- 1School of Engineering, University of California, Merced, Merced, CA, United States
- 2Water Systems Management Lab, School of Engineering, University of California, Merced, Merced, CA, United States
- 3Center for Water for Latin America and the Caribbean, School of Engineering and Science, Tecnológico de Monterrey, Monterrey, Mexico
Freshwater aquatic ecosystems are highly sensitive to flow regime alteration caused by anthropogenic activities, including river regulation and atmospheric warming-induced climate change. Either climate change or reservoir operations are among the main drivers of changes in the flow regime of rivers globally. Using modeled unregulated and simulated regulated streamflow under historical and future climate scenarios, this study evaluated potential changes to the flow regime due to climate change and reservoir operations for the major tributaries of the San Joaquin River Basin, California United States. We selected a set of Indicators of Hydrologic Alteration (IHA) to evaluate historical and projected future trends of streamflow dynamics: rise and fall rates, durations and counts of low and high pulses, and the magnitude of extremes. Results show that most indicators have pronounced departures from baseline conditions under anticipated future climate conditions given existing reservoir operations. For example, the high pulse count decreases during regulated flow conditions compared to increased frequency under unregulated flow conditions. Finally, we observed a higher degree of flow regime alteration due to reservoir operations than climate change. The degree of alteration ranges from 1.0 to 9.0% across the basin among all future climate scenarios, while reservoir operations alter the flow regime with a degree of alteration from 8.0 to 25%. This study extends multi-dimensional hydrologic alteration analysis to inform climate adaptation strategies in managed river systems.
Introduction
The natural flow regime heavily influences river geomorphological and ecosystem processes, affecting diverse abiotic and biotic components (Poff et al., 1997; Poff and Zimmerman, 2010). Consequently, changes in the flow regime have a potential negative impact on aquatic ecosystems and functions (Bunn and Arthington, 2002), not limited to biodiversity and productivity. Altered hydrology can result in perturbed sediment dynamics, limited habitat formation, poor biogeochemical cycling, invasion of non-native species, and disruption of phenological cues (Poff et al., 1997; Bunn and Arthington, 2002; Grantham et al., 2010; Poff and Zimmerman, 2010; Yarnell et al., 2010). Recently, there has been increased attention on how reservoir operations and water diversions affect the natural flow threatening riverine ecosystem (Grantham et al., 2010; Rheinheimer and Viers, 2015; Yang et al., 2018). Many rivers have already lost their natural conditions, due to reservoir operations such as hydropower, irrigation, and other human activities (Poff and Zimmerman, 2010; Fong et al., 2016; Hayes et al., 2018; Grill et al., 2019; Kuriqi et al., 2019). Although several efforts have been made to manage environmental flows and establish the scientific foundation to understand the mechanism of flow alteration (Richter et al., 1996; Poff et al., 1997; Bunn and Arthington, 2002; Poff and Zimmerman, 2010), adequate studies that have investigated the effect of both reservoir operations and climate change specifically for the San Joaquin River Basins where hydropower generation is predominant do not exist to date.
Understanding the harmful impacts caused by regulation and climate change on flow alteration are equally important to maintain healthy ecosystems. The fourth Climate Change Assessment suggests global temperatures will increase between 3.1 and 4.9°C (5.6–8.8°F) while the water supply from snowpack will decline by two-thirds by 2,100 (Pachauri and Reisinger, 2007). Such changes will significantly influence the timing and magnitude of river flow (Hidalgo et al., 2009; Das et al., 2013; Knowles et al., 2018). Knowles et al. (2018) suggest that projections of streamflow implied by climate models are essential to understand climate change impacts on hydrology and operations, as climate change can further stress already impacted ecosystems. However, the nature of the climate change involves uncertaintities and is not fully understood yet, in a systematic way. Moreover, there is no single climate model that fully captures the intricate details of hydrological processes, however considering historical hydrology might lead to poor environmental water planning (John et al., 2020). Therefore, there is a crucial need to understand the impacts caused by reservoir operations and anticipated climate change, in order to set realistic managed environmental flows and their ecological goals.
Gathering reliable ecological data can be costly and time-consuming. Further, many times existing datasets are insufficient to analyze complex ecological characteristics (Richter et al., 1996; Yang et al., 2018). However, many hydrologic indices have been proposed for different studies to overcome that problem (Richter et al., 1996; Clausen and Biggs, 1997, 2000; Pettit et al., 2001; Olden and Poff, 2003). Richter et al. (1996) offer a statistical approach, the Indicators of Hydrological Alteration (IHA), to characterize the temporal variability in hydrologic regimes, from which biologically relevant statistical attributes can be derived (Gibson et al., 2005; Law, 2013; Huang et al., 2017). These IHA metrics are widely used in assessing the impacts of anthropogenic activities such as dam regulation and agricultural diversion as well as climate change on river flow regimes (Richter et al., 1996, 1998; Magilligan and Nislow, 2005; Yan et al., 2010; Xue et al., 2017; Kakouei et al., 2018, 2018). The use of IHA metrics can improve establishing suitable strategies for planning, conservation and restoration of impacted ecosystems with the anticipated knowledge of flow regimes at the various phases of project implementation, including climate change studies.
The primary goal of this research was to quantify the impact of both reservoir operations and climate change on the river flow of the four major river basin in the San Joaquin River (SJR) in California, United States. For this, we used modeled hydrology, simulated realistic reservoir operations (e.g., flood control rules, instream flow requirements and urban/agricultural deliveries), and evaluated IHA metrics as a proxy for streamflow changes create four scenarios: free-flowing and regulated hydrology, both under past and future climate conditions. This analysis aims to answer the following research questions: 1) How do the flow metrics vary between the past and near future projections (2031–2060)? 2) What changes do they show under natural and regulated flow regimes, in both, past and near future projections? 3) What is the degree of hydrological alteration caused by climate change and river regulation?
To answer these questions, we integrated a water allocation model for the SJR basins. In particular, this research examines the flow regime at the outlet of all these basins, concentrating on ecologically relevant metrics that best represent the main aspects of the flow regimes and their sensitiveness to climate change (Olden and Poff, 2003; Rheinheimer and Viers, 2015; Langhammer and Bernsteinová, 2020). To analyze the statistical significance of differences, we employed nonparametric trend tests for each metric. We further quantified the degree of alteration integrating all metrics as a complementary result, showing a deviated hydrological regime from historical flow regimes at the basin outlet. Finally, we discussed policy and management implications from this study, followed by key limitations and future research avenues.
Study Area
The San Joaquin River (SJR) is one of California’s two major river systems draining California’s Central Valley into the ecologically sensitive Sacramento-San Joaquin Delta (CWB, 2019). With an area of 41,130 km2, the SJR basin yields an average annual surface runoff of about 2 km3 (1.6 million-acre-feet). The SJR comprises nine tributaries, including Cosumnes, Mokelumne, Calaveras, Stanislaus, Tuolumne, Merced, Chowchilla, Fresno, and Upper San Joaquin Rivers. This study considers a highly regulated network of reservoirs and hydropower facilities within the four most agriculturally important basins with the main tributary rivers: Stanislaus, Tuolumne, Merced, and Upper San Joaquin located in the Central Sierra Nevada (Figure 1). These subbasins are highly regulated and are crucial to water management in the state. The main catchment characteristics of each basin are presented in Table 1, and the hydropower facilities within each basin are included in Supplementary Tables S1–S4.
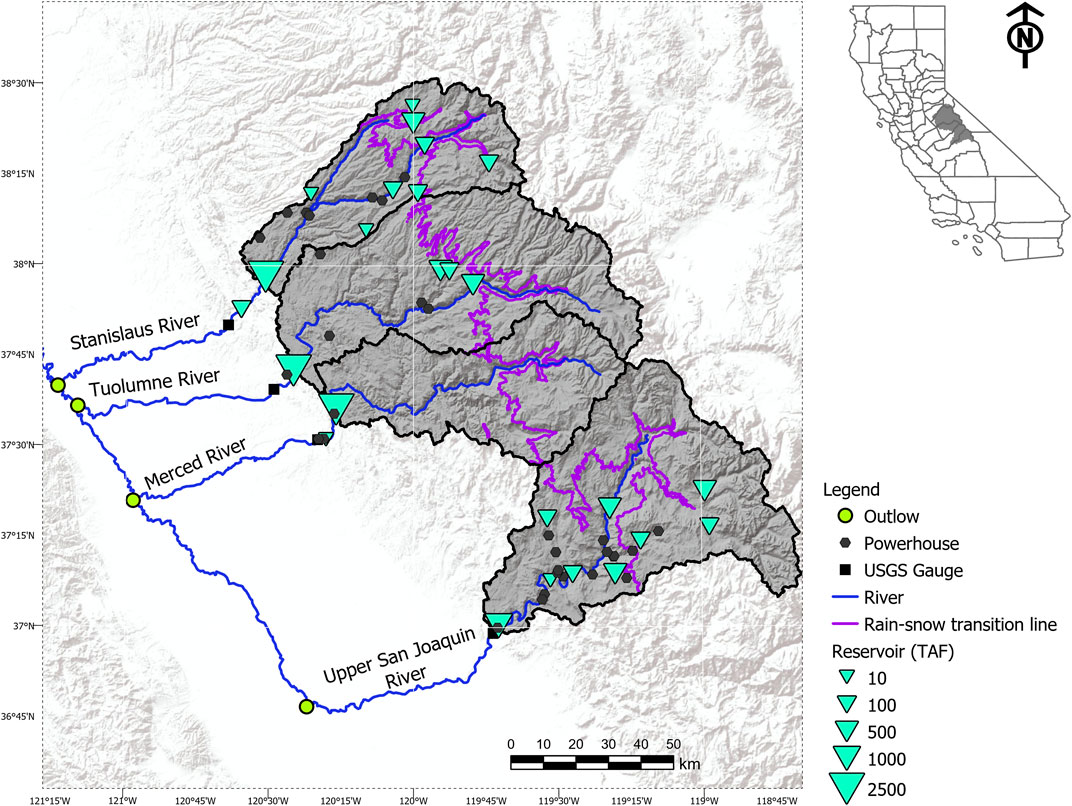
FIGURE 1. Study area map, San Joaquin River system, encompassing the four major basins: Stanislaus, Merced, Tuolumne, and Upper San Joaquin and their major characteristics (Source: Data Basin (Peterman, 2002)). The USGS stations in black squares are the locations where IHA analyses were performed.
Each basin has a major storage reservoir at its lowest elevation (basin outflow), formed by terminal “rim dams.” These multipurpose reservoirs manage water for flood control, recreation, urban and agricultural demand, environmental needs, and hydropower generation. The reservoirs and powerhouses in the region have a wide range of storage and generation capacities, ranging from less than 12 to 3,085 million m3 and from less than 5 MW to over 500 MW, respectively (Table 1 and Supplementary Tables S1–S4).
The main purpose for regulation in the Stanislaus and Upper San Joaquin basins is hydropower generation, meanwhile the Tuolumne and Merced basins are driven by agricultural and/or urban water supply demands. Due to chronic anthropogenic effects in the past and the construction of both the rim dams and a vast array of high elevation reservoirs, the SJR system has been facing challenges in maintaining river ecosystems (Obegi and Wearn, 2016). Environmental flows in the region are prescribed generally as minimum and sometimes also maximum instreamflows, with flows varying between certain thresholds dependent upon various water year type classifications. Consequently, the State Water Resources Control Board recommended maintaining 30–50% of the natural flows in the SJR and its tributaries to reduce the environmental impacts of flow regulation (SWRCB, 2018). The high elevation reservoirs are operated independently by different utilities, mostly driven by hydropower revenue. The rim dams, on the other hand, as large storage facilities that feed into the SJR, are the focus of restoration for the SWRCB proposal that encompasses the restoration of the lower SJR as well as the Sacramento-San Joaquin Delta located downstream. In addition, the SJR is vulnerable to climate change even though hydropower production tends not to be greatly affected (Madani and Lund, 2009; Vicuña et al., 2011; Ligare et al., 2012; Rheinheimer and Viers, 2015).
Methods
Data Sources
This study employed daily gridded (1/16°) runoff data developed by Livneh et al. (2015), forcing the Variable Infiltration Capacity (VIC) model (Liang et al., 1994), with observed meteorological data from NOAA/OAR/ESRL PSD (National Oceanic and Atmospheric Administration/Oceanic and Atmospheric Research/Earth System Research Laboratory Physical Sciences Division), Boulder, Colorado, United States (Berkeley, 2017; NOAA, 2017; UC). Hereafter, the historical hydrology dataset (1950–2013) is referred to as “Livneh” data.
For the future scenario (2030–2060), we considered climate-altered hydrology data from ten Global Circulation Models (GCMs) identified by the California Department of Water Resources (CA, 2015) and California’s fourth Climate Change Assessment as the best representative to California (Herman et al., 2018). The GCMs are forced by the Representative Concentration Pathway (RCP) 8.5, a “business-as-usual” greenhouse gas emissions scenario. These data were generated by the Scripps Institution of Oceanography, as well as the monthly bias-corrected data used for bias-correction. Livneh and GCMs datasets were bias-corrected at basin level using bias-corrected monthly data available with the daily data. In addition, subbasin hydrology was bias-corrected when needed with reference to observed streamflow and storage data from USGS gauges by employing the quantile mapping and the linear scaling approaches (Maskey, 2021).
The basin outflows are regulated sites below the hydropower stations at each rim dam. All rim dams are operated primarily for agricultural water supply, and flood control, among other competing human demands, while releasing environmental flows to maintain instream flow requirements at designated locations according to federal regulatory requirements. Hydropower is always the lowest priority at the rim dams, even if hydropower is ultimately a major benefit of these multipurpose reservoirs. To simulate water allocation in the region, we used a water systems simulation and optimization model built on Pywr, a Python package for generalised network resource allocation (Tomlinson et al., 2020). Then, we assumed historical water and hydropower demand under future climate conditions.
Indicators of Hydrological Alteration Framework
This study employed a suite of Indicators of Hydrological Alteration (IHA) metrics developed by Richter et al. (1996) to evaluate the impacts of reservoir operation and climate change on flow regimes downstream of the rim dams. IHA encompasses 33 metrics grouped into five categories describing a flow regime in terms of the magnitude of monthly flow, magnitude and duration of extreme annual flows, the base flow condition, the timing of annual extreme flow conditions, occurrence and duration of high and low pulses, and rate and frequency of flow change (Table 2).
First, we analyzed all IHA metrics per water year basis for identifying the flow parameters that provide better insight into the degree of hydrological alteration. In this regard, we concentrated on groups 4 and 5 (except reversals) in addition to the magnitude of annual extremes. We also incorporated the analysis of flow duration curves to describe the flow regime changes further. The primary goal of this study was to quantify the impact of reservoir operations on river flow regime and compare it to the impact of climate change on the natural (unregulated) hydrology. Using expert judgment and knowledge based on prior work on flow alteration in the region (Grantham et al., 2014; Rheinheimer and Viers, 2015), we concluded a pre-selection of metrics would be more representative of changes significant to environmental flows, as well as avoid redundancy, many matrics are correlated. Therefore, a subset of eight flow metrics were selected for the specific purpose of this study: 1) the number and duration of the low and high pulse, 2) rise and fall rate, and additional 3) magnitudes of extremes, in addition to flow duration curves.
Flow duration curve is characterized by the cumulative frequency curve of sorted daily flow
where
High pulse count and duration refer to the number and duration (in days) of flow events above 75-percentile of daily flow over a year, respectively.
Low pulse count and duration refer to the number and duration (in days) of flow events below 25-percentile of daily flows over a year, respectively.
Rise rate is represented by the mean of all positive differences between consecutive daily mean flows within each year. The fall rate is the average of all negative differences between consecutive daily flows. Magnitude of annual extremes is defined as highest and lowest flows within each year.
Trend Test: Mann-Kendall Test
Managing ecosystem is difficult due to unpredictable variability of climate and hydrological attributes and such processes have often been widely assumed as stationary (Nathan et al., 2019). Therefore we assessed the IHA metrics to identify possible trends reflecting the reservoir operations and climate change impact. For this, we used the nonparametric Mann-Kendall test (Mann, 1945; Kendall, 1948; Barbalić and Kuspilić, 2014; Tian et al., 2019). The null hypothesis,
where
If
We tested selected indicators separately for the pre-impact and post-impact periods, although all metrics were calculated (Supplementary Materials, Supplementary Figures S1–S13, and Supplementary Tables S5, S10). We considered the historical timespan for each basin for pre-impact as listed in Table 1 and future years for post-impact analysis to assess climate change impacts. We considered the pre-and post-impact as natural (unregulated) and regulated hydrology, and historical and future climate driven-hydrology.
Degree of Alteration
To quantify the impacts of regulation on the study area, we estimated the DOA as Xue et al. (2017) suggested. Richter et al. (1998) defined the degree of hydrological alteration of a flow regime for each indicator, in percentage, as:
where
The DOA is then classified within five categories: slight alteration (<20%), low alteration (20–40%), moderate alteration (40–60%), high alteration (60–80%), and severe alteration (>80%) (Xue et al., 2017).
Results
Flow Duration Curves
Figure 2 shows the flow duration curve for regulated and unregulated flows under historical and future climate scenarios for each basin. Regulated flows show lower variability of daily flows with stepped curves, while unregulated flows exhibit smoother curves with steep declines. That is caused by the prescribed static environmental flows, that might vary in magnitude within seasons or water year types, removing part of the natural flow variation. In all cases, the higher the probability of exceedance, the lower the flow. To detect shifting extremes, Figure 2 also shows two thresholds at 28.3 and 2.83 cms [1,000 cubic feet per second (cfs) and 100 cfs, respectively]. The highest flows are mostly removed by the river regulation, with a lower reduction in the Upper San Joaquin due to its rim dam’s lower storage capacity, mean while the lowest flows tend to be reduced in the Merced and Upper San Joaquin, and absent in the Tuolumne and Stanislaus basins.
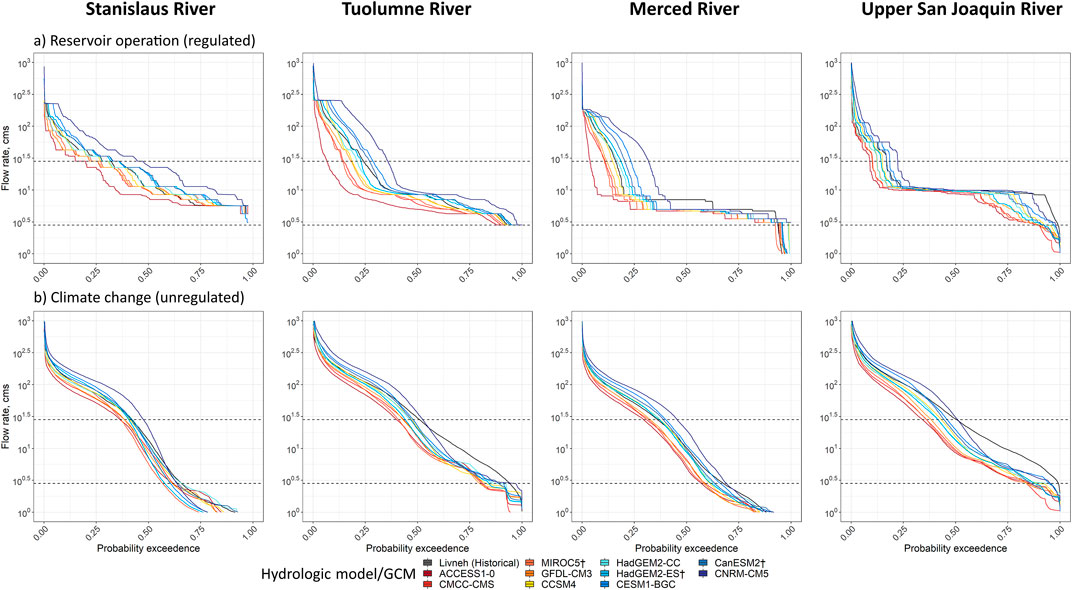
FIGURE 2. Flow duration curve for regulated (top) and unregulated (bottom) by scenarios. The top and bottom horizontal lines are the lines of 28 and 2.83 cms, respectively.
Indicators of Hydrological Alteration Metrics
While high pulse due to climate change may occur as high as 21 days annually in the Upper San Joaquin River, the Tuolumne, and Merced River can experience above 17 high pulse every year (Supplementary Figures S1, top). In warmer climate projections, high pulse counts decrease with regulation in Stanislaus and Tuolumne Rivers. At the same time, the other basins show no distinct variability in high pulse counts caused by climate change. However, cooler climate models show increased high pulse count in all basins under regulation, but no distinct patterns in high pulse counts in unregulated hydrology. Supplementary Tables S5, S6 include median values and quartiles of selected metrics among all 10 GCMs and corresponding relative changes from historical flows, showing an increase in the occurrence of high pulses caused by climate change, which is removed with regulation, leading to a decrease in high pulse counts for the Merced River basin (increased by 3.3%), possibly because it is the only basin with no high-elevation reservoirs to contribute to flow regulation.
On the other hand, low pulse count can be increased by 85.0% in the Stanislaus River, for example, in the unregulated hydrology, meanwhile it decreases by 2.5% in the Tuolumne River (Supplementary Figures S5, bottom). Supplementary Tables S5, S6 show an increase in median low pulse counts in the future, although it slightly decreases in the Upper San Joaquin River under regulation.
High pulse duration is increased in the future climate by 51.0, 31.3, 10.0, and 7.3% in the Stanislaus, Tuolumne, Merced, and Upper San Joaquin River, in order (Supplementary Figures S2, top). Supplementary Figures S2 also depicts increased high pulse duration under regulation, except for the Tuolumne River, where high pulse duration decreases by 15.2%. Tables 3, 4 reported that the median changes in high pulse duration vary from 34.3 to 56.1% for regulated flows and from -12.5–17.4% for unregulated flows.
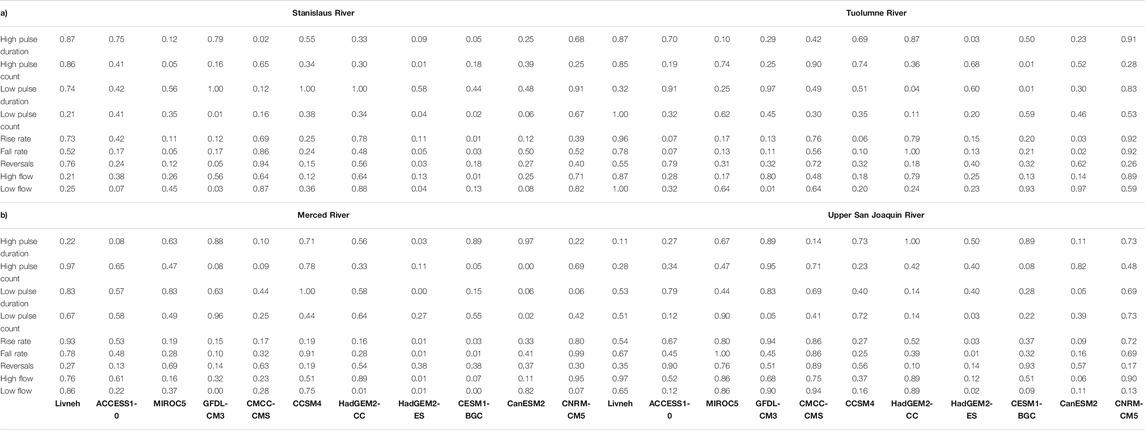
TABLE 3. p-value of Mann-Kendall test to identify significant monotonic trend in selected IHA metrics for regulated flows in the 1) Stanislaus (left) and Tuolumne (right); and 2) Merced (left) and Upper San Joaquin (right). The range of p-value varies from 0 to 1, implied by the color from dark blue to red.
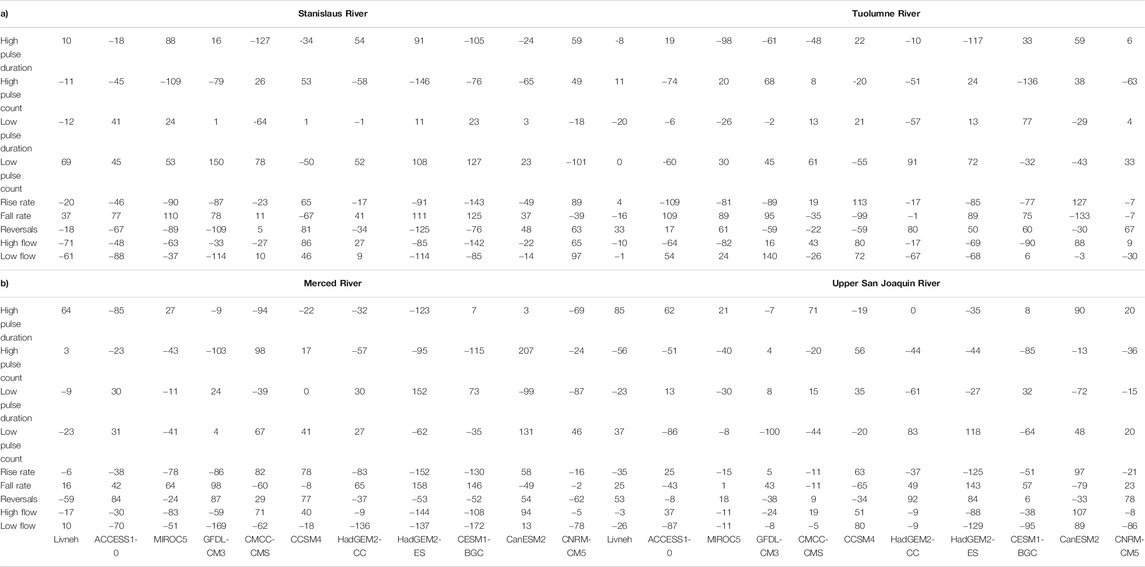
TABLE 4. Mann-Kendall test statistics implying increasing and decreasing trends of selected IHA metrics. Pink represents positive trends while cyan reflects negative trends in 1) Stanislaus (left) and Tuolumne (right); and 2) Merced (left) and Upper San Joaquin (right).
Low pulse duration tends to be decreased under climate change in the unregulated flows, by 29.6% in the Merced River, while the other basins show increased low pulse duration (Supplementary Figures S2, bottom). However, low pulse duration increases in regulated flows, in order, by 31.2, 250.0, 43.4, and 51.7% in the Stanislaus, Tuolumne, Merced, and Upper San Joaquin Rivers, respectively. Therefore, climate change might not cause a decrease in low pulse duration in the natural hydrology for most basins, however, river regulation will likely cause that even when the natural environment shows an opposite trend. Supplementary Table S6 reports that the median flows of low pulse duration are decreased by 2.9, 5.6, 54.3, and 17.7%, meanwhile median low pulse duration in regulated flows increases by 15.1, 74.6, 480.4, and 108.4% for the GCMs compared to historical flows in the Stanislaus, Tuolumne, Merced, and Upper San Joaquin basins, respectively (Supplementary Table S5).
Supplementary Figures S3 depicts changes in rise rate and fall rate for historical and future scenarios. Both show high variability demonstrating frequent fluctuations between water surplus and deficit throughout the study period (Langhammer and Bernsteinová, 2020). Unregulated hydrology exhibits either an increased or a decreased rise rate among GCMs in terms of its variability. Among all, the coolest/wettest climate projection (CNRM-CM5), exhibits a higher rise rate (Supplementary Figures S3, top). However, the median values of rise rates presented in Table 3 are always higher than historical rise rates in all basins, ranging from 21.4 to 79.6 cms/day, on average. Supplementary Figures S3, top, also shows increased rise rates in the unregulated hydrology for all basins among all models. Supplementary Table S6 lists median rise rates increase by 32.8, 49.4, 39.0, and 69.6% for the Stanislaus, Tuolumne, Merced, and Upper San Joaquin Rivers, respectively.
Supplementary Figures S3 (bottom) shows decreased or increased fall rates, specifically in regulated flows in the Stanislaus and Tuolumne Rivers. Except for projections from MIROC5 in the Upper San Joaquin River, the Merced and Upper San Joaquin Rivers exhibit increased fall rates considering the future hydrology and the current reservoir operations. Unregulated hydrology experiences an increased fall rate for all basins with increased variance. However, median flow values show a higher fall rate in historical regulated flows, as reported in Supplementary Tables S5, S6, with even higher fall rates occurring in the historical unregulated flows.
The variability of the magnitude of maximum annual river flow observed in Supplementary Figures S4 suggests that higher flows tend to occur in cooler/wetter GCMs either with or without regulation in all basins, suggesting that the regulation in these basins might not control higher flows under a greater occurrence of flood events. Supplementary Tables S5, S6 report increased medians of maximum flow by 57.2, 72, 65.7, and 81.3% in the Stanislaus, Tuolumne, Merced, and Upper San Joaquin Rivers.
Future unregulated low flows are always lower than historical low flows. The future regulated low flows are either increased or decreased depending on the GCMs (Supplementary Figures S13), suggesting that regulation might or not avoid this negative impact of climate change on the natural hydrology. However, the median values among all 10 GCMs in Supplementary Tables S5, S6 are lower than historical flows.
As presented in Supplementary Figures S5, decreased January flow is observed for regulated flows in all future scenaros in the Merced River, except for CNRM-CM5 (coolest/wettest). In contrast, unregulated flows in January are always increased in the Stanislaus River. February regulated flows increase under cooler climate projections in all four basins (Supplementary Figures S6). Both regulated and unregulated March flows increase in all GCMs, most notably for the cooler/wetter projections (Supplementary Figures S7), likely related to the occurrence of earlier snowmelt. Supplementary Tables S57, S8 show decreased median values of both regulated and unregulated summer flows, and different signatures of 1-, 3-, 7-, 30-, and 90-days minimum and maximum flows for unregulated and regulated hydrology (Supplementary Figures S8–S11). Median changes in the baseflow index in all basins are decreased for regulated and unregulated flow (Supplementary Figures S12, Supplementary Tables S7, S8), indicating the lower contribution of the baseflow to the total streamflow in the near future, making rivers more dependent on precipitation.
Under regulation, events of maximum flow are shifted to later in the year. In contrast, minimum flow events may happen earlier in the Stanislaus and Merced (Supplementary Tables S5). Meanwhile, the opposite is observed in the Tuolumne and Upper San Joaquin. Unlike regulated flows, both extreme events occur earlier in the unregulated flows (Supplementary Tables S8). Supplementary Tables S7 lists increased reversals for regulated flows, except for the Stanislaus River. Among them, the Upper San Joaquin River has the highest increase in reversals. Supplementary Tables S8 reports increased reversals in unregulated hydrology for all basins under future climate projections. Overall, the results show that the hydrological alteration caused by regulation is more pronounced than those of climate change, however their concomitant effect tend to have varying effects depending on the basin and its degree of regulation.
Statistical Test on Trends of IHA Metrics
This study investigated the significance of trends in all IHA parameters except for zero flow days by employing Mann-Kendall tests (Equations 2–3). Supplementary Table S5 lists these results, concentrating on the 8 IHA metrics selected for this study (see Section 3.2). As shown, IHA metrics derived from historical (un)regulated flows do not exhibit any trends, although some GCMs show very few trends in future signatures. Furthermore, trends are not consistent in all four basins and between regulated and unregulated flows. For instance, regulated flows show a trend in low pulse count, rise rate, fall rate, and high flow in the CESM1-BGC scenario for the Stanislaus River and the rise rate and fall rate in the CanESM2 for the Tuolumne River (Table 3a). Likewise, unregulated flows exhibit trends in low flow implied by HadGEM2-CC and HadGEM2-ES in the Merced and HadGEM2-ES and CESM1-BGC in the Upper San Joaquin River (Supplementary Tables S6B). Finally, trends in IHA metrics were more observed under regulated than unregulated flow regimes.
The Mann-Kendall test statistics that infer increasing or decreasing trends of IHA metrics are included in Table 4. The fall rate and low pulse number exhibit a higher chance of increasing trends in the regulated flows in the Stanislaus River. The rise rate has a high chance of decreasing trends overtime (Table 4a). In the Upper San Joaquin River, wetter climate projections show a significant decreasing trend in high pulse duration under regulation and a significantly increasing fall rate trend with a cooler climate (Supplementary Figures S10A). There are no basic patterns across the study area and even among individual indicators. However, the lack of trends is driven by an increase in variance in most cases, and most GCMs imply decreasing trends under the regulated flow regime compared to the unregulated.
Degree of Hydrological Alteration
We finally quantified the DOA on each basin employing Eqs 4, 5, integrating all 33 IHA metrics. Figure 3 summarizes the overall DOA for each GCMs in each basin. As seen, the DOA for regulated flows is more than twice as high as the one of unregulated flows. Considering future scenarios, some GCMs show a DOA five times higher in unregulated flows. However, while the range of DOA for regulated flows across the study area among all climate scenarios is 9.4–24.5%, the range for unregulated flows varies only from 1.8–8.6%.
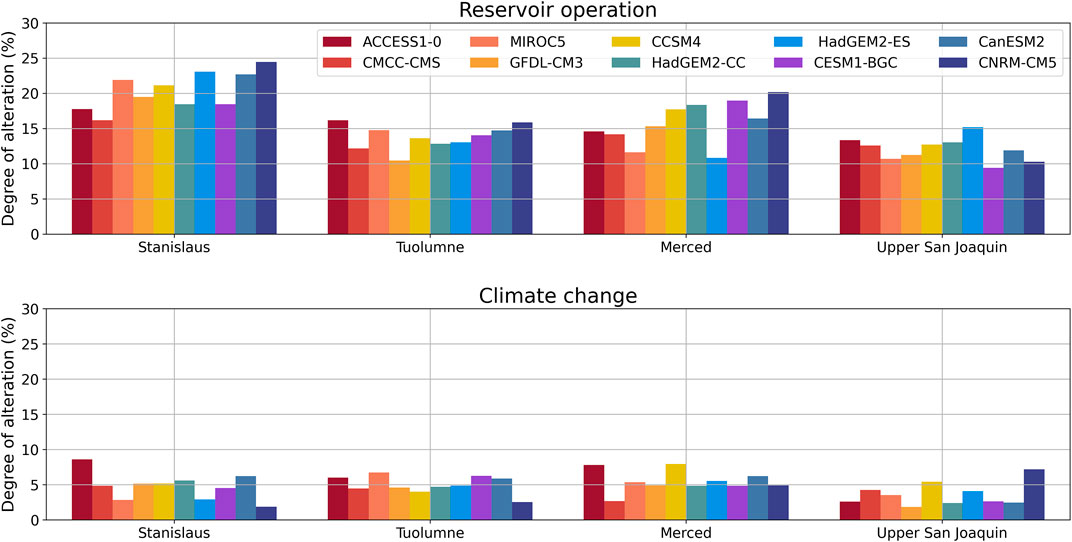
FIGURE 3. Degree of alterations integrating all 33 IHA metrics implied by GCMs, ordered from warmer/drier climate to cooler/wetter climate.
Figure 3 portrays the highest degree of alteration in the Stanislaus River in regulated flows (24.5%). The Upper San Joaquin River is the least impacted basin (as low as 9.4%). Conversely, Merced River’s unregulated flows show greater alteration, and the Upper San Joaquin is still least impacted. To summarize, the alterations are in the range of 20–40%, considered as low alteration in the regulated flows, and alteration in the unregulated flow driven by climate change shows slight alteration with a DOA <20%. In conclusion, the hydrological flow regime may be less altered by climate change than by reservoir operations.
Discussion
This research investigated the hydrological alteration in the flow regime of the San Joaquin River basins over the past 3 decades and the future 3 decades employing ecologically relevant IHA metrics using modeled historical and projected future hydrologic data used as inputs to a water allocation simulation model. We explored the hydrological alteration due to hydropower operation as well as climate change. Though our study has limitations regarding the relatively short period of data analyzed, modeling results, and inconsistent behavior of IHA metrics, some implications from this study can be drawn.
In brief, the flow regimes downstream of hydropower facilities are likely more altered by reservoir operations rather than by climate change. Understanding such changes can help identifying strategies to conserve and restore ecosystems already stressed by human intervention under a changing climate, such as focusing on maintaining stream temperature and creating a favorable habitat downstream (Null et al., 2013; Rheinheimer and Viers, 2015; Yasarer and Sturm, 2016). We observed more stepped and wider flow duration curves at each location, what is even more pronounced under the climate changre scenarios for regulated flows, when compared to unregulated flows. Stepped flow duration curves indicate intermittent flow variation due to reservoir operations in particular the maintenance of static instreamflow requirements that might impact the movement, establishment and environmental cues for aquatic life.
We investigated the variability of IHA metrics among all ten GCMs across the study area, concentrating on the outlet of each basin, downstream of existing powerhouses. Even though the metrics greatly vary, and specific trends are difficult to discern, some notable trends are apparent in specific cases. For instance, the decreased high pulse counts and increased high pulse counts are observed in regulated and to a greater extent in unregulated flows. As such, less high pulses under regulation tend to provide less nutrients and habitat to the aquatic life along the riverbank, the opposite effect that could be promoted by the greater high pulse counts found in the unregulated flows (Xue et al., 2017). We also confirm that a low pulse count tends to increase under a warmer climate, which might cause the loss of native species, as observed elsewhere (Gao et al., 2018; Kakouei et al., 2018). Although most GCMs show decreased high pulse duration under unregulated flow regime, increased high pulse rate under the regulated flow regime leads to unstable river flow due to increased high and low pulse duration (Richter et al., 1996). There is an increase in the rise rate of unregulated flows, which are greater under regulation caused by the dam (Magilligan and Nislow, 2005). These climate change impacts likely caused by flood control rules lead to tradeoffs between environmental water users and economics (Rheinheimer and Viers, 2015). The occurrence of more extremes reservoirs tend to be fuller in the rainy season and emptier in the dry season when surface water is most needed for irrigation and hydropower generation, and at the same time minimum and maximum instreamflow requirements also need to be maintained in both cases.
We also showed that winter flows tend to increase in cooler/wetter climate scenarios, although it might not be suitable for native species (Gibson et al., 2005; Gao et al., 2018). Decreased summer flow might indicate increased stream temperature and reduced dissolved oxygen, which is also unsuitable for local species (Gibson et al., 2005; Yang et al., 2017). Finally, we also observe that warmer climate projections and river regulation lead to decrease in the magnitude of high flows, which may not be favorable conditions for creating new habitats (Poff, 2002). Further, a decrease in low flows caused by regulation may detach the flood plain from the river, resulting in loss of off-channel aquatic life (Gibson et al., 2005). Considering that, SWRCB’s proposal of setting an effective target as a percentage of natural flow might not be the best decision to overcome these challenges, as the natural hydrology tends to be already affected by climate change in the near future. Therefore, environmental water management needs to be more carefully planned as these disturbances are exacerbated under regulation. A more effective ecological conservation can be achieved through the implementation of the functional flows framework, as proposed by (Granhtam et al., 2010; Granhtam et al., 2014), for the for California’s rivers. The functional flow regime retains key components of the natural hydrograph that support biophysical processes across the riverscape (e.g., wet season flood flows and spring recession flows) that provide cues for fish migration and reproduction, for example (Yarnell et al., 2020, 2015).
This work utilized a traditional approach to investigating the effects of climate change on river flow using the Mann-Kendall test. However, there are no clear trends in most metrics in time and space, even among GCMs. The lack of trends in flow metrics is driven by an increase in variance in most cases, and most GCMs imply decreasing trends under the regulated flow regime compared to the unregulated. Nevertheless, the flow regime differs between the regulated and unregulated hydrology under historical and future climate change scenarios. This study shows that reservoir operation’s impact on river flow regimes leads to higher DOA than changes in hydroclimatic conditions in the main tributaries of the SJR. We found that the DOA for regulated flows is more than twice as high as the ones caused by climate change. Arheimer et al. (2017) also observed greater impacts caused by hydropower installation and associated river regulation than climate change in snow-fed river basins as the SJR. However, both the future climate and reservoir operations can influence ecological processes in aquatic ecosystems and conjunctively further alter the functioning of ecosystems (Gibson et al., 2005; Rheinheimer and Viers, 2015).
This study demonstrated the practical application of IHA metrics to categorize both unregulated and reservoir-induced regulations to river flows. In addition to studying IHA metrics, we also assessed the magnitude of extremes. Such enhancement has the potential to recommend flow metrics in any river basin process, such as in-stream flow requirements, drought assessment, and of course, environmental flow recommendations that lead to ecological restoration activities within the river basin (Yarnell et al., 2020, 2015). In addition, this study is applicable elsewhere to investigate flow alteration induced by climate change and other human-induced activities such as urban development, agriculture, and beyond. Finally, such a holistic approach can be combined with ecological models to assist water and land managers in prioritizing ecological protection plans.
Limitations and Future Work
The present study focused on the impact of reservoir operation and climate change on river flow. Although the four basins also have agricultural and/or urban water users, this study does not implicitly address the impacts of demand-driven diversions, as they generally occur at or right below the rim dams. We also do not incorporate other ecosystem characteristics, changes in energy demand, and mechanistic relationships between abiotic conditions and biotic quality. The combined effect of dam operation and other anthropogenic activities that impact downstream water quality also need attention in future work.
This study could be improved by integrating information from other climate variables, including other river flow conditions such as water quality and annual water volume that categorize different water year types in detail. Since there are 33 IHA metrics, most are redundant (Olden and Poff, 2003). Nevertheless, they are useful in devising a simpler metric similar to water year types implemented by DWR. Future work could integrate all metrics using a robust machine learning classifier, e.g., Random Forest, support vector machine. Such may be useful to predict the flow metrics 1 year ahead by establishing transition probability matrices among integrated IHA metrics (Puente et al., 2017). Knowledge of future IHA metrics such as monthly average flow and low flow could be useful to daily downscale using a deterministic approach, e.g., Maskey et al. (2019), and opens further advancement with knowledge of daily instream flow requirements for aquatic life.
Conclusion
This study investigated climate change and flow regulation effects on hydrological alteration in the San Joaquin River basin. We adopted a systematic research protocol to concisely interpret the IHA metrics derived below hydropower facilities in the past and future climate. The result revealed flow alterations that can lead to ecosystem degradation caused by river regulation and future climate scenarios implied by ten GCMs. As observed in this study, hydrological alteration in both cases can affect frequency, duration, and change rate. In addition, dam construction led to decreased winter flows, as water is saved to be used later in the year, causing an increase in summer flows, which exacerbate ecosystem disturbance. In both cases, the changes in the flow regime alter the ecological behavior of the river basins. Some alterations may or may not be beneficial. For instance, a decrease in low flow may detach the floodplain from the mainstream, while increased high flow may benefit aquatic life. While hydrological alteration due to climate changes will be gradual, reservoir operations abruptly alter the flow regime.
Our results indicate significant deviations in the future inferred from the trend test and DOA. However, only some models and specific basins show some trends, therefore, the interpretation of IHA is more model-specific and site-specific, and even metric-specific. The higher range of DOA values suggests more attention should be given to impacts from reservoir operations compared to climate change. The flow design suggested by SWRCB can help mitigate the impacts of regulation, but will not avoid the impacts of climate change that will likely already be experienced in the coming decades. Therefore, we suggest the adoption of functional flows as a better approach.
Hydrological changes driven by agricultural deliveries cannot be directly estimated as diversions occur at or right below the reservoirs, impacting water storage but not environmental flows directly. In addition, the river regulation can help ameliorate some of the harmful effects of warming, such as increasing toxic compounds, lowering oxygen content, and reducing desired pH levels. Nevertheless, such adverse impact can be overcome with a more detailed scientific study integrating human and climate change impact on river flow.
We have demonstrated the usage of the IHA framework for investigating changes in river flow regimes associated with reservoir operations and climate change. Findings from this study suggest riverine ecosystem processes are sensitive to changes in IHA metrics, which augment empirical knowledge on ecological response, environmental drivers, and so on to support and establish environmental flow standards. Therefore, similar studies elsewhere offer suitable management decisions in maintaining minimum flow requirements needed to sustain downstream ecosystems below multipurpose reservoirs under different climate scenarios.
Data Availability Statement
The original contributions presented in the study are included in the article/Supplementary Material, further inquiries can be directed to the corresponding author.
Author Contributions
MM: conceptualization, methodology, coding, original drafting. GFD: review and edits, quality control. AR: review and edits, quality control, project management. DR: model development, review, and edits, quality control. JM-A: review. JV: conceptualization, supervision, review and editing, funding acquisition.
Funding
Partial funding for this research was provided by the United States Department of Energy (DOE) United States-China Clean-Energy Research Center - Water Energy Technologies CERC-WET (Grant No. DE-IA0000018) and by the California Energy Commission (CEC 300–15-004).
Conflict of Interest
The authors declare that the research was conducted in the absence of any commercial or financial relationships that could be construed as a potential conflict of interest.
Publisher’s Note
All claims expressed in this article are solely those of the authors and do not necessarily represent those of their affiliated organizations, or those of the publisher, the editors, and the reviewers. Any product that may be evaluated in this article, or claim that may be made by its manufacturer, is not guaranteed or endorsed by the publisher.
Acknowledgments
We would like to thank Daniel Nover, Aditya Sood, Jenny Ta for their early ideas about this study and the UC Merced Spatial Analysis and Research Center for helpful information in preparing a map for the study area. We also highly appreciate reviewer for useful suggestions that improved our manuscript.
Supplementary Material
The Supplementary Material for this article can be found online at: https://www.frontiersin.org/articles/10.3389/fenvs.2022.765426/full#supplementary-material
References
Arheimer, B., Donnelly, C., and Lindström, G. (2017). Regulation of Snow-Fed Rivers Affects Flow Regimes More Than Climate Change. Nat. Commun. 8, 62–69. doi:10.1038/s41467-017-00092-8
Barbalić, D., and Kuspilić, N. (2014). Trends of Indicators of Hydrological Alterations. Građevinar 66, 613–624. doi:10.14256/jce.1003.2014
Berkeley, U. C. (2017). Livneh Data Clipped to California-Nevada. Available at: http://albers.cnr.berkeley.edu/data/noaa/livneh/CA_NV/(accessed February 10, 2021).
Bunn, S. E., and Arthington, A. H. (2002). Basic Principles and Ecological Consequences of Altered Flow Regimes for Aquatic Biodiversity. Environ. Manage. 30, 492–507. doi:10.1007/s00267-002-2737-0
Ca, D. W. R. (2015). Perspectives and Guidance for Climate Change Analysis. Sacramento: California Department of Water Resources.
Clausen, B., and Biggs, B. J. F. (2000). Flow Variables for Ecological Studies in Temperate Streams: Groupings Based on Covariance. J. Hydrol. 237, 184–197. doi:10.1016/s0022-1694(00)00306-1
Clausen, B., and Biggs, B. (1997). Relationships between Benthic Biota and Hydrological Indices in New Zealand Streams. Freshw. Biol. 38, 327–342. doi:10.1046/j.1365-2427.1997.00230.x
Das, T., Maurer, E. P., Pierce, D. W., Dettinger, M. D., and Cayan, D. R. (2013). Increases in Flood Magnitudes in California under Warming Climates. J. Hydrol. 501, 101–110. doi:10.1016/j.jhydrol.2013.07.042
Fong, C. S., Yarnell, S. M., and Viers, J. H. (2016). Pulsed Flow Wave Attenuation on a Regulated Montane River. River Res. Applic. 32, 1047–1058. doi:10.1002/rra.2925
Gao, B., Li, J., and Wang, X. (2018). Analyzing Changes in the Flow Regime of the Yangtze River Using the Eco-Flow Metrics and IHA Metrics. Water 10, 1552. doi:10.3390/w10111552
Gibson, C. A., Meyer, J. L., Poff, N. L., Hay, L. E., and Georgakakos, A. (2005). Flow Regime Alterations under Changing Climate in Two River Basins: Implications for Freshwater Ecosystems. River Res. Applic. 21, 849–864. doi:10.1002/rra.855
Grantham, T. E., Merenlender, A. M., and Resh, V. H. (2010). Climatic Influences and Anthropogenic Stressors: an Integrated Framework for Streamflow Management in Mediterranean-Climate California, U.S.A. Freshw. Biol. 55, 188–204. doi:10.1111/j.1365-2427.2009.02379.x
Grantham, T. E., Viers, J. H., and Moyle, P. B. (2014). Systematic Screening of Dams for Environmental Flow Assessment and Implementation. BioScience 64, 1006–1018. doi:10.1093/biosci/biu159
Grill, G., Lehner, B., Thieme, M., Geenen, B., Tickner, D., Antonelli, F., et al. (2019). Mapping the World's Free-Flowing Rivers. Nature 569, 215–221. doi:10.1038/s41586-019-1111-9
Hayes, D. S., Brändle, J. M., Seliger, C., Zeiringer, B., Ferreira, T., and Schmutz, S. (2018). Advancing towards Functional Environmental Flows for Temperate Floodplain Rivers. Sci. Total Environ. 633, 1089–1104. doi:10.1016/j.scitotenv.2018.03.221
Herman, J., Fefer, M., Dogan, M., Jenkins, M., Medellín-Azuara, J., and Lund, J. R. (2018). Advancing Hydro-Economic Optimization to Identify Vulnerabilities and Adaptation Opportunities in California’s Water System: A Report for California’s Fourth Climate Change Assessment. Sacramento: California Natural Resources Agency.
Hidalgo, H. G., Das, T., Dettinger, M. D., Cayan, D. R., Pierce, D. W., Barnett, T. P., et al. (2009). Detection and Attribution of Streamflow Timing Changes to Climate Change in the Western United States. J. Clim. 22, 3838–3855. doi:10.1175/2009jcli2470.1
Huang, F., Li, F., Zhang, N., Chen, Q., Qian, B., Guo, L., et al. (2017). A Histogram Comparison Approach for Assessing Hydrologic Regime Alteration. River Res. Applic. 33, 809–822. doi:10.1002/rra.3130
John, A., Nathan, R., Horne, A., Stewardson, M., and Webb, J. A. (2020). How to Incorporate Climate Change into Modelling Environmental Water Outcomes: a Review. J. Water Clim. Change 11, 327–340. doi:10.2166/wcc.2020.263
Kakouei, K., Kiesel, J., Domisch, S., Irving, K. S., Jähnig, S. C., and Kail, J. (2018). Projected Effects of Climate-Change-Induced Flow Alterations on Stream Macroinvertebrate Abundances. Ecol. Evol. 8, 3393–3409. doi:10.1002/ece3.3907
Knowles, N., Cronkite‐Ratcliff, C., Pierce, D. W., and Cayan, D. R. (2018). Responses of Unimpaired Flows, Storage, and Managed Flows to Scenarios of Climate Change in the San Francisco Bay‐Delta Watershed. Water Resour. Res. 54, 7631–7650. doi:10.1029/2018wr022852
Kuriqi, A., Pinheiro, A. N., Sordo-Ward, A., and Garrote, L. (2019). Influence of Hydrologically Based Environmental Flow Methods on Flow Alteration and Energy Production in a Run-Of-River Hydropower Plant. J. Clean. Prod. 232, 1028–1042. doi:10.1016/j.jclepro.2019.05.358
Langhammer, J., and Bernsteinová, J. (2020). Which Aspects of Hydrological Regime in Mid-latitude Montane Basins Are Affected by Climate Change? Water 12, 2279. doi:10.3390/w12082279
Law, J. I. H. A. (2013). This Package Implements the Nature Conservancy’s Indicators of Hydrologic Alteration Software in R. Available at: https://rdrr.io/rforge/IHA/.
Liang, X., Lettenmaier, D. P., Wood, E. F., and Burges, S. J. (1994). A Simple Hydrologically Based Model of Land Surface Water and Energy Fluxes for General Circulation Models. J. Geophys. Res. 99, 14415–14428. doi:10.1029/94jd00483
Ligare, S. T., Viers, J. H., Null, S. E., Rheinheimer, D. E., and Mount, J. F. (2012). Non-uniform Changes to Whitewater Recreation in California's Sierra Nevada from Regional Climate Warming. River Res. Applic. 28, 1299–1311. doi:10.1002/rra.1522
Livneh, B., Bohn, T. J., Pierce, D. W., Munoz-Arriola, F., Nijssen, B., Vose, R., et al. (2015). A Spatially Comprehensive, Hydrometeorological Data Set for Mexico, the U.S., and Southern Canada 1950-2013. Sci. Data 2, 150042–150112. doi:10.1038/sdata.2015.42
Madani, K., and Lund, J. R. (2009). Modeling California’s High‐elevation Hydropower Systems in Energy Units. Water Resour. Res. 45. doi:10.1029/2008wr007206
Magilligan, F. J., and Nislow, K. H. (2005). Changes in Hydrologic Regime by Dams. Geomorphology 71, 61–78. doi:10.1016/j.geomorph.2004.08.017
Mann, H. B. (1945). Nonparametric Tests against Trend. Econometrica 13, 245–259. doi:10.2307/1907187
Maskey, M. L. (2021). A Generalized Package for Streamflow Bias Correction. Merced: VICE/Water Systems Management Lab, University of California.
Maskey, M. L., Puente, C. E., and Sivakumar, B. (2019). Temporal Downscaling Rainfall and Streamflow Records through a Deterministic Fractal Geometric Approach. J. hydrologyJ Hydrol 568, 447–461. doi:10.1016/j.jhydrol.2018.09.014
Nathan, R. J., McMahon, T. A., Peel, M. C., and Horne, A. (2019). Assessing the Degree of Hydrologic Stress Due to Climate Change. Climatic Change 156, 87–104. doi:10.1007/s10584-019-02497-4
Noaa., Home. (2017). NOAA Physical Sciences Laboratory. Available at: https://psl.noaa.gov/(accessed May 1, 2021).
Null, S. E., Ligare, S. T., and Viers, J. H. (2013). A Method to Consider whether Dams Mitigate Climate Change Effects on Stream Temperatures. J. Am. Water Resour. Assoc. 49, 1456–1472. doi:10.1111/jawr.12102
Obegi, D., and Wearn, A. (2016). SAVING THE LOWER SAN JOAQUIN RIVER AND ITS TRIBUTARIES. New York: Natural Resources Defense Council.
Olden, J. D., and Poff, N. L. (2003). Redundancy and the Choice of Hydrologic Indices for Characterizing Streamflow Regimes. River Res. Applic. 19, 101–121. doi:10.1002/rra.700
Pachauri, R. K., and Reisinger, A. (20072007). IPCC Fourth Assessment Report. Geneva, Switzerland: IPCC Geneva.
Peterman, W. (2002). DEM of California, USA | Data Basin. 90 M DEM Calif USA Data Basin. Available at: https://databasin.org/datasets/78ac54fabd594db5a39f6629514752c0/(accessed May 11, 2021).
Pettit, N. E., Froend, R. H., and Davies, P. M. (2001). Identifying the Natural Flow Regime and the Relationship with Riparian Vegetation for Two Contrasting Western Australian Rivers. Regul. Rivers: Res. Mgmt. 17, 201–215. doi:10.1002/rrr.624
Poff, N. L., Allan, J. D., Bain, M. B., Karr, J. R., Prestegaard, K. L., Richter, B. D., et al. (1997). The Natural Flow Regime. BioScience 47, 769–784. doi:10.2307/1313099
Poff, N. L. (2002). Ecological Response to and Management of Increased Flooding Caused by Climate Change. Phil. Trans. R. Soc. Lond. Ser. A: Math. Phys. Eng. Sci. 360, 1497–1510. doi:10.1098/rsta.2002.1012
Poff, N. L., and Zimmerman, J. K. H. (2010). Ecological Responses to Altered Flow Regimes: a Literature Review to Inform the Science and Management of Environmental Flows. Freshw. Biol. 55, 194–205. doi:10.1111/j.1365-2427.2009.02272.x
Puente, C. E., Maskey, M. L., and Sivakumar, B. (2017). Combining Fractals and Multifractals to Model Geoscience Records. Fractals Concepts Appl. Geosci.. Boca Raton: CRC Press, 297–332. doi:10.1201/9781315152264-11
Rheinheimer, D. E., and Viers, J. H. (2015). Combined Effects of Reservoir Operations and Climate Warming on the Flow Regime of Hydropower Bypass Reaches of California's Sierra Nevada. River Res. Applic. 31, 269–279. doi:10.1002/rra.2749
Richter, B. D., Baumgartner, J. V., Braun, D. P., and Powell, J. (1998). A Spatial Assessment of Hydrologic Alteration within a River Network. Regul. Rivers: Res. Mgmt. 14, 329–340. doi:10.1002/(sici)1099-1646(199807/08)14:4<329::aid-rrr505>3.0.co;2-e
Richter, B. D., Baumgartner, J. V., Powell, J., and Braun, D. P. (1996). A Method for Assessing Hydrologic Alteration within Ecosystems. Conservation Biol. 10, 1163–1174. doi:10.1046/j.1523-1739.1996.10041163.x
Swrcb, S. W. R. C. B. (2018). Water Quality Control Plan for the San Francisco Bay/Sacramento-San Joaquin Delta Estuary. Sacramento: State Water Resources Control Board.
Tian, X., Zhao, G., Mu, X., Zhang, P., Tian, P., Gao, P., et al. (2019). Hydrologic Alteration and Possible Underlying Causes in the Wuding River, China. Sci. Total Environ. 693, 133556. doi:10.1016/j.scitotenv.2019.07.362
Tomlinson, J. E., Arnott, J. H., and Harou, J. J. (2020). A Water Resource Simulator in Python. Environ. Model. Softw. 126, 104635. doi:10.1016/j.envsoft.2020.104635
Vicuña, S., Dracup, J. A., and Dale, L. (2011). Climate Change Impacts on Two High-Elevation Hydropower Systems in California. Clim. Change 109, 151–169.
Xue, L., Zhang, H., Yang, C., Zhang, L., and Sun, C. (2017). Quantitative Assessment of Hydrological Alteration Caused by Irrigation Projects in the Tarim River basin, China. Sci. Rep. 7, 1–13. doi:10.1038/s41598-017-04583-y
Yan, Y., Yang, Z., Liu, Q., and Sun, T. (2010). Assessing Effects of Dam Operation on Flow Regimes in the Lower Yellow River. Proced. Environ. Sci. 2, 507–516. doi:10.1016/j.proenv.2010.10.055
Yang, T., Cui, T., Xu, C.-Y., Ciais, P., and Shi, P. (2017). Development of a New IHA Method for Impact Assessment of Climate Change on Flow Regime. Glob. Planet. Change 156, 68–79. doi:10.1016/j.gloplacha.2017.07.006
Yang, Y., Yang, Z., Yin, X.-a., and Liu, Q. (2018). A Framework for Assessing Flow Regime Alterations Resulting from the Effects of Climate Change and Human Disturbance. Hydrological Sci. J. 63, 441–456. doi:10.1080/02626667.2018.1430897
Yarnell, S. M., Petts, G. E., Schmidt, J. C., Whipple, A. A., Beller, E. E., Dahm, C. N., et al. (2015). Functional Flows in Modified Riverscapes: Hydrographs, Habitats and Opportunities. BioScience 65, 963–972. doi:10.1093/biosci/biv102
Yarnell, S. M., Stein, E. D., Webb, J. A., Grantham, T., Lusardi, R. A., Zimmerman, J., et al. (2020). A Functional Flows Approach to Selecting Ecologically Relevant Flow Metrics for Environmental Flow Applications. River Res. Applic 36, 318–324. doi:10.1002/rra.3575
Yarnell, S. M., Viers, J. H., and Mount, J. F. (2010). Ecology and Management of the spring Snowmelt Recession. Bioscience 60, 114–127. doi:10.1525/bio.2010.60.2.6
Keywords: river regulation, indicators of hydrologic alteration, climate change, hydropower, san joaquin river system
Citation: Maskey ML, Facincani Dourado G, Rallings AM, Rheinheimer DE, Medellín-Azuara J and Viers JH (2022) Assessing Hydrological Alteration Caused by Climate Change and Reservoir Operations in the San Joaquin River Basin, California. Front. Environ. Sci. 10:765426. doi: 10.3389/fenvs.2022.765426
Received: 27 August 2021; Accepted: 11 February 2022;
Published: 03 March 2022.
Edited by:
Eric D. Stein, Southern California Coastal Water Research Project, United StatesReviewed by:
Xiaoduo Pan, Institute of Tibetan Plateau Research, (CAS), ChinaAvril C Horne, The University of Melbourne, Australia
Copyright © 2022 Maskey, Facincani Dourado, Rallings, Rheinheimer, Medellín-Azuara and Viers. This is an open-access article distributed under the terms of the Creative Commons Attribution License (CC BY). The use, distribution or reproduction in other forums is permitted, provided the original author(s) and the copyright owner(s) are credited and that the original publication in this journal is cited, in accordance with accepted academic practice. No use, distribution or reproduction is permitted which does not comply with these terms.
*Correspondence: Mahesh L. Maskey, bW1hc2tleUB1Y21lcmNlZC5lZHU=