- Department of Civil and Environmental Engineering, National University of Singapore, Singapore, Singapore
In recent years, increasing attention has been given for reclamation and reuse of water (wastewater and stormwater) in the context of augmenting water supplies. Constructed wetland (CW) systems make use of natural substrates, plants, and microbes for decontamination of wastewater and stormwater. These nature-based water treatment systems are cost-effective and sustainable. This review critically analyzes the recent advances on the application of CW systems for removal of total suspended solids (TSS), various chemical (nutrients including total nitrogen and total phosphorus, heavy metals, and organics) and microbial pollutants (Escherichia coli, enterococci, fecal coliforms, etc.) in wastewater and stormwater. Furthermore, the influence of key factors including CW configurations, substrates, vegetation, ambient temperature/seasonal changes, oxygen levels and hydraulic retention time on the performance of CW systems are discussed. Insights into various pollutant removal mechanisms, microbial diversity and modeling (kinetics, hydrological and mechanistic) are provided. CW systems show good performance for removal of diverse pollutants from wastewater and stormwater. The pollutant removal mechanisms include physical (sedimentation and filtration), chemical (sorption, complexation and precipitation) and biological (biodegradation, microbial transformation and microbial/plant assimilation) processes. The dominant microbial communities enriched in CW systems include nitrifiers, denitrifiers and organic biodegraders. The key knowledge gaps in the development of multifunctional CW systems are highlighted. We believe that this critical review would help urban planners, environmental engineers and managers with implementation of innovative strategies for wastewater and stormwater reclamation and reuse to alleviate water stress in urban areas and to contribute to environmental sustainability. Moreover, this review would help to optimize the performance of CW systems as well as to develop regulatory guidelines for installation, operation and maintenance of CW systems.
Introduction
The level of water consumption rate has significantly increased in cities due to urbanization, industrialization, and population growth. This increase in turn exerts unprecedented pressures on water resources (e.g., water scarcity and water pollution management) (Ghennandi et al., 2007). Moreover, the use of water for various domestic and industrial activities has led to generation of a high volume of wastewater. It remains a great challenge to decontaminate wastewater to reduce pollutant concentrations below the water quality standard specified by the local regulatory agencies prior to its discharge to receiving water bodies and/or reuse as non-potable water. The pollutant characteristics (the quality and quantity of pollutants) of wastewater mainly depend on their sources. The key pollutants detected in wastewater include nutrients (nitrogen and phosphorus), heavy metals (Cd, Cr, Cu, Hg, Pb, Ni, etc.), biodegradable organic materials and non-biodegradable organic materials (detergents, pesticides, fat, oil, grease, solvents, etc.) and microorganisms (pathogenic bacteria and viruses) (Henze and Comeau, 2008). The typical levels of total suspended solids (TSS), total nitrogen (TN) and total phosphorus (TP) in municipal wastewater are 250–600 mg/L, 30–100 mg/L and 6–25 mg/L, respectively (Henze and Comeau, 2008). Heavy metals are usually detected in the range of 0.003–1.0 mg/L, while microorganisms (e.g. coliform) are found between 1011 and 1013 colony forming units/mL (CFU/100 ml) (Henze and Comeau, 2008).
Wastewater reclamation, recycling and reuse are increasingly adopted specifically in urban areas to alleviate water stress and to contribute to sustainable management of water resources (Asano, 2005). Reclamation and reuse of treated wastewater at their source of generation is strongly encouraged since this approach uses simple and cost-effective water treatment technologies with minimal environmental impacts compared to the centralized water treatment system (House et al., 1999). The reclaimed wastewater can be reutilized for non-potable purposes including land irrigation, toilet flushing, car washing, and esthetic and recreational activities (Tao et al., 2017).
In addition to wastewater, reclamation and reuse of stormwater is considered as an important strategy in urban areas to reduce freshwater demand (Tao et al., 2017; Mark and Henriette, 2021). The stormwater characteristics (types of pollutants and their concentrations) vary depending on the types of landscapes/impervious surfaces in which rainwater flows (Song et al., 2019; Biswal et al., 2022). Rainfall characteristics such as rainfall intensity, duration and antecedent dry periods also affect the stormwater quality (Poudyal et al., 2021). Moreover, first flush positively influences the pollutants loads in stormwater (Kayhanian et al., 2012). Specifically, a strong first flush could lead to a maximum washout and discharge of pollutants (up to 40% by mass) to the initial runoff (Han et al., 2006). Among various land uses, the concentration of pollutants in stormwater such as heavy metals and polycyclic aromatic hydrocarbons (PAHs) is usually higher in highways and car parking areas than others (residential, industrial, road, and commercial runoff) (Lau et al., 2009; Song et al., 2019). Pollutants in runoff are detected in two forms namely particulate and/or dissolved forms (Kayhanian et al., 2012). In urban runoff (with atmospheric deposition), among heavy metals, Zn is predominant in large particles (>10 µm), whereas Cu, Cd, Ni, and Pb are mainly present in small particles (<10 µm) (Gunawardena et al., 2013). Kayhanian et al. (2012) also reported that heavy metals are highly concentrated in smaller particles, e.g., in highway stormwater sediment, the concentration of Zn was 1,189 µg/g in sediments with size ranging from 25–38 µm, but a lower concentration of Zn (259 µg/g) was observed in larger size sediments (850–2000 µm). The typical level of TSS, TN and TP in stormwater ranges from 11–430 mg/L, 0.3–2.74 mg/L and 0.16–3.52 mg/L, respectively (Biswal et al., 2022). Heavy metals (Zn, Cu, and Pb) are detected between 2 and 730 µg/L, while organics (e.g., PAHs and PCBs (polychlorinated biphenyls) and microbial pollutants (e.g., fecal coliform) are usually found in very low levels (mainly ng/L) up to 104 CFU/100 ml, respectively (Biswal et al., 2022).
Prior to the reuse of wastewater and stormwater as a non-potable water resource (recreational and/or environmental applications), it is required to treat the water to reduce contaminants concentration to the levels set by the local government authorities. Moreover, the discharge of untreated wastewater and stormwater to the receiving aquatic systems could degrade water quality, leading to eutrophication due to high concentrations of inorganic nutrients in the untreated wastewater/stormwater and thus pose risks to aquatic organisms (Chislock et al., 2013). Reclamation and reuse of water has many benefits including the recycling of nutrients and water to the land, rebuild stream flows and support life in aquatic ecosystems (Greenway, 2005).
CW systems are considered as effective, low-cost, environmentally-friendly and sustainable technologies for treatment of various types of wastewater (e.g., municipal, domestic, agricultural and industrial wastewaters), stormwater and even treatment of sludge (dewatering and drying) (Stefanakis, 2016; Dotro et al., 2017; Tao et al., 2017). CW systems are also regarded as natural treatment systems (eco-technology) which use natural materials (sand, gravel and vegetation) and naturally occurring processes (plant- and microorganisms-driven bioprocesses) to decontaminate polluted waters (Stefanakis, 2016). In addition to water/wastewater treatment, CW acts as flood control systems in terms of receiving and storing excess runoff in urban areas (Stefanakis, 2016). Moreover, CW provides several ecosystems services/ecological benefits including enhanced biodiversity, increased environmental aesthetic values, improved air quality, carbon sequestration, reduced urban heat island effects, etc. (Moore and Hunt, 2012). The land requirements, operational and maintenance costs of CW systems are low compared to the conventional water and wastewater treatment technologies (Vymazal et al., 2006).
CW systems are considered to be the important promising step of conventionally treated wastewater for its reuse (Ghennandi et al., 2007). Several studies have reported that CW systems integrated with solar/UV(ultraviolet) light-based disinfection, photocatalysis and soil/sand filters, etc.) show better pollutant removal performance than a single CW system (Chen et al., 2011; Álvarez et al., 2017; Russo et al., 2019). The integrated systems are highly effective for reduction of the levels of microbial pollutants (e.g., total coliforms, enterococci and Escherichia coli) in wastewater (Russo et al., 2019). The performance of CW systems depends on various factors including their configurations, inflow concentrations of pollutants, hydraulic loads, plant species types, etc. (Galletti et al., 2010; Liang et al., 2017).
Literature search in the scientific database (e.g., Scopus) over the last 10 years (2012–2021) (Supplementary Figure S1) shows that significant research has been conducted on the reclamation and reuse of wastewater/stormwater using CW systems. The publication data were extracted from the Scopus database using two key words namely “constructed wetlands” and “water reclamation” as the keyword in the Scopus document search engine. Since numerous scientific publications are available in literature on constructed wetlands for water reclamation and reuse, the following criteria were adopted to screen and select the most relevant and recent articles for inclusion in this review. The articles were collected from Google Scholar and Scopus database by using keywords relevant to the respective sections in the review. The articles which were published in the last 10 years (2012–2021) were considered for a critical analysis. Deeper analysis of the published articles illustrate that continual efforts have been made to understand various aspects of CW systems and explore improvement of these systems according to the changes of inflow wastewater/stormwater characteristics and climatic conditions. Most of the earlier reviews have focused on the potential reclamation of mainly wastewater (Tram VO et al., 2014; Wu et al., 2015; Tao et al., 2017). The pollutant loads in stormwater are relatively lower than those in wastewater, but limited information is available on the reclamation and reuse of stormwater. Since the characteristics pollutants and their loads in wastewater and stormwater are different, the treatment efficiency and associated processes in CW systems could be dissimilar. To our knowledge, an in-depth comparative evaluation of the effectiveness CW systems for decontamination wastewater and stormwater has never been attempted. In addition, there is a lack of insights into pollutant removal mechanisms, the influence of operational factors such as hydraulic loading rate, pollutant loads, hydraulic retention time, etc. and that of microbial diversity in CW facilities on the overall performance of CW systems. To make further advances with the design and configuration of this nature-based technology, this review critically analyzes the recent advancements of the performance of single and integrative CW systems for removal of diverse chemical and microbial pollutants from wastewater and stormwater. The influence of various operational and environmental parameters on the performance of CW systems is discussed. Insights into physicochemical and biological mechanisms involved in the removal of pollutants are provided. The diversity of microbial communities enriched in the CW systems is highlighted. Various types of modeling which are applied to describe the kinetics, hydrology and pollutant removal characteristics in CW are briefly presented. This review identifies the major knowledge gaps in the design and operation of comprehensive CW systems for decontamination of wastewater and stormwater, and recommends the potential research areas which are worthy of investigation in future. The review outcomes would help to design innovative and multifunctional CW systems with high potential to reclaim wastewater and stormwater and with resistance to changing climates. We believe this critical review would be of interest to water and wastewater professionals, environmental scientists and engineers and other stakeholders for selection of a suitable single CW system (surface or sub-surface wetland CW) or integrative units (hybrid CWs or CW plus supplementary physicochemical treatment systems) based on the wastewater/stormwater qualities and the target reuse purposes.
Classification of Constructed Wetland Systems
CW systems are classified into different types based on the hydrology, water flow direction and plant species diversity (Stottmeister et al., 2003; Herath and Vithanage, 2015) (Figure 1). In terms of water flow characteristics, CW systems are classified as free water surface (FWS) and subsurface flow (SF) CW systems. SF-based CW systems are further sub-divided into horizontal subsurface flow (HSF) and vertical flow (VF) units. However, based on the diversity of vegetation species, CW systems are categorized into three types namely 1) emergent macrophyte CW, 2) submerged macrophyte CW, and 3) floating treatment wetland (FTW) systems. Among the three types of CW systems, rooted emergent macrophytes are commonly used (Stefanakis, 2016). Integration of two or more different types of wetlands (e.g., surface plus sub-surface flow systems) is referred to as hybrid/combined systems. French VF-based CW systems provide both sludge and wastewater treatment (Dotro et al., 2017). CW systems are also classified as saturated and unsaturated systems. For example, free water surface or horizontal subsurface flow CWs are referred to saturated systems, whereas vertical flow CWs are called as unsaturated systems (Lyu et al., 2018). Between HSF and VF-based CW systems, VF is more effective as intermittent vertical flow increases dissolved oxygen levels in the soil media which in turn improve the pollutant removal efficiency through aerobic biodegradation (Brix, 1993). Among FWS and SF-based CW systems, the former type is largely used in Australia and North America (Vymazal, 2011), while the latter type is mainly applied in Europe and China since it needs less land requirements and shows high efficiency for removal of diverse pollutants (Wang P. et al., 2016). Hybrid constructed wetlands (mainly a combination of HSF—VF) are mostly used in Asia and Europe (Vymazal, 2013). The advantages and disadvantages of surface flow (FWS) and subsurface (horizontal and vertical) flow-based CW systems are presented in Table 1.
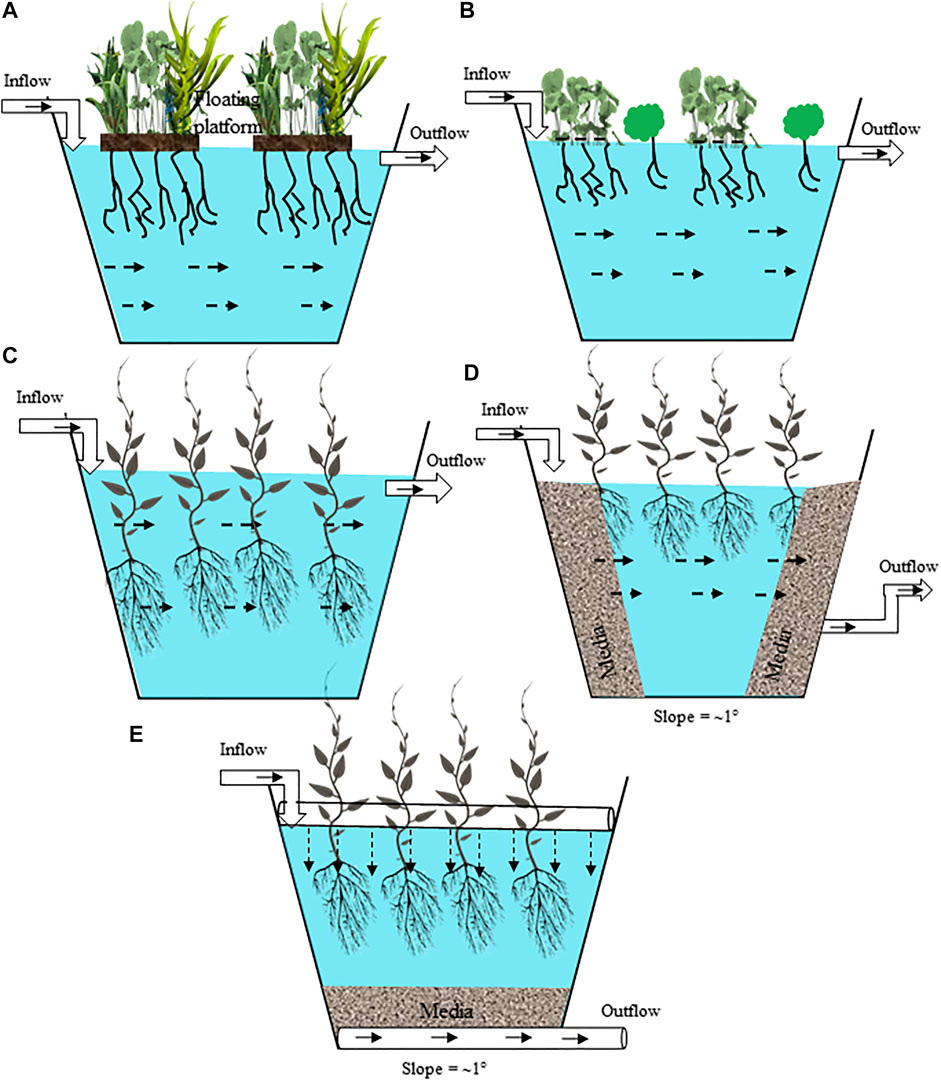
FIGURE 1. A schematic diagram of various types of constructed wetland (CW) systems. (A) Floating treatment wetland system, (B) Wetland with free-floating plants, (C) Horizontal surface flow/wetland with emergent plant system, (D) Horizontal subsurface flow wetland system, and (E) Vertical subsurface flow wetland system.
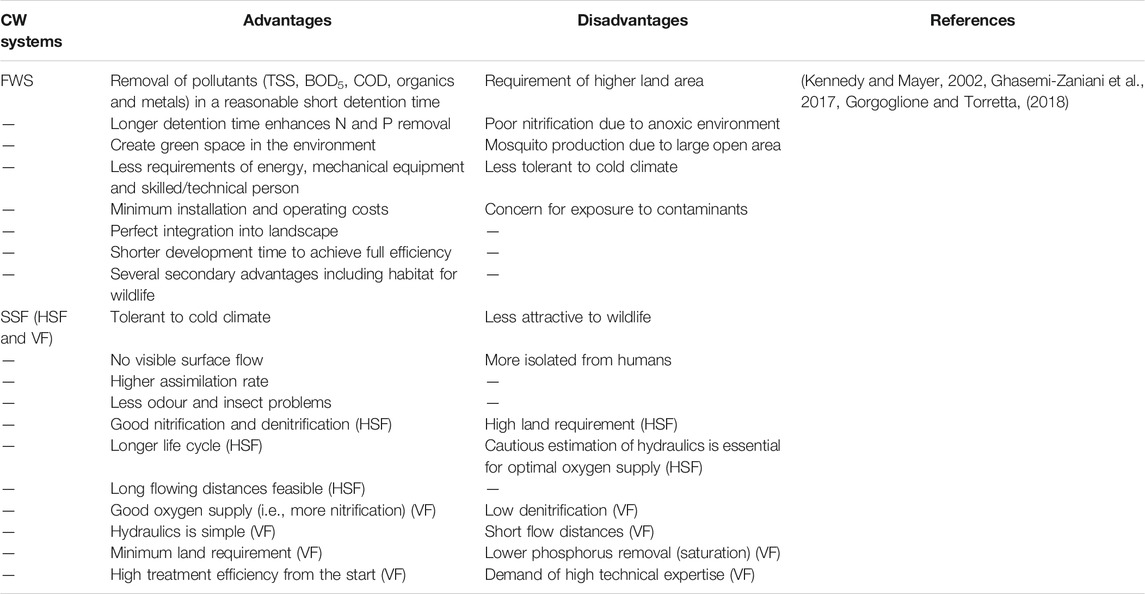
TABLE 1. Advantages and disadvantages of free water surface (FWS) and subsurface flow (SSF which include horizontal flow (HSF) and vertical flow (VF) constructed wetland (CW) systems for wastewater reclamation and reuse.
Wastewater Treatment by Constructed Wetland Systems
Removal of Total Suspended Solids (TSS) and Chemical Pollutants
CW systems, also known as biofilters, are capable of removal of total suspended solids (TSS) and several chemical pollutants including chemical oxygen demand (COD), biochemical oxygen demand (BOD5), nutrients including total nitrogen (TN) and total phosphorus (TP), heavy metals and organics from wastewater (Hench et al., 2003; Zhang et al., 2014) (Table 2). In a mesocosm (HSF)- and microcosm (VF)-based CW systems planted with emergent plants (e.g., Canna indica) and fed with saline wastewater, the removal of nitrogen and phosphorus was nearly 100% and 94–100% under low and high influent loads (Liang et al., 2017). In a laboratory-scale VF-based CW system which was vegetated with Cyperus alternifolius (umbrella grass), the CW achieved 35–60% removal of TN and 77–80% removal of COD after 23 days of operation (Bilgin et al., 2014). Giácoman-Vallejos et al. (2015) investigated the performance of an HSF-based CW system for treatment of domestic and swine wastewaters, and this system was more effective for decontamination of domestic wastewater than (COD: 75% and TSS: 84% removal) than swine wastewater (COD: 72% and TSS: 78% removal). Xu et al. (2009) explored the feasibility of reclamation of steel wastewater using vertical flow CW supplemented with manganese ore as one of the media components, and authors have found that the system was effective for removal of various pollutants including COD (55%), ammonia (67%) and TP (93%) which was higher than the performance of gravel-based CW system (COD: 31%, ammonia: 58% and TP: 78%).
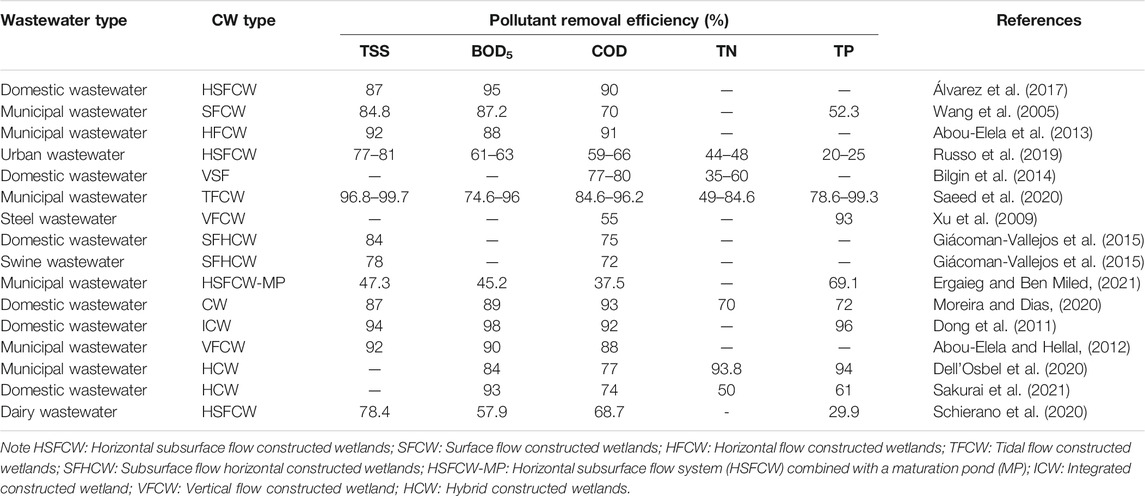
TABLE 2. Performance of various types of constructed wetlands (CW) for removal of TSS and diverse chemical pollutants from wastewater. The detailed information is provided in supplementary material (Supplementary Table S1).
In a hybrid system consisting of a HSF-based CW system with a maturation pond, the removal of TSS, BOD5, COD, TKN and TP from secondary municipal wastewater was 47, 45, 38, 57 and 69%, respectively (Ergaieg and Ben Miled, 2021). In another study which explored the performance of a pilot-scale VF and HSF-based CW hybrid systems, it was found that the hybrid units were effective for removal of various pollutants from secondary effluent from a wastewater treatment plant, i.e. the reduction of concentrations of NH4+-N, TN, TP and COD was 82, 70, 78 and 32.3%, respectively (Xu et al., 2016). A recent study on wastewater treatment using a hybrid constructed wetland (combination of multiple systems including floating treatment wetlands, HSF and VF CW), the combined unit shows high performance for removal of diverse pollutants including COD (77%), BOD5 (84%), TN (93.8%) and TP (94%) (Dell’Osbel et al., 2020). Sakurai et al. (2021) showed that a hybrid system which consists of HSF and VF CW system was capable of reduction various pollutants from anaerobically digested blackwater, i.e., the system achieved 74% COD, 93% BOD5, 50% TN, and 61% TP reduction. Moreover, the hybrid wetland showed high removal (93%) of antibiotics (ciprofloxacin). According to Vymazal (2013), hybrid CW systems demonstrate higher treatment performance than single units specifically for the removal of TN.
Removal of Microbial Pollutants
Microbial contaminants namely fecal coliforms, enterococci, coliphage and pathogens (Salmonella, Shigella, etc.) are frequently detected in wastewater, which pose detrimental effects on public health (Naidoo and Olaniran, 2014). Several studies have been conducted to examine the effectiveness of CW systems for removal of both fecal indicator organisms and pathogens. In an HSF wastewater wetland system planted with Typha domingensis, the reduction of fecal coliform was one order of magnitude, i.e., the concentration of fecal coliform in influent and effluent was 1.5 × 103 and 6.0 × 102, respectively (Schierano et al., 2020). However, no consistent results were reached on the removal of Escherichia coli and Pseudomona aeruginosa since the two bacteria were detected in multiple effluent samples collected at various time intervals. According to a recent study on an integrated system containing HSF-VF CW systems fed with wastewater from a local wastewater treatment plant, the hybrid system was efficient with the reduction of the concentration of pathogenic bacteria including E. coli and total coliforms (99% or ∼4.5 log10 units) (Vega De Lille et al., 2021). García et al. (2013) evaluated the performance of a hybrid system (VF and HSF combination) for removal of pathogens in a tropical climatic environment, and the system achieved a high removal of E. coli (3 log units), total coliform (4 log units) and Helminth eggs (90%). Alufasi et al. (2017) studied the occurrence and removal of various microbial pollutants including enteric bacteria in both water and sediments of surface flow constructed wetlands. The die-off rates (log10 per day) of fecal coliforms and Salmonella typhimurium in water were 0.256 and 0.345, respectively, whereas in sediment, the die-off rates were 0.151 and 0.312, respectively. Additionally, the die-off rates (log10 per day) of coliphage and Giardia in water were 0.397 and 0.029, respectively, while in sediments, the die-off rates were 0.107 and 0.37, respectively (Alufasi et al., 2017). In HSF-based CW systems vegetated with two macrophytes namely Typha dominguensis and Typha latifolia, the removal efficiencies for faecal coliform and total coliform from domestic and swine wastewater were 86–91% and 80–82%, respectively (Giácoman-Vallejos et al., 2015).
A recent study on hybrid systems (HSF-based CW with maturation pond) revealed that the removal of fecal bacteria including fecal coliform (FC) and fecal streptococci (FS) from municipal secondary wastewater varied between 1.13 and 0.59 log units, respectively (Ergaieg and Ben Miled, 2021). Lamori et al. (2019) applied two types of microbiological methods for quantification of the removal of faecal indicator bacteria from wastewater in CW systems, and the wetlands achieved a 50% reduction of E. coli as evaluated by a culture-based technique. However, a quantitative polymerase chain reaction (qPCR)-based analysis showed only 6.7% reduction of E. coli. Additionally, the concentration of Enterococcus and Human-associated Bacteroidales (HF183) decreased by 84 and 67%, respectively. A combined system (ponds plus surface flow CW) treating municipal wastewater demonstrated high performance for reduction of concentration of faecal coliforms (>99.97%) and total bacteria (>99.998%) (Wang et al., 2005). The removal of microbial pollutants from wastewater by CW systems with different configurations is summarized in Table 3. Overall, the results of these studies indicate that the removal of microbial pollutants by different CW systems was different. This difference is potentially due to differences in the configuration of CW systems (e.g., planting schemes including plant species diversity and density and media components), hydrology and initial microbial concentration as well as survivability and physiology (sizes and cell properties namely hydrophobicity) of microbes.
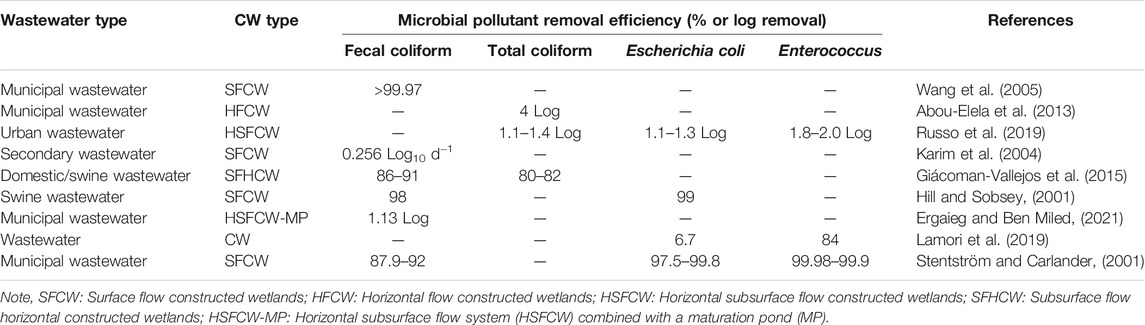
TABLE 3. Performance of various types of constructed wetlands (CW) for removal of diverse microbial pollutants from wastewater. The detailed information is provided in supplementary material (Supplementary Table S2).
Stormwater Treatment by Constructed Wetland Systems
Removal of Total Suspended Solids (TSS) and Chemical Pollutants
The performance of single and hybrid CW systems for the removal of diverse chemical pollutants from stormwater has been reported in literature (Headley and Tanner, 2012; Malaviya and Singh, 2012) (Table 4). Schmitt et al. (2015) reported that the stormwater pollutant removal performance of a hybrid CW system consisting of sedimentation pond (SP) and a SFCW was very high, i.e., >90% TSS, 70–98% COD, > 79% TN and >77% TP. In a pilot-scale HSF CW system treating agricultural runoff, the decontamination efficiency of stormwater wetland for various pollutants was very high, i.e., 74% COD, 80% BOD5, 57% TSS, 80% TP and 55% TN reduction was noticed (Grinberga et al., 2021). Choi et al. (2021) employed a HSF CW system for treatment of runoff from road and parking areas, and the stormwater wetland was effective for removal of various key chemical contaminants namely TSS (71.2%), COD (57.2%), nutrients including TN (∼46%) and TP (∼55%) (Choi et al., 2021). In a field-scale study, it was observed that with integration of a floating treatment wetland (FTW) system with a typical treatment pond, the pollutant removal performance was good (41% TSS, 40% Zn and 39% Cu reduction) (Borne et al., 2013). Kabenge et al. (2018) explored the performance of a microcosm subsurface VF-based CW system vegetated with two different plants (Typha latifolia and Scirpus lacustris) for the removal of diverse chemical pollutants in runoff from a parking yard, and the removal of COD, TN and TP was 76, 73 and 63%, respectively. In another field-scale CW system, Hafeznezami et al. (2012) explored removal of four heavy metals (Cd, Cu, Zn and Pb) from runoff, and the percentage of removal of Cd, Zn and Cu was 24, 18 and 11%, respectively. The reduction of Pb was insignificant which was possibly due to its low concentration in the influent. In an integrated constructed wetland system fed with runoff from farmyard, the system showed high capability of removal of diverse pollutants including TSS (93.7%), COD (94.9%), BOD5 (97.6%), ammonia (99%), nitrate (74%) and molybdate reactive phosphorus (91.8%) (Mustafa et al., 2009). Together, a number of studies reported a high percentage removal of key pollutants namely TSS, TN and TP in urban stormwater by CW systems (Choi et al., 2021; Dharmasena et al., 2021; Grinberga et al., 2021). Moreover, the effectiveness of CW systems for the removal of organic pollutants [PAHs, PCBs and emerging contaminants including pharmaceutical and personal care products (PPCPs)] is not fully understood yet which should be investigated in future research.
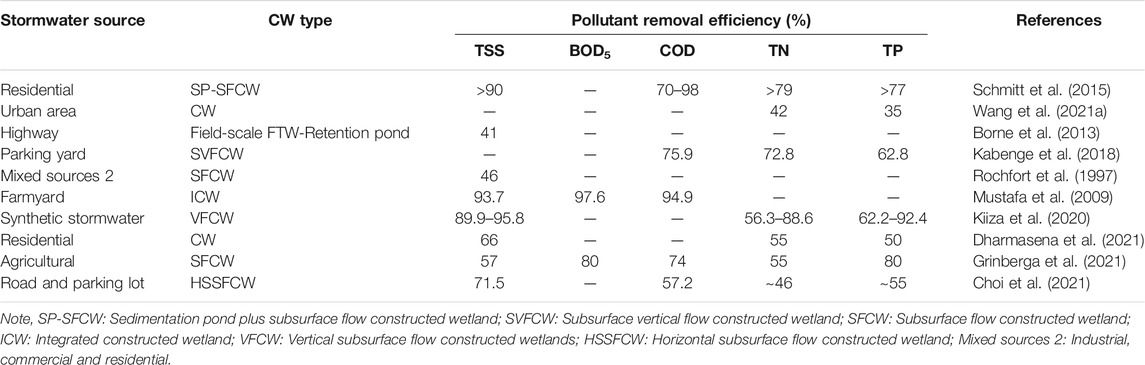
TABLE 4. Performance of various types of constructed wetlands (CW) for removal of TSS and diverse chemical pollutants from stormwater. The detailed information is provided in supplementary material (Supplementary Table S3).
Removal of Microbial Pollutants
The microbial loads in stormwater could deteriorate the quality of receiving water bodies. The performance of CW systems for removal of microbial contaminants in stormwater has been investigated in many studies (Davies and Bavor, 2000; Meng et al., 2018). In an Australian study, it was observed that the surface-flow-based CW system was effective for the removal of E. coli (average log removal: 0.96; range: 0.19–1.79). However, higher concentration of a pathogenic bacterium (Campylobacter spp.) was noticed in the effluent than in the influent (average log removal: 0.05; range: -0.9–1.25) (Meng et al., 2018). The authors explained that the higher concentration Campylobacter spp. In the effluent was possibly due to external deposition of waterfowl faeces. The treated stormwater was not suitable for reuse since the concentration of both microorganisms (E. coli: 4950 MPN/L and Campylobacter spp.: 832 MPN/L) in the effluent was above the Australian Guidelines for Water Recycling (<1.0 × 102 MPN/L) (Meng et al., 2018). Among the various CW configurations, the HSF-based CW system shows higher performance for the reduction of microbial pollutants compared to the FWS-based CW system. However, hybrid wetland systems are the most effective for removal of microbes which is possibly due to a longer hydraulic retention time (Wu et al., 2016). A CW system designed for treatment of residential runoff showed higher removal of Enterococci (49–61%) than E. coli (–15–45%) (Hathaway et al., 2009c). The negative removal refers to higher concentration of E. coli in the effluent than in the influent which could be due to the intrusion of microbial contaminants into CW systems by external sources. Hathaway et al. (2009) monitored the performance of two stormwater constructed wetlands for the removal of E. coli and Enterococci, and the removal of E. coli varied between 33–96%, while the reduction of Enterococci ranged between 56–98%. The lower performance of the CW system for the removal of E. coli is thought to be due to the lack of appropriate vegetation species in the CW.
Méndez et al. (2009) applied various stormwater quality improvement devices (SQIDs) including a SF CW system for the removal of fecal coliforms in stormwater, and observed that the fecal coliform concentration decreased by 20–98% under various rainfall event intensities (Méndez et al., 2009). A recent study evaluated the performance of two detention basins which were incorporated with CW for the removal of indictor bacteria (E. coli) from urban runoff, and the removal of E. coli was very low in one of the detention facilities (only 4% reduction), while in another basin, a high variation of E. coli removal was noticed (Guerrero et al., 2020). In an integrated constructed wetland system, the removal of total coliforms and E. coli from farmyard runoff was 99.2 and 99.9%, respectively (Mustafa et al., 2009). In total, limited studies have been conducted on the evaluation of the performance of CW systems for removal of fecal indicator bacteria and pathogens from surface runoff. The removal of microbial pollutants from stormwater in various CW systems is summarized in Table 5. The results of the above studies indicate that CW systems are in general effective for removal of microbial pollutants. However, the percentage removal among microbes varies which is possibly due to different microbes possessing different traits for their growth and survival. They may also have different adsorption affinities towards the wetland substrates which may be mainly responsible for variations in the removal of microorganisms.
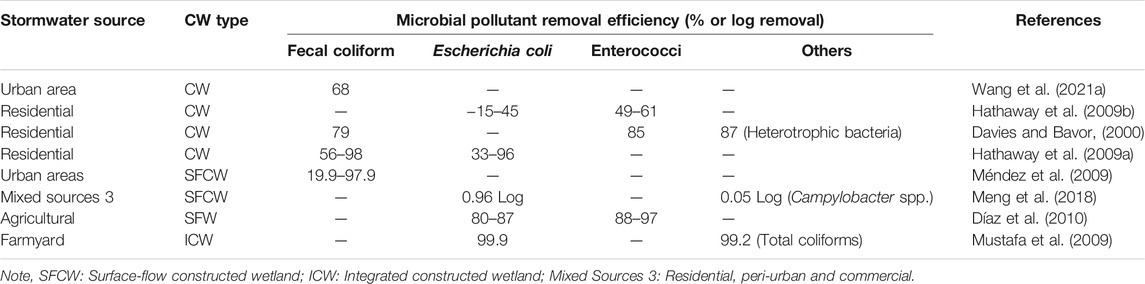
TABLE 5. Performance of various types of constructed wetlands (CW) for removal of diverse microbial pollutants from stormwater. The detailed information is provided in supplementary material (Supplementary Table S4).
Discussion on the Quality of Constructed Wetland Effluent for Potential Reuse or Discharge Into Environment
Based on the quality of effluents produced from constructed wetlands, the reclaimed water can be reused for various purposes such as support of recreational activities (swimming pools, sports events, etc.), discharge into agricultural land for crop production, augmentation of freshwater (rivers, lakes, ponds, etc.) and groundwater (aquifer recharge) (Tao et al., 2017; Almuktar et al., 2018). Several studies have reported that constructed wetland effluent met the local water quality standard for recreational/agricultural reuse or discharge into surface waters (Díaz et al., 2010; Trang et al., 2010; Al-Wahaibi et al., 2021). Díaz et al. (2010) found that the concentration of E. coli in the effluent of surface flow wetlands was mostly (nearly 93% of the sampling time) below the USEPA standard for recreational water (i.e., 126 CFU/100 ml), while that of enterococci was below the standard (33 CFU/100 ml) only 30% of the sampling time. A recent study on a full-scale vertical flow CW indicated that with step feeding of external carbon, the nitrate concentration in the effluent was below the Oman irrigation standard (11.3 mg NO3-N/L) (Al-Wahaibi et al., 2021). In pilot-scale horizontal subsurface flow CW systems treating municipal wastewater, the treated effluent from wetlands was reported to satisfy most of the Vietnamese national standards (except ammonia and TN) for discharge of treated effluent into surface waters (Trang et al., 2010). Another pilot-scale study on a multistage horizontal subsurface flow CW fed with raw urban wastewater and operated with two different water heights (10 vs. 40 cm), it was observed that the treatment efficiency of CW was higher when it was run at low water height (10 cm) (Herrera-Melián et al., 2020). The treated effluent (e.g., COD, TSS, turbidity and E. coli concentration) showed high degree of compliance to the Spanish National Regulation for discharge of treated effluent into the environment and possible reuse of treated effluent for various purposes (Herrera-Melián et al., 2020). Lu et al. (2016) investigated the impacts of various filter media (maifanite, steel slag, bamboo charcoal and limestone) in vertical upward-flow subsurface-flow CW for treatment of rural household sewage. Among the four media materials used, maifanite stone demonstrated a high pollutant removal performance and the treated effluent met the Chinese discharge standard (GB18918-2002). Another study on a combined system consisting of ponds and a surface flow constructed wetland, the concentration of faecal coliforms and total bacteria in the CW effluent were below the Chinese National standard for discharge of treated effluent from municipal wastewater treatment plant (Wang et al., 2005).
Comparison of Effectiveness of CW Systems for the Removal of Pollutants From Wastewater and Stormwater
For statistical evaluation of the performance of CW systems for the removal of pollutants from wastewater and stormwater, a Box and Whisker plot was generated (Figure 2) using the treatment efficiency data presented in Table 2 (wastewater) and Table 4 (stormwater). For statistical analysis, only TSS and chemical pollutants are selected since limited studies have been performed on the removal of microbial pollutants specifically from stormwater in CW systems. Four key pollutants namely COD, TSS, TN and TP are chosen since most of the studies have monitored the performance of wastewater wetlands and stormwater wetlands for removal of these four pollutants. The data were analyzed using the Student’s t-test at p value of 0.05 to evaluate whether the pollutant treatment efficiency between wastewater CW and stormwater CW is statistically significant. The analysis shows that CW systems show similar performance (except TSS) for decontamination of wastewater and stormwater since the t-test computation revealed that the difference of pollutant removal efficiency between wastewater CW and stormwater CW is not statistically significant (p > 0.05). CW systems demonstrate a slightly higher performance for the removal of TSS from wastewater than the ones used for the treatment of stormwater (statistically not significant, p > 0.05). Future studies are warranted to elucidate the potential reasons for the better TSS removal performance in wastewater CW systems.
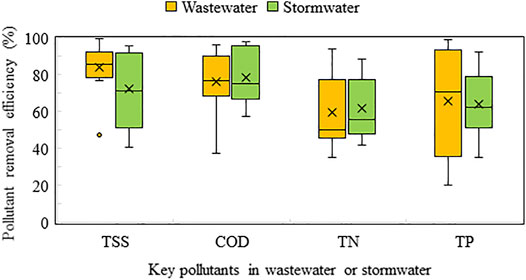
FIGURE 2. Box and Whisker plot showing comparative evaluation on the removal of TSS and chemical pollutants (COD, TN and TP) from wastewater and stormwater in constructed wetland systems. The box represents the interquartile range (IQR), minimum and maximum values (whisker), median value (line inside the box), the mean value (cross inside the box), outliers (dot, outside of box).
Our literature review analysis shows that the selection of a specific type of CW systems depends on multiple factors including the quantity of wastewater/stormwater to be treated, the quality of wastewater/stormwater (concentrations of TSS, chemical and microbial pollutants), the target effluent quality (e.g., water quality standard set by the local authorities) to be achieved, prevailing local climatic conditions (hot, arid, semi-arid, cold, tropical, etc.), the cost of the CW systems and the ease of their operation and maintenance, etc.
Microbial Diversity in Constructed Wetland Systems
The major sources of microorganisms into constructed wetlands include the inflow of wastewater/stormwater, substrate media and the surrounding environment (e.g., soil and atmosphere). The microorganisms present in the substrate layer (e.g., soil matrix) and/or biofilm developed in the plant root zones play a critical role in the removal of pollutants in CW systems (Despland et al., 2014; Lv et al., 2017). Thus, the presence of stable and healthy microbial community could provide optimum pollutant removal efficiency in long-term operation. Most of the studies have used 16S rRNA gene amplicon sequencing method for microbial characterization as well as to understand the richness, diversity, composition of enriched microbial communities as well as microbial activity and metabolic pathways in the CW systems (Arroyo et al., 2013; Sánchez, 2017). Internal factors (e.g., pH, substrate media composition and their depth, the presence/absence of vegetation, the quantity and quality of pollutants, availability of organic carbon) and external factors (e.g., ambient temperature and Sun light) could impact the CW microenvironments, and thus change the microbial flora in CW ecosystems (Verduzo Garibay et al., 2021). The dynamics of microbial communities enriched in CW ecosystems treating wastewater and stormwater are briefly described below.
Microbial Community Dynamics in CW Systems Treating Wastewater
The microbial diversity in CW systems for treatment of wastewater could vary depending on the nature of wastewater (e.g., domestic, industrial, agriculture, poultry farm, etc.) to be treated in CW. Herein, the results of a few reports on dynamics of microbial communities in CW treating wastewater are critically analyzed. In SF-based CW systems treating swine waste, the four key phyla in the communities were Proteobacteria (34.94%), Bacteroidetes (22.04%), Firmicutes (9.86%) and Cyanobacteriachloroplast (6.22%) which indicate that Proteobacteria and Bacteroidetes accounted for the majority of bacteria (more than 50%) in the CW system (Ibekwe et al., 2016). Additionally, statistical computation (e.g., Canonical correspondence analysis) indicates that ammonia and phosphate significantly impact microbial dynamics in wetland systems. Despland et al. (2014) found that the diversity of microbial communities enriched in soil (bottom) and Bauxsol™ pellet (top) layers in an unplanted CW was different, i.e., ammonia oxidizing bacteria and archaea were detected in soil layer. However, aerobic ammonia-oxidizing bacteria, anaerobic ammonia-oxidizing bacteria (anammox) and denitrifying microbes were found in Bauxsol™ pellets. These results indicate that the oxygen gradient profile from top (aerobic) to bottom (anoxic/anerobic) in the substrate could shape the structure of CW microbiome and their functions. Ruppelt et al. (2020) observed the highest microbial activity (e.g., fluorescence diacetate hydrolysis) in the top layer of a long filter media within the depth of 0.75 m. However, relatively uniform microbial activity was noticed in a relatively shorter media filter (0.50 m deep) of VF-based CW systems. Moreover, the phyla namely Proteobacteria and Actinobacteria were the major bacteria in the CW media filter, but based on the taxonomic classification at the genus level, the abundance of genus Flavobacterium columnare was higher in the long filter. A recent work analyzed microbial communities in different zones of a FWS CS system treating wastewater, and revealed the high abundance of organic pollutants degrading microbes namely Smithella, Ignavibacterium, and Methanothrix in phytofilters, and the low abundance of Parcubacteria, Proteiniclasticum and Macellibacteroides in the sedimentation tank (Semenov et al., 2020).
The planted vegetation could also influence the abundance of microbial communities in CW systems. In general, vegetation enhances microbial activity and metabolic richness in the biofilm (Lv et al., 2017). Wang et al. (2016) reported that in a CW planted with Iris pseudacorus, two phyla namely Proteobacteria (51%) and Bacteroidetes (34%) accounted for the total bacterial population, while Proteobacteria (72%) and Bacteroidetes (23%) were the dominant bacteria in a CW vegetated with Typha orientalis Presl (Wang P. et al., 2016). Moreover, a high number of nitrifying bacteria (e.g., Nitrosomonas, Nitrosospira and Nitrospira) were enriched in I. pseudacorus than in T. orientalis Presl vegetated CW system. Arroyo et al. (2013) analyzed microbial communities in mesocosm-scale CW systems treating metal-rich (As and Zn) wastewater and noticed that Proteobacteria was the dominant phylum in the enriched microorganisms. Moreover, phylogenetic analysis showed that among the three design parameters (i.e., vegetation, flow, and substrates), vegetation and water flow characteristics considerably influence the structure and composition of the microbial communities. Overall, based on the wastewater qualities, diverse microbial communities including nitrifiers, denitrifiers, and organic degraders are detected in CW systems treating wastewater.
Microbial Community Dynamics in CW Systems Treating Stormwater
In addition to the management of stormwater, CW systems purify stormwater. Microbiological-based processes significantly contribute to decontamination of stormwater. Literature search reveals that limited works have been conducted on the characterization of microbial flora abundant in stormwater treated by CW systems (Urakawa et al., 2017; Bonetti et al., 2021; Choi et al., 2021). The key findings of these studies are discussed here. A recent work characterized microbial communities in the soil collected from CW systems treating stormwater, and found high diversity among the communities, i.e., 45% nitrogen transforming microbes, 14% iron/sulfate cycling microbes and 4% methanotrophs (Bonetti et al., 2021). The enrichment of methanotrophs was primarily responsible for the emission of greenhouse gases (methane) into the environment, and it causes a 3-fold increase of global warning potential. At the phylum level, Proteobacteria (which includes classes of Alpha-, Beta-, Gamma-, and Delta-) were the dominant bacteria. Urakawa et al. (2017) performed microbial characterization of samples collected from various components of stormwater treated by floating CW systems including plant roots biofilm, surrounding water samples and biofilm developed in the pot and floating mat foam. The enriched microbiomes were grouped into the following five categories: sulfate reduction, aerobic sulfur oxidation, anaerobic sulfur oxidation, methanotrophy, methylotrophy, nitrification and photoautotrophy. The predominant bacteria in the root biofilm were Alphaproteobacteria and Cyanobacteria (classification at class level), and Anabaena, Rhizobium and Rhodobacter (classification at genus level) (Urakawa et al., 2017). Similar microbial profiles were observed in the surrounding water samples (similarity: 49–57%), but pot biofilm communities were different (similarity: 7–22%) Additionally, Rhizobium species were mainly detected in plant root biomass which suggests that root exudates secreted by plants may selectively promote the growth of a specific community like Rhizobium (Urakawa et al., 2017). The pot and foam biofilm samples were mainly enriched with Pseudomonas spp. which may contribute to the removal of pollutants (e.g., mainly nutrients). Huang et al. (2018) also observed a shift of microbial communities in stormwater treated by CW systems since the phyla Proteobacteria (Beta- and Gamma-class) and Bacteriodetes were mainly found in the influent of water samples, while Cyanobacteria were the key bacteria in effluent samples.
In addition to CW systems, researchers have also investigated microbial communities in natural (e.g., river-based) wetland systems (Cao et al., 2017). It was observed that communities related to different biogeochemical cycling including biological sulfur transformation (Desulfobacterales, Syntrophobacterales (at order level) and Thiobacillus (at genus level)), nitrogen transformation (Nitrospirae) and methanogenesis (Methanomicrobia order in archaea) were detected in the sediments collected from four different locations in two rivers (Cao et al., 2017). Moreover, several low-abundance phyla namely Firmicutes, Acidobacteria, Verrucomicrobia, and Actinobacteria, etc. were found in water samples. A recent study characterized microbial communities in a HSF CW system fed with stormwater from road and parking lot, and the analysis revealed that the phyla namely Proteobacteria (37.8%), Actinobacteria (15.9%) and Acidobacteria (13.4%) were predominant in CW system (Choi et al., 2021). The microbial counts in soil collected from various zones in the CW facility varied between 36,110–47,836 copies/g of soil (Choi et al., 2021). In a CW system treating stormwater from a parking lot, Bledsoe et al. (2020) analyzed microorganisms enriched in two different zones (permanently flooded and shallow land areas) to evaluate greenhouse gas (methane) emission potential of the CW system. The methane production rate was higher in the permanently flooded zone than in the shallow land area. Microbial analysis showed that several methane producing bacteria namely Methanomicrobia, Methylocystis, and Syntrophaceae were enriched in the permanently flooded zones. In the shallow land, the dominant bacteria were unclassified bacteria Betaproteobacteria, Acidobacteria, Gp6 and Geobacter (Bledsoe et al., 2020). The composition of microbial communities enriched in different CW systems operated under various climatic conditions are presented in Table 6. Together, nitrogen metabolism microbes including nitrifiers and denitrifiers as well as methanogens are frequently detected in CW systems treating stormwater (Urakawa et al., 2017; Bledsoe et al., 2020; Bonetti et al., 2021). Sulfate reducing bacteria and sulfur oxidizing bacteria are also enriched in sediments (Fu et al., 2021).
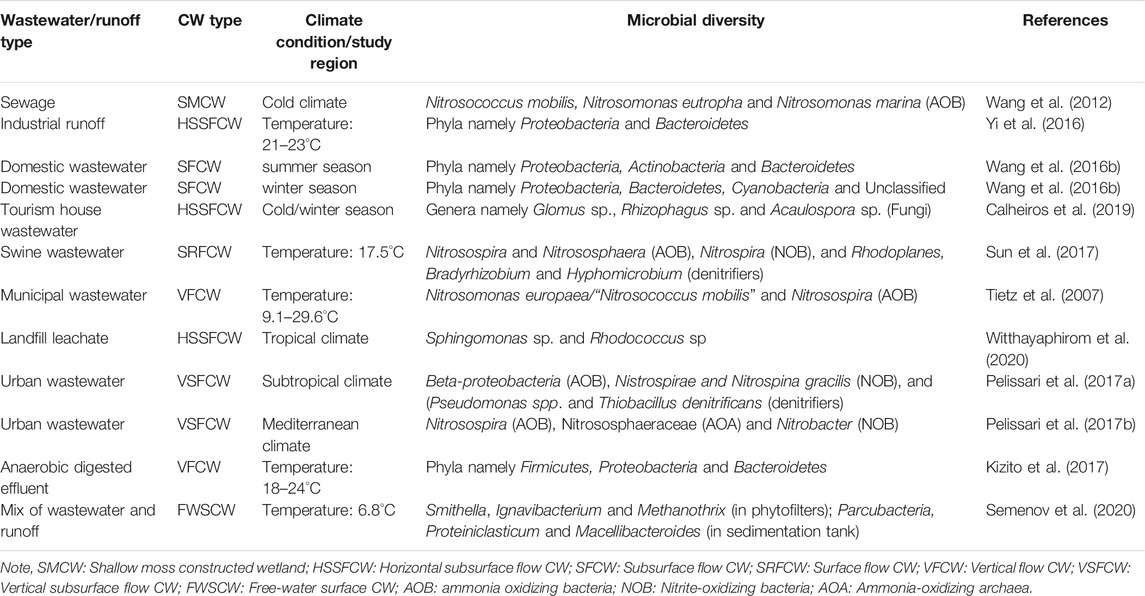
TABLE 6. Microbial diversity enriched in constructed wetlands (CW) under various climatic conditions/regions.
Factors Impacting Pollutants Removal Performance of Constructed Wetlands
The interactions between abiotic and biotic components as well as external factors could influence the treatment efficiency of CW systems. Several parameters including CW physical configurations, hydraulics, substrates, plant species diversity, dissolved oxygen (DO) level, climatic conditions (e.g., temperature/season) etc. impact the pollutant removal performance of CW systems (Zhu et al., 2014; Herrera-Cárdenas et al., 2016). The pollutant removal efficiency varies with a change of CW system configurations as reported by Chen et al. (2016). Chen et al. (2016) compared the antibiotics removal performance of three different CW configurations namely SF and SSF (VF and HSF CW) wetland facilities, and SSF systems (89.1–98.9%) show higher performance for the removal of antibiotics (e.g., erythromycin) than the SF system (76%). Moreover, among the HSF and VF configurations, HSF (98.9%) had higher capability for the removal of pollutants compared to the VF unit (89.1%). Xu et al. (2016) also observed differences in the reduction of pollutants in pilot-scale HSF-based and VF-based CW systems. The former type showed high performance for the removal of COD (74%) and ammonia (79%), while the latter one was effective mainly for the total nitrogen removal (64%) (Xu et al., 2016).
Hydraulic loading rate (HLR) could affect the performance of CW systems, and in most of the cases, the pollutant removal efficiency decreased with an increase of HLR (Trang et al., 2010). In HSF-based CW systems treating a mixture of wastewater and stormwater, it was observed that the removal efficiency of TN and TP constantly decreased with a gradual increase of HLR (31, 62, 104 and 146 mm/day). Also, the TN and the TP removal efficiency decreased from 84 to 16% and from 99 to 72%, respectively with the rise of HLR from 31 to 146 mm/day (Trang et al., 2010). In a pilot-scale HSF CW system, the removal efficiency of COD, TN and TP was 95, 95 and 95%, respectively at a HLR of 0.025 m/day, but the removal efficiency decreased to 91, 87 and 89% when the HLR was doubled (0.05 m/day) (Angassa et al., 2019).
Hydraulic retention time (HRT) is one of the important operational parameters which impacts the effectiveness of CW systems (see supplementary material, Supplementary Table S5). Sultana et al. (2016) investigated the COD removal from cheese whey wastewater in pilot-scale unplanted and planted (reed: Phragmites australis) HSF-based CW systems at four different HRTs (1, 2, 4 and 8 days). In both CW systems, the percentage of COD removal was reduced with a decrease of HRT, i.e., in an unplanted CW system; the percentage of COD removal decreased from 100 to 76% when HRT was reduced from 8 days to 1 day. However, in the vegetated cell, a similar decrease of performance was also noticed (a reduction from 100 to 76% with a reduction of HRT from 8 days to 1 day). In VF-based CW systems, Sarmento et al. (2013) evaluated the influence of four distinct HRTs (1, 2, 3 and 4 days) on the decontamination of various pollutants from swine wastewater. It was observed that the pollutant removal efficiency was enhanced with the rise of HRT up to 3 days, and then decreased. In a recent pilot-scale study on the treatment of synthetic wastewater using a vertical subsurface flow CW vegetated with two plants namely Typha latifolia and Phragmites australis, the pollutant (e.g., COD) removal efficiency constantly increased with the rise of HRT from 2 days (69.4%), 4 days (77.6%), 6 days (86.3%), 8 days (86.4%) and 10 days (88.8%) (Shruthi and Shivashankara, 2021b). Another work from the same research group using a horizontal subsurface flow CW run under similar operational conditions (e.g., HRT) also reported a similar relationship between HRT and pollutant removal, i.e., COD removal increased by 65.0, 74.4, 82.8, 86.1 and 88.3% with increase of HRT by 2, 4, 6, 8 and 10 days, respectively (Shruthi and Shivashankara, 2021a). Badhe et al. (2014) explored the performance of a free surface, up-flow CW planted with Typha latifolia for removal COD, ammonia and phosphate under two different HRTs (1.5 and 2.5 days), and the pollutant removal efficiency was slightly higher at HRT of 1.5 days (COD: 65.02%, ammonia: 4.9% and phosphate: 11.1%) than 2.5 days (COD: 55.5%, ammonia: 4% and phosphate: 11%). In a surface flow CW, its operation at higher HRT positively influenced the reduction of microbial pollutant concentration, i.e., 91% E. coli removal attained at HRT of 11.6 days, but the removal efficiency declined to 66% when the system was operated at 0.9 days HRT (Díaz et al., 2010).
Plants are one of the important components in CW systems and could influence the decontamination performance of wetlands. Sarmento et al. (2013) compared the performance of three plant species namely Cyperus sp. (grass), Heliconia rostrata (shrub) and Hedychium coronarium (herbaceous) for the removal of various pollutants from swine wastewater in VF-based CW systems, and found that Cyperus sp. was effective for the removal of various pollutants (COD: 69%, TKN: 57%, NH4+: 62% and TP: 64%). In addition to plant species diversity, plant root characteristics (length, biomass, architecture, etc.) could also impact the rate of uptake of pollutants since among the three types of plants namely Canna (flowering plant), Phragmites australis (reed) and Cyprus papyrus (flowering seed plant) vegetated in VF-based CW systems investigated for the decontamination of municipal wastewater, the uptake of nitrogen and phosphorus as well as the removal fecal indicator bacteria was higher in Canna than the other two vegetations (Abou-Elela and Hellal, 2012). Canna showed better performance since the roots were spread broadly and uniformly in the CW filter bed. Diversity of vegetation species in CW influenced the removal of nitrogen more compared to that of phosphorus (Liang et al., 2017). Diversity of plant species planted in various CW systems operated under diverse climatic conditions (warm, cold, tropical, arid, semi-arid, etc.) are presented in Table 7.
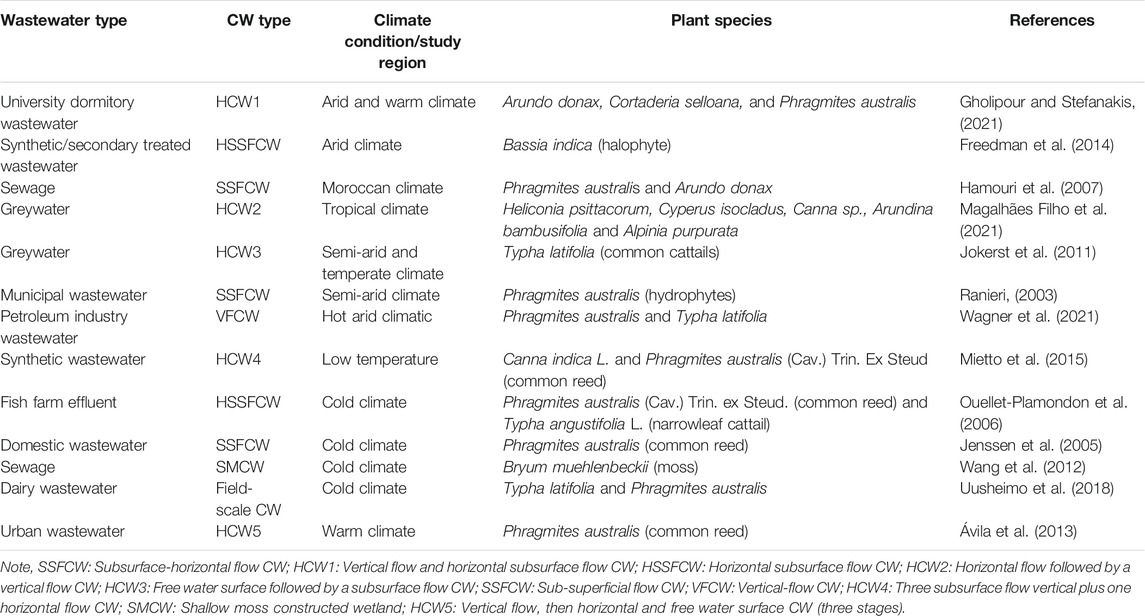
TABLE 7. Diversity of plant species used in constructed wetlands (CW) under various climatic conditions/regions.
The filter media composition significantly influences the pollutant removal efficiency of CW systems. To achieve high performance in CW systems, it is necessary to choose substrates which have high ecological activity and possess high adsorption capacity (Chen et al., 2009). A recent study on tidal flow CW systems (planted with Phragmites or Vetiver) reported that organic-based media (biochar, coco-peat and coal) exhibited higher pollutant removal performance (N: 71–85% and organics: 84–96%) than waste (slag)/construction-based media (gravel and concrete block) (N: 49–69% and organics: 74–95%). Among these materials, slag and construction media were effective for removal of phosphorus (≥93%) (Saeed et al., 2020). Li et al. (2019) showed that addition of biochar into SF CW systems enhanced the nitrogen removal efficiency, i.e., with the addition of 10% (V/V) biochar, the nitrate removal was increased by 5%, while with further increase of the biochar dose to 20% (V/V), the nitrate removal was enhanced by 10%.
The removal of nitrogen and COD is influenced by the carbon/nitrogen (C/N) ratio. Zhu et al. (2014) observed an increase of the removal of TN, nitrate and COD from wastewater with the rise of the C/N ratio in HSF-based CW systems, and the C/N ratio of five demonstrated excellent removal performance. However, ammonia removal exhibited an opposite trend with the rise of C/N ratio due to the decrease of dissolved oxygen levels, caused by biodegradation of organic components (Zhu et al., 2014). According to Li et al. (2014), the denitrification rate was enhanced at higher C/N ratio (e.g., C/N = 14.3). For nitrification and organic degradation, high DO concentration is favorable since Li et al. (2014) observed that with increases of DO concentration from 1.3 mg/L to 3.4 mg/L in horizontal subsurface flow CW (HSFCW) systems, the removal of COD and TN increased from 87.2 to 55%–90.9 and 88.1%, respectively. In addition to artificial aeration, vegetation of a suitable plant can provide sufficient oxygen to CW systems since a recent study had reported that the plantation of Vallisneria natans provided the required oxygen to the CW system and higher nitrogen removal (90% NH4+-N) was achieved (Fu et al., 2021).
A study compared the effects of three different parameters, i.e., HRTs (1, 3 and 5 days), substrate types (river gravel, fine and coarse volcanic gravel), plant types (Cyperus papyrus, Phragmites australis and Thypa latiffolia) and organic loads from effluent from a wastewater treatment plant on removal micropollutants (Herrera-Cárdenas et al., 2016). This study revealed that HRT was the dominant factor which significantly impacts the treatment process in HSF CW systems (Herrera-Cárdenas et al., 2016). The removal of micropollutants varied between 55 and 99%, while the removal of organics ranged from 70 to 75%. Moreover, at the HRT of 1 day, the BOD5 removal was nearly 75%, but with increase of HRT to 3/5 days, the BOD5 removal was enhanced to nearly 90%.
The pollutant removal performance of CW systems is influenced by seasonal variations. For example, according to Wang et al. (2021), CW systems were very effective for the removal of TN, TP and particulate phosphate in spring and summer, but less effective in autumn and winter (Wang et al., 2021a). Dong et al. (2011) observed a change in the pollutant removal performance of an integrated CW system with the change of season. For example, the removal of TN and TP from wastewater was 98% each in summer. However, the removal efficiency decreased to 96% each in winter. The reduction in the performance at lower temperature is possibly due to a decrease of microbial activities and diffusion rates (Dong et al., 2011).
Microbial degradation is one of the important pathways for removal of pollutants from wastewater/stormwater in CW systems. Thus, in addition to CW operational parameters and surrounding climatic conditions, the abundance and diversity of various functional microbial communities (nitrifiers, denitrifiers, organic carbon degraders, etc.) could influence the treatment performance of CW systems. Notably, the enrichment of functional microbes depends on the several factors including CW design configurations and vegetation (Zhang et al., 2018). CW systems with saturated and aerated designs show high microbial activity and metabolic richness (Zhang et al., 2018). Zhou et al. (2020) found that with intermittent aeration in a subsurface flow CW, microbial abundance increased, while microbial diversity decreased. Wang et al. (2016) assessed the effect of two different plants (Iris pseudacorus and Typha orientalis Presl) in a subsurface flow CW on microbial communities and reported that vegetation positively influences bacterial richness and diversity, and the abundance of nitrifying bacteria (Nitrosomonas, Nitrosospira and Nitrospira) was higher in CW systems planted with Iris pseudacorus than Typha orientalis Presl. Hathaway et al. (2011) reported that for CW systems treating stormwater, designs with higher HRT and lower stormwater velocities are beneficial to achieve higher microbial pollutants removal by sedimentation and degradation. According to Wadzuk et al. (2010), low inflow velocity, higher HRT and vegetation favor suspended solids removal in CW systems. Together, planted subsurface flow/hybrid configurations, aerobic environment (high DO levels) with operations at low HLR, high HRT and relatively high environmental temperature (e.g., summer season) positively influence the performance of CW systems for the removal of pollutants from wastewater and stormwater (Supplementary Figure S2).
Pollutant Removal Mechanisms
The removal of pollutants from wastewater/stormwater is mainly mediated by three internal components of CW systems such as 1) vegetated plants, 2) enriched microorganisms/biofilms developed in the plant root zone, and 3) filter media/substrate (Liang et al., 2002; Rajan et al., 2018; Sandoval-Herazo et al., 2018). The physicochemical and biological processes which contribute to pollutants removal include sedimentation, filtration, adsorption, decomposition, chemical transformation, volatilization, biological assimilation and biodegradation (Walker and Hurl, 2002). Physicochemical processes namely sedimentation, filtration and sorption are generally considered as the predominant mechanisms for removal of solids (e.g., TSS) (Struck et al., 2008). The nutrients (nitrogen and phosphorus) are removed by both physicochemical and biological processes. In CW systems, N transformation and removal are carried out by multiple processes including adsorption, desorption, mineralization (ammonification), nitrification, denitrification, ammonia volatilization, nitrogen fixation, anaerobic ammonia oxidation (anammox), plant and microbial uptake, etc. (García et al., 2010). Nitrification and dentification are the dominant biological processes for the removal of nitrogen in CW systems (Dong and Sun, 2007). In nitrification, ammonia is converted to nitrate through the nitrite intermediate (Biswal et al., 2021), and the process is mediated by ammonia oxidizing bacteria (e.g., Nitrosomonas) and nitrite oxidizing bacteria (e.g., Nitrosospira and Nitrospira) (Wang P. et al., 2016). In denitrification, nitrate is reduced to nitrogen gas through multiple steps, and the microbial transformation is mediated by denitrifying bacteria (e.g., Denitratisoma, Planctomyces, Magnetospira, Pseudomonas spp. and Dechloromonas) (Verduzo Garibay et al., 2021).
The key physicochemical processes for the P removal include adsorption/desorption and chemical precipitation (García et al., 2010). P can be taken up by plants through roots and be stored in various parts of the plant (García et al., 2010). Unlike the N removal by microbial processes, the presence of phosphate accumulating organisms (PAOs) in wastewater/stormwater can uptake and store P within cells, and the process is called enhanced biological phosphorus removal (EBPR) (García et al., 2010). A recent study explored the feasibility of EBPR in an intermittent aeration CW, and found that 71.25% phosphate was removed by aerobic PAOs (APAOs), while 28.75% was removed by denitrifying PAOs (DPAOs) (Wang et al., 2021b). Sedimentation is the main mechanism responsible for the removal of heavy metals in natural or CW systems (Walker and Hurl, 2002). For the removal of organic pollutants, the major biological processes (redox conditions) associated with the transformation of organic pollutants include aerobic respiration (biodegradation) and anaerobic processes (fermentation, sulfate reduction and methanogenesis), as well as the uptake by plants and metabolization (García et al., 2010; Lyu et al., 2018). The biodegradation of organic pollutants is facilitated by aerobic organic degraders (e.g., Proteobacteria, Zobellella, Thauera, Pseudomonas, Aeromonas, etc.) and/or anaerobic organic degraders (e.g., Methanobacteriales, Methanomicrobiales, Methanosarcinales, etc.) (Verduzo Garibay et al., 2021). The key removal mechanisms for microbial contaminants in CW systems include filtration, adsorption, sedimentation, and natural die-off because of starvation/predation by higher organisms (Wu et al., 2016). The potential mechanisms responsible for the removal of chemical and microbial pollutants from wastewater and stormwater are summarized in Figure 3.
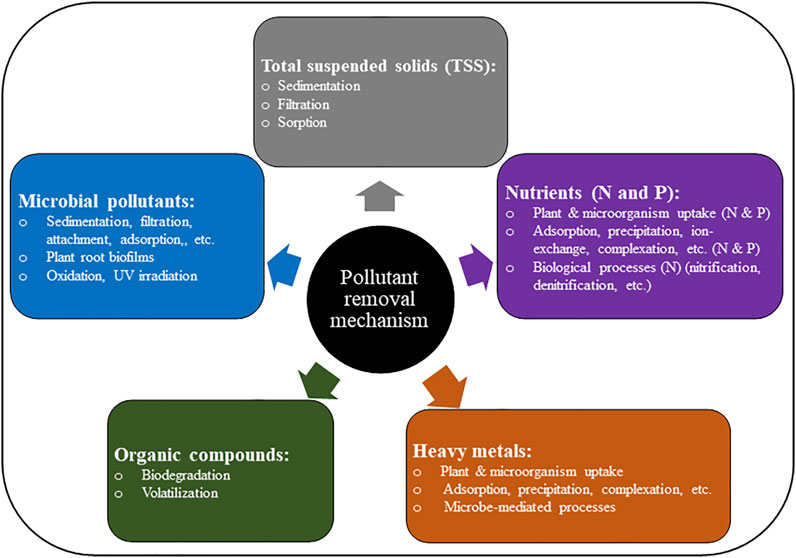
FIGURE 3. Potential mechanisms for the removal of chemical and microbial pollutants from wastewater/stormwater in CW systems.
Modelling of Constructed Wetland Systems
A growing number of research articles have been published on modelling of various aspects (e.g., kinetics, hydrological, mechanistic, etc.) of CW systems. The models have been developed to simulate various processes (hydrological, physicochemical, biological, etc.) in CW systems, and optimizations are made based on modelling results to enhance the function and performance (decontamination efficiency) of CW systems. The kinetics, hydrological and mechanistic models are briefly described below.
Kinetics Model
Several studies have employed the first-order decay model (Eq. 1) to predict the performance of CW systems for the removal of pollutants from wastewater and stormwater (Struck et al., 2008; Boutilier et al., 2009).
Where, C0 = initial concentration of pollutant (at time 0), Ct = final concentration of pollutant (at time t), t = reaction time (e.g., h), and K = reaction rate constant (e.g., h−1). Struck et al. (2008) monitored inactivation rate constants of E. coli, fecal coliforms and Enterococci in stormwater treated by CW systems over 6 months of operation and K varied between 0.0597–0.1894, 0.0536–0.3277, 0.0594–0.2112 h−1, respectively. Boutilier et al. (2009) also analyzed inactivation rate of E. coli in wastewater removed by CW systems using the first-order decay model, and found that the rate constant (K) ranged from 0.09 d−1 (at 7.6°C) to 0.18 d−1 (at 22.8°C). Chen et al. (2009) found that the removal of heavy metals (Cu, Pb, and Zn) from wastewater can be well described by the first-order dynamic model, and the computed rate constants for Zn removal using two different media namely gravel and coke were 0.1222 and 0.2326 h−1, respectively. For Cu removal, the rate constants for the two media were 0.3739 and 0.2017 h−1. The Pb removal adsorption data was not well fitted to the first order model. The rate constant values demonstrate that the two media have different adsorption capabilities for different metals. Lyu et al. (2018) noticed that the removal of pesticide tebuconazole in unsaturated and saturated CW systems can be well explained by an area-based first-order kinetic model, and the rate constants for planted CW systems (3.1–10.9 cm d−1) was higher than non-planted wetlands (1.7–2.6 cm d−1). In total, the removal rate constant varied with change of type of pollutants and filter media characteristics.
Hydrological Model
Hydrological models are applied to describe the hydrological behavior of constructed wetland systems. The ecological performance of CW also depends on its hydrological regime. Knowles and Davies (2011) employed a finite element analysis (FEA) model to determine linkage between clogging and hydraulics in HSF CW systems. It was assumed that the clogging was due to transport of particles and a single collector efficiency model was adopted to describe the clogging behavior in wetland systems. In freshwater wetlands, the hydrological processes were studied using a simple daily mass-balance water budget model (Zhang and Mitsch, 2005). This model comprises both inflows and outflows, two key processes namely precipitation, evapotranspiration and groundwater seepage. The calculated coefficient of prediction efficiency was 0.70. Chazarenc et al. (2003) reported that HRT distributions of the HSF-CW can be calculated using the impulsion tracer method. Moreover, in non-ideal flow conditions, the dispersion plug flow model and stirred tanks in series model can be applied to study the hydrodynamics in constructed wetlands. Martinez-Martinez et al. (2014) used Soil and Water Assessment Tool (SWAT) to investigate the effect of wetland restoration on streamflow rates and peak flows in the Shiawassee River watershed of Michigan, and the authors found that increasing wetland area was beneficial than wetland depth for long-term daily streamflow. In this work, the model was amended to let ponded water to interact with the soil and aquifer. Fournel et al. (2013) showed that HYDRUS-1D was effective to simulate hydrodynamic of vertical flow wetlands treating urban runoff. Overall, a suitable hydrological model can be selected based on the CW configurations and flow characteristics.
Mechanistic Model
Mechanistic models are useful to understand the pollutant transport and various mechanisms that contribute to the removal of contaminants in CW systems. Several process-based (biological and geochemical) models have been developed to understand various processes in CW facilities (Bayona et al., 2009; Langergraber, 2011b). Lyu et al. (2018) applied both artificial neural network (ANN) and linear regression (LR) models to compute pollutant removal efficiency of a pesticide (tebuconazole) in unsaturated and saturated constructed wetlands, and observed that ANN (R2 = 0.85) provided more accurate estimation than LR (R2 = 0.81) on the removal of tebuconazole. Another study also employed ANN method for prediction of various pollutants (e.g., TN and TP) from urban stormwater in VF CW systems (Kiiza et al., 2020). Chavan and Dennett, (2008) used wetlands water quality model (WWQM) to assess nutrients (N and P) and sediments removal in a CW system. The model predicted that the CW system will retain 62% nitrogen, 38% phosphorus and 84% sediments. Huang et al. (2015) applied a numerical model (SubWet 2.0) to model diverse pollutants (BOD5, ammonia, nitrate and TP) removal in VF CW systems under two inflow characteristics (stormwater and domestic wastewater). Under both inflow conditions, the model performance was better for BOD5 and TP since the computed correlation coefficients (R) for BOD5 (R = 0.79–0.84) and TP (R = 0.94–0.98) were higher than ammonia (R = 0.60–0.70) and nitrate (R = 0.48–0.97). A recent study developed a wetland water quality model (PCSWWM: 1-D Stormwater Management Model), and validated the model using water quality data collected from a field-scale CW system. The correlation coefficients for TSS, TN and TP were 0.749, 0.491 and 0.856, respectively (Dharmasena et al., 2021). Applying various statistical models, Hijosa-Valsero et al. (2011) found that wastewater physicochemical parameters (pH, temperature, DO level, redox potential and conductivity), and the presence of plants positively influence the removal of organic matter and PPCPs in CW systems. Moreover, multiple regression equation and regression trees (called CHAID: Chi-square Automatic Interaction Detector) offered numerical estimations of removal efficiencies of various contaminants in CW systems.
Together, extensive studies have been carried out on the application of kinetics, hydrologic and mechanistic-based models for the prediction of hydrological patterns and the performance of CW ecosystems with high accuracy. Among various types of models, the process-based models are usually less popular due to their complexity in structure and usage (Langergraber, 2011a). Application of a robust and simplified computer-based model for prediction of performance (both hydraulics and pollutants decontamination) of CW systems would help design engineers and stakeholders to select a suitable configuration of CW system for treatment of wastewater and stormwater. Model-based studies would also help to develop design guidelines for installation, operation and maintenance of CW systems.
Future Research Directions
➢ Numerous studies have been conducted on the reclamation of wastewater using CW systems. However, limited information is available on stormwater reclamation using CW systems. Moreover, integrative and comparative studies focusing on treatment of both wastewater and stormwater by CW system for the removal of chemical and microbial pollutants are currently lacking. Specifically, pilot-scale and field-scale studies are needed to better evaluate the decontamination efficiency of single and hybrid CW systems with respect to both wastewater and stormwater and assess the treatment performance of CW systems with changes in pollutant loads (wastewater vs. stormwater).
➢ For wider application of CW systems for treatment of wastewater containing diverse pollutants, specific guidelines including CW design, installation, operation and maintenance should be established. The detailed guidelines including CW configuration (length, breadth and height), selection of filter materials (media components, depth, permeability, particle size distribution, etc.), selection of planting schemes (diversity and density of vegetation), and operation (initial operational conditions) and maintenance of CW systems at various stages of operations including the startup phase, vegetation growth phase and over a long-term establishment.
➢ More research works are needed to evaluate the performance of CW facilities in harsh environmental settings including cold climate, arid region and tropical environment. Challenging environmental conditions including cold and warm weather conditions could impact hydrology and ecology of CW systems. Thus, the rate of abiotic and biotic processes which contribute to removal of pollutants could be altered.
➢ Log-term field studies are required to evaluate the stability and sustainability of CW systems as well as to understand various operational and environmental conditions that impact the pollutant fate, transport and removal in wetlands. Data from long-term experiments would also help for development of guidelines for CW design, installation, operation and maintenance. These guidelines will enhance the construction quality, reduce the potential breakdown of wetland systems, and increase the life span of CW systems. Moreover, the guidelines may help in the decision making by local regulatory agencies to set up new standards or revise existing standards for improvement of the CW effluent quality to qualify for discharge into aquatic environments or for reuse of the treated effluent for various purposes.
➢ In depth experiments are necessary for the development of novel substrates with high adsorption capacity, low leaching potential of secondary pollutants and regeneration using simple and cost-effective methods. In CW systems, sand and gravel are commonly used as filter materials. To reduce the demand of natural materials and the overall operating cost, potential use of waste materials (construction waste, incineration ash, etc.) as filter media should be explored in future. Moreover, the cause of clogging phenomenon occurring in porous media is not fully understood yet. The clogging of filter media could reduce the treatment efficiency of CW systems, leading to frequent maintenance of CW systems in terms of media regeneration/replacement. Thus, future research works in this direction are warranted.
➢ Since vegetation is one of the important components of CW systems, future research should focus on conducting a series of phytoremediation experiments using locally available plants to select the plant species that can provide optimum phytoremediation potential to diverse pollutants even under extreme climatic conditions (e.g., high salinity, warmer climate and prolonged drought period). In addition to native vegetation, screening of exotic plants should be explored in future. Planting of both native and exotic plants may show better treatment performance than that of individual species. Moreover, guidelines should be developed to control the growth of weeds or other invasive plants in wetland systems.
➢ Although numerous modelling works have been conducted on single CW system, limited information is currently available on the accuracy of models in terms of the prediction of hydraulic and pollutant removal performance in hybrid CW systems since they usually show better performance than single CW system. Since CW systems contain diverse complex processes, the development of a sophisticated and compressive model would help understand interactions between abiotic and biotic components, complex processes as well as the optimization of CW performance. An artificial intelligence-based model would help the system operator to adjust the operational conditions based on the fluctuation of hydrology, pollutant loads, climatic conditions, etc. A well calibrated and validated model could also help decision makers for the selection of a suitable CW unit based on the requirements of the local region.
Conclusion
This review paper compressively analyzes the recent advancement on the reclamation and reuse of water (wastewater and stormwater) in CW systems. Among various CW configurations, subsurface (horizontal and vertical) flow/hybrid systems are effective for the removal of chemical and microbial pollutants from wastewater and stormwater. The reclaimed water can be reused as non-potable water. Physicochemical processes (sedimentation, adsorption, precipitation) and microbiological processes (biodegradation, assimilation, nitrification and denitrification) and plant-mediated processes (phytoremediation and assimilation) mainly contribute to the removal of pollutants in CW systems. The key microbial flora enriched in CW ecosystems include nitrifying bacteria, denitrifying bacteria and organic matter degrading microorganisms. Additionally, the CW microbiome is mainly dominated by two phyla namely Proteobacteria and Bacteroidetes. Key operational/environmental parameters such as low HLR, high HRT, high dissolved oxygen contents and operation in summer season accelerate pollutant removal efficiency of CW systems. In addition to experimental works, numerous kinetics, hydrologic and mechanistic-based models are developed for the prediction of function and performance of CW systems. Overall, this critical review advances our knowledge on potential reclamation of wastewater and stormwater using CW systems. This review would also assist environmental engineers, managers and urban planners for the selection and installation of a suitable configuration of CW system for management and treatment of wastewater and urban stormwater.
Author Contributions
BB: Conceptualization, Investigation, Methodology, Writing—original draft, review and editing. RB: Conceptualization, Supervision, Writing—review and editing.
Funding
The postdoctoral Research Fellow appointment of BB was supported by the National Research Foundation (NRF), Singapore, and Ministry of National Development (MND), Singapore under its Cities of Tomorrow R&D Programme (CoT Award COT-V4-2019-6).
Conflict of Interest
The authors declare that the research was conducted in the absence of any commercial or financial relationships that could be construed as a potential conflict of interest.
Publisher’s Note
All claims expressed in this article are solely those of the authors and do not necessarily represent those of their affiliated organizations, or those of the publisher, the editors and the reviewers. Any product that may be evaluated in this article, or claim that may be made by its manufacturer, is not guaranteed or endorsed by the publisher.
Supplementary Material
The Supplementary Material for this article can be found online at: https://www.frontiersin.org/articles/10.3389/fenvs.2022.836289/full#supplementary-material
References
Abou-Elela, S. I., Golinelli, G., Saad El-Tabl, A., and Hellal, M. S. (2013). Treatment of Municipal Wastewater Using Horizontal Flow Constructed Wetlands in Egypt. Water Sci. Technol. 69, 38–47. doi:10.2166/wst.2013.530
Abou-Elela, S. I., and Hellal, M. S. (2012). Municipal Wastewater Treatment Using Vertical Flow Constructed Wetlands Planted with Canna, Phragmites and Cyprus. Ecol. Eng. 47, 209–213. doi:10.1016/j.ecoleng.2012.06.044
Al-Wahaibi, B. M., Jafary, T., Al-Mamun, A., Baawain, M. S., Aghbashlo, M., Tabatabaei, M., et al. (2021). Operational Modifications of a Full-Scale Experimental Vertical Flow Constructed Wetland with Effluent Recirculation to Optimize Total Nitrogen Removal. J. Clean. Prod. 296, 126558. doi:10.1016/j.jclepro.2021.126558
Almuktar, S. A. A. A. N., Abed, S. N., and Scholz, M. (2018). Wetlands for Wastewater Treatment and Subsequent Recycling of Treated Effluent: a Review. Environ. Sci. Pollut. Res. 25, 23595–23623. doi:10.1007/s11356-018-2629-3
Alufasi, R., Gere, J., Chakauya, E., Lebea, P., Parawira, W., and Chingwaru, W. (2017). Mechanisms of Pathogen Removal by Macrophytes in Constructed Wetlands. Environ. Techn. Rev. 6, 135–144. doi:10.1080/21622515.2017.1325940
Álvarez, J. A., Ávila, C., Otter, P., Kilian, R., Istenič, D., Rolletschek, M., et al. (2017). Constructed Wetlands and Solar-Driven Disinfection Technologies for Sustainable Wastewater Treatment and Reclamation in Rural India: SWINGS Project. Water Sci. Technol. 76, 1474–1489. doi:10.2166/wst.2017.329
Angassa, K., Leta, S., Mulat, W., Kloos, H., and Meers, E. (2019). Effect of Hydraulic Loading on Bioremediation of Municipal Wastewater Using Constructed Wetland Planted with Vetiver Grass, Addis Ababa, Ethiopia. Nanotechnol. Environ. Eng. 4, 6. doi:10.1007/s41204-018-0053-z
Arroyo, P., Ansola, G., and Miera, L. E. S. d. (2013). Effects of Substrate, Vegetation and Flow on Arsenic and Zinc Removal Efficiency and Microbial Diversity in Constructed Wetlands. Ecol. Eng. 51, 95–103. doi:10.1016/j.ecoleng.2012.12.013
Ávila, C., Garfí, M., and García, J. (2013). Three-stage Hybrid Constructed Wetland System for Wastewater Treatment and Reuse in Warm Climate Regions. Ecol. Eng. 61, 43–49. doi:10.1016/j.ecoleng.2013.09.048
Badhe, N., Saha, S., Biswas, R., and Nandy, T. (2014). Role of Algal Biofilm in Improving the Performance of Free Surface, Up-Flow Constructed Wetland. Bioresour. Techn. 169, 596–604. doi:10.1016/j.biortech.2014.07.050
Bayona, J., García, J., Brovelli, A., Baechler, S., Rossi, L., and Barry, D. (20092009). Comprehensive Process-Based Modelling of Sand Filters and Subsurface Flow Constructed wetlandsWETPOL 2009: Wetland Pollution Dynamics and Control. Barcelona, Spain, September.
Bilgin, M., Şimşek, İ., and Tulun, Ş. (2014). Treatment of Domestic Wastewater Using a Lab-Scale Activated Sludge/vertical Flow Subsurface Constructed Wetlands by Using Cyperus Alternifolius. Ecol. Eng. 70, 362–365. doi:10.1016/j.ecoleng.2014.06.032
Biswal, B. K., Vijayaraghavan, K., Adam, M. G., Lee Tsen-Tieng, D., Davis, A. P., and Balasubramanian, R. (2021). Biological Nitrogen Removal from Stormwater in Bioretention Cells: a Critical Review. Crit. Rev. Biotechnol. 0, 1–23. doi:10.1080/07388551.2021.1969888
Biswal, B. K., Vijayaraghavan, K., Tsen-Tieng, D. L., and Balasubramanian, R. (2022). Biochar-based Bioretention Systems for Removal of Chemical and Microbial Pollutants from Stormwater: A Critical Review. J. Hazard. Mater. 422, 126886. doi:10.1016/j.jhazmat.2021.126886
Bledsoe, R. B., Bean, E. Z., Austin, S. S., and Peralta, A. L. (2020). A Microbial Perspective on Balancing Trade-Offs in Ecosystem Functions in a Constructed Stormwater Wetland. Ecol. Eng. 158, 106000. doi:10.1016/j.ecoleng.2020.106000
Bonetti, G., Trevathan-Tackett, S. M., Hebert, N., Carnell, P. E., and Macreadie, P. I. (2021). Microbial Community Dynamics behind Major Release of Methane in Constructed Wetlands. Appl. Soil Ecol. 167, 104163. doi:10.1016/j.apsoil.2021.104163
Borne, K. E., Fassman, E. A., and Tanner, C. C. (2013). Floating Treatment Wetland Retrofit to Improve Stormwater Pond Performance for Suspended Solids, Copper and Zinc. Ecol. Eng. 54, 173–182. doi:10.1016/j.ecoleng.2013.01.031
Boutilier, L., Jamieson, R., Gordon, R., Lake, C., and Hart, W. (2009). Adsorption, Sedimentation, and Inactivation of E. coli within Wastewater Treatment Wetlands. Water Res. 43, 4370–4380. doi:10.1016/j.watres.2009.06.039
Brix, H. (2020). Wastewater Treatment in Constructed Wetlands: System Design, Removal Processes, and Treatment Performance. Constructed Wetlands Water Qual. Improvement, 9–22. doi:10.1201/9781003069997-3
Calheiros, C. S. C., Pereira, S. I. A., Franco, A. R., and Castro, P. M. L. (2019). Diverse Arbuscular Mycorrhizal Fungi (AMF) Communities Colonize Plants Inhabiting a Constructed Wetland for Wastewater Treatment. Water 11, 1535. doi:10.3390/w11081535
Cao, Q., Wang, H., Chen, X., Wang, R., and Liu, J. (2017). Composition and Distribution of Microbial Communities in Natural River Wetlands and Corresponding Constructed Wetlands. Ecol. Eng. 98, 40–48. doi:10.1016/j.ecoleng.2016.10.063
Chavan, P. V., and Dennett, K. E. (2007). Wetland Simulation Model for Nitrogen, Phosphorus, and Sediments Retention in Constructed Wetlands. Water Air Soil Pollut. 187, 109–118. doi:10.1007/s11270-007-9501-2
Chazarenc, F., Merlin, G., and Gonthier, Y. (2003). Hydrodynamics of Horizontal Subsurface Flow Constructed Wetlands. Ecol. Eng. 21, 165–173. doi:10.1016/j.ecoleng.2003.12.001
Chen, J., Ying, G.-G., Wei, X.-D., Liu, Y.-S., Liu, S.-S., Hu, L.-X., et al. (2016). Removal of Antibiotics and Antibiotic Resistance Genes from Domestic Sewage by Constructed Wetlands: Effect of Flow Configuration and Plant Species. Sci. Total Environ. 571, 974–982. doi:10.1016/j.scitotenv.2016.07.085
Chen, K.-C., Wang, Y.-H., and Lu, Y.-C. (2011). Treatment of Polluted Water for Reclamation Using Photocatalysis and Constructed Wetlands. Catal. Today 175, 276–282. doi:10.1016/j.cattod.2011.06.013
Chen, M., Tang, Y., Li, X., and Yu, Z. (2009). Study on the Heavy Metals Removal Efficiencies of Constructed Wetlands with Different Substrates. Jwarp 01, 22–28. doi:10.4236/jwarp.2009.11004
Chislock, M. F., Doster, E., Zitomer, R. A., and Wilson, A. E. (2013). Eutrophication: Causes, Consequences, and Controls in Aquatic Ecosystems. Nat. Educ. Knowl. 4, 10.
Choi, H., Geronimo, F. K. F., Jeon, M., and Kim, L.-H. (2021). Investigation of the Factors Affecting the Treatment Performance of a Stormwater Horizontal Subsurface Flow Constructed Wetland Treating Road and Parking Lot Runoff. Water 13, 1242. doi:10.3390/w13091242
Davies, C. M., and Bavor, H. J. (2000). The Fate of Stormwater-Associated Bacteria in Constructed Wetland and Water Pollution Control Pond Systems. J. Appl. Microbiol. 89, 349–360. doi:10.1046/j.1365-2672.2000.01118.x
Dell'Osbel, N., Colares, G. S., Oliveira, G. A., Rodrigues, L. R., da Silva, F. P., Rodriguez, A. L., et al. (2020). Hybrid Constructed Wetlands for the Treatment of Urban Wastewaters: Increased Nutrient Removal and Landscape Potential. Ecol. Eng. 158, 106072. doi:10.1016/j.ecoleng.2020.106072
Despland, L. M., Clark, M. W., Vancov, T., and Aragno, M. (2014). Nutrient Removal and Microbial Communities' Development in a Young Unplanted Constructed Wetland Using Bauxsol Pellets to Treat Wastewater. Sci. Total Environ. 484, 167–175. doi:10.1016/j.scitotenv.2014.03.030
Dharmasena, T., Chua, L. H. C., Barron, N., and Zhang, H. (2021). Performance Assessment of a Constructed Wetland Using a Numerical Modelling Approach. Ecol. Eng. 173, 106441. doi:10.1016/j.ecoleng.2021.106441
Díaz, F. J., O’Geen, A. T., and Dahlgren, R. A. (2010). Efficacy of Constructed Wetlands for Removal of Bacterial Contamination from Agricultural Return Flows. Agric. Water Manag. 97, 1813–1821. doi:10.1016/j.agwat.2010.06.015
Dong, Y., Wiliński, P. R., Dzakpasu, M., and Scholz, M. (2011). Impact of Hydraulic Loading Rate and Season on Water Contaminant Reductions within Integrated Constructed Wetlands. Wetlands 31, 499–509. doi:10.1007/s13157-011-0176-5
Dong, Z., and Sun, T. (2007). A Potential New Process for Improving Nitrogen Removal in Constructed Wetlands-Promoting Coexistence of Partial-Nitrification and ANAMMOX. Ecol. Eng. 31, 69–78. doi:10.1016/j.ecoleng.2007.04.009
Dotro, G., Langergraber, G., Molle, P., Nivala, J., Puigagut, J., Stein, O., et al. (2021). Treatment Wetlands. London, United Kingdom: IWA Publishing. doi:10.2166/9781780408774
El Hamouri, B., Nazih, J., and Lahjouj, J. (2007). Subsurface-horizontal Flow Constructed Wetland for Sewage Treatment under Moroccan Climate Conditions. Desalination 215, 153–158. doi:10.1016/j.desal.2006.11.018
Ergaieg, K., and Ben Miled, T. (2021). Full-scale Hybrid Constructed Wetlands Monitoring for Decentralized Tertiary Treatment of Municipal Wastewater. Arab. J. Geosci. 14, 1407. doi:10.1007/s12517-021-07776-y
Fournel, J., Forquet, N., Molle, P., and Grasmick, A. (2013). Modeling Constructed Wetlands with Variably Saturated Vertical Subsurface-Flow for Urban Stormwater Treatment. Ecol. Eng. 55, 1–8. doi:10.1016/j.ecoleng.2013.02.004
Freedman, A., Gross, A., Shelef, O., Rachmilevitch, S., and Arnon, S. (2014). Salt Uptake and Evapotranspiration under Arid Conditions in Horizontal Subsurface Flow Constructed Wetland Planted with Halophytes. Ecol. Eng. 70, 282–286. doi:10.1016/j.ecoleng.2014.06.012
Fu, F., Huang, S., Hu, H., Lu, Y., Wang, Y., Yuan, J., et al. (2021). Transformation of N and S Pollutants and Characterization of Microbial Communities in Constructed Wetlands with Vallisneria Natans. J. Water Process Eng. 42, 102186. doi:10.1016/j.jwpe.2021.102186
Galletti, A., Verlicchi, P., and Ranieri, E. (2010). Removal and Accumulation of Cu, Ni and Zn in Horizontal Subsurface Flow Constructed Wetlands: Contribution of Vegetation and Filling Medium. Sci. Total Environ. 408, 5097–5105. doi:10.1016/j.scitotenv.2010.07.045
García, J. A., Paredes, D., and Cubillos, J. A. (2013). Effect of Plants and the Combination of Wetland Treatment Type Systems on Pathogen Removal in Tropical Climate Conditions. Ecol. Eng. 58, 57–62. doi:10.1016/j.ecoleng.2013.06.010
García, J., Rousseau, D. P. L., Morató, J., Lesage, E., Matamoros, V., and Bayona, J. M. (2010). Contaminant Removal Processes in Subsurface-Flow Constructed Wetlands: A Review. Crit. Rev. Environ. Sci. Techn. 40, 561–661. doi:10.1080/10643380802471076
Ghasemi-Zaniani, M., Eslamian, Saeid., Ostad-Ali-Askari, K., and Singh, V. (2017). Irrigation with Waste Water Treated by Constructed Wetlands. Int. J. Res. Stud. Agric. Sci. 3, 18–34. doi:10.20431/2454-6224.0311002
Ghennandi, A., Bixio, D., and Thoeye, C. (2007). The Role of Free Water Surface Constructed Wetlands as Polishing Step in Municipal Wastewater Reclamation and Reuse. Sci. Total Environ. 380, 247–258. doi:10.1016/j.ecoleng.2006.12.038
Gholipour, A., and Stefanakis, A. I. (2021). A Full-Scale Anaerobic Baffled Reactor and Hybrid Constructed Wetland for university Dormitory Wastewater Treatment and Reuse in an Arid and Warm Climate. Ecol. Eng. 170, 106360. doi:10.1016/j.ecoleng.2021.106360
Giácoman-Vallejos, G., Ponce-Caballero, C., and Champagne, P. (2015). Pathogen Removal from Domestic and Swine Wastewater by Experimental Constructed Wetlands. Water Sci. Technol. 71, 1263–1270. doi:10.2166/wst.2015.102
Gorgoglione, A., and Torretta, V. (2018). Sustainable Management and Successful Application of Constructed Wetlands: A Critical Review. Sustainability 10, 3910. doi:10.3390/su10113910
Greenway, M. (2005). The Role of Constructed Wetlands in Secondary Effluent Treatment and Water Reuse in Subtropical and Arid Australia. Ecol. Eng. 25, 501–509. doi:10.1016/j.ecoleng.2005.07.008
Grinberga, L., Lauva, D., and Lagzdins, A. (2021). Treatment of Storm Water from Agricultural Catchment in Pilot Scale Constructed Wetland. Environ. Clim. Technol. 25, 640–649. doi:10.2478/rtuect-2021-0048
Guerrero, J., Mahmoud, A., Alam, T., Chowdhury, M. A., Adetayo, A., Ernest, A., et al. (2020). Water Quality Improvement and Pollutant Removal by Two Regional Detention Facilities with Constructed Wetlands in South Texas. Sustainability 12, 2844. doi:10.3390/su12072844
Gunawardena, J., Egodawatta, P., Ayoko, G. A., and Goonetilleke, A. (2013). Atmospheric Deposition as a Source of Heavy Metals in Urban Stormwater. Atmos. Environ. 68, 235–242. doi:10.1016/j.atmosenv.2012.11.062
Hafeznezami, S., Kim, J.-L., and Redman, J. (2012). Evaluating Removal Efficiency of Heavy Metals in Constructed Wetlands. J. Environ. Eng. 138, 475–482. doi:10.1061/(ASCE)EE.1943-7870.0000478
Han, Y. H., Lau, S. L., Kayhanian, M., and Stenstrom, M. K. (2006). Correlation Analysis Among Highway Stormwater Pollutants and Characteristics. Water Sci. Technol. 53, 235–243. doi:10.2166/wst.2006.057
Hathaway, J., Hunt, W., and Jadlocki, S. (2009a). Indicator Bacteria Removal in Storm-Water Best Management Practices in Charlotte, North Carolina. J. Environ. Eng. - J. Env. Eng-asce 135. doi:10.1061/(ASCE)EE.1943-7870.0000107
Hathaway, J., Hunt, W., Wright, J., and Jadlocki, S. (2009b). Field Evaluation of Indicator Bacteria Removal by Stormwater BMPs in North Carolina. World Environ Water Resour. Congr. 2009, 1–10. doi:10.1061/41036(342)112
Hathaway, J. M., Hunt, W. F., Graves, A. K., Bass, K. L., and Caldwell, A. (2011). Exploring Fecal Indicator Bacteria in a Constructed Stormwater Wetland. Water Sci. Technol. 63, 2707–2712. doi:10.2166/wst.2011.539
Hathaway, J. M., Hunt, W. F., Wright, J. D., and Jadlocki, S. J. (2009c). Field Evaluation of Indicator Bacteria Removal by Stormwater BMPs in North Carolina. World Environ Congr. 2009 Gt. Rivers 2009. doi:10.1061/41036(342)112
Headley, T. R., and Tanner, C. C. (2012). Constructed Wetlands with Floating Emergent Macrophytes: An Innovative Stormwater Treatment Technology. Crit. Rev. Environ. Sci. Techn. 42, 2261–2310. doi:10.1080/10643389.2011.574108
Hench, K. R., Bissonnette, G. K., Sexstone, A. J., Coleman, J. G., Garbutt, K., and Skousen, J. G. (2003). Fate of Physical, Chemical, and Microbial Contaminants in Domestic Wastewater Following Treatment by Small Constructed Wetlands. Water Res. 37, 921–927. doi:10.1016/S0043-1354(02)00377-9
Henze, M., and Comeau, Y. (2008). Wastewater Characterization,” in Biological Wastewater Treatment: Principles, Modelling And Design. London, United Kingdom: IWA Publishing.
Herath, I., and Vithanage, M. (2015). “Phytoremediation in Constructed Wetlands,”. Editors A. A. Ansari, S. S. Gill, R. Gill, G. R. Lanza, and L. Newman (Cham: Springer International Publishing), Vol. 2, 243–263. doi:10.1007/978-3-319-10969-5_21 Phytoremediation: Manag. Environ. Contaminants
Herrera-Cárdenas, J., Navarro, A. E., and Torres, E. (2016). Effects of Porous media, Macrophyte Type and Hydraulic Retention Time on the Removal of Organic Load and Micropollutants in Constructed Wetlands. J. Environ. Sci. Health A 51, 380–388. doi:10.1080/10934529.2015.1120512
Herrera-Melián, J. A., Mendoza-Aguiar, M., Guedes-Alonso, R., García-Jiménez, P., Carrasco-Acosta, M., and Ranieri, E. (2020). Multistage Horizontal Subsurface Flow vs. Hybrid Constructed Wetlands for the Treatment of Raw Urban Wastewater. Sustainability 12, 5102. doi:10.3390/su12125102
Hijosa-Valsero, M., Sidrach-Cardona, R., Martín-Villacorta, J., Cruz Valsero-Blanco, M., Bayona, J. M., and Bécares, E. (2011). Statistical Modelling of Organic Matter and Emerging Pollutants Removal in Constructed Wetlands. Bioresour. Techn. 102, 4981–4988. doi:10.1016/j.biortech.2011.01.063
Hill, V. R., and Sobsey, M. D. (2001). Removal of Salmonella and Microbial Indicators in Constructed Wetlands Treating Swine Wastewater. Water Sci. Technol. 44, 215–222. doi:10.2166/wst.2001.0832
House, C. H., Bergmann, B. A., Stomp, A. M., and Frederick, D. J. (1999). Combining Constructed Wetlands and Aquatic and Soil Filters for Reclamation and Reuse of Water. Ecol. Eng. 12, 27–38. doi:10.1016/S0925-8574(98)00052-4
Huang, J. J., Gao, X., Balch, G., Wootton, B., Jørgensen, S. E., and Anderson, B. (2015). Modelling of Vertical Subsurface Flow Constructed Wetlands for Treatment of Domestic Sewage and Stormwater Runoff by Subwet 2.0. Ecol. Eng. 74, 8–12. doi:10.1016/j.ecoleng.2014.10.027
Huang, X., Rippy, M. A., Mehring, A. S., Winfrey, B. K., Jiang, S. C., and Grant, S. B. (2018). Shifts in Dissolved Organic Matter and Microbial Community Composition Are Associated with Enhanced Removal of Fecal Pollutants in Urban Stormwater Wetlands. Water Res. 137, 310–323. doi:10.1016/j.watres.2018.03.020
Ibekwe, A. M., Ma, J., Murinda, S., and Reddy, G. B. (2016). Bacterial Community Dynamics in Surface Flow Constructed Wetlands for the Treatment of Swine Waste. Sci. Total Environ. 544, 68–76. doi:10.1016/j.scitotenv.2015.11.139
Jenssen, P. D., Mæhlum, T., Krogstad, T., and Vråle, L. (2005). High Performance Constructed Wetlands for Cold Climates. J. Environ. Sci. Health Part A 40, 1343–1353. doi:10.1081/ESE-200055846
Jokerst, A., Sharvelle, S. E., Hollowed, M. E., and Roesner, L. A. (2011). Seasonal Performance of an Outdoor Constructed Wetland for Graywater Treatment in a Temperate Climate. Water Environ. Res. 83, 2187–2198. doi:10.2175/106143011x12989211841412
Kabenge, I., Ouma, G., Aboagye, D., and Banadda, N. (2018). Performance of a Constructed Wetland as an Upstream Intervention for Stormwater Runoff Quality Management. Environ. Sci. Pollut. Res. 25, 36765–36774. doi:10.1007/s11356-018-3580-z
Karim, M. R., Manshadi, F. D., Karpiscak, M. M., and Gerba, C. P. (2004). The Persistence and Removal of Enteric Pathogens in Constructed Wetlands. Water Res. 38, 1831–1837. doi:10.1016/j.watres.2003.12.029
Kayhanian, M., Fruchtman, B. D., Gulliver, J. S., Montanaro, C., Ranieri, E., and Wuertz, S. (2012). Review of Highway Runoff Characteristics: Comparative Analysis and Universal Implications. Water Res. 46, 6609–6624. doi:10.1016/j.watres.2012.07.026
Kennedy, G., and Mayer, T. (2002). Natural and Constructed Wetlands in Canada: An Overview. Water Qual. Res. J. 37, 295–325. doi:10.2166/wqrj.2002.020
Kiiza, C., Pan, S.-q., Bockelmann-Evans, B., and Babatunde, A. (2020). Predicting Pollutant Removal in Constructed Wetlands Using Artificial Neural Networks (ANNs). Water Sci. Eng. 13, 14–23. doi:10.1016/j.wse.2020.03.005
Kizito, S., Lv, T., Wu, S., Ajmal, Z., Luo, H., and Dong, R. (2017). Treatment of Anaerobic Digested Effluent in Biochar-Packed Vertical Flow Constructed Wetland Columns: Role of media and Tidal Operation. Sci. Total Environ. 592, 197–205. doi:10.1016/j.scitotenv.2017.03.125
Knowles, P. R., and Davies, P. A. (2010). “A Finite Element Approach to Modelling the Hydrological Regime in Horizontal Subsurface Flow Constructed Wetlands for Wastewater Treatment,” in Water and Nutrient Management in Natural and Constructed Wetlands. Editor J. Vymazal (Dordrecht: Springer Netherlands), 85–101. doi:10.1007/978-90-481-9585-5_8
Lamori, J. G., Xue, J., Rachmadi, A. T., Lopez, G. U., Kitajima, M., Gerba, C. P., et al. (2019). Removal of Fecal Indicator Bacteria and Antibiotic Resistant Genes in Constructed Wetlands. Environ. Sci. Pollut. Res. 26, 10188–10197. doi:10.1007/s11356-019-04468-9
Langergraber, G. (2011a). Numerical Modelling: a Tool for Better Constructed Wetland Design? Water Sci. Technol. 64, 14–21. doi:10.2166/wst.2011.520
Langergraber, G. (2010b). “Process Based Models for Subsurface Flow Constructed Wetlands,” in Water and Nutrient Management in Natural and Constructed Wetlands. Editor J. Vymazal (Dordrecht: Springer Netherlands), 21–35. doi:10.1007/978-90-481-9585-5_3
Li, F., Lu, L., Zheng, X., Ngo, H. H., Liang, S., Guo, W., et al. (2014). Enhanced Nitrogen Removal in Constructed Wetlands: Effects of Dissolved Oxygen and Step-Feeding. Bioresour. Techn. 169, 395–402. doi:10.1016/j.biortech.2014.07.004
Li, J., Fan, J., Liu, D., Hu, Z., and Zhang, J. (2019). Enhanced Nitrogen Removal in Biochar-Added Surface Flow Constructed Wetlands: Dealing with Seasonal Variation in the north China. Environ. Sci. Pollut. Res. 26, 3675–3684. doi:10.1007/s11356-018-3895-9
Liang, W., Wu, Z. B., and Zhou, Q. (2002). Analysis of Substrate Microorganisms in the Constructed Wetland and Their Correlation with Wastewater Purification. China Environ. Sci. 19, 121
Liang, Y., Zhu, H., Bañuelos, G., Yan, B., Shutes, B., Cheng, X., et al. (2017). Removal of Nutrients in saline Wastewater Using Constructed Wetlands: Plant Species, Influent Loads and Salinity Levels as Influencing Factors. Chemosphere 187, 52–61. doi:10.1016/j.chemosphere.2017.08.087
Lu, S., Zhang, X., Wang, J., and Pei, L. (2016). Impacts of Different media on Constructed Wetlands for Rural Household Sewage Treatment. J. Clean. Prod. 127, 325–330. doi:10.1016/j.jclepro.2016.03.166
Lv, T., Carvalho, P. N., Zhang, L., Zhang, Y., Button, M., Arias, C. A., et al. (2017). Functionality of Microbial Communities in Constructed Wetlands Used for Pesticide Remediation: Influence of System Design and Sampling Strategy. Water Res. 110, 241–251. doi:10.1016/j.watres.2016.12.021
Lyu, T., Zhang, L., Xu, X., Arias, C. A., Brix, H., and Carvalho, P. N. (2018). Removal of the Pesticide Tebuconazole in Constructed Wetlands: Design Comparison, Influencing Factors and Modelling. Environ. Pollut. 233, 71–80. doi:10.1016/j.envpol.2017.10.040
Magalhães Filho, F. J. C., de Souza Filho, J. C. M., and Paulo, P. L. (2021). Multistage Constructed Wetland in the Treatment of Greywater under Tropical Conditions: Performance, Operation, and Maintenance. Recycling 6, 63. doi:10.3390/recycling6040063
Malaviya, P., and Singh, A. (2012). Constructed Wetlands for Management of Urban Stormwater Runoff. Crit. Rev. Environ. Sci. Techn. 42, 2153–2214. doi:10.1080/10643389.2011.574107
Mark, M., and Henriette, E. (2021). Stormwater Capture, Reuse, and Treatment for Multipurpose Benefits. World Environ. Water Resour. Congr. 2008, 1–10. doi:10.1061/40976(316)426
Martinez-Martinez, E., Nejadhashemi, A. P., Woznicki, S. A., and Love, B. J. (2014). Modeling the Hydrological Significance of Wetland Restoration Scenarios. J. Environ. Manage. 133, 121–134. doi:10.1016/j.jenvman.2013.11.046
Méndez, H., Geary, P. M., and Dunstan, R. H. (2009). Surface Wetlands for the Treatment of Pathogens in Stormwater: Three Case Studies at Lake Macquarie, NSW, Australia. Water Sci. Technol. 60, 1257–1263. doi:10.2166/wst.2009.470
Meng, Z., Chandrasena, G., Henry, R., Deletic, A., Kolotelo, P., and McCarthy, D. (2018). Stormwater Constructed Wetlands: A Source or a Sink of Campylobacter Spp. Water Res. 131, 218–227. doi:10.1016/j.watres.2017.12.045
Mietto, A., Politeo, M., Breschigliaro, S., and Borin, M. (2015). Temperature Influence on Nitrogen Removal in a Hybrid Constructed Wetland System in Northern Italy. Ecol. Eng. 75, 291–302. doi:10.1016/j.ecoleng.2014.11.027
Moore, T. L. C., and Hunt, W. F. (2012). Ecosystem Service Provision by Stormwater Wetlands and Ponds - A Means for Evaluation? Water Res. 46, 6811–6823. doi:10.1016/j.watres.2011.11.026
Moreira, F. D., and Dias, E. H. O. (2020). Constructed Wetlands Applied in Rural Sanitation: A Review. Environ. Res. 190, 110016. doi:10.1016/j.envres.2020.110016
Mustafa, A., Scholz, M., Harrington, R., and Carroll, P. (2009). Long-term Performance of a Representative Integrated Constructed Wetland Treating Farmyard Runoff. Ecol. Eng. 35, 779–790. doi:10.1016/j.ecoleng.2008.12.008
Naidoo, S., and Olaniran, A. (2014). Treated Wastewater Effluent as a Source of Microbial Pollution of Surface Water Resources. Ijerph 11, 249–270. doi:10.3390/ijerph110100249
Ouellet-Plamondon, C., Chazarenc, F., Comeau, Y., and Brisson, J. (2006). Artificial Aeration to Increase Pollutant Removal Efficiency of Constructed Wetlands in Cold Climate. Ecol. Eng. 27, 258–264. doi:10.1016/j.ecoleng.2006.03.006
Pelissari, C., Ávila, C., Trein, C. M., García, J., de Armas, R. D., and Sezerino, P. H. (2017a). Nitrogen Transforming Bacteria within a Full-Scale Partially Saturated Vertical Subsurface Flow Constructed Wetland Treating Urban Wastewater. Sci. Total Environ. 574, 390–399. doi:10.1016/j.scitotenv.2016.08.207
Pelissari, C., Guivernau, M., Viñas, M., de Souza, S. S., García, J., Sezerino, P. H., et al. (2017b). Unraveling the Active Microbial Populations Involved in Nitrogen Utilization in a Vertical Subsurface Flow Constructed Wetland Treating Urban Wastewater. Sci. Total Environ. 584-585 (585), 642–650. doi:10.1016/j.scitotenv.2017.01.091
Poudyal, S., Cochrane, T. A., and Bello-Mendoza, R. (2021). Carpark Pollutant Yields from First Flush Stormwater Runoff. Environ. Challenges 5, 100301. doi:10.1016/j.envc.2021.100301
Rajan, R. J., Sudarsan, J. S. S., and Nithiyanantham, S. (2018). Microbial Population Dynamics in Constructed Wetlands: Review of Recent Advancements for Wastewater Treatment. Environ. Eng. Res. 24, 181–190. doi:10.4491/eer.2018.127
Ranieri, E. (2003). Hydraulics of Sub-superficial Flow Constructed Wetlands in Semi Arid Climate Conditions. Water Sci. Technol. 47, 49–55. doi:10.2166/wst.2003.0670
Rochfort, Q. J., Watt, W. E., Marsalek, J., Anderson, B. C., and Crowder, A. A. (1997). Field-Scale Studies of Subsurface Flow Constructed Wetlands for Stormwater Quality Enhancement. Water Qual. Res. J. 32, 101–118. doi:10.2166/wqrj.1997.008
Ruppelt, J. P., Tondera, K., Wallace, S. J., Button, M., Pinnekamp, J., and Weber, K. P. (2020). Assessing the Role of Microbial Communities in the Performance of Constructed Wetlands Used to Treat Combined Sewer Overflows. Sci. Total Environ. 736, 139519. doi:10.1016/j.scitotenv.2020.139519
Russo, N., Marzo, A., Randazzo, C., Caggia, C., Toscano, A., and Cirelli, G. L. (2019). Constructed Wetlands Combined with Disinfection Systems for Removal of Urban Wastewater Contaminants. Sci. Total Environ. 656, 558–566. doi:10.1016/j.scitotenv.2018.11.417
Saeed, T., Miah, M. J., Khan, T., and Ove, A. (2020). Pollutant Removal Employing Tidal Flow Constructed Wetlands: Media and Feeding Strategies. Chem. Eng. J. 382, 122874. doi:10.1016/j.cej.2019.122874
Sakurai, K. S. I., Pompei, C. M. E., Tomita, I. N., Santos-Neto, Á. J., and Silva, G. H. R. (2021). Hybrid Constructed Wetlands as post-treatment of blackwater: An Assessment of the Removal of Antibiotics. J. Environ. Manage. 278, 111552. doi:10.1016/j.jenvman.2020.111552
Sánchez, O. (2017). Constructed Wetlands Revisited: Microbial Diversity in the -omics Era. Microb. Ecol. 73, 722–733. doi:10.1007/s00248-016-0881-y
Sandoval-Herazo, L., Alvarado-Lassman, A., Marín-Muñiz, J., Méndez-Contreras, J., and Zamora-Castro, S. A. (2018). Effects of the Use of Ornamental Plants and Different Substrates in the Removal of Wastewater Pollutants through Microcosms of Constructed Wetlands. Sustainability 10, 1594. doi:10.3390/su10051594
Sarmento, A. P., Borges, A. C., and de Matos, A. T. (2013). Effect of Cultivated Species and Retention Time on the Performance of Constructed Wetlands. Environ. Techn. 34, 961–965. doi:10.1080/09593330.2012.724210
Schierano, M. C., Panigatti, M. C., Maine, M. A., Griffa, C. A., and Boglione, R. (2020). Horizontal Subsurface Flow Constructed Wetland for Tertiary Treatment of Dairy Wastewater: Removal Efficiencies and Plant Uptake. J. Environ. Manage. 272, 111094. doi:10.1016/j.jenvman.2020.111094
Schmitt, N., Wanko, A., Laurent, J., Bois, P., Molle, P., and Mosé, R. (2015). Constructed Wetlands Treating Stormwater from Separate Sewer Networks in a Residential Strasbourg Urban Catchment Area: Micropollutant Removal and Fate. J. Environ. Chem. Eng. 3, 2816–2824. doi:10.1016/j.jece.2015.10.008
Semenov, M. V., Krasnov, G. S., Rybka, K. Y., Kharitonov, S. L., Zavgorodnyaya, Y. A., Yudina, A. V., et al. (2020). Spatial Changes in Microbial Communities along Different Functional Zones of a Free-Water Surface Wetland. Microorganisms 8, 1604. doi:10.3390/microorganisms8101604
Shruthi, R., and Shivashankara, G. P. (2021a). Effect of HRT and Seasons on the Performance of Pilot-Scale Horizontal Subsurface Flow Constructed Wetland to Treat Rural Wastewater. Water Pract. Technol. 17, 445–455. doi:10.2166/wpt.2021.104
Shruthi, R., and Shivashankara, G. P. (2021b). Investigation on the Performance Evaluation of Vertical Subsurface Flow Constructed Wetland for the Treatment of Rural Wastewater. Water Sci. Technol. 85, 16–26. doi:10.2166/wst.2021.507
Song, H., Qin, T., Wang, J., and Wong, T. H. F. (2019). Characteristics of Stormwater Quality in Singapore Catchments in 9 Different Types of Land Use. Water 11, 1089. doi:10.3390/w11051089
Stefanakis, A. I. (2016). Constructed Wetlands: Description and Benefits of an Eco-Tech Water Treatment System, 281–303. doi:10.4018/978-1-4666-9559-7.ch012
Stentström, T. A., and Carlander, A. (2001). Occurrence and Die-Off of Indicator Organisms in the Sediment in Two Constructed Wetlands. Water Sci. Technol. 44, 223–230. doi:10.2166/wst.2001.0833
Stottmeister, U., Wießner, A., Kuschk, P., Kappelmeyer, U., Kästner, M., Bederski, O., et al. (2003). Effects of Plants and Microorganisms in Constructed Wetlands for Wastewater Treatment. Biotechnol. Adv. 22, 93–117. doi:10.1016/j.biotechadv.2003.08.010
Struck, S., Selvakumar, A., and Borst, M. (20082008). Prediction of Effluent Quality from Retention Ponds and Constructed Wetlands for Managing Bacterial Stressors in StormWater Runoff. J. Irrig. Drain. Eng. - J. IRRIG DRAIN Eng-asce 134134 (567), 5. doi:10.1061/(ASCE)0733-943710.1061/(asce)0733-9437(2008)134:5(567)
Sultana, M.-Y., Mourti, C., Tatoulis, T., Akratos, C. S., Tekerlekopoulou, A. G., and Vayenas, D. V. (2016). Effect of Hydraulic Retention Time, Temperature, and Organic Load on a Horizontal Subsurface Flow Constructed Wetland Treating Cheese Whey Wastewater. J. Chem. Technol. Biotechnol. 91, 726–732. doi:10.1002/jctb.4637
Sun, H., Liu, F., Xu, S., Wu, S., Zhuang, G., Deng, Y., et al. (2017). Myriophyllum Aquaticum Constructed Wetland Effectively Removes Nitrogen in Swine Wastewater. Front. Microbiol. 8. doi:10.3389/fmicb.2017.01932
Tao, W., Sauba, K., Fattah, K. P., and Smith, J. R. (2017). Designing Constructed Wetlands for Reclamation of Pretreated Wastewater and Stormwater. Rev. Environ. Sci. Biotechnol. 16, 37–57. doi:10.1007/s11157-016-9419-5
Tietz, A., Hornek, R., Langergraber, G., Kreuzinger, N., and Haberl, R. (2007). Diversity of Ammonia Oxidising Bacteria in a Vertical Flow Constructed Wetland. Water Sci. Technol. 56, 241–247. doi:10.2166/wst.2007.505
Tram Vo, P., Ngo, H. H., Guo, W., Zhou, J. L., Nguyen, P. D., Listowski, A., et al. (2014). A Mini-Review on the Impacts of Climate Change on Wastewater Reclamation and Reuse. Sci. Total Environ. 494-495, 9–17. doi:10.1016/j.scitotenv.2014.06.090
Trang, N. T. D., Konnerup, D., Schierup, H.-H., Chiem, N. H., Tuan, L. A., and Brix, H. (2010). Kinetics of Pollutant Removal from Domestic Wastewater in a Tropical Horizontal Subsurface Flow Constructed Wetland System: Effects of Hydraulic Loading Rate. Ecol. Eng. 36, 527–535. doi:10.1016/j.ecoleng.2009.11.022
Urakawa, H., Dettmar, D. L., and Thomas, S. (2017). The Uniqueness and Biogeochemical Cycling of Plant Root Microbial Communities in a Floating Treatment Wetland. Ecol. Eng. 108, 573–580. doi:10.1016/j.ecoleng.2017.06.066
Uusheimo, S., Huotari, J., Tulonen, T., Aalto, S. L., Rissanen, A. J., and Arvola, L. (2018). High Nitrogen Removal in a Constructed Wetland Receiving Treated Wastewater in a Cold Climate. Environ. Sci. Technol. 52, 13343–13350. doi:10.1021/acs.est.8b03032
Vega De Lille, M. I., Hernández Cardona, M. A., Tzakum Xicum, Y. A., Giácoman-Vallejos, G., and Quintal-Franco, C. A. (2021). Hybrid Constructed Wetlands System for Domestic Wastewater Treatment under Tropical Climate: Effect of Recirculation Strategies on Nitrogen Removal. Ecol. Eng. 166, 106243. doi:10.1016/j.ecoleng.2021.106243
Verduzo Garibay, M., Fernández del Castillo, A., de Anda, J., Senés-Guerrero, C., and Gradilla-Hernández, M. S. (2021). Structure and Activity of Microbial Communities in Response to Environmental, Operational, and Design Factors in Constructed Wetlands. Int. J. Environ. Sci. Technol. doi:10.1007/s13762-021-03719-y
Vymazal, J. (2011). Constructed Wetlands for Wastewater Treatment: Five Decades of Experience. Environ. Sci. Technol. 45, 61–69. doi:10.1021/es101403q
Vymazal, J., Greenway, M., Tonderski, K., Brix, H., and Mander, Ü. (2006). “Constructed Wetlands for Wastewater Treatment,” in Wetlands and Natural Resource Management. Editors J. T. A. Verhoeven, B. Beltman, R. Bobbink, and D. F. Whigham (Berlin, Heidelberg: Springer Berlin Heidelberg), 69–96. doi:10.1007/978-3-540-33187-2_5
Vymazal, J. (2013). The Use of Hybrid Constructed Wetlands for Wastewater Treatment with Special Attention to Nitrogen Removal: A Review of a Recent Development. Water Res. 47, 4795–4811. doi:10.1016/j.watres.2013.05.029
Wadzuk, B. M., Rea, M., Woodruff, G., Flynn, K., and Traver, R. G. (2010). Water-Quality Performance of a Constructed Stormwater Wetland for All Flow Conditions1. JAWRA J. Am. Water Resour. Assoc. 46, 385–394. doi:10.1111/j.1752-1688.2009.00408.x
Wagner, T. V., Al-Manji, F., Xue, J., Wetser, K., de Wilde, V., Parsons, J. R., et al. (2021). Effects of Salinity on the Treatment of Synthetic Petroleum-Industry Wastewater in Pilot Vertical Flow Constructed Wetlands under Simulated Hot Arid Climatic Conditions. Environ. Sci. Pollut. Res. 28, 2172–2181. doi:10.1007/s11356-020-10584-8
Walker, D. J., and Hurl, S. (2002). The Reduction of Heavy Metals in a Stormwater Wetland. Ecol. Eng. 18, 407–414. doi:10.1016/S0925-8574(01)00101-X
Wang, F., Liu, Y., Ma, Y., Wu, X., and Yang, H. (2012). Characterization of Nitrification and Microbial Community in a Shallow moss Constructed Wetland at Cold Temperatures. Ecol. Eng. 42, 124–129. doi:10.1016/j.ecoleng.2012.01.006
Wang, J., Wang, W., Xiong, J., Li, L., Zhao, B., Sohail, I., et al. (2021a). A Constructed Wetland System with Aquatic Macrophytes for Cleaning Contaminated Runoff/storm Water from Urban Area in Florida. J. Environ. Manage. 280, 111794. doi:10.1016/j.jenvman.2020.111794
Wang, J., Xia, L., Chen, J., Wang, X., Wu, H., Li, D., et al. (2021b). Synergistic Simultaneous Nitrification-Endogenous Denitrification and EBPR for Advanced Nitrogen and Phosphorus Removal in Constructed Wetlands. Chem. Eng. J. 420, 127605. doi:10.1016/j.cej.2020.127605
Wang, L., Peng, J., Wang, B., and Cao, R. (2005). Performance of a Combined Eco-System of Ponds and Constructed Wetlands for Wastewater Reclamation and Reuse. Water Sci. Technol. 51, 315–323. doi:10.2166/wst.2005.0490
Wang, P., Zhang, H., Zuo, J., Zhao, D., Zou, X., Zhu, Z., et al. (2016a). A Hardy Plant Facilitates Nitrogen Removal via Microbial Communities in Subsurface Flow Constructed Wetlands in Winter. Sci. Rep. 6, 33600. doi:10.1038/srep33600
Wang, Q., Xie, H., Ngo, H. H., Guo, W., Zhang, J., Liu, C., et al. (2016b). Microbial Abundance and Community in Subsurface Flow Constructed Wetland Microcosms: Role of Plant Presence. Environ. Sci. Pollut. Res. 23, 4036–4045. doi:10.1007/s11356-015-4286-0
Witthayaphirom, C., Chiemchaisri, C., Chiemchaisri, W., Ogata, Y., Ebie, Y., and Ishigaki, T. (2020). Organic Micro-pollutant Removals from Landfill Leachate in Horizontal Subsurface Flow Constructed Wetland Operated in the Tropical Climate. J. Water Process Eng. 38, 101581. doi:10.1016/j.jwpe.2020.101581
Wu, H., Zhang, J., Ngo, H. H., Guo, W., Hu, Z., Liang, S., et al. (2015). A Review on the Sustainability of Constructed Wetlands for Wastewater Treatment: Design and Operation. Bioresour. Techn. 175, 594–601. doi:10.1016/j.biortech.2014.10.068
Wu, S., Carvalho, P. N., Müller, J. A., Manoj, V. R., and Dong, R. (2016). Sanitation in Constructed Wetlands: A Review on the Removal of Human Pathogens and Fecal Indicators. Sci. Total Environ. 541, 8–22. doi:10.1016/j.scitotenv.2015.09.047
Xu, J.-C., Chen, G., Huang, X.-F., Li, G.-M., Liu, J., Yang, N., et al. (2009). Iron and Manganese Removal by Using Manganese Ore Constructed Wetlands in the Reclamation of Steel Wastewater. J. Hazard. Mater. 169, 309–317. doi:10.1016/j.jhazmat.2009.03.074
Xu, M., Liu, W., Li, C., Xiao, C., Ding, L., Xu, K., et al. (2016). Evaluation of the Treatment Performance and Microbial Communities of a Combined Constructed Wetland Used to Treat Industrial Park Wastewater. Environ. Sci. Pollut. Res. 23, 10990–11001. doi:10.1007/s11356-016-6181-8
Yi, X.-H., Jing, D.-D., Wan, J., Ma, Y., and Wang, Y. (2016). Temporal and Spatial Variations of Contaminant Removal, Enzyme Activities, and Microbial Community Structure in a Pilot Horizontal Subsurface Flow Constructed Wetland Purifying Industrial Runoff. Environ. Sci. Pollut. Res. 23, 8565–8576. doi:10.1007/s11356-016-6083-9
Zhang, D. Q., Jinadasa, K. B. S. N., Gersberg, R. M., Liu, Y., Ng, W. J., and Tan, S. K. (2014). Application of Constructed Wetlands for Wastewater Treatment in Developing Countries - A Review of Recent Developments (2000-2013). J. Environ. Manage. 141, 116–131. doi:10.1016/j.jenvman.2014.03.015
Zhang, L., Lyu, T., Zhang, Y., Button, M., Arias, C. A., Weber, K. P., et al. (2018). Impacts of Design Configuration and Plants on the Functionality of the Microbial Community of Mesocosm-Scale Constructed Wetlands Treating Ibuprofen. Water Res. 131, 228–238. doi:10.1016/j.watres.2017.12.050
Zhang, L., and Mitsch, W. J. (2005). Modelling Hydrological Processes in Created Freshwater Wetlands: an Integrated System Approach. Environ. Model. Softw. 20, 935–946. doi:10.1016/j.envsoft.2004.03.020
Zhou, X., Chen, Z., Li, Z., and Wu, H. (2020). Impacts of Aeration and Biochar Addition on Extracellular Polymeric Substances and Microbial Communities in Constructed Wetlands for Low C/N Wastewater Treatment: Implications for Clogging. Chem. Eng. J. 396, 125349. doi:10.1016/j.cej.2020.125349
Keywords: water reclamation and reuse, constructed wetlands, stormwater, emergent macrophytes, pollutant reduction
Citation: Biswal BK and Balasubramanian R (2022) Constructed Wetlands for Reclamation and Reuse of Wastewater and Urban Stormwater: A Review. Front. Environ. Sci. 10:836289. doi: 10.3389/fenvs.2022.836289
Received: 15 December 2021; Accepted: 17 February 2022;
Published: 09 March 2022.
Edited by:
Saleem Saleh AlSaleem, Qassim University, Saudi ArabiaReviewed by:
Hongbo Liu, University of Shanghai for Science and Technology, ChinaHusnain Haider, Qassim University, Saudi Arabia
Bing Guo, University of Surrey, United Kingdom
Copyright © 2022 Biswal and Balasubramanian. This is an open-access article distributed under the terms of the Creative Commons Attribution License (CC BY). The use, distribution or reproduction in other forums is permitted, provided the original author(s) and the copyright owner(s) are credited and that the original publication in this journal is cited, in accordance with accepted academic practice. No use, distribution or reproduction is permitted which does not comply with these terms.
*Correspondence: Basanta Kumar Biswal, Y2VlYmJrQG51cy5lZHUuc2c=; Rajasekhar Balasubramanian, Y2VlcmJhbGFAbnVzLmVkdS5zZw==