were plated on Brain Heart Infusion (BHI) agar and Sabouraud dextrose (SD) agar, respectively, and incubated at 37°C for 24 h. Then, spectrophotometrically standardized suspensions in saline solution (0.9% NaCl) containing 109 cells/ml (λ = 530 nm and OD = 1.306) for E. coli and 106 cells/ml (λ = 530 nm and OD = 0.380) for C. albicans were diluted in BHI broth to a concentration of 107 cells/ml for bacteria and 105 cells/ml in SD broth for fungal species.
Suspensions were then filtered separately through a sterile Ag@ACF mounted on the filtration unit. Samples were analyzed at 15, 30, 45, 60, 120 min, and 24 h after filtration. After serial dilution, filtrates were plated onto specific culture media (BHI agar for E. coli and SD agar for C. albicans), and the number of colony forming units per milliliter (CFU/mL) was determined after 24-h incubation at 37°C. All tests were performed in triplicate.
Filamentous fungi were analyzed according to Cronin et al. (2014). Standardized suspensions of 105 spores/ml in RPMI 1640 broth and 104 spores/mL in SD broth for A. niger and P. funiculosum, respectively, were obtained in a Neubauer chamber. Ag@ACF filtration occurred for 2 h. Samples were collected every 30 min and quantified in a Neubauer chamber to verify the number of spores/mL. We plated 20 µl of A. niger and P. funiculosum solutions onto potato and SD agar, respectively. Plates were incubated at 30°C for 7 days, for visual analysis of fungal growth regarding hyphal expansion and pigmentation variation. All tests were performed in triplicate.
2.6 Field emission scanning electron microscopy (FEG-SEM) of Ag@ACF after filtration
After final filtration of the contaminated solution, Ag@ACF samples were fixed with glutaraldehyde for 24 h, followed by dehydration at increasing ethanol concentrations for 1 min, and then analyzed by field emission scanning electron microscopy to visualize the adhesion and morphology of the studied microorganisms.
2.7 Toxicity analysis of Ag@ACF-filtered water samples
Ag@ACF-filtered water toxicity was evaluated using three experimental models: cytotoxicity for Vero cells, and ecotoxicity for Drosophila melanogaster and Raphidocelis subcapitata.
2.7.1 Cytotoxicity evaluation for Vero cells
Following ISO 10993-5 methodology, 200 µl of a suspension containing 104 Vero cells (ECACC 84113001) were seeded into a 96-well plate and exposed to Dulbecco’s modified Eagle’s medium (DMEM) enriched with 10% inactivated fetal bovine serum (FBS) and supplemented with penicillin/streptomycin 1% Ag@ACF-treated medium. For analysis, the DMEM medium was filtered through Ag@ACF for 2 and 24 h. Cell viability was determined 24 and 48 h after exposure to Ag@ACF-treated medium (2 and 24 h) using MTT assay (3-4,5-dimethylthiazol-2yl-2,5-diphenyltetrazolium; Sigma, St Louis, United States) at 570 nm (EL808IU, Biotek, Vermont, United States). Two separate experiments were performed in triplicate. Results were expressed as percent viable cells (%), using the number of cells grown in wells without exposure to Ag@ACF-treated DMEM medium as a negative control.
Cell viability percentages were classified as: 0 (non-cytotoxic), no reduction in cell growth; 1 (mild), only slight observable growth inhibition; 2 (mild), no more than 50% growth inhibition; 3 (moderate), more than 50% observable growth inhibition; 4 (severe), almost complete or complete destruction of cell layers. Negative control was set as 100% cell viability (ISO 10993).
2.7.2 Toxicity evaluation for Drosophila melanogaster
Evaluation of treated water toxicity for the D. melanogaster model was performed according to Sampaio et al. (2018) using 4–10-day old wild type (WT) Canton-s females. Distilled water treated by Ag@ACF for 2 and 24 h was used to prepare the base medium for fly food, replacing the distilled water making up the original base medium. For the analysis, 80 flies were randomly divided into four independent experimental groups and previously stored in tubes for 6 h without food. They were then transferred to tubes with medium containing Ag@ACF-treated water (treatment group) and untreated water (control group). Ecotoxicity was analyzed for eight consecutive days, checking the number of survivors to obtain a survival curve.
2.7.3 Toxicity evaluation for Raphidocelis subcapitata
Chlorophyceae R. subcapitata was cultivated, in an exponential growth phase, on a sterile L.C Oligo culture medium (AFNOR—Association Française de Normalisation, 1980) without ethylenediaminetetraacetic acid (EDTA) inside Erlenmeyer flasks. The culture was kept under illumination (with a 12/12-h photoperiod) of 80 μmol m−2 s−1 and controlled temperatures at 25°C ± 2°C (ABNT Brazilian Association of Technical Standards, 2011). Algae cells were collected by centrifugation and counted in a Neubauer chamber. Treatment was performed at the following concentrations: a) 100% culture medium (control), b) 50% treated water and 50% culture medium, and c) 100% treated water. All treatments used a cell density of 5 × 104 cells/ml, in a final volume of 100 ml, stored in a polycarbonate Erlenmeyer flask. Performed in triplicate and under aseptic conditions, the experiments were conducted in 96 h under the same photoperiod, light intensity and temperature conditions used to maintain the microalgae. We extracted 2 ml aliquots of the samples at the beginning and end of the experiments. An optical microscope evaluated algal density by cell counting in a Neubauer chamber at 400× magnification. Microalgae growth rate in the different treatments was calculated according to Fogg (1975). The toxic effect referred to the reduction in algal growth rate values after treatment with Ag@ACF-treated water in the filtration unit, compared to that obtained in the control (L.C. Oligo medium).
2.8 Statistical analysis
Statistical analysis was performed via GraphPad Prism 7.0 and Toxstat 3.3 software. Normality tests were performed. Ag@ACF filtering potential data relative to baseline (t = 0) and scores obtained at each evaluation period were compared using the Kruskal–Wallis test, followed by Dunn’s post-hoc multiple comparison test for E. coli, C. albicans, A. niger, and P. funiculosum. Toxicity results from the D. melanogaster model were evaluated by a log-rank test (Mantel-Cox) to compare the survival curve plotted via Kaplan-Meier test. Toxicity for R. supcaptata was evaluated by ANOVA, followed by Tukey’s post-hoc test. Significance level was set at 5%.
3 Results
3.1 Material characterization
3.1.1 Field emission scanning electron microscopy (FEG-SEM)
Both ACF and Ag@ACF samples analyzed by FEG-SEM showed similar morphologies: massive needled felt with grooves all over their surface, probably from their manufacturing process and porosity (Figure 2) (Rodrigues et al., 2018). We can also observe that ACF has a slightly rough and intact surface with no apparent defects. In the Ag@ACF sample, we can see white dots scattered throughout the filaments, possibly related to Ag deposition. Ag deposition did not alter the morphology of the felt filaments, and presented a non-uniform particulate distribution and mean particle diameter of 121 ± 0.062 nm.
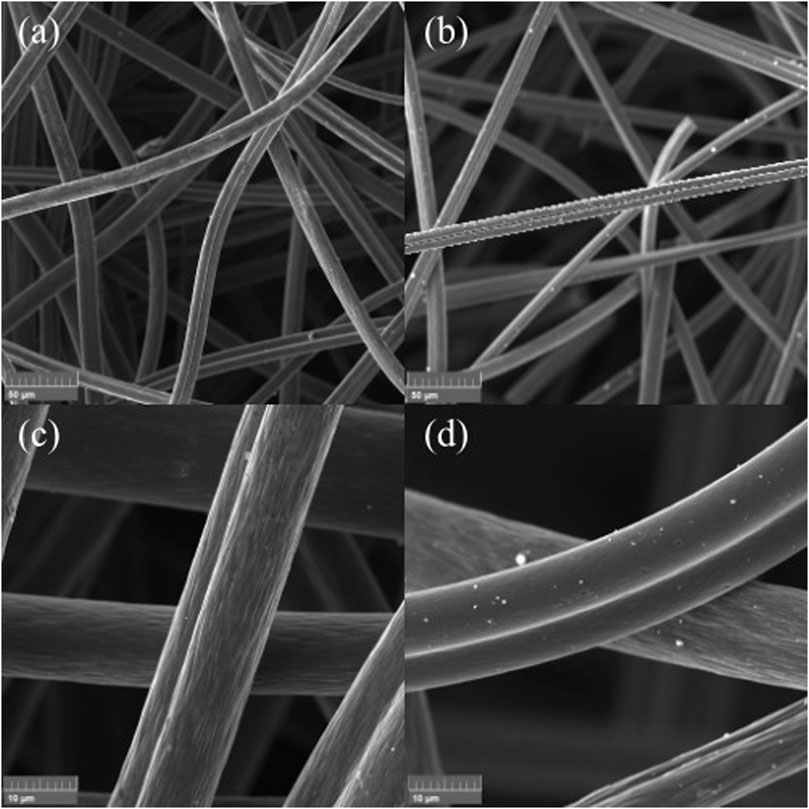
FIGURE 2. ACF and Ag@ACF FEG-SEM micrographs: (A) ACF at 1 kx magnification; (B) Ag@ACF at 1kx magnification; (C) ACF at 5kx magnification; (D) Ag@ACF at 5kx magnification.
3.1.2 Raman spectroscopy
Regarding the Raman shift spectra of both felt samples, we observed two bands characteristic of amorphous carbon materials: the D band at approximately 1350 cm−1 and the G band at approximately 1600 cm−1 (Figure 3A). These bands provide information about the degree of disorder of the material and possible defects in its carbon structure (D band), as well as about its degree of graphitization (G band) (Cançado et al., 2004; Claramunt et al., 2005).
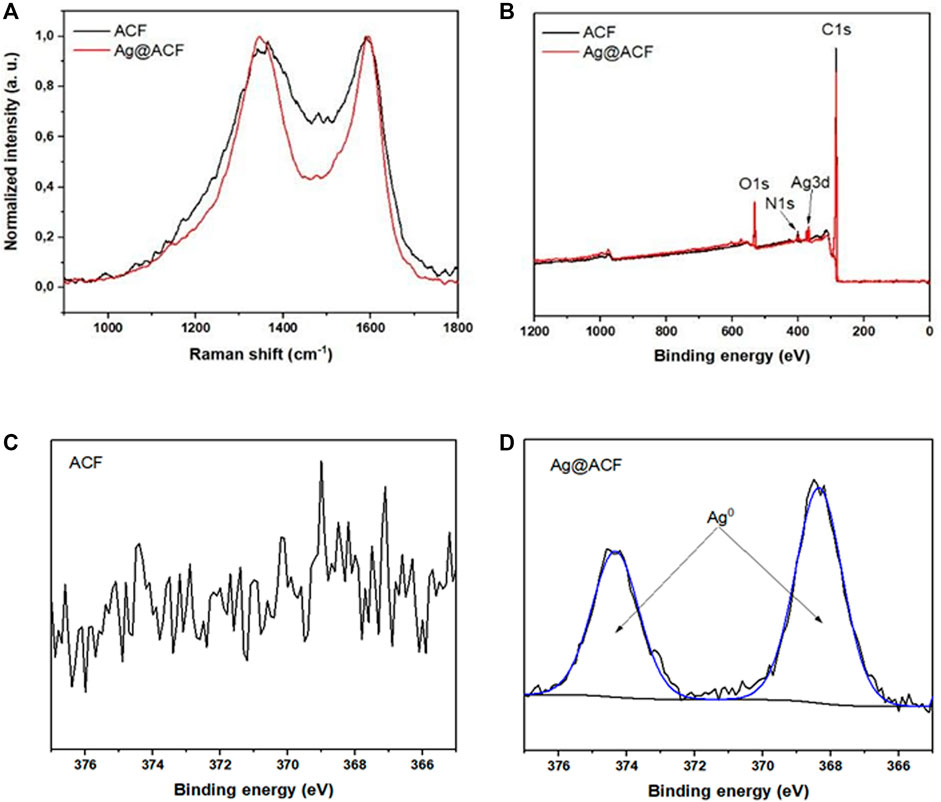
FIGURE 3. Spectral analyses: (A) Raman spectrum of ACF and Ag@ACF; (B) XPS spectra of ACF and Ag@ACF; (C) High resolution spectrum of ACF; and (D) Ag@ACF samples for Ag3d.
3.1.3 X-ray photoelectron spectroscopy (XPS)
We performed XPS analysis and high-resolution spectra. Figure 3B shows the elements present on the surface of the samples, from which we quantified the concentrations for each element identified (Table 1). After spontaneous deposition of Ag, XPS identified this element on the surface of the Ag@ACF sample, in addition to oxygenated functional groups. Figures 3C, D show the high-resolution spectra of Ag present in ACF and Ag@ACF, respectively. In case of deposition, Ag0 or metallic silver was identified only in the Ag@ACF sample (Tjeng et al., 1990). According to the XPS spectra, we can observe C-OH and/or C-O-C functional groups on the carbon felt surface, which act as the main active sites for deposition of silver nanocrystals. Thus, Ag+ ions (from the aqueous AgNO3 solution) are perhaps electrostatically adsorbed onto the carbon fiber surface and then reduced by these oxygenated functional groups (Vanitha et al., 2015).
3.2 Detection of chemical elements in the water sample filtered by Ag@ACF
According to spectrophotometric analysis, silver (Ag) showed the highest concentrations (0.25 mg/L–0.96 mg/L at 2 h and 24 h, respectively). Important for R. subcapitata development, we also analyzed copper (Cu) (0.02–0.04 mg/L at 2 and 24 h, respectively), zinc (Zn) (0.11–0.09 mg/L at 2 and 24 h, respectively), phosphorus (P) (0 mg/L for both times). Supplementary Table S1 summarizes all the analyzed elements.
3.3 Evaluation of Ag@ACF microbial retention efficiency
We investigated the microbial reduction by Ag@ACF filtration of suspensions containing E. coli, C. albicans, A. niger and P. funiculosum. Figure 4 shows the results of microbial counts after Ag@ACF filtration from 15 min to 24 h of treatment. Its capacity to reduce E. coli, C. albicans, and A. niger counts was time dependent. Supplementary Table S2 presents the mean, standard deviation and median of the antimicrobial effect of Ag@ACF.
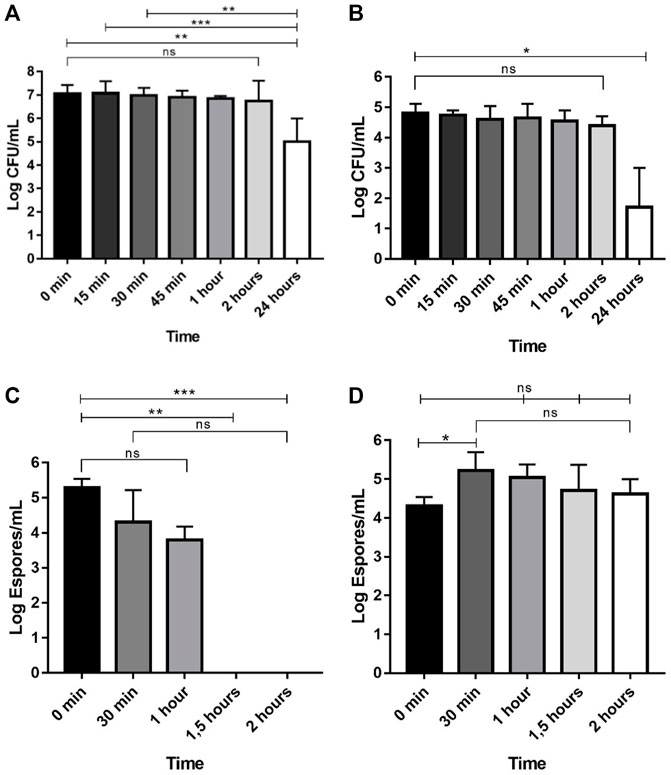
FIGURE 4. Antimicrobial activity of (A) E. coli; (B) C. albicans; (C) A. niger; (D) P. funiculosum after treatment with Ag@ACF filtration at different times. The graphs show the median and range data. Data were analyzed by ANOVA using the Kruskal–Wallis test, followed by Dunn’s post-hoc multiple comparison test. (n.s = not significant; *p < 0.05; **p < 0.01; ***p < 0.001).
We identified a significant reduction de 97.8% in E. coli counts after a 24 h (p = 0.0012) filtration compared to control (0 h) (Figure 4A). However, reduction was observed between 30 min (p = 0.0004) and 1 h (p = 0.0076) when compared 24 h of filtration with 22.3 and 40.4% of bacterial reduction, respectively. C. albicans counts showed a significant reduction after 24 h (100% reduction) of treatment when compared to the control (p = 0.0290) group (Figure 4B). Similarly, A. niger presented significant reduction at 1.5 (p = 0.0022; 99.4% of reduction) and 2 h (p = 0.0006; 99.8% of reduction) compared to control (Figure 4C). For P. funiculosum (Figure 4D), significant difference was observed only after 30 min (p = 0.0123), however there was no reduction observed in filtration process.
We evaluated the effect of Ag@ACF filtration on A. niger and P. funiculosum suspensions by visual analysis (Figure 5). Fungal growth showed reduction between 30 min and 2 h for A. niger, but a similar effect was not observed for P. funiculosum. These results are in agreement with the statistical analysis. Moreover, during P. funiculosum growth, we observed variations in the pink pigmentation of the colonies, with yellow halos and flattening of the central area (black and gray arrows). The white and, filamentous outer margin showed a reduction in that edge over time, demonstrating changes in colony expansion (yellow arrow).
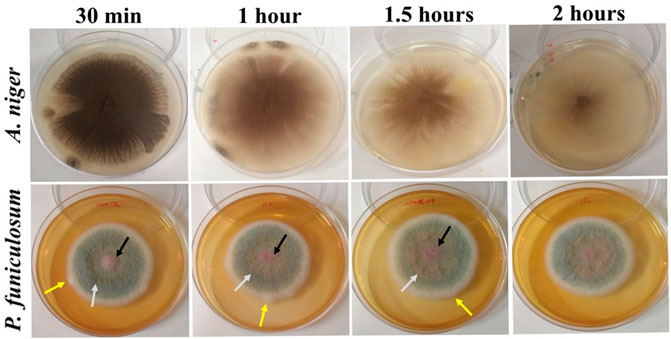
FIGURE 5. Fungal growth after treatment with Ag@ACF filtration from 30 min to 2 h. Ag@ACF filtration showed a strong antifungal effect for A. niger, with effective growth reduction over time. P. funiculosum had a slight reduction effect with a pink central area and diffuse high to flat growth (black and gray arrows) over treatment time and a slight growth reduction of the outer margin (yellow arrow).
3.4 Field emission scanning electron microscopy (FEG-SEM) of Ag@ACF after filtering
Micrographs of the Ag@ACF sample after final filtration of the E. coli, A. niger, and P. funiculosum suspensions were taken. Figure 6A shows the arrangement of the carbon fibers in the textile. Figure 6C shows E. coli cells retained on the felt without morphological changes, whereas Figure 6D shows unaltered bacterial cells. Figure 6B shows a matrix possibly formed by the reaction between the carbon fibers and the culture medium, resulting in a layer of organic matter, which may have prevented the visualization of Ag particles.
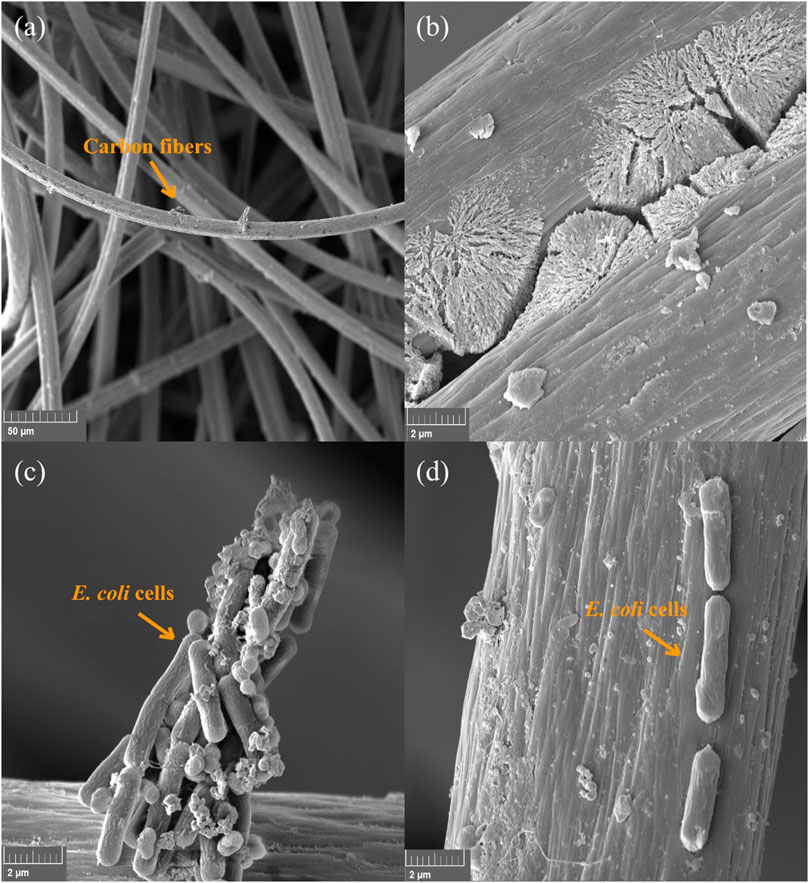
FIGURE 6. FEG-SEM micrographs at 1kx and 20kx magnification. In (A) Carbon fibers (1.00 kx); (B) Matrix possibly formed by the reaction between the carbon fibers and the culture medium (20.0 kx); (C) E. coli cells without morphological changes (20.0 kx); (D) E. coli cells retained unchanged in the formed matrix (20.0 kx).
3.5 Toxicity analysis of Ag@ACF-filtred water samples
3.5.1 Cytotoxicity evaluation for Vero cells
Cell viability after 24 h of exposure in DMEM medium treated with Ag@ACF for 2 h was classified as grade 0 (non-cytotoxic) and in DMEM medium treated with Ag@ACF for 24 h as grade 2 (mild). After 48 h of exposure at both treatment times, cell viability was classified as grade 1 (mild) with 87% viability when exposed to the 2-h treatment and 85% viability when exposed to the 24-h treatment (Figure 7).
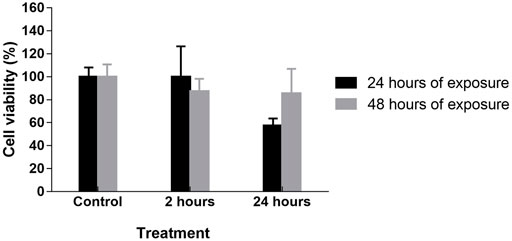
FIGURE 7. Analysis of Ag@ACF cytotoxicity expressed by cell viability percentage of Vero cells, DMEM treated for 2 and 24 h. Cytotoxicity assessed by MTT assay and cell viability evaluation verified after 24 and 48 h of exposure in Ag@ACF-treated DMEM medium.
3.5.2 Toxicity evaluation for Drosophila melanogaster
D. melanogaster flies fed water filtered through Ag@ACF for 2 (Figure 8A) and 24 h (Figure 8B) showed survival rates of 96.67% and 100%, respectively, allowing to classify the water sample as non-toxic.
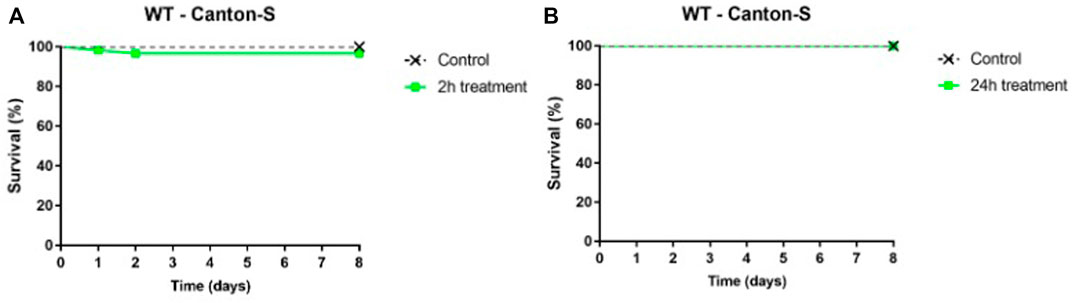
FIGURE 8. Kaplan-Meier survival curves of WT–Canton-S flies fed a medium containing Ag@ACF-treated water for (A) 2 and (B) 24 h and standard culture medium (control). Dashed line represents the longevity of the group fed a medium containing Ag@ACF-treated water (green) and negative control (standard medium) (black). n = 20 flies/group.
3.5.3 Toxicity evaluation for Raphidocelis subcapitata
Within 2 h of treatment, the mean algal growth rate obtained at 50% (-0.02 days−1) and 100% (0.10 days−1) were significantly lower (p < 0.05) compared to that obtained for the control group (0.59 days−1) (Figure 9A). We observed a similar effect for the 24-h treatment, where the mean values of microalgae growth rate at 50% and 100% (−0.05 days−1 and 0.04 days−1, respectively) were significantly lower (p < 0.05) that recorded in the control treatment (Figure 9B).
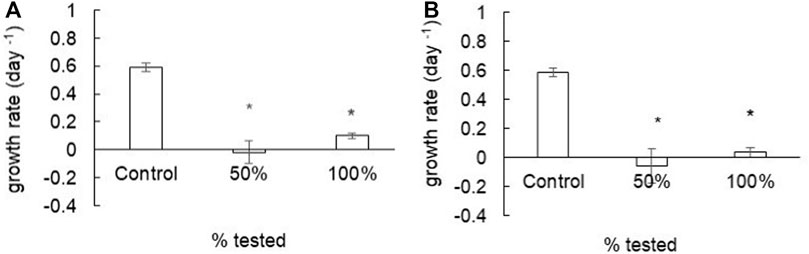
FIGURE 9. Mean algal growth rate after treatment with Ag@ACF-treated water. In (A) water treated for 2 h and (B) water treated for 24 h. Data were analyzed using ANOVA, followed by Tukey’s post-hoc test (*p < 0.05).
4 Discussion
This study incorporated Ag particles into PAN-based activated carbon nanofibers to improve particle adsorption and microbial reduction. The analyses performed showed an heterogeneous Ag impregnation distribution along the fibers, which may have positively influenced the antimicrobial activity, since the NPs dispersed on the material’s surface might be more accessible to microorganisms (Khare et al., 2014; Arakawa et al., 2019).
Ag@ACF filtration of microbial suspension for 24 h showed time-dependent reduction in E. coli and C. albicans with microbial reduction of 97.8% and 100%, respectively. A. niger fungal filtration showed a 99.4 and 99.8% reduction after 1.5 and 2 h filtration. No growth inhibition was detected for P. funiculosum over the analyzed times. A similar result was observed in a study carried out by Fujioka et al. (2019) which evaluated the removal of an industrial scale sand filter in a drinking water treatment plant and observed a microbial reduction of 95.2%–99.3%. Similarly, a filtration of E. coli suspension with a ceramic filter in the absence and presence of colloidal silver obtained 97.8 and 100% reduction, respectively (Oyanedel-Craver and Smith, 2008).
Our study aimed at the impregnation of silver in ACF as an antimicrobial material during the filtration process. Silver nanoparticles have demonstrated high antimicrobial efficacy against different microbial species (Cavassin et al., 2015) and demonstrated action in altering efflux pumps, alteration, and accumulation in membranes, causing rupture and leakage of intracellular content, in addition to internal damage when in contact with the genetic material (DNA) (Bruna et al., 2021). However, the antimicrobial and cytotoxic action of AgNP may be dependent on the nanoparticle size (Jeong et al., 2014). Antimicrobial activity in different sizes of Ag nanoparticles was also observed by Oya et al. (1996) on activated carbon fibers containing 100–200 nm Ag particles for water filtration. Zhang et al. (2015) observed antimicrobial activity on multiple species, such as S. aureus, E. coli, and C. albicans of activated carbon fiber impregnated with Ag particles ranging from 20–40 nm. Bacterial inhibition of E. coli was observed in ACF covered by different concentrations of Ag and average particles ranging from 12.13 to 15.52 nm (Yoon et al., 2008). Inhibitory effect was also observed for A. niger after contact with nanocomposite films dispersed with AgNPs with a mean diameter of 8.6 nm (Pinto et al., 2013). No studies have been found so far that have studied the contact between P. funiculosum and AgNP.
Although Ag@ACF filtration was ineffective in inhibiting P. funiculosum, we observed colony morphological changes with variation in colony pigmentation and outer margin, suggesting possible Ag@ACF-related alterations. We found no previous research on the Ag mechanism of action on P. funiculosum and its possible consequences on pigmentation. Future studies are needed to verify its causes and other effects on the fungus phenotype.
Its potential application in water treatment was also evaluated by toxicity tests using different in vitro and in vivo models representative of different biological complexities. First, we performed cytotoxicity tests on Vero cells, widely applied to evaluate the effects of chemicals and toxins (Ammerman et al., 2008). Next on D. melanogaster, a fast-living, reliable, robust, economically viable model organism that has been successfully studied to investigate the toxicity of nanomaterials, including AgNPs (Alaraby et al., 2015). And lastly on R. subcapitata, a primary producer present in freshwater and a reference species for studying metal toxicity (Expósito et al., 2017; Kleiven et al., 2019).
Water filtered for 24 and 48 h were non-cytotoxic and slightly cytotoxic for Vero cells, respectively. Pannerselvam et al., 2021 obtained an IC50 of 20 mg/L for AgNPs (70–90 nm) and IC50 of 5 mg/L for AgNO3. Quintero-Quiroz et al. (2019) reported a median lethal concentration (LC50) of 19.11 mg/L after a 24-h exposure of Vero and NH3T3 cells to AgNPs (diameter ∼10.30 nm). These authors observed viability above 80% at concentrations below 5 μg/ml. Thus, it appears that IC50 and LC50 obtained with smaller sized AgNPs are higher than the concentrations of 0.25 and 0.96 mg/L evaluated in the present study. Differences in concentrations and toxic effects may be related to different AgNPs preparation methods, particle size, and exposure time (Saleh et al., 2020).
Water samples filtered for 2 and 24 h by Ag@ACF showed no toxic effect for D. melanogaster. Previous studies reported D. melanogaster toxicity only at higher concentrations than those used in the present study. Acute toxic effect on flies occurred at a concentration of 20 mg/L (29 ± 4 nm), at which 50% of the flies tested showed developmental difficulties during pupae release, did not complete the development cycle, and presented changes in body pigmentation (Panacek et al., 2011). At the concentration of 100 mg/L, 97% of the larvae died and no pupae formed. However, Panacek et al., 2011 prepared AgNPs via microencapsulation using mannitol as the encapsulating agent, which may have increased its ingestion by flies. Similar result was reported by Ahamed et al. (2010), in which AgNPs at 50–100 mg/L (10.91 ± 4.58 nm) induced the heat shock protein 70 expression, oxidative stress and apoptosis in D. melanogaster cells.
Results showed a toxic effect for R. subcapitata with significant reduction in the average growth rate by 50% and 100%. Among the chemical elements detected in the treated water analysis, Cu, Fe, Mg, P, Se, and Zn can be considered nutrients for R. subcapitata, depending on the concentration. In concentrations above those considered essential, however, these elements can be toxic for the organism. According to the literature, the 96-h EC50 value of Cu for R. subcapitata is between 0.071 and 0.137 mg/L (Oliveira-Filho et al., 2004), 96-h EC50 of Zn for the species is 0.179 mg/L (Rodgher et al., 2020), and the limits of trophic state levels for P can range from 0.306 to 77.02 mg/L (eutrophic or hypereutrophic environment) (Tito and Luna, 2020; Cunha et al., 2021). The concentrations of the chemical elements detected in the samples were below those considered toxic to microalgae; thus, even with the presence of elements that favor microalgae development, mainly P, the presence of Ag at concentrations above the tolerable level would result in a toxic effect.
Wang et al. (2012) evaluated the toxicity of three commercial AgNPs and AgNO3 materials in different aquatic organisms including R. subcapitata and obtained EC50 values of 0.29 µM, 8.31 µM, 37.14 µM and 196.55 µM in tests with AgNO3 (free ions), DIS-nAg (dispersant-prepared AgNPs–15 nm), PVP-nAg (coated AgNPs–80 nm) and Bare-nAg (uncoated AgNPs–35 nm), respectively. These results pointed to an inverse correlation between particle size and its toxic effect, showing that the smaller the particle size, the greater the observed toxic effect. Moreover, both AgNPs and Ag ions affect organisms, with the latter having the greatest effect. Kleiven et al., 2019 observed similar effects, obtaining greater growth reduction for R. subcapitata on exposure to 0.008 and 0.025 mg/L AgNO3. These studies show that both metallic and ionic Ag act on organisms; however, Ag speciation is considered more relevant for toxicity than total metal concentration (Köser et al., 2017). Research also indicates that Ag ions contribute greatly to the toxicity of R. subcapitata, which may be caused by the high ion accumulation capacity of microalgae, since the bioconcentration of ions in phytoplankton is higher than in zooplankton or fish (Fortin et al., 2001).
Despite the promising antimicrobial activity, ICP spectrometer analysis of the Ag@ACF-treated water (Supplementary Table S1) identified a limitation in the study regarding Ag deposition above the limit values established by WHO (0.1 mg/L) and CONAMA (0.01 mg/L), suggesting Ag detachment from the surface of the material. Since we used the filter unit for dynamic filtration with continuous circulation from 2 to 24 h, this movement may have contributed to greater Ag detachment and, consequently, to the toxicity of R. subcapitata.
Hence, new methods to impregnate Ag into felt should be analyzed in future studies to mitigate this limitation. Future evaluation of different methods aimed at improving the characteristics, effect and availability of Ag to pathogenic microorganisms should be conducted to improve the material and reduce its metal release. AgNPs optimized by chemical reduction (Quintero-Quiroz et al., 2019) and by variations in physicochemical characteristics (Pannerselvam et al., 2021) showed good antimicrobial effect and are potential alternatives to be considered.
5 Conclusion
Silver nanoparticles were successfully incorporated into textile carbon fibers using a cheap and electroless method.
The production of textile PAN is five times less expensive than special acrylic fiber and for this reason, it could be a cheaper option.
Microbial suspensions filtered for 24 h through textile carbon fibers decorated with nanoparticles of Ag (Ag@ACF) showed time-dependent reduction for E. coli and C. albicans, with reductions of 97.8% and 100%, respectively. A. niger fungal suspension filtered with Ag@ACF showed a 99.4 and 99.8% cell count reduction after 1.5 and 2 h. Filtration by Ag@ACF did not inhibited P. funiculosum suspension after 1.5 and 2 h filtration. Water filtered with Ag@ACF for 24 and 48 h were non-cytotoxic and slightly cytotoxic for Vero cells, respectively.
Water samples filtered for 2 and 24 h with Ag@ACF showed no toxic effect for D. melanogaster, although showed some degree of toxicity was observed for R. subcapitata. Textile carbon fibers decorated with nanoparticles of Ag (Ag@ACF) is a promising material for water treatment.
Data availability statement
The original contributions presented in the study are included in the article/Supplementary Material, further inquiries can be directed to the corresponding author.
Author contributions
IM: conceptualization, methodology, investigation, resources, formal analysis, writing - original draft. AS: methodology, investigation, resources, formal analysis, writing - original draft. GL: conceptualization, methodology, investigation, resources. MC: methodology, investigation, resources, formal analysis. SR: methodology, investigation, resources, formal analysis. AR-S: methodology, investigation, resources. MB: methodology, investigation, resources. JM: methodology, investigation, resources. CK-I: methodology, conceptualization, supervision, writing review and editing.
Funding
This study was supported by the Brazilian National Council for Scientific and Technological Development (CNPq) with scientific initiation fellowship (IMM) and Research fellowship CNPq 309762/2021-9 (CKI). Also by Coordination for the Improvement of Higher Education (CAPES)—Finance Code 001 (ACRS).
Conflict of interest
Author JM was employed by company JMHP Consultoria em Materiais e Informática LDTA.
The remaining authors declare that the research was conducted in the absence of any commercial or financial relationships that could be construed as a potential conflict of interest.
Publisher’s note
All claims expressed in this article are solely those of the authors and do not necessarily represent those of their affiliated organizations, or those of the publisher, the editors and the reviewers. Any product that may be evaluated in this article, or claim that may be made by its manufacturer, is not guaranteed or endorsed by the publisher.
Supplementary material
The Supplementary Material for this article can be found online at: https://www.frontiersin.org/articles/10.3389/fenvs.2022.1100583/full#supplementary-material
References
ABNT - Brazilian Association of Technical Standards (2011). Chronic toxicity. Test method with algae (Chlorophyceae). (Associação Brasileira de Normas Técnicas. Toxicidade crônica. Método de ensaio com algas (Chlorophyceae)). Rio de Janeiro, Brazil: ABNT NBR 12648.
Abrigo, M., Kingshott, P., and McArthur, S. L. (2015). Electrospun polystyrene fiber diameter influencing bacterial attachment, proliferation, and growth. ACS Appl. Mater. Interfaces 7, 7644–7652. doi:10.1021/acsami.5b00453
AFNOR - Association Française de Normalisation (1980). Water tests. Experimental standard T90-304. Determination of the inhibition of growth of Scenedesmus subspicatus by a substance. Paris: AFNOR. (French Association for Standardization. Essais dês eause. Norme experimentale T90-304. Determination de I’inhibition de Scenesdesmus subspicatus croissance par une substance).
Ahamed, M., Posgai, R., Gorey, T. J., Nielsen, M., Hussain, S. M., and Rowe, J. J. (2010). Silver nanoparticles induced heat shock protein 70, oxidative stress and apoptosis in Drosophila melanogaster. Toxicol. Appl. Pharmacol. 242, 263–269. doi:10.1016/j.taap.2009.10.016
Ahmed, W., Hamilton, K., Toze, S., Cook, S., and Page, D. (2019). A review on microbial contaminants in stormwater runoff and outfalls: Potential health risks and mitigation strategies. Sci. Total Environ. 692, 1304–1321. doi:10.1016/j.scitotenv.2019.07.055
Alaraby, M., Annangi, B., Hernández, A., Creus, A., and Marcos, R. (2015). A comprehensive study of the harmful effects of ZnO nanoparticles using Drosophila melanogaster as an in vivo model. J. Hazard. Mater. 296, 166–174. doi:10.1016/j.jhazmat.2015.04.053
Ammerman, N. C., Beier-Sexton, M., and Azad, A. F. (2008). Growth and maintenance of Vero cell lines. Curr. Protoc. Microbiol. 11, Appendix 4E. doi:10.1002/9780471729259.mca04es11
Arakawa, F. S., Shimabuku-Biadola, Q. L., de Lima Bazana, S., Silva, M. F., de Abreu Filho, B. A., and Bergamasco, R. (2019). Activated carbon impregnation with Ag and Cu composed nanoparticles for Escherichia coli contaminated water treatment. Can. J. Chem. Eng. 97, 2408–2418. doi:10.1002/cjce.23471
Benn, T., Cavanagh, B., Hristovski, K., Posner, J. D., and Westerhoff, P. (2010). The release of nanosilver from consumer products used in the home. J. Environ. Qual. 39, 1875–1882. doi:10.2134/jeq2009.0363
Bianco, K., Albano, R. M., de Oliveira, S. S. A., Nascimento, A. P. A., dos Santos, T., and Clementino, M. M. (2020). Possible health impacts due to animal and human fecal pollution in water intended for drinking water supply of Rio de Janeiro, Brazil. J. Water Supply Res. Technol. - AQUA 69, 70–84. doi:10.2166/aqua.2019.061
Bruna, T., Maldonado-Bravo, F., Jara, P., and Caro, N. (2021)., Silver nanoparticles and their antibacterial applications. Int. J. Mol. Sci. 22. PMCID, 7202PMC8268496. PMID: 34281254. doi:10.3390/ijms22137202
Cançado, L. G., Pimenta, M. A., Neves, B. R. A., Dantas, M. S. S., and Jorio, A. (2004). Influence of the atomic structure on the Raman spectra of graphite edges. Phys. Rev. Lett. 93 (24), 247401. doi:10.1103/PhysRevLett.93.247401
Cavassin, E. D., de Figueiredo, L. F. P., Otoch, J. P., Seckler, M. M., de Oliveira, R. A., Franco, F. F., et al. (2015). Comparison of methods to detect the in vitro activity of silver nanoparticles (AgNP) against multidrug resistant bacteria. J. Nanobiotechnol. 13, 64–16. doi:10.1186/s12951-015-0120-6
Chaloupka, K., Malam, Y., and Seifalian, A. M. (2010). Nanosilver as a new generation of nanoproduct in biomedical applications. Trends Biotechnol. 28, 580–588. doi:10.1016/j.tibtech.2010.07.006
Claramunt, S., Varea, A., López-Díaz, D., Velázquez, M. M., Cornet, A., and Cirera, A. (2015). The importance of interbands on the interpretation of the Raman spectrum of graphene oxide. J. Phys. Chem. C 119 (18), 10123–10129. doi:10.1021/acs.jpcc.5b01590
Cronin, L. J., Mildren, R. P., Moffitt, M., Lauto, A., Morton, C. O., and Stack, C. M. (2014). An investigation into the inhibitory effect of ultraviolet radiation on Trichophyton rubrum. Lasers Med. Sci. 29, 157–163. doi:10.1007/s10103-013-1287-4
Cunha, D. G. F., Finkler, N. R., Lamparelli, M. C., Calijuri, M. do C., Dodds, W. K., and Carlson, R. E. (2021). Characterizing trophic state in tropical/subtropical reservoirs: Deviations among indexes in the lower latitudes. Environ. Manag. 68 (4), 491–504. doi:10.1007/s00267-021-01521-7
Expósito, N., Kumar, V., Sierra, J., Schuhmacher, M., and Giménez Papiol, G. (2017). Performance of Raphidocelis subcapitata exposed to heavy metal mixtures. Sci. Total Environ. 601– 602, 865–873. doi:10.1016/j.scitotenv.2017.05.177
Fogg, G. E. (1975). Algal cultures and phytoplankton ecology. 2nd ed. University of Wisconsin Press, 140. Madison, Wisconsin.
Fujioka, T., Ueyama, T., Mingliang, F., and Leddy, M. (2019). Online assessment of sand filter performance for bacterial removal in a full-scale drinking water treatment plant. Chemosphere 229, 509–514. doi:10.1016/j.chemosphere.2019.04.197
Hageskal, G., Lima, N., and Skaar, I. (2009). The study of fungi in drinking water. Mycol. Res. 113, 165–172. doi:10.1016/j.mycres.2008.10.002
International Organization of Standardization (2009). Biological evaluation of medical devices—Part 5: Tests for in vitro cytotoxicity. Geneva, Switzerland: ISO, 13. ISO 10993-5:2009.
Jeong, Y., Lim, D. W., and Choi, J. (2014). Assessment of size-dependent antimicrobial and cytotoxic properties of silver nanoparticles. Adv. Mater. Sci. Eng. 2014, 1–6. doi:10.1155/2014/763807
Kalantzi, I., Mylona, K., Toncelli, C., Bucheli, T. D., Knauer, K., Pergantis, S. A., et al. (2019). Ecotoxicity of silver nanoparticles on plankton organisms: A review. J. Nanoparticle Res. 21, 65. doi:10.1007/s11051-019-4504-7
Khare, P., Sharma, A., and Verma, N. (2014). Synthesis of phenolic precursor-based porous carbon beads in situ dispersed with copper-silver bimetal nanoparticles for antibacterial applications. J. Colloid Interface Sci. 418, 216–224. doi:10.1016/j.jcis.2013.12.026
Kleiven, M., Macken, A., and Oughton, D. H. (2019). Growth inhibition in Raphidocelis subcapita – evidence of nanospecific toxicity of silver nanoparticles. Chemosphere 221, 785–792. doi:10.1016/j.chemosphere.2019.01.055
Köser, J., Engelke, M., Hoppe, M., Nogowski, A., Filser, J., and Thöming, J. (2017). Predictability of silver nanoparticle speciation and toxicity in ecotoxicological media. Environ. Sci. Nano 4, 1470–1483. doi:10.1039/c7en00026j
Lee, T., Ooi, C.-H., Othman, R., and Yeoh, F.-Y. (2014). Activated carbon fiber - the hybrid of carbon fiber and activated carbon. Rev. Adv. Mat. Sci 36, 118–136.
Lorenz, C., Windler, L., von Goetz, N., Lehmann, R. P., Schuppler, M., Hungerbühler, K., et al. (2012). Characterization of silver release from commercially available functional (nano)textiles. Chemosphere 89, 817–824. doi:10.1016/j.chemosphere.2012.04.063
Marcuzzo, J. S. (2012). Portuguese (doctorate in materials and manufacturing processes) – technological institute of aeronautics. São José dos Campos, 152.Ultra-fast production of activated carbon fibers from textile PAN fiber (Produção ultrarrápida de fibras de carbono ativadas a partir de fibra PAN têxtil).
Marcuzzo, J. S., Cuña, A., Tancredi, N., Mendez, E., and Bernardi, H. H. (2016). Microporus activated carbon fiber felt from Brazilian textile PAN fiber: preparation, characterization and application as super capacitor electrode. Rev. Bras. Apl. Vácuo. 35, 58. doi:10.17563/rbav.v35i2.1022
National Council for the Environmentand Resolution, N.° (3572). 2005. Conselho Nacional do Meio Ambiente. Resolução n° 357 de 17/03/2005. Dispõe sobre a classificação dos corpos de água para o seu enquadramento, bem como estabelecimento das condições e padrões de lançamento de efluentes), 58–63. Provides for the classification of water bodies for their framing, as well as the establishment of conditions and standards for the release of effluents. Brasília, seção I.
Oliveira, B. R., Barreto Crespo, M. T., San Romão, M. v., Benoliel, M. J., Samson, R. A., and Pereira, V. J. (2013). New insights concerning the occurrence of fungi in water sources and their potential pathogenicity. Water Res. 47, 6338–6347. doi:10.1016/j.watres.2013.08.004
Oliveira-Filho, E. C., Matos Lopes, R., and Roma Paumgartten, F. J. (2004). Comparative study on the susceptibility of freshwater species to copper-based pesticides. Chemosphere 56, 369–374. doi:10.1016/j.chemosphere.2004.04.026
Oya, A., Yoshida, S., Alcaniz-Monge, J., and Linares-Solano, A. (1996). Preparation and Properties of an antibacterial activated carbon fiber containing mesopores. Carbon.
Oyanedel-Craver, V. A., and Smith, J. A. (2008). Sustainable colloidal-silver-impregnated ceramic filter for point-of-use water treatment. Environ. Sci. Technol. 42 (3), 927–933. doi:10.1021/es071268u
Panacek, A., Prucek, R., Safarova, D., Dittrich, M., Richtrova, J., Benickova, K., et al. (2011). Acute and chronic toxicity effects of silver nanoparticles (NPs) on Drosophila melanogaster. Environ. Sci. Technol. 45, 4974–4979. doi:10.1021/es104216b
Pannerselvam, B., Alagumuthu, T. S., Cinnaiyan, S. K., Al-Dhabi, N. A., Ponmurugan, K., Saravanan, M., et al. (2021). In vitro cytotoxicity and antibacterial activity of optimized silver nanoparticles against wound infectious bacteria and their morphological studies. J. Clust. Sci. 32, 63–76. doi:10.1007/s10876-020-01759-x
Park, S. J., and Jang, Y. S. (2003). Preparation and characterization of activated carbon fibers supported with silver metal for antibacterial behavior. J. Colloid Interface Sci. 261, 238–243. doi:10.1016/S0021-9797(03)00083-3
Pichel, N., Vivar, M., and Fuentes, M. (2019). The problem of drinking water access: A review of disinfection technologies with an emphasis on solar treatment methods. Chemosphere 218, 1014–1030. doi:10.1016/j.chemosphere.2018.11.205
Pinto, R. J. B., Almeida, A., Fernandes, S. C. M., Freire, C. S. R., Silvestre, A. J. D., Neto, C. P., et al. (2013). Antifungal activity of transparent nanocomposite thin films of pullulan and silver against Aspergillus Niger. Colloids Surfaces B Biointerfaces 103, 143–148. doi:10.1016/j.colsurfb.2012.09.045
Quintero-Quiroz, C., Acevedo, N., Zapata-Giraldo, J., Botero, L. E., Quintero, J., Zárate-Trivinõ, D., et al. (2019). Optimization of silver nanoparticle synthesis by chemical reduction and evaluation of its antimicrobial and toxic activity. Biomaterials Res. 23, 27. doi:10.1186/s40824-019-0173-y
Rodgher, S., Contador, T. M., Rocha, G. S., and Espindola, E. L. G. (2020). Effect of phosphorus on the toxicity of zinc to the microalga Raphidocelis subcapitata. An. Acad. Bras. Ciencias 92, e20190050. doi:10.1590/0001-3765202020190050
Rodrigues, A. C., da Silva, E. L., Quirino, S. F., Cuña, A., Marcuzzo, J. S., Matsushima, J. T., et al. (2018). Ag@activated carbon felt composite as electrode for supercapacitors and a study of three different aqueous electrolytes. Mater. Res. 22 (1). doi:10.1590/1980-5373-MR-2018-0530
Saleh, R. H., Al-Mukhtar, E. J., Alkhafaji, Z. A., al Hasnawy, M. H., and Al-Hasnawy, H. H. (2020). Antibacterial and cytotoxic effects of silver nanoparticles on Staphylococcus aurous and normal Vero cells. Ann. Trop. Med. Public Health 23, 13–17. doi:10.36295/ASRO.2020.2363
Sampaio, A. G., Gontijo, A. V. L., Araujo, H. M., and Koga-Ito, C. Y. (2018). In vivo efficacy of ellagic acid against Candida albicans in a Drosophila melanogaster infection model. Antimicrob. Agents Chemother. 62, e01716-e01718. doi:10.1128/AAC.01716-18
Silva, J. B., Piva, C., Falavigna-Guilherme, A. L., Rossoni, D. F., and de Ornelas Toledo, M. J. (2016). Spatial distribution and enteroparasite contamination in peridomiciliar soil and water in the Apucaraninha Indigenous Land, southern Brazil. Environ. Monit. Assess. 188, 217. doi:10.1007/s10661-016-5216-4
Tito, J. R., and Luna, L. G. (2020). Trophic status of 24 water reservoirs in eastern Cuba(Estado trófico de 24 embalses de agua en el oriente de Cuba. Cuban J. Chem. Cuba. De Química) 32 (1), 135–152.
Tjeng, L. H., Meinders, M. B. J., van Elp, J., Ghijsen, J., Sawatzky, G. A., and Johnson, R. L. (1990). Electronic structure ofAg2O. Phys. Rev. B 41, 3190–3199. doi:10.1103/PhysRevB.41.3190
Tran, N. H., Gin, K. Y. H., and Ngo, H. H. (2015). Fecal pollution source tracking toolbox for identification, evaluation and characterization of fecal contamination in receiving urban surface waters and groundwater. Sci. Total Environ. 538, 38–57. doi:10.1016/j.scitotenv.2015.07.155
Vanitha, M., KeerthiCao, P., and Balasubramanian, N. (2015). Ag nanocrystals anchored CeO2/graphene nanocomposite for enhanced supercapacitor applications. J. Alloys Compd. 644, 534–544. doi:10.1016/j.jallcom.2015.03.221
Wang, Z., Chen, J., Li, X., Shao, J., and Peijnenburg, W. J. G. M. (2012). Aquatic toxicity of nanosilver colloids to different trophic organisms: Contributions of particles and free silver ion. Environ. Toxicol. Chem. 31, 2408–2413. doi:10.1002/etc.1964
Who, (2017). Guidelines for drinking-water quality: Fourth edition incorporating the first addendum. Geneva: World Health Organization. Licence: CC BY-NC-SA 3.0 IGO.
Who, (2019). Water, sanitation, hygiene and health: A primer for health professionals. Geneva: World Health Organization. WHO/CED/PHE/WSH/19.149) Licence: CC BY-NC-SA 3.0 IGO.
WWAP (UNESCO World Water Assessment Programme) (2019). The united nations world water development report 2019: Leaving No one behind. Paris: UNESCO.
Wwap (United Nations World Water Assessment Programme), (2017). The united nations world water development report 2017. Wastewater: The untapped resource. Paris: UNESCO.
Yoon, K. Y., Jeong, H. B., Chul, W. P., and Hwang, J. (2008). Antimicrobial effect of silver particles on bacterial contamination of activated carbon fibers. Environ. Sci. Technol. 42, 1251–1255. doi:10.1021/es0720199
Keywords: water treatment, decontamination, silver nanoparticles, antimicrobial effect, toxicity
Citation: Martins IM, Sampaio AG, Lima GMG, Oliveira e Campos MAC, Rodgher S, Rodrigues-Siqueli AC, Baldan MR, Marcuzzo JS and Koga-Ito CY (2023) Application of textile (PAN-based) activated carbon fibers decorated with silver nanoparticles in water treatment. Front. Environ. Sci. 10:1100583. doi: 10.3389/fenvs.2022.1100583
Received: 16 November 2022; Accepted: 19 December 2022;
Published: 10 January 2023.
Edited by:
Belkis Sulbaran-Rangel, University of Guadalajara, MexicoReviewed by:
Hasbleidy Palacios Hinestroza, University of Guadalajara, MexicoPhilippe Le Coustumer, Université de Bordeaux, France
Copyright © 2023 Martins, Sampaio, Lima, Oliveira e Campos, Rodgher, Rodrigues-Siqueli, Baldan, Marcuzzo and Koga-Ito. This is an open-access article distributed under the terms of the Creative Commons Attribution License (CC BY). The use, distribution or reproduction in other forums is permitted, provided the original author(s) and the copyright owner(s) are credited and that the original publication in this journal is cited, in accordance with accepted academic practice. No use, distribution or reproduction is permitted which does not comply with these terms.
*Correspondence: Cristiane Y. Koga-Ito, Y3Jpc3RpYW5lLmtvZ2EtaXRvQHVuZXNwLmJy