- School of the Environment, Washington State University Vancouver, Vancouver, WA, United States
Accurately quantifying the diffusive flux of CH4 between sediments and the overlying water column is crucial when constructing CH4 budgets in lakes and reservoirs. Although a variety of ex situ and in situ techniques exist for determining this flux, no reviews have provided a comprehensive, comparative overview of these approaches or discussed implications of measurement method on flux estimation. Here, we critically review methods applied in 163 peer-reviewed studies to estimate diffusive CH4 fluxes from lake sediments, including sediment incubations, benthic chambers, and modeling approaches applied in the sediment or water column. For each method, we summarize the approach, discuss limitations and advantages, and summarize published comparisons between different methods. In addition, we examine how method limitations have likely shaped knowledge gaps in current understanding of lake CH4 dynamics. Finally, we call for the development and application of new methods, along with additional testing and intercomparison of existing methods, in order to advance understanding of lake CH4 fluxes.
1 Introduction
Lakes and reservoirs are collectively responsible for an estimated 9%–27% (70–175 Tg) of global methane (CH4) emissions annually (Rosentreter et al., 2021). The majority of CH4 in these ecosystems is thought to be produced in sediments (Peeters et al., 2019), where high organic carbon availability and anoxic conditions favor methanogenesis (Bastviken, 2009). Methane in sediments can then cross the sediment-water interface (SWI) via diffusion or ebullition (bubbling) into the water column, from whence it may ultimately reach the atmosphere. Typically, up to 50% of CH4 emissions from lakes and reservoirs occurs via diffusion rather than ebullition (Bastviken et al., 2004; Bastviken et al., 2008; Deemer et al., 2016). Although bubbling from sediments is certainly an important pathway for CH4 transport (DelSontro et al., 2010; Beaulieu et al., 2016), here we focus on the diffusive flux of CH4 from sediments, which is a critical component of understanding lentic greenhouse gas cycling and emissions.
Methane diffusion from lake and reservoir sediments is highly variable in both space and time. In a synthesis of measurements from 23 lakes and reservoirs, diffusive CH4 fluxes across the SWI varied up to three orders of magnitude across and within systems (Adams, 2005). The wide variability in rates is due, in part, to physical and biological factors that influence rates of CH4 production (methanogenesis) and oxidation (methanotrophy) within sediments, as well as controls on the diffusive transport of CH4 out of sediments. For example, greater sediment CH4 production rates have been linked to higher lake productivity (West et al., 2016; D’Ambrosio and Harrison, 2021), warmer temperatures (Duc et al., 2010), organic matter availability (Berberich et al., 2019; Praetzel et al., 2020), and anoxic conditions above and within sediments (Liikanen and Martikainen, 2003; Huttunen et al., 2006). Methane oxidation, which can consume significant amounts of CH4 at the SWI or deeper in the sediment profile, is controlled by the availability of CH4 and electron acceptors such as oxygen, nitrate, iron oxides, manganese oxides, and sulfate (Kuivila et al., 1988; Clayer et al., 2016; van Grinsven et al., 2020). The diffusive release of CH4 out of sediments is, in turn, influenced by the net supply of CH4 (i.e., production—oxidation), and can be affected by conditions and processes above sediments such as the CH4 concentration in water overlying sediments, oxidation at the sediment surface (Bosse et al., 1993; Rolletschek, 1997), near-bed turbulence (D’Ambrosio, 2022), surface waves (Hofmann et al., 2010), and sediment resuspension (Bussmann, 2005).
The wide range of methods used to measure the CH4 diffusion from sediments may play an important role in the large variability in reported fluxes. Approaches such as sediment incubations, sediment models, and water column models have differing spatiotemporal resolutions, sampling requirements, and limitations, all of which influence resulting flux measurements. Although explicit comparisons of multiple approaches for measuring CH4 emission across the air-water interface have been extensive (St. Louis et al., 2000; Schubert et al., 2012; Deemer et al., 2016), there is no similar synthesis of methods for measuring for CH4 diffusion across the SWI. A critical examination of methodologies used to estimate this flux is needed to: 1) understand the advantages and disadvantages of current approaches; 2) recognize how limitations of common techniques shape gaps in our current view of CH4 dynamics in lakes and reservoirs; and 3) identify future method developments needed to advance understanding of the supply and transport of CH4 in lacustrine systems.
Here, we critically review methodologies from 163 studies reporting CH4 diffusive flux and/or methanogenesis in lake or reservoir sediments (references provided in the Supplementary Data File). From these studies, we classify typical methods for measuring this flux into three broad categories: sediment incubations and benthic chambers, modeling approaches applied in the sediment, and modeling approaches applied in the water column. We summarize each approach, describe the utility, advantages, and disadvantages for each, and examine previous studies explicitly comparing measurements from multiple techniques. Finally, we discuss how existing approaches have shaped knowledge gaps in lacustrine CH4 cycling, and we provide recommendations for future methodological developments needed to improve the quantification of sediment CH4 flux and its role in lake and reservoir carbon budgets.
2 Methodologies
2.1 Sediment Incubations & Benthic Chambers
2.1.1 Approach Summary
Sediment incubations have historically been the most common approach for estimating diffusive CH4 fluxes from lake sediments (Figure 1). Incubation-based estimates can be categorized as ex situ sediment incubations, typically performed in the lab, or in situ incubations, usually performed by deploying benthic chambers to the lakebed.
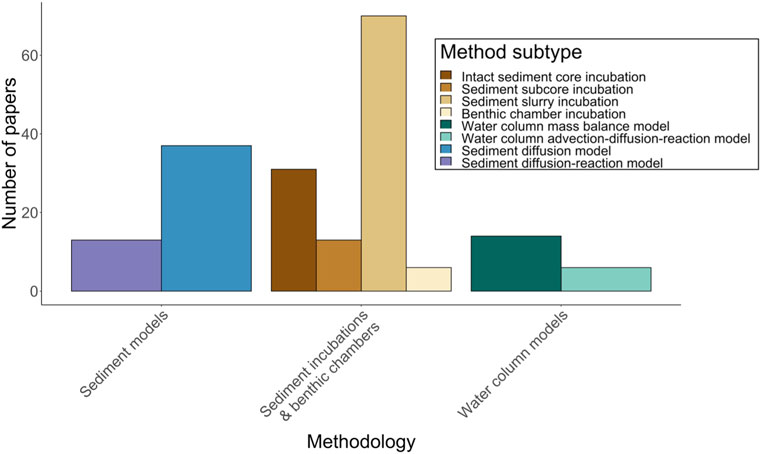
FIGURE 1. Number of studies measuring sediment methanogenesis and/or the flux of CH4 from sediments. Broad subcategories of methodology are shown in legend. Ten sediment incubation studies are excluded from the plot because incubation subtype could not be determined, however they are included in the Supplementary Data File of references (Supplementary Table S1).
Ex situ incubations are the most common and measure CH4 flux in vitro from intact sediment cores, subcores, or sediment-water slurries (Figure 1). Intact cores can be collected via piston, gravity, or box coring, and may be segmented into smaller subcores prior to incubation (Mudroch and MacKnight, 1991). Slurries are subsampled from a core or sediment grab and then homogenized prior to incubation, often through mixing with lake water or deionized water. Sediment cores, subcores, or slurries are placed in incubation chambers, such as acrylic tubes for cores (Sturm et al., 2014) or glass vials for subcores or slurries (Wagener et al., 1990), then sealed with gastight stoppers. Vial headspaces above sediments may be filled partially or completely with lake water or deionized water. Anoxic or oxic conditions are then established in the chambers prior to incubation, depending on the study objectives. Anoxic incubations are often flushed and evacuated with an inert gas (e.g., N2 or He; Liikanen et al., 2002a; Praetzel et al., 2020), whereas oxic incubations use air (Sweerts et al., 1991; Chmiel et al., 2016). Incubations usually take place either in a temperature-controlled water bath (Kelly and Chynoweth, 1981) or at in situ depth in the water column (Algesten et al., 2005). Core incubation chambers are often, although not always, equipped with a magnetic stirrer that gently mixes water above the core without disturbing the SWI (Frenzel et al., 1990; Leal et al., 2007). Some core incubations also use constant flow-through systems that pump lake water from an outside reservoir into the overlying water of cores. This maintains oxic or anoxic conditions throughout the incubation and prevents the accumulation or depletion of compounds that may alter CH4 production or consumption rates (Liikanen et al., 2002d; Bussmann, 2005).
A small subset of incubations is performed in situ using a benthic chamber approach (sometimes called a static chamber; Figure 1). Benthic chambers are open-bottomed boxes or cylinders constructed of acrylic, steel, or plastic, and are implanted several centimeters into the sediment for in situ incubation (Viollier et al., 2003). Chambers can be installed manually with scuba divers (Duchemin et al., 1995) or using a benthic lander, which is designed to implant the chamber remotely after being lowered to a lake bottom (Devol, 1987; Kuivila et al., 1988; Urban et al., 1997; Maerki et al., 2009). Chamber designs often include pumps or paddles that continuously circulate water within a chamber throughout the incubation (Devol, 1987; Colas et al., 2021).
Incubation duration varies substantially, in part as a function of technique. Ex situ incubations typically last days (Jones et al., 1982; Hershey et al., 2015) to weeks (Liikanen et al., 2002b; Dan et al., 2004); however some can last months (Martinez-Cruz et al., 2017; Valle et al., 2018) to years (Nozhevnikova et al., 2007; Isidorova et al., 2019). Benthic chamber deployments are usually shorter, lasting from hours to a day (Kuivila et al., 1988; Urban et al., 1997). Incubations are generally sampled at multiple time points to determine CH4 concentrations, with the rate of change in concentrations over time used to calculate fluxes. For ex situ incubations, headspace gas or water overlying sediment can be sampled using syringes, or flow from an external reservoir may be used to push water out of the incubation chamber to be sampled. For benthic chambers, samples of water are extracted either manually via tubing/pumps to the surface (Yavitt et al., 1992; Duchemin et al., 1995) or automatically with programmable syringes (Devol, 1987; Kuivila et al., 1988; Urban et al., 1997; Maerki et al., 2009).
Samples collected during incubations are analyzed for CH4 concentration using gas chromatography or laser absorption spectrometry. Samples in the gas phase can be analyzed immediately. Samples in liquid phase are first prepared using headspace equilibration, a technique which injects an inert gaseous headspace (often ultrapure helium), and then agitates the water in order to equilibrate dissolved CH4 with the gaseous phase prior to analysis (McAullife, 1971; Magen et al., 2014). Henry’s law is then used to determine the amount of CH4 originally present in the liquid phase.
The rate at which CH4 is produced in the incubation over time is used to estimate sediment diffusive flux. In benthic chambers, intact core, and some subcore incubations, the production rate is typically expressed in areal units (i.e., CH4 produced per m2 of core horizontal cross-sectional area incubated) and therefore assumed to be equivalent to diffusive CH4 flux across the SWI (
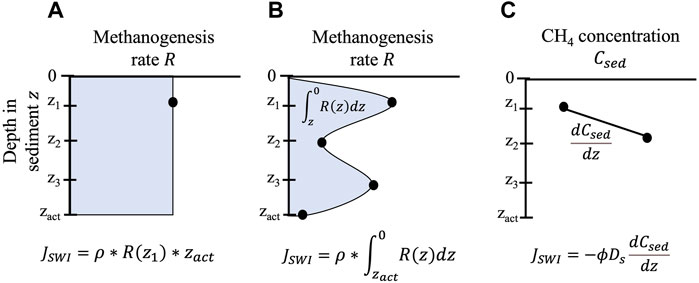
FIGURE 2. Multiple approaches for measuring diffusive flux from sediments (
2.1.2 Approach Advantages
Incubations and benthic chambers provide a highly controlled, customizable environment for researchers to manipulate environmental conditions, and thus they are often used to evaluate drivers of CH4 processing (Table 1). For example, previous incubation studies have investigated how sediment CH4 flux and/or methanogenesis rates respond to shifting temperatures (Zeikus and Winfrey, 1976; Duc et al., 2010), nutrient concentrations (Stadmark and Leonardson, 2007; Rodriguez et al., 2018), organic matter deposition (West et al., 2012; Grasset et al., 2018), oxygen availability (Liikanen et al., 2002c; Liikanen and Martikainen, 2003), and supply of electron acceptors likely to be involved in CH4 oxidation (Karvinen et al., 2015; Rissanen et al., 2017). These experimental manipulations are impractical or impossible to apply in most benthic chamber experiments, yet they are important for identifying which environmental drivers exert control on methanogenesis and methanotrophy within lake sediments (Bastviken, 2009; Borrel et al., 2011). Additionally, incubations are unique in that they can be used to partition out gross rates of CH4 production and oxidation, rather than net rates. This is usually done by injecting the incubation with isotopically-labeled methanogenic or methanotrophic substrate (e.g., 14C-labeled acetate/bicarbonate or 14CH4, respectively) and tracking the radioactivity and concentration of CH4 in the incubation over time (Kuivila et al., 1989; Nüsslein et al., 2001; Pimenov et al., 2010).
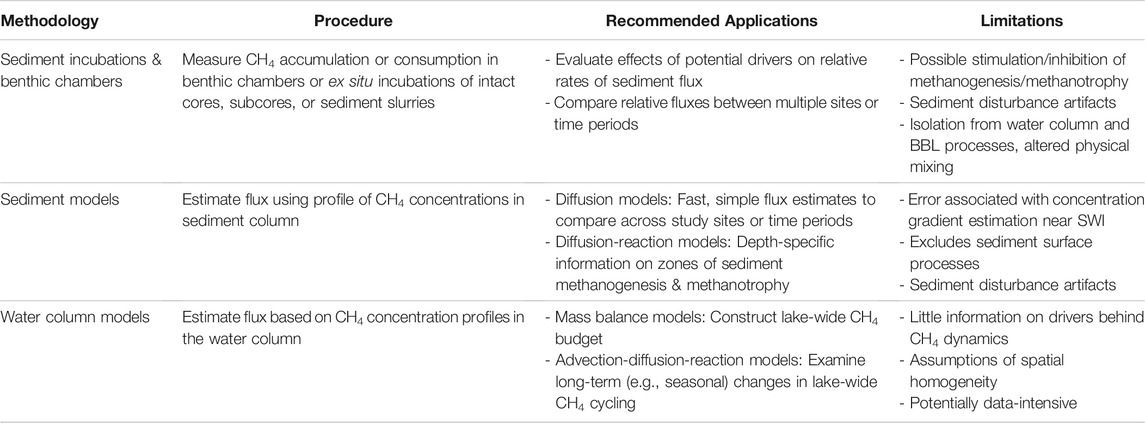
TABLE 1. Summary of procedures, recommended applications, and limitations of each method used to measure diffusive CH4 flux from lake sediments.
Incubations are also popular in part due to their simplicity, reproducibility, and relatively simple sampling requirements. Benthic chambers and/or ex situ incubation containers are generally constructed from low-cost materials and incubations can be repeated with sediments from (or at) multiple sampling locations, time points, and study systems (Table 1). Furthermore, CH4 flux from an incubation can be determined from just a few samples of CH4 concentrations over time, typically just a few hours to a day, or less. Other approaches, such as water column models (Section 2.3), often require significantly more data such as sediment ebullition estimates, temperature profiles in the water column, and/or CH4 oxidation measurements.
Lastly, although here we focus on methods for measuring CH4 diffusion from sediments, an advantage of incubations is that some set-ups can be modified to measure diffusion and ebullition simultaneously (Liikanen et al., 2002c). While a full discussion of these incubation designs is beyond the scope of this review, the capacity to measure diffusive and ebullitive flux from sediments can be advantageous given bubbling is an important CH4 transport pathway in many lakes and reservoirs (DelSontro et al., 2011, 2015).
2.1.3 Approach Limitations
An important limitation of incubations and benthic chambers is that they provide a spatiotemporal snapshot of CH4 diffusion from sediments. Rates from this method are relevant to the sediment incubated, which typically has a surface area of a few square centimeters to a square meter or less (Figure 3). Assumptions that flux estimates based on incubations are applicable lake-wide are questionable, given previous work has found significant differences in sediment CH4 production and fluxes between profundal and littoral sites (Liikanen et al., 2003; Li et al., 2021) or between locations with different organic matter inputs (Berberich et al., 2019; Praetzel et al., 2020). Rate estimates based on incubations also represent a flux averaged only over the incubation duration, usually hours or days (Figure 3). Incubations therefore do not generally account for temporal shifts across seasons (Nüsslein and Conrad, 2000; Itoh et al., 2015) or during lake stratification and mixing (Liikanen et al., 2002b; Vachon et al., 2019). Additionally, volumetric or gravimetric CH4 production rates measured in incubations are often converted into a flux by assuming rates are constant over a specified active layer depth (Figure 2A). This assumption runs counter to many studies demonstrating that methanogenesis changes significantly with lake sediment depth (Chan et al., 2005; Lofton et al., 2015; Yang et al., 2017; Praetzel et al., 2020).
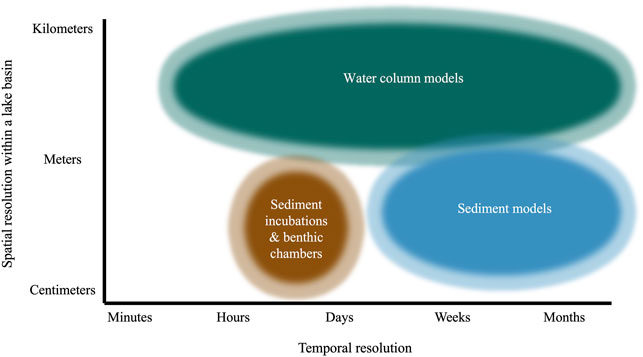
FIGURE 3. Conceptual map showing the typical temporal and spatial resolution of common methodologies included in Figure 1. Temporal resolution represents the typical time period over which measurements from each method are made or averaged over. Spatial resolution indicates the spatial scale over which measurements from each method are made or averaged across within an individual lake basin.
Ex situ incubations and benthic chambers also largely isolate sediments from lake-scale processes that may influence flux across the SWI (Table 1). For example, internal waves can drive fluctuations in turbulence and mixing in the boundary layer overlying sediments (Henderson, 2016). These fluctuations in near-bed conditions have been demonstrated to influence fluxes of oxygen, nitrate, and CH4 across the SWI on hourly to daily time scales (Lorke et al., 2003; Brand et al., 2008; Bryant et al., 2010; D’Ambrosio, 2022). Internal waves can also drive redox changes at the SWI, which may influence within-sediment rates of methanogenesis and methanotrophy (Frindte et al., 2013; Frindte et al., 2015). The effects of such boundary layer and surface sediment dynamics on flux are not considered within traditional incubation setups. Furthermore, although surface waves and sediment resuspension have also been tied to variations in diffusive CH4 release from lake sediments (Bussmann, 2005; Hofmann et al., 2010), only one study has simulated the effects of resuspension in their incubation microcosm design (Bussmann 2005).
For ex situ incubations specifically, sampling artifacts from sediment collection and preparation are important to consider (Table 1). Previous work has posited that sediment slurrying can stimulate methanogenesis (Kelly and Chynoweth, 1980; Frenzel et al., 1990) or inhibit methanotrophy (Su et al., 2019) compared to in situ rates (Table 1). In contrast, intact and subcore incubations avoid slurrying in order to preserve sediment layering and structure, in part because methanogenesis and methanotrophy change significantly with sediment depth (Chan et al., 2005; Martinez-Cruz et al., 2018). However, in gas-rich sediments, stratigraphy can be significantly disturbed by core sampling due to changes in hydrostatic pressure and temperature during core withdrawal (Dück et al., 2019b). It is similarly challenging to collect cores in soft-bottom sediments without altering sediment structure (Blomqvist, 1991). Given these limitations, incubations are best suited for measuring relative (rather than absolute) rates of diffusive CH4 release from sediments (Table 1).
Lastly, fluxes estimated with incubations are sensitive to incubation duration because conditions within the chamber can change over time. For example, oxygen may be depleted over the course of benthic chamber incubations (Duchemin et al., 1995), which may affect rates of CH4 production and oxidation given the sensitivity of methanogens and methanotrophs to oxygen availability (Borrel et al., 2011; Thottathil et al., 2019). Such oxygen depletion can be avoided by limiting the duration of benthic chamber deployments (Urban et al., 1997) and by monitoring oxygen concentrations in benthic chambers. For ex situ incubations, an initial lag phase of CH4 production may also occur as methanogens or methanotrophs acclimate to vial conditions and/or compete with other microorganisms present (Torres et al., 2011; Grasset et al., 2021). However, lag phases are not observed in all incubations (Chan et al., 2005; Valle et al., 2018; Li et al., 2020). Furthermore, CH4 production may decrease over time in long-term incubations (Isidorova et al., 2019), likely due to the gradual depletion of methanogenic precursors such as acetate. Consequently, ex situ incubations that are shorter than a typical lag phase or long enough to deplete methanogenic substrates may underestimate rates of CH4 release from sediments.
2.2 Sediment CH4 Models
2.2.1 Approach Summary
Modeling CH4 in the sediment column is a common method for estimating CH4 flux across the SWI of lakes and reservoirs (Figure 1). Sediment models for estimating CH4 fluxes across the SWI can be considered as falling into one of two main categories: 1) diffusion models, which estimate CH4 flux solely based on molecular diffusion; and 2) diffusion-reaction models, which also account for the effects of microbial reactions and/or bioirrigation on CH4 flux (Figure 1). In the former case, flux is estimated between two discrete depths in the sediment column using Fick’s first law of diffusion (Figure 2C):
where
Beyond molecular diffusion considered in Eq. 1, processes such as bioirrigation or microbial reactions may also affect CH4 distribution in sediments. A more comprehensive diffusion-reaction model can account for how these processes work in concert with molecular diffusion to influence CH4 concentrations in the sediment. Normally, such a diffusion-reaction model is applied at many discrete vertical layers in the sediment column, rather than the two discrete depths considered in the diffusion model of Eq. 1. First, the effect of molecular diffusion on CH4 distribution throughout the sediment column is considered, according to Fick’s second law of diffusion:
where
In some cases, an additional term is added to Eq. 3 to account for the effects of bioirrigation on CH4 transport (Bartosiewicz et al., 2016, 2021; Clayer et al., 2016). Bioirrigation and bioturbation describe how biologically driven water circulation through sediments and sediment mixing, respectively, affect solute transport between sediment porewater and the overlying water (Kristensen et al., 2012). Burrows serve as conduits between the sediment porewater and the overlying water, creating a concentration gradient that drives additional diffusive transport of CH4:
where
From Eq. 5, there are multiple routes to calculate a flux across the SWI. Both PROFILE and REC solve for a depth profile of
where
All sediment models covered here require measurements of CH4 concentrations in the sediment porewater, which can be obtained a variety of ways. The simplest technique is collecting an intact sediment core, subsampling into core slices by depth, then measuring CH4 concentration in each subsample using headspace equilibration (Section 2.1.1). Another technique measures CH4 concentrations using a small probe inserted at various depths in the sediment core (Bussmann and Schink, 2006). Here, methane from the sediment diffuses across the permeable membrane of a probe inserted at a specific sediment depth, and a constant flow of carrier gas to the probe flushes the CH4 to a gas chromatograph for determination of concentration. Other approaches rely on the extraction of sediment porewater, which can be analyzed for CH4 concentration with headspace equilibration (Section 2.1.1). Porewater can be extracted with squeezers, metal or plastic devices that use pistons or gas pressure to compress sediment and force out interstitial porewater. Porewater from different depths in a core may be extracted using squeezers on various core subsections (Reeburgh, 1967) or by using squeezers built to compress entire cores and fitted with ports at various sampling depths (Jahnke, 1988). A recently developed technique involves using a modified squeezer to subsample sediment from different depths into copper tubing, then centrifuging aliquots from the tubes to extract the porewater (Tyroller et al., 2016). Alternatively, small, porous Rhizon tubes can be inserted into different depths of the sediment. Porewater is then drawn into the tubes using suction from an attached vacuum pump, syringe, or evacuated test tube. Rhizons were originally developed for use in terrestrial soils, but have subsequently been adapted to sample porewater in saturated sediments from aquatic systems (Seeberg-Elverfeldt et al., 2005).
Porewater may also be sampled without collecting a core by deploying a “peeper” directly into the sediment (Hesslein, 1976). Peepers are acrylic samplers containing an array of small wells spaced several centimeters apart in the vertical. Sampling wells are filled with anoxic water and covered with a permeable membrane made of cellulose-acetate or polysulfone. Peepers are typically left in the sediment for several weeks to equilibrate via dialysis, then sampled and analyzed for CH4 using headspace equilibration.
2.2.2 Approach Advantages
Sediment models are highly customizable depending on the lake system and level of sampling effort required. For example, a simple diffusion model (Eq. 1) to estimate flux requires sample collection as basic as measuring one CH4 concentration gradient close to the SWI. Due to these relatively simple data requirements, sediment diffusion models are often employed as a fast, easy way to compare fluxes across multiple lakes (Adams, 2005; Huttunen et al., 2006), sampling sites (Zhang et al., 2020; Li et al., 2021), or time periods (Table 1; Rolletschek 1997).
In contrast, more sophisticated diffusion-reaction models (Eqs 3–5) can be constructed to account for the effects of microbial CH4 production, oxidation, bioturbation, and/or bioirrigation on CH4 transport out of sediments. Although these more comprehensive models require detailed sampling of CH4 concentrations in the sediment column, their implementation can be made easier with freely available software used to solve the differential equations involved, such as PROFILE or REC (Berg et al., 1998; Lettmann et al., 2012). Diffusion-reaction models also can be used to solve for depth profiles of methanogenesis and methanotrophy in the sediment, which are powerful tools for examining the locations, controls, and microbial communities involved in CH4 supply and transport (Table 1). For example, comparing rates of CH4 production and oxidation in the sediment profile with observed fluxes across the SWI can highlight the importance of methanotrophy in substantially reducing CH4 release into the hypolimnion (Koschorreck et al., 2008; Rahalkar et al., 2009; Norði et al., 2013).
2.2.3 Approach Limitations
Estimates from sediment modeling rely on porewater CH4 concentrations (Eq. 1), which are the net result of equilibration with the surrounding sediment column and overlying water. Measurements are therefore only representative of the sediment sampled (usually centimeters to a meter or less) and its close surroundings (likely several meters; Figure 3). Accordingly, assumptions that measurements from sediment models characterize fluxes lake-wide should be interpreted with caution, given the significant spatial variability in sediment CH4 dynamics across different sites within the same lake (Section 2.1.3).
Moreover, flux estimates from sediment models should be considered an average across weeks to months (Figure 3), the time scale on which equilibration of CH4 concentrations in sediments typically occurs (Harper et al., 1997). Therefore, similar to incubations, these estimates do not account for short-term fluctuations in processes that may influence CH4 diffusion across the SWI (Section 2.1.3). Unless modeling is repeated across multiple dates and sites, changes across seasons due to changing temperature, deposition of organic matter, or other environmental factors (Thebrath et al., 1993; Murase and Sugimoto, 2002; Yang et al., 2018) are also not considered. This seasonal variability may be particularly pronounced in lakes that thermally stratify and develop seasonal hypolimnetic hypoxia, as the resulting shifts in oxygen availability to surface sediments can profoundly influence sediment CH4 oxidation and flux (Liikanen et al., 2003).
Typical techniques for measuring CH4 concentrations in porewater, required for sediment modeling, also come with logistical hurdles and sampling artifacts (Table 1). Peepers can be challenging to deploy, since they require 1–3 weeks for equilibration and may need to be installed by scuba divers (Hesslein, 1976; Bufflap and Allen, 1995). Gas loss is a common sampling artifact during sediment core collection (Paull et al., 2000), peeper extraction (Adams, 2005), and headspace equilibration (Tyroller et al., 2016), resulting in underestimated CH4 concentrations (and therefore inaccurate gradients). Exposure of sediment cores and/or peepers to oxygen during collection (Bufflap and Allen, 1995) is another potential problem that may stimulate methanotrophy and therefore influence sampled concentrations. Applying noble gas tracer (Tyroller et al., 2016) or freeze coring (Dück et al., 2019a) methods to analyze CH4 concentrations in sediments may address some of these sampling artifacts.
In particular, sediment diffusion models (Eq. 1) are inherently limited by the spatial resolution of porewater sampling. The CH4 gradient used in Eq. 1 should be measured as close to the SWI as possible, because CH4 concentrations can change rapidly within just a few millimeters of the sediment surface (Bussmann and Schink, 2006). However, accurately sampling CH4 concentrations close to the SWI can be challenging with conventional porewater measurement techniques (Table 1). For example, peeper samplers are typically limited to a vertical resolution of a centimeter or more (Harper et al., 1997), making it difficult to accurately measure steep gradients near the SWI. Furthermore, the surface sediments where CH4 gradients are steepest can be inadvertently lost when using some conventional coring techniques (Shirayama and Fukushima, 1995; Ostrovsky, 2000), especially in soft, unconsolidated sediments (Blomqvist, 1991). To resolve this issue, CH4 gradients at the SWI are sometimes calculated based on concentrations in the rest of the porewater profile using linear regression or exponential models (Schubert et al., 2011; Maeck et al., 2013; Zhang et al., 2020). Alternatively, a CH4 gradient measured between the overlying water and the uppermost sampled point in the sediment profile may be a suitable substitute for the SWI gradient (Klump et al., 2009).
2.3 Water Column Modeling
2.3.1 Approach Summary
The diffusion of CH4 from sediments is an important component of many models simulating CH4 dynamics in all or part of the lake water column. Many water column models rely on other methods described in this paper, such as incubations or sediment modeling (Sections 2.1, 2.2), to estimate the flux of CH4 across the SWI and use the measured value as model input. In this section, we focus on water column modeling approaches that estimate CH4 diffusive flux from sediments through mathematical expressions within the model structure, rather than previously described methods. These water column models can be classified into two groups: 1) mass balances used to calculate CH4 mass quantities in the water column; and 2) advection-diffusion-reaction models used to simulate CH4 concentrations in the water column (Figure 1).
Mass balances are the most common water column model used to quantify CH4 diffusion from sediments (Figure 1). Researchers applying a mass balance approach create a conceptual model of the sources, sinks, and transformations affecting the mass of CH4 present in the water column. Most often, this mass balance is focused on the lake hypolimnion during thermal stratification. The exchange of gases and solutes between the epilimnion and hypolimnion is significantly slowed throughout the stratification period, often resulting in CH4 accumulation below the thermocline (Eckert and Conrad, 2007). The hypolimnion during stratification can therefore be thought of as a largely closed system with sediments as the primary source of accumulating CH4. As an example, a simple conceptual model outlining the sources, sinks, and transformations of CH4 in the lake hypolimnion during thermal stratification is shown in Figure 4. Using this model, a mass balance can be used to link the observed rate of change in the mass of dissolved CH4 in the hypolimnion over time (
where
where
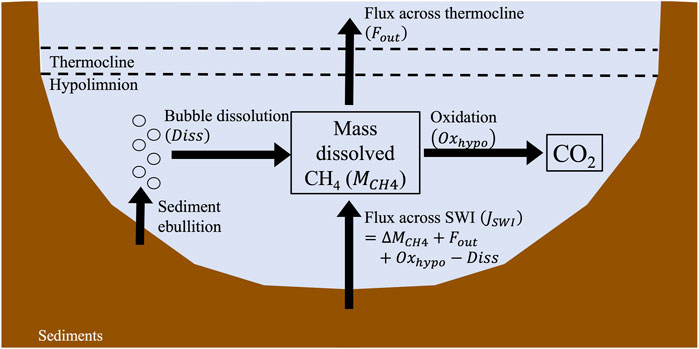
FIGURE 4. Example mass balance model of a lake hypolimnion. Note arrows are not drawn to scale. See text for details (Section 2.3).
The
where
A small subset of water column models that include a term for diffusive CH4 flux from sediments are advection-diffusion-reaction models. We will provide a brief overview here, however readers are referred to previous detailed descriptions for a full discussion of these models (Durisch-Kaiser et al., 2011; Tan et al., 2015; Stepanenko et al., 2016; Sabrekov et al., 2017; Schmid et al., 2017). These models consider how molecular diffusion affects CH4 transport in the water column, similar to how diffusion and diffusion-reaction models in the sediment consider the effects of molecular diffusion in the sediment profile (Section 2.2.1). However, unlike sediment models, advection is often included as an important term influencing CH4 transport in the water column. The diffusive flux of CH4 from sediments is usually included as a reaction term within the advection-diffusion-reaction model and can be solved for in multiple ways. In models that contain separate modules for the sediment and the water column, flux can be calculated using empirical relationships between methanogenesis and easily-measured sediment characteristics, such as temperature, labile carbon content, and/or pH (Stepanenko et al., 2011; Tan et al., 2015; Sabrekov et al., 2017). Methanogenesis rates can then be depth-integrated to estimate of flux out of sediments (Figure 2B). In models that only contain a water column module, flux can be parameterized by comparing observed and modeled CH4 concentration profiles in the water column (Durisch-Kaiser et al., 2011; Schmid et al., 2017).
2.3.2 Approach Advantages
Water column models are useful for determining the flux of CH4 across the SWI without relying on direct measurements of CH4 concentrations within the sediment porewater. Accordingly, these models avoid potential artifacts when sampling sediments for CH4, such as the escape of gases, loss of surface sediments, or oxygen exposure during sediment collection (Section 2.2.3). Water column models also provide flux estimates integrated over space and time (Figure 3), which can be advantageous when researchers are more concerned with observing system-wide CH4 dynamics than quantifying small-scale processes within the sediment or at the SWI (Table 1). For example, coupling CH4 mass balance information from the hypolimnion with monitoring of surface emissions can track whether CH4 released from sediments is ultimately stored, oxidized, or emitted (Matthews et al., 2005; Bastviken et al., 2008). Furthermore, some water column models can simultaneously determine the contribution of diffusion and ebullition to flux from lake sediments (Tan et al., 2015; Stepanenko et al., 2016), which can be advantageous in systems where bubbling is significant. For example, by modeling CH4 concentrations in the water column of Lake Kinneret, authors determined that ebullition was the primary pathway for surface CH4 emission and that methanotrophs oxidized the vast majority of dissolved CH4 in the water column (Schmid et al., 2017).
Another advantage of water column models is that they are highly customizable. On one hand, more terms could be added to the mass balance represented by Eq. 7 as needed, such as source terms for water column CH4 production (Donis et al., 2017; Günthel et al., 2020) and/or release from CH4 seeps (Schmid et al., 2007; Bornemann et al., 2016). However, more complicated models may require more sampling effort (Table 1). For example, advection-diffusion-reaction models of CH4 concentrations in the water column typically require more data than mass balances, such as meteorological information (Tan et al., 2015) and/or detailed temperature profiles (Sabrekov et al., 2017). On the other hand, modeling and sampling effort can sometimes be significantly simplified, for example in lakes where the last three terms in Eq. 7 can be considered negligible. In these cases, a mass balance can be performed with only lake bathymetry information and multiple hypolimnetic CH4 concentration profiles measured throughout lake stratification (Eq. 9).
2.3.3 Approach Limitations
When using water column models, it is important to consider the desired temporal resolution for model output. Water column models can be run on a range of time steps from minutes to months (Figure 3), depending on the level of data input and amount of computational time available. Many models also often assume spatial homogeneity of CH4 fluxes from sediments (Table 1). For example, mass balances similar to Eqs 7–9 typically provide no information on the flux of CH4 from sediments not in contact with the hypolimnion, such as shallow littoral regions that can be significant sources of CH4 in many lakes and reservoirs (Encinas Fernández et al., 2016). Nevertheless, Eqs 7–9 can be adjusted for use in other layers of the water column in order to estimate the diffusive CH4 flux from sediments lake-wide (Strayer and Tiedje, 1978) or specifically from sediments in contact with the epilimnion and/or metalimnion (Bastviken et al., 2008).
In contrast, advection-diffusion-reaction models applied in the water column focus on the basin-wide distribution of CH4, and therefore some do consider spatial heterogeneity in flux. For example, researchers applying an advection-diffusion-reaction model in the water column of Lake Kuivajärvi estimated CH4 release from sediments for five different depth zones (littoral to profundal) throughout a 6 month period (Stepanenko et al., 2016). Similarly, some advection-diffusion-reaction models account for how methanogenesis rates may vary spatially across sediment layers due to changing substrate quality and quantity (Tan et al., 2015). However, others assume CH4 diffusion from sediments is spatiotemporally constant throughout the entire lake (Durisch-Kaiser et al., 2011), which is not the case in many lakes and reservoirs (Bastviken et al., 2008; Berberich et al., 2019).
Water column models are also limited in their ability to determine drivers of diffusive CH4 flux from sediments (Table 1). Mass balances say little about the possible controls on flux unless they are combined with other data, such as organic carbon deposition rates, temperature profiles, or oxygen concentrations. Similarly, advection-diffusion-reaction models in the water column often rely on model parameterization or empirical equations to calculate flux across the SWI, neither of which directly link CH4 dynamics to causal drivers. In contrast, other methodologies (such as incubations performed under different experimental conditions) can directly test how CH4 flux changes with shifting environmental variables.
3 Methodological Comparisons
As described above, each method for measuring the diffusive flux of CH4 from lake sediments has particular advantages, limitations, and capacities to answer scientific questions about lacustrine CH4 cycling. When choosing which method is appropriate for one’s work, previous studies explicitly comparing results from multiple approaches are informative. Careful method intercomparisons are useful in that when results from different approaches are comparable, they can build confidence in our ability to estimate CH4 fluxes. Alternatively, when method intercomparisons reveal vastly divergent flux estimates across different techniques, they can highlight whether certain approaches are associated with systematic biases and indicate important areas for further investigation. Examples of such comparison studies are limited, comprising just 13% of our dataset (Figure 5; Section 4.4). In this section, we focus on this small subset of comparative studies to highlight a few important insights into the implications of method choice for the estimation of sediment CH4 fluxes.
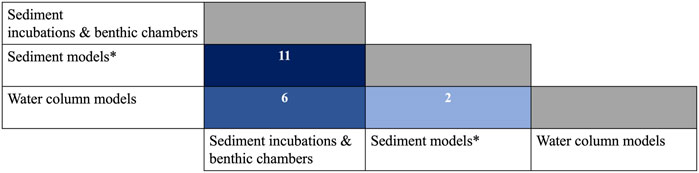
FIGURE 5. Matrix showing number of studies that have compared multiple methodologies for calculating the diffusive flux of CH4 from sediments. Blue boxes show number of existing studies, with lighter blue indicating fewer studies and darker blue indicating a larger number of studies. Asterisk indicates that there are n = 4 studies, not shown in the matrix here, that compared fluxes between two subtypes of sediment models (diffusion and diffusion-reaction models). All comparative studies are identified in the Supplementary data file (Supplementary Table S1).
3.1 Sediment Diffusion Models and Surface Sediment Processes
Previous comparisons of incubations and diffusion models applied in the sediment (Fick’s first law, Eq. 1) emphasize the challenge of accurately measuring processes near the SWI. Compared to sediment incubations, sediment diffusion models are reported to sometimes overestimate (Sweerts et al., 1991; Sinke et al., 1992; Li et al., 2018) and sometimes underestimate (Frenzel et al., 1990; Thebrath et al., 1993) the diffusive flux of CH4 from sediments. The discrepancy between incubation and diffusion model-based estimates may stem from several causes. For example, sediment concentration profiles cannot capture how rapid processes at the sediment surface may influence flux (Urban et al., 1997) and (Table 1). Sediments near the SWI are often an important location for sediment CH4 oxidation (Frenzel et al., 1990; Schubert et al., 2011; Hershey et al., 2014) or methanogenesis (Winfrey and Zeikus, 1979; Liikanen et al., 2002b; Dan et al., 2004), driving rapid changes in CH4 concentrations within just a few millimeters or centimeters of the SWI (Bussmann and Schink. 2006). These concentration changes may be unresolvable by the limited spatial resolution of porewater samples (Harper et al., 1997), resulting in measurement error for sediment diffusion model-based estimates (Table 1). In contrast, incubation using benthic chambers or intact cores measure CH4 accumulation above the SWI, and therefore they incorporate the potential effects of rapid surface processes on flux. Intact core incubations or benthic chambers may therefore be preferable to a sediment diffusion modeling approach in systems where significant CH4 processing in the sediment occurs within several millimeters of the SWI.
3.2 Incubation Effects on Microbial Rates
Comparisons between sediment incubations and other approaches suggest that incubations may overestimate fluxes by stimulating methanogenesis and/or inhibiting methanotrophy. For example, in Lake Constance, sediment fluxes based on slurry incubations were 3–4 times larger than estimates from sediment diffusion modeling (1,400 and 369 μmol CH4 m−2 d−1, respectively; Frenzel et al., 1990). In a eutrophic maar lake, incubation-based sediment fluxes (16 mmol CH4 m−2 d−1) were about tenfold higher than sediment diffusion model estimates (1.5 mmol CH4 m−2 d−1; Fahrner et al., 2008). Similarly, other studies report larger estimates of CH4 diffusive fluxes from incubations than hypolimnetic mass balances. In Frain’s Lake and Third Sister Lake, flux estimates based on incubations performed between 0 and 20 cm sediment depth were 2–5 times higher than flux estimates based on a hypolimnetic mass balance (Kelly and Chynoweth, 1980). Incubations measured a sediment flux of 2,600 µmol CH4 m−2 d−1 in the profundal zone of Blelham Tarn, 2–10 times higher than flux estimates from a hypolimnetic mass balance (235–1,244 μmol CH4 m−2 d−1; Jones and Simon 1981). The overestimation of methanogenesis by sediment incubation has also been noted in studies of other flooded soils (Blodau and Moore, 2003), where some incubation-based rates of CH4 production in peatland soils were found to be more than 10 times larger than estimates from sediment modeling. Sediment collection and handling prior to incubation may disturb sediment microstructure, possibly stimulating methanogenesis by providing better contact for methanogens to access substrate (Kelly and Chynoweth, 1980). Incubation preparation (e.g., sediment slurrying or headspace flushing) may also stimulate CH4 production by degassing or diluting compounds that would otherwise inhibit methanogens (Kelly and Chynoweth, 1980).
Larger sediment fluxes as a result of stimulated methanogenesis may be further compounded by the possible inhibition of CH4 oxidation by sediment slurrying, which may destroy aggregates important for methanotrophs and their syntrophic partners (Su et al., 2019). Methanotrophy is also positively correlated with CH4 availability (Thottathil et al., 2019), so degassing during sediment core sampling or incubation preparation could slow CH4 oxidation compared to in situ rates. The possible effects of incubation on methanogenesis and methanotrophy suggests that this approach may be more suitable for comparing relative rather than absolute fluxes.
3.3 Comparing CH4 Flux & CH4 Production
Comparing fluxes across methods also highlights the difference between measurements of CH4 flux and CH4 production. Confusingly, these terms are sometimes used interchangeably in the literature, despite the fact that the former technically refers to the rate of CH4 transport across a plane (e.g., the SWI) and the latter represents a rate of methanogenesis. The confusion in terminology is further compounded by the fact that CH4 production rates measured at discrete depths throughout the sediment profile can be depth-integrated into a flux out of sediments (Figures 2A,B). Previous studies have reported similar estimates of depth-integrated CH4 production from sediment diffusion-reaction modeling (Eqs 3–5) and flux across the SWI from sediment diffusion models (Eq. 1; Bartosiewicz et al., 2016; Steinsberger et al., 2019). However, other studies have noted a mismatch between estimates of CH4 diffusion based on sediment diffusion models and depth-integrated methanogenesis based on sediment diffusion-reaction models, attributing the difference to some produced CH4 being oxidized anaerobically (Norði et al., 2013) or forming bubbles (Adler et al., 2011) in the sediment profile before it could diffuse into the water column. Others have calculated sediment ebullition specifically as the difference between depth-integrated methanogenesis and the diffusive CH4 flux across the SWI (Langenegger et al., 2019).
Depth-integrated production also may not accurately represent flux if the sampling spatial resolution is too coarse to capture significant changes in methanogenesis within the sediment profile (Figure 2B). Methanogenesis can double or triple within just a few centimeters in the sediment column of some lakes (Chan et al., 2005; Yang et al., 2017), yet depth integration often assumes CH4 production is constant across sediment layers ranging in thickness from 3 to 5 cm (Bretz and Whalen, 2014; Berberich et al., 2019) to 20 cm or more (West et al., 2016). In these cases, depth-integrated production may be significantly different from flux. The possibility of misrepresenting flux by depth-integrating methanogenesis rates further underscores the advantage of approaches that measure the release of CH4 from sediments without sampling sediments directly, such as benthic chambers.
3.4 Sediment Depth Matters
Lastly, considering what sediment depths are characterized by a method is important when comparing techniques. The majority (60%) of incubation studies in our dataset base sediment CH4 flux or production measurements on samples taken from a sediment depth ≤20 cm. This is important because when using methods reliant on sediment sampling, such as sediment modeling or some incubations, fluxes may be underestimated if sampling excludes depths with significant methanogenesis. For example, Kelly and Chynoweth (1980) reported fluxes measured with core incubations sectioned from 0 to 3 cm in the sediment column were 2–4 times lower than fluxes measured with cores sectioned from 0 to 20 cm. Although the authors attribute the stark difference in fluxes to a possible artificial stimulation of methanogenesis in deeper layers of incubated sediment (Section 3.2), there are multiple examples in the literature of active and significant methanogenesis deep in the sediment profile. Production rates from 25 to 30 cm in the sediment profile of oligotrophic Lake Constance were observed to be almost as high as those from surface sediments (Rothfuss et al., 1997). Similarly, methanogenesis from 90 to 130 cm in the sediments of mesotrophic Lake Ätäskö were comparable to rates observed at 10–30 cm (Rissanen et al., 2017), and the highest methanogenesis rates were observed below 50 cm in cores from a thermokarst lake (Heslop et al., 2015) and an acidic lake (Koschorreck et al., 2008).
Taken together, these examples suggest that the common assumption of negligible methanogenesis in deep sediments is incorrect, at least some of the time. Highly organic-rich sediment columns (e.g., those within thermokarst lakes) may exhibit significant methanogenesis beyond surface sediments, whereas sandy, porous sediment columns may not have the carbon stores to support such microbial activity deeper in the profile. Alternatively, highly acidic or basic lakes may contain surface sediments with a pH that inhibits methanogenesis; yet methanogens may be active deeper in the sediment profile where conditions are more favorable. The control that sampled sediment depth exerts on flux estimates from some ex situ approaches highlights the advantage of methodologies that consider the entire sediment column in their measurements, such as in situ methods or techniques that estimate flux based on water column measurements.
4 Recommendations & Future Directions
The strengths and limitations of each method for measuring diffusive CH4 flux from sediments should influence one’s choice of approach for different research applications. Thus far, we have focused on limitations of individual methods (Section 2) or between pairs or small groups of methods (Section 3). Here, we take a broader perspective to 1) discuss how limitations across the full suite of approaches have shaped gaps in current understanding of lacustrine CH4 cycling; and 2) recommend future methodological developments needed to address these gaps.
4.1 Short-Term Temporal Variability
Little is known about short-term (sub-hourly) variation in diffusive CH4 fluxes from sediments, largely because most available approaches operate on hourly to monthly or seasonal time scales (Figure 3). A better characterization of short-term variability is necessary for identifying the implications of temporal resolution on measured fluxes. For example, previous studies have stressed the possibility of under- or over-estimating surface emissions from reservoirs and impounded rivers due to inadequate sampling frequency (Maeck et al., 2014; Wik et al., 2016; Harrison et al., 2017; Marcon et al., 2019). No similar studies exist for CH4 diffusion from lake sediments, despite recent evidence that diffusive flux can change significantly within hours (D’Ambrosio, 2022). Advancing understanding of short-term variability would therefore be instructive when determining the appropriate method and sampling frequency for future work.
Flux gradient approaches are promising, emerging tools for characterizing short-term variability in CH4 fluxes. Such methods can be applied to measure fluxes on a time scale of minutes to hours across the lake bottom boundary layer (BBL), a region of the water column extending up to several meters above the lakebed and a zone where currents are affected by friction with sediments (Henderson, 2016). Although flux measured in the BBL is not technically equivalent to flux across the SWI, estimates from the BBL are valuable because they quantify the rate at which CH4 is transported from sediments into the lake interior, from which it may ultimately be emitted to the atmosphere. Using a flux gradient technique, flux estimates are made in situ by combining measurements in the BBL of vertical concentration gradients, turbulent mixing, and temperature stratification (McGillis et al., 2011). Recently, the method has been adapted for use in a lake BBL for the first time (D’Ambrosio, 2022), whereas previous applications have focused on fluxes of momentum, oxygen, or total alkalinity in marine BBLs (Reidenbach et al., 2006; McGillis et al., 2011; Turk et al., 2015; Takeshita et al., 2016). This methodological advancement allowed for the estimation of CH4 fluxes on an hourly basis within the BBL of a eutrophic reservoir, revealing significant sub-daily variations in CH4 transport (D’Ambrosio, 2022).
The development of other techniques that operate on a short temporal scale would also be helpful, such as eddy correlation (eddy flux) techniques that have already been developed for fluxes of other dissolved gases such as CO2 and O2 across the SWI (Berg et al., 2013; Kokic et al., 2016). Unfortunately, eddy flux techniques require in situ concentration measurements with a temporal resolution <1 s (Donis et al., 2015), which are beyond the capability of dissolved CH4 instruments at present; response times for aqueous CH4 sensors are, at this writing, limited to 12 s–40 min response times (Damgaard and Revsbech, 1997; Webb et al., 2016; Xiao et al., 2020). The development of aqueous CH4 sensors with faster response times would facilitate the development of eddy correlation approaches for measuring CH4 flux from lakebeds. Alternatively, relaxed eddy accumulation techniques avoid the need for fast-response sensors and have been used to measure fluxes of oxygen, temp, and suspended matter across the SWI in a shallow riverine lake (Lemaire et al., 2017). However, both eddy flux and relaxed eddy accumulation approaches perform best in consistently turbulent conditions, and therefore may be of limited utility in lakes and reservoirs where near-bed turbulence is intermittent (Simpson et al., 2011; Henderson, 2016). Flux gradient approaches, adapted for use in moderately stratified lake BBLs (D’Ambrosio, 2022), may be the best alternative in these cases.
4.2 Boundary Layer Dynamics
Methodological limitations have also obscured the relationship between BBL conditions and SWI CH4 diffusion. In stratified lakes and reservoirs, internal waves and seiches can drive changes in BBL mixing and biogeochemistry that occur on timescales of minutes to hours (Wüest and Lorke, 2003; Bernhardt et al., 2014). It is well-established that such periodic changes in BBL turbulence exert significant control on the diffusive flux of other redox-active solutes to and from sediments, such as oxygen and nitrate (Lorke et al., 2003; Brand et al., 2008, 2009; Bryant et al., 2010). However, most available approaches for measuring CH4 diffusion from sediments cannot capture how such boundary layer processes influence flux. Current techniques largely rely on ex situ analyses (Table 1) performed on hourly to seasonal time scales (Figure 3). Consequently, boundary layer conditions have been largely overlooked as a potential factor influencing the diffusive flux of CH4 out of sediments.
Future work that couples BBL observations with CH4 flux measurements from sediments in space and time is needed to investigate how boundary layer dynamics may influence the rate and timing of CH4 transport into the water column. For example, flux gradient approaches do not isolate sediments from lake-scale processes in the boundary layer that may influence flux (D’Ambrosio, 2022), unlike ex situ techniques such as sediment incubations. Application of the flux gradient approach has illustrated how fluctuations in BBL turbulent mixing may exert an important control on CH4 transport from sediments into the water column (D’Ambrosio, 2022). The development of eddy flux and/or relaxed eddy accumulation techniques may also be helpful in this regard (Section 4.1). However, further measurements in other lake systems and a closer examination of potential causal links between boundary layer dynamics and CH4 transport are still needed. For example, on seasonal time scales, methanotrophs can regulate the upwards flux of CH4 in the water column by responding readily to changes in concentrations of CH4 and electron acceptors (Graf et al., 2018; Mayr et al., 2020a; Mayr et al., 2020b). On shorter time scales, it is unknown if methanotrophs exert a similar control on CH4 flux from sediments in response to fluctuating concentrations of CH4 and electron acceptors in the BBL.
4.3 Developing Modeling Approaches
Available methods for measuring diffusive CH4 flux across the SWI rely on direct sampling of the sediments or the water column. Our capacity to measure flux is therefore limited in lakes that are remote, seasonally inaccessible, or ice-covered. Moreover, a sampling bias towards measurements made over short-term deployments and during the summer months is likely, as noted in the literature of surface CH4 emissions from lakes (Wik et al., 2016; Jansen et al., 2020). Consequently, current estimates of the diffusive flux of CH4 from sediments are focused on physically accessible lakes and reservoirs, likely during the warmer months. Expanding the spatiotemporal coverage of measurements would provide novel insight into the variability and controls on CH4 release from lake sediments in a wide variety of lakes and reservoirs.
Developing models that estimate CH4 diffusion from sediments using easily-measured lake characteristics, rather than measurements of CH4 concentrations in the sediments and/or water column, could improve the spatiotemporal coverage of methane flux estimates. Multiple models of surface CH4 emissions from easily-measured lake characteristics have been created (Bastviken et al., 2004; Harrison et al., 2021). Similar techniques for predicting CH4 diffusion from lake sediments have not been developed, yet there is evidence that some basic lake characteristics could be useful in predicting sediment diffusive flux. For example, a recent study successfully modeled sediment CH4 production as a function of particulate organic matter supply and reactivity (Grasset et al., 2021). Moreover, a recent meta-analysis of over 60 lakes and reservoirs suggests sediment CH4 production rates can be linked to trophic status (D’Ambrosio and Harrison, 2021), which can be readily measured on site with surface water sampling. Exploring the power of lake characteristics to predict CH4 diffusion from sediments could elucidate how the supply of CH4 to the lake water column potentially changes across seasons, latitudes, lake types, and other system characteristics, uninhibited by direct sampling effort.
4.4 Expanding Method Comparisons
Of the 163 studies included in this review, just 13% (n = 22) used multiple methods to estimate CH4 diffusion from sediments (Figure 5; Supplementary Table S1). Approximately half of the comparative studies in our dataset compare the most popular approaches (sediment incubations and sediment diffusion models; Figure 1; Supplementary Table S1). To our knowledge, less common methods such as water column models have fewer direct comparisons (Figure 5). Comparisons between approaches in one or multiple lakes are common in studies of CH4 surface emission (Schubert et al., 2012; Podgrajsek et al., 2014; Deemer et al., 2016; Sanches et al., 2019), and oxidation in the water column or sediments (Bastviken et al., 2002; Su et al., 2019), but are uncommon in the study of CH4 diffusion from sediments. More method comparisons would benefit the field because observed agreement or deviation between approaches highlights methodological limitations and potential caveats when considering estimates between studies.
5 Conclusion
The diffusive flux of CH4 from sediments is a critical source of CH4 to lake and reservoir ecosystems, which collectively contribute significantly to global greenhouse gas emissions. Here, we critically assess the most popular methods for quantifying CH4 diffusion from sediments, including incubations, diffusion and diffusion-reaction models applied in the sediment, and mass balance and advection-diffusion-reaction models applied in the water column. We also discuss lessons learned from the small body of studies that directly compare some of these methods, including 1) estimating flux across the SWI with sediment diffusion models is challenging because these models are quite sensitive to surface sediment CH4 concentration gradients, which are difficult to measure at an appropriate spatial resolution; 2) incubation-based approaches can bias flux estimates by stimulating methanogenesis and/or inhibiting methanotrophy in sediments; 3) depth-integrating methanogenesis rates to estimate flux can lead to error if the sampling spatial resolution is not fine enough to resolve changes in CH4 production with depth; and 4) most studies estimate flux based on sampling shallow sediments (<20 cm depth), and therefore may not consider the possibility of significant CH4 production demonstrated deeper in the sediments of some systems. Furthermore, we explore how methodological limitations have shaped knowledge gaps regarding the rate and timing of CH4 supply to lake interiors. Specifically, the coarse spatiotemporal resolution of most established methods contributes to a poor understanding of the short-term variability of diffusive flux from sediments and the potential control of boundary layer conditions. Furthermore, the spatiotemporal coverage of diffusive flux estimates from different systems is likely biased towards physically accessible lakes sampled during the summer months.
We also identify several important avenues for future methodological advancement. In situ approaches with sub-hourly temporal resolution are needed to address questions of short-term variability and the role of boundary layer conditions. Recent applications of flux gradient techniques and possible future adaptations of eddy correlation or relaxed eddy accumulation approaches could be useful tools to this end. Expanding CH4 flux measurements using these techniques can help inform models that predict diffusive flux from sediments using basic lake characteristics, possibly improving the future spatiotemporal coverage of estimates. More quantitative comparisons between techniques at the finest and coarsest spatiotemporal resolution considered here (cm/min scales and whole lake/seasonal scales, respectively) would highlight additional strengths weaknesses of additional methods, point out additional knowledge gaps, and clarify the implications of methodological approach for flux estimation.
Author Contributions
SD performed the literature review and wrote the manuscript. JH provided scientific and editorial support.
Funding
This work was supported by funding from the NSF GRFP #1347973 and #1842493 to SD and funding from NSF DEB 1355211 and NSF DISES 2109259 to JH.
Conflict of Interest
The authors declare that the research was conducted in the absence of any commercial or financial relationships that could be construed as a potential conflict of interest.
Publisher’s Note
All claims expressed in this article are solely those of the authors and do not necessarily represent those of their affiliated organizations, or those of the publisher, the editors, and the reviewers. Any product that may be evaluated in this article, or claim that may be made by its manufacturer, is not guaranteed or endorsed by the publisher.
Acknowledgments
The authors thank Kevan Moffett, David Butman, Stephen Henderson, and multiple reviewers for their helpful feedback on this manuscript.
Supplementary Material
The Supplementary Material for this article can be found online at: https://www.frontiersin.org/articles/10.3389/fenvs.2022.850070/full#supplementary-material
References
Adams, D. D. (2005). “Diffuse Flux of Greenhouse Gases - Methane and Carbon Dioxide - at the Sediment-Water Interface of Some Lakes and Reservoirs of the World,” in Greenhouse Gas Emissions—Fluxes and Processes. Editors M. Garneau, A. Tremblay, C. Roehm, and L. Varfalvy (Springer Berlin Heidelberg), 129–153. doi:10.1007/978-3-540-26643-3_6
Addess, J. M., and Effler, S. W. (1996). Summer Methane Fluxes and Fall Oxygen Resources of Onondaga Lake. Lake Reservoir Manage. 12, 91–101. doi:10.1080/07438149609354000
Adler, M., Eckert, W., and Sivan, O. (2011). Quantifying Rates of Methanogenesis and Methanotrophy in Lake Kinneret Sediments (Israel) Using Pore-Water Profiles. Limnol. Oceanogr. 56, 1525–1535. doi:10.4319/lo.2011.56.4.1525
Algesten, G., Sobek, S., Bergström, A.-K., Jonsson, A., Tranvik, L. J., and Jansson, M. (2005). Contribution of Sediment Respiration to Summer CO2 Emission from Low Productive Boreal and Subarctic Lakes. Microb. Ecol. 50, 529–535. doi:10.1007/s00248-005-5007-x
Bartosiewicz, M., Laurion, I., Clayer, F., and Maranger, R. (2016). Heat-Wave Effects on Oxygen, Nutrients, and Phytoplankton Can Alter Global Warming Potential of Gases Emitted from a Small Shallow Lake. Environ. Sci. Technol. 50, 6267–6275. doi:10.1021/acs.est.5b06312
Bartosiewicz, M., Maranger, R., Przytulska, A., and Laurion, I. (2021). Effects of Phytoplankton Blooms on Fluxes and Emissions of Greenhouse Gases in a Eutrophic lake. Water Res. 196, 116985. doi:10.1016/j.watres.2021.116985
Bastviken, D., Cole, J. J., Pace, M. L., and Van de Bogert, M. C. (2008). Fates of Methane from Different lake Habitats: Connecting Whole-lake Budgets and CH4 emissions. J. Geophys. Res. 113, a–n. doi:10.1029/2007JG000608
Bastviken, D., Cole, J., Pace, M., and Tranvik, L. (2004). Methane Emissions from Lakes: Dependence of lake Characteristics, Two Regional Assessments, and a Global Estimate. Glob. Biogeochem. Cycles 18, a–n. doi:10.1029/2004GB002238
Bastviken, D., Ejlertsson, J., and Tranvik, L. (2002). Measurement of Methane Oxidation in Lakes: A Comparison of Methods. Environ. Sci. Technol. 36, 3354–3361. doi:10.1021/ES010311P
Bastviken, D. (2009). “Methane,” in Encyclopedia of Inland Waters (Academic Press), 783–805. doi:10.1016/b978-012370626-3.00117-4
Beaulieu, J., McManus, M., and Nietch, C. (2016). Estimates of Reservoir Methane Emissions Based on a Spatially-Balanced Probabilistic-Survey. Limnol. Oceanogr. 61, S27–S40. doi:10.1109/JPHOT.2015.XXXXXXX10.1002/lno.10284
Bédard, C., and Knowles, R. (1991). Hypolimnetic O2 Consumption, Denitrification, and Methanogenesis in a Thermally Stratified lake. Can. J. Fish. Aquat. Sci. 48, 1048–1054.
Berberich, M. E., Beaulieu, J. J., Hamilton, T. L., Waldo, S., and Buffam, I. (2019). Spatial Variability of Sediment Methane Production and Methanogen Communities within a Eutrophic Reservoir: Importance of Organic Matter Source and Quantity. Limnol. Oceanogr. 65, 1336–1358. doi:10.1002/lno.11392
Berg, P., Long, M. H., Huettel, M., Rheuban, J. E., McGlathery, K. J., Howarth, R. W., et al. (2013). Eddy Correlation Measurements of Oxygen Fluxes in Permeable Sediments Exposed to Varying Current Flow and Light. Limnol. Oceanogr. 58, 1329–1343. doi:10.4319/lo.2013.58.4.1329
Berg, P., Risgaard-Petersen, N., and Rysgaard, S. (1998). Interpretation of Measured Concentration Profiles in Sediment Pore Water. Limnol. Oceanogr. 43, 1500–1510. doi:10.4319/lo.1998.43.7.1500
Berner, R. (1980). Early Diagenesis: A Theoretical Approach. Princeton, NJ: Princeton University Press.
Bernhardt, J., Kirillin, G., and Hupfer, M. (2014). Periodic Convection within Littoral lake Sediments on the Background of Seiche-Driven Oxygen Fluctuations. Limnol. Oceanogr. 4, 17–33. doi:10.1215/21573689-2683238
Blodau, C., and Moore, T. R. (2003). Experimental Response of Peatland Carbon Dynamics to a Water Table Fluctuation. Aquat. Sci. - Res. Across Boundaries 65, 47–62. doi:10.1007/s000270300004
Blomqvist, S. (1991). Quantitative Sampling of Soft-Bottom Sediments: Problems and Solutions. Mar. Ecol. Prog. Ser. 72, 295–304. doi:10.3354/meps072295
Bornemann, M., Bussmann, I., Tichy, L., Deutzmann, J., Schink, B., and Pester, M. (2016). Methane Release from Sediment Seeps to the Atmosphere Is Counteracted by Highly Active Methylococcaceae in the Water Column of Deep Oligotrophic Lake Constance. FEMS Microbiol. Ecol. 92, fiw123–11. doi:10.1093/femsec/fiw123
Borrel, G., Jézéquel, D., Biderre-Petit, C., Morel-Desrosiers, N., Morel, J.-P., Peyret, P., et al. (2011). Production and Consumption of Methane in Freshwater lake Ecosystems. Res. Microbiol. 162, 832–847. doi:10.1016/j.resmic.2011.06.004
Bosse, U., Frenzel, P., and Conrad, R. (1993). Inhibition of Methane Oxidation by Ammonium in the Surface Layer of a Littoral Sediment. FEMS Microbiol. Ecol. 13, 123–134. doi:10.1016/0168-6496(93)90030-B10.1111/j.1574-6941.1993.tb00058.x
Boudreau, B. P. (1984). On the Equivalence of Nonlocal and Radial-Diffusion Models for Porewater Irrigation. J. Mar. Res. 42, 731–735. doi:10.1357/002224084788505924
Brand, A., Dinkel, C., and Wehrli, B. (2009). Influence of the Diffusive Boundary Layer on Solute Dynamics in the Sediments of a Seiche-Driven lake: A Model Study. J. Geophys. Res. 114, 1–12. doi:10.1029/2008JG000755
Brand, A., McGinnis, D. F., Wehrli, B., and Wüest, A. (2008). Intermittent Oxygen Flux from the interior into the Bottom Boundary of Lakes as Observed by Eddy Correlation. Limnol. Oceanogr. 53, 1997–2006. doi:10.4319/lo.2008.53.5.1997
Bretz, K., and Whalen, S. (2014). Methane Cycling Dynamics in Sediments of Alaskan Arctic Foothill Lakes. Iw 4, 65–78. doi:10.5268/IW-4.1.637
Bryant, L. D., Lorrai, C., McGinnis, D. F., Brand, A., Est, A. W., and Little, J. C. (2010). Variable Sediment Oxygen Uptake in Response to Dynamic Forcing. Limnol. Oceanogr. 55, 950–964. doi:10.4319/lo.2010.55.2.0950
Bufflap, S. E., and Allen, H. E. (1995). Sediment Pore Water Collection Methods for Trace Metal Analysis: A Review. Water Res. 29, 165–177. doi:10.1016/0043-1354(94)E0105-F
Bussmann, I. (2005). Methane Release through Resuspension of Littoral Sediment. Biogeochemistry 74, 283–302. doi:10.1007/s.10.1007/s10533-004-2223-2
Bussmann, I., and Schink, B. (2006). A Modified Diffusion-Based Methane Sensor and its Application in Freshwater Sediment. Limnol. Oceanogr. Methods 4, 275–283. doi:10.4319/lom.2006.4.275
Carignan, R., and Lean, D. R. S. (1991). Regeneration of Dissolved Substances in a Seasonally Anoxic lake: The Relative Importance of Processes Occurring in the Water Column and in the Sediments. Limnol. Oceanogr. 36, 683–707. doi:10.4319/lo.1991.36.4.0683
Chan, O. C., Claus, P., Casper, P., Ulrich, A., Lueders, T., and Conrad, R. (2005). Vertical Distribution of Structure and Function of the Methanogenic Archaeal Community in Lake Dagow Sediment. Environ. Microbiol. 7, 1139–1149. doi:10.1111/j.1462-2920.2005.00790.x
Chmiel, H. E., Kokic, J., Denfeld, B. A., Einarsdóttir, K., Wallin, M. B., Koehler, B., et al. (2016). The Role of Sediments in the Carbon Budget of a Small Boreal lake. Limnol. Oceanogr. 61, 1814–1825. doi:10.1002/lno.10336
Clayer, F., Gobeil, C., and Tessier, A. (2016). Rates and Pathways of Sedimentary Organic Matter Mineralization in Two Basins of a Boreal lake: Emphasis on Methanogenesis and Methanotrophy. Limnol. Oceanogr. 61, S131–S149. doi:10.1002/lno.10323
Clayer, F., Moritz, A., Gélinas, Y., Tessier, A., and Gobeil, C. (2018). Modeling the Carbon Isotope Signatures of Methane and Dissolved Inorganic Carbon to Unravel Mineralization Pathways in Boreal lake Sediments. Geochimica et Cosmochimica Acta 229, 36–52. doi:10.1016/j.gca.2018.02.012
Colas, F., Baudoin, J.-M., Bonin, P., Cabrol, L., Daufresne, M., Lassus, R., et al. (2021). Ecosystem Maturity Modulates Greenhouse Gases Fluxes from Artificial Lakes. Sci. Total Environ. 760, 144046. doi:10.1016/j.scitotenv.2020.144046
D'Ambrosio, S. L., and Harrison, J. A. (2021). Methanogenesis Exceeds CH4 Consumption in Eutrophic lake Sediments. Limnol Oceanogr Lett. 6, 173–181. doi:10.1002/lol2.10192
D’Ambrosio, S. L. (2022). Methane Flux from Lacustrine Sediments: Advancing Methodologies, Determining Drivers, and Characterizing Variability across Spatiotemporal Scales.
Damgaard, L. R., and Revsbech, N. P. (1997). A Microscale Biosensor for Methane Containing Methanotrophic Bacteria and an Internal Oxygen Reservoir. Anal. Chem. 69, 2262–2267. doi:10.1021/ac9611576
Dan, J., Kumai, T., Sugimoto, A., and Murase, J. (2004). Biotic and Abiotic Methane Releases from Lake Biwa Sediment Slurry. Limnology 5, 149–154. doi:10.1007/s10201-004-0124-7
Deemer, B. R., Harrison, J. A., Li, S., Beaulieu, J. J., DelSontro, T., Barros, N., et al. (2016). Greenhouse Gas Emissions from Reservoir Water Surfaces: A New Global Synthesis. Bioscience 66, 949–964. doi:10.1093/biosci/biw117
DelSontro, T., Kunz, M. J., Kempter, T., Wüest, A., Wehrli, B., and Senn, D. B. (2011). Spatial Heterogeneity of Methane Ebullition in a Large Tropical Reservoir. Environ. Sci. Technol. 45, 9866–9873. doi:10.1021/es2005545
DelSontro, T., Mcginnis, D. F., Sobek, S., Ostrovsky, I., and Wehrli, B. (2010). Extreme Methane Emissions from a Swiss Hydropower Reservoir: Contribution from Bubbling Sediments. Environ. Sci. Technol. 44, 2419–2425. doi:10.1021/es9031369
DelSontro, T., McGinnis, D. F., Wehrli, B., and Ostrovsky, I. (2015). Size Does Matter: Importance of Large Bubbles and Small-Scale Hot Spots for Methane Transport. Environ. Sci. Technol. 49, 1268–1276. doi:10.1021/es5054286
Devol, A. H. (1987). Verification of Flux Measurements Made with In Situ Benthic chambers. Deep Sea Res. A. Oceanographic Res. Pap. 34, 1007–1026. doi:10.1016/0198-0149(87)90050-1
Donis, D., Flury, S., Stöckli, A., Spangenberg, J. E., Vachon, D., and McGinnis, D. F. (2017). Full-scale Evaluation of Methane Production under Oxic Conditions in a Mesotrophic lake. Nat. Commun. 8, 1661. doi:10.1038/s41467-017-01648-4
Donis, D., Holtappels, M., Noss, C., Cathalot, C., Hancke, K., Polsenaere, P., et al. (2015). An Assessment of the Precision and Confidence of Aquatic Eddy Correlation Measurements. J. Atmos. Ocean. Technol. 32, 642–655. doi:10.1175/JTECH-D-14-00089.1
Duc, N. T., Crill, P., and Bastviken, D. (2010). Implications of Temperature and Sediment Characteristics on Methane Formation and Oxidation in lake Sediments. Biogeochemistry 100, 185–196. doi:10.1007/s10533-010-9415-8
Duchemin, E., Lucotte, M., Canuel, R., and Chamberland, A. (1995). Production of the Greenhouse Gases CH4 and CO2 by Hydroelectric Reservoirs of the Boreal Region. Glob. Biogeochem. Cycles 9, 529–540. doi:10.1029/95GB02202
Dück, Y., Liu, L., Lorke, A., Ostrovsky, I., Katsman, R., and Jokiel, C. (2019a). A Novel Freeze Corer for Characterization of Methane Bubbles and Assessment of Coring Disturbances. Limnol. Oceanogr. Methods 17, 305–319. doi:10.1002/lom3.10315
Dück, Y., Lorke, A., Jokiel, C., and Gierse, J. (2019b). Laboratory and Field Investigations on Freeze and Gravity Core Sampling and Assessment of Coring Disturbances with Implications on Gas Bubble Characterization. Limnol. Oceanogr. Methods 17, 585–606. doi:10.1002/lom3.10335
Durisch-Kaiser, E., Schmid, M., Peeters, F., Kipfer, R., Dinkel, C., Diem, T., et al. (2011). What Prevents Outgassing of Methane to the Atmosphere in Lake Tanganyika? J. Geophys. Res. 116, G02022. doi:10.1029/2010JG001323
Eckert, W., and Conrad, R. (2007). Sulfide and Methane Evolution in the Hypolimnion of a Subtropical lake: A Three-Year Study. Biogeochemistry 82, 67–76. doi:10.1007/s10533-006-9053-3
Encinas Fernández, J., Peeters, F., and Hofmann, H. (2016). On the Methane Paradox: Transport from Shallow Water Zones rather Than In Situ Methanogenesis Is the Major Source of CH4 in the Open Surface Water of Lakes. J. Geophys. Res. Biogeosci. 121, 2717–2726. doi:10.1002/2016JG003586
Epping, E. H. G., and Helder, W. (1997). Oxygen Budgets Calculated Fromin Situ Oxygen Microprofiles for Northern Adriatic Sediments. Continental Shelf Res. 17, 1737–1764. doi:10.1016/S0278-4343(97)00039-3
Fahrner, S., Radke, M., Karger, D., and Blodau, C. (2008). Organic Matter Mineralisation in the Hypolimnion of an Eutrophic Maar lake. Aquat. Sci. 70, 225–237. doi:10.1007/s00027-008-8008-2
Flury, S., Glud, R. N., Premke, K., and McGinnis, D. F. (2015). Effect of Sediment Gas Voids and Ebullition on Benthic Solute Exchange. Environ. Sci. Technol. 49, 10413–10420. doi:10.1021/acs.est.5b01967
Frenzel, P., Thebrath, B., and Conrad, R. (1990). Oxidation of Methane in the Oxic Surface Layer of a Deep lake Sediment (Lake Constance). FEMS Microbiol. Ecol. 73, 149–158. doi:10.1111/j.1574-6968.1990.tb03935.x
Frindte, K., Allgaier, M., Grossart, H.-P., and Eckert, W. (2015). Microbial Response to Experimentally Controlled Redox Transitions at the Sediment Water Interface. PLoS One 10, e0143428. doi:10.1371/journal.pone.0143428
Frindte, K., Eckert, W., Attermeyer, K., and Grossart, H.-P. (2013). Internal Wave-Induced Redox Shifts Affect Biogeochemistry and Microbial Activity in Sediments: A Simulation experiment. Biogeochemistry 113, 423–434. doi:10.1007/s10533-012-9769-1
Graf, J. S., Mayr, M. J., Marchant, H. K., Tienken, D., Hach, P. F., Brand, A., et al. (2018). Bloom of a Denitrifying Methanotroph, ' Candidatus Methylomirabilis Limnetica', in a Deep Stratified lake. Environ. Microbiol. 20, 2598–2614. doi:10.1111/1462-2920.14285
Grasset, C., Mendonça, R., Villamor Saucedo, G., Bastviken, D., Roland, F., and Sobek, S. (2018). Large but Variable Methane Production in Anoxic Freshwater Sediment upon Addition of Allochthonous and Autochthonous Organic Matter. Limnol. Oceanogr. 63, 1488–1501. doi:10.1002/lno.10786
Grasset, C., Moras, S., Isidorova, A., Couture, R. M., Linkhorst, A., and Sobek, S. (2021). An Empirical Model to Predict Methane Production in Inland Water Sediment from Particular Organic Matter Supply and Reactivity. Limnol. Oceanogr. 66, 3643–3655–13. doi:10.1002/lno.11905
Günthel, M., Klawonn, I., Woodhouse, J., Bižić, M., Ionescu, D., Ganzert, L., et al. (2020). Photosynthesis‐driven Methane Production in Oxic lake Water as an Important Contributor to Methane Emission. Limnol. Oceanogr. 65, 2853–2865–13. doi:10.1002/lno.11557
Harper, M. P., Davison, W., and Tych, W. (1997). Temporal, Spatial, and Resolution Constraints for In Situ Sampling Devices Using Diffusional Equilibration: Dialysis and DET. Environ. Sci. Technol. 31, 3110–3119. doi:10.1021/es9700515
Harrison, J. A., Deemer, B. R., Birchfield, M. K., and O’Malley, M. T. (2017). Reservoir Water-Level Drawdowns Accelerate and Amplify Methane Emission. Environ. Sci. Technol. 51, 1267–1277. doi:10.1017/CBO9781107415324.00410.1021/acs.est.6b03185
Harrison, J. A., Prairie, Y. T., Soued, C., and Mercier-Blais, S. (2021). Year-2020 Global Distribution and Pathways of Reservoir Methane and Carbon Dioxide Emissions According to the Greenhouse Gas from Reservoirs (G- Res) Model. Glob. Biogeochem. Cycles, 0–3. doi:10.1029/2020GB006888.This
Henderson, S. M. (2016). Turbulent Production in an Internal Wave Bottom Boundary Layer Maintained by a Vertically Propagating Seiche. J. Geophys. Res. Oceans 121, 2481–2498. doi:10.1002/2015JC011071.Received
Hershey, A. E., Northington, R. M., Hart-Smith, J., Bostick, M., and Whalen, S. C. (2015). Methane Efflux and Oxidation, and Use of Methane-Derived Carbon by Larval Chironomini, in Arctic lake Sediments. Limnol. Oceanogr. 60, 276–285. doi:10.1002/lno.10023
Hershey, A. E., Northington, R. M., and Whalen, S. C. (2014). Substrate Limitation of Sediment Methane Flux, Methane Oxidation and Use of Stable Isotopes for Assessing Methanogenesis Pathways in a Small Arctic lake. Biogeochemistry 117, 325–336. doi:10.1007/s10533-013-9864-y
Heslop, J. K., Walter Anthony, K. M., Sepulveda-Jauregui, A., Martinez-Cruz, K., Bondurant, A., Grosse, G., et al. (2015). Thermokarst lake Methanogenesis along a Complete Talik Profile. Biogeosciences 12, 4317–4331. doi:10.5194/bg-12-4317-2015
Hesslein, R. H. (1976). An In Situ Sampler for Close Interval Pore Water Studies1. Limnol. Oceanogr. 21, 912–914. doi:10.4319/lo.1976.21.6.0912
Hofmann, H., Federwisch, L., and Peeters, F. (2010). Wave-induced Release of Methane: Littoral Zones as Source of Methane in Lakes. Limnol. Oceanogr. 55, 1990–2000. doi:10.4319/lo.2010.55.5.1990
Huttunen, J. T., Väisänen, T. S., Hellsten, S. K., and Martikainen, P. J. (2006). Methane Fluxes at the Sediment–Water Interface in Some Boreal Lakes and Reservoirs. Boreal Environ. Res. 11, 27–34.
Isidorova, A., Grasset, C., Mendonça, R., and Sobek, S. (2019). Methane Formation in Tropical Reservoirs Predicted from Sediment Age and Nitrogen. Sci. Rep. 9, 1–9. doi:10.1038/s41598-019-47346-7
Itoh, M., Kobayashi, Y., Chen, T.-Y., Tokida, T., Fukui, M., Kojima, H., et al. (2015). Effect of Interannual Variation in winter Vertical Mixing on CH4 dynamics in a Subtropical Reservoir. J. Geophys. Res. Biogeosci. 120, 1246–1261. doi:10.1002/2015JG002972
Jahnke, R. A. (1988). A Simple, Reliable, and Inexpensive Pore-Water Sampler1. Limnol. Oceanogr. 33, 483–487. doi:10.4319/lo.1988.33.3.0483
Jansen, J., Thornton, B. F., Wik, M., MacIntyre, S., and Crill, P. M. (2020). Temperature Proxies as a Solution to Biased Sampling of Lake Methane Emissions. Geophys. Res. Lett. 47. doi:10.1029/2020GL088647
Jones, J. G., and Simon, B. M. (1981). Differences in Microbial Decomposition Processes in Profundal and Littoral Lake Sediments, with Particular Reference to the Nitrogen Cycle. Microbiology 123, 297–312. doi:10.1099/00221287-123-2-297
Jones, J. G., Simon, B. M., and Gardener, S. (1982). Factors Affecting Methanogenesis and Associated Anaerobic Processes in the Sediments of a Stratified Eutrophic Lake. Microbiology 128, 1–11. doi:10.1017/CBO9781107415324.00410.1099/00221287-128-1-1
Karvinen, A., Lehtinen, L., and Kankaala, P. (2015). Variable Effects of Iron (Fe (III)) Additions on Potential Methane Production in Boreal Lake Littoral Sediments. Wetlands 35, 137–146. doi:10.1007/s13157-014-0602-6
Kelly, C. A., and Chynoweth, D. P. (1980). Comparison of In Situ and In Vitro Rates of Methane Release in Freshwater Sediments. Appl. Environ. Microbiol. 40, 287–293. doi:10.1128/aem.40.2.287-293.1980
Kelly, C. A., and Chynoweth, D. P. (1981). The Contributions of Temperature and of the Input of Organic Matter in Controlling Rates of Sediment Methanogenesis1. Limnol. Oceanogr. 26, 891–897. doi:10.4319/lo.1981.26.5.0891
Kelly, C. A., Rudd, J. W. M., and Schindler, D. W. (1988). Carbon and Electron Flow via Methanogenesis, SO42- NO3-, Fe3+, and Mn4+ Reduction in the Anoxic Hypolimnia of three lakes. Arch. Hydrobiol Beih Ergebn Limnol 31, 333–344.
Klump, J. V., Fitzgerald, S. A., and Waplesa, J. T. (2009). Benthic Biogeochemical Cycling, Nutrient Stoichiometry, and Carbon and Nitrogen Mass Balances in a Eutrophic Freshwater bay. Limnol. Oceanogr. 54, 692–712. doi:10.4319/lo.2009.54.3.0692
Kokic, J., Sahlée, E., Brand, A., and Sobek, S. (2016). Low Sediment-Water Gas Exchange in a Small Boreal lake. J. Geophys. Res. Biogeosci. 121, 2493–2505. doi:10.1002/2016JG003372
Koschorreck, M., Wendt-Potthoff, K., Scharf, B., and Richnow, H. H. (2008). Methanogenesis in the Sediment of the Acidic Lake Caviahue in Argentina. J. Volcanology Geothermal Res. 178, 197–204. doi:10.1016/j.jvolgeores.2008.06.017
Kristensen, E., Penha-Lopes, G., Delefosse, M., Valdemarsen, T., Quintana, C., and Banta, G. (2012). What Is Bioturbation? the Need for a Precise Definition for Fauna in Aquatic Sciences. Mar. Ecol. Prog. Ser. 446, 285–302. doi:10.3354/meps09506
Kuivila, K. M., Murray, J. W., Devol, A. H., Lidstrom, M. E., and Reimers, C. E. (1988). Methane Cycling in the Sediments of Lake Washington. Limnol. Oceanogr. 33, 571–581. doi:10.4319/lo.1988.33.4.0571
Kuivila, K. M., Murray, J. W., Devol, A. H., and Novelli, P. C. (1989). Methane Production, Sulfate Reduction and Competition for Substrates in the Sediments of Lake Washington. Geochimica et Cosmochimica Acta 53, 409–416. doi:10.1016/0016-7037(89)90392-x
Langenegger, T., Vachon, D., Donis, D., and McGinnis, D. F. (2019). What the Bubble Knows: Lake Methane Dynamics Revealed by Sediment Gas Bubble Composition. Limnol. Oceanogr. 64, 1526–1544. doi:10.1002/lno.11133
Leal, J. J. F., Dos Santos Furtado, A. L., De Assis Esteves, F., Bozelli, R. L., and Figueiredo-Barros, M. P. (2007). The Role of Campsurus Notatus (Ephemeroptera: Polymitarcytidae) Bioturbation and Sediment Quality on Potential Gas Fluxes in a Tropical lake. Hydrobiologia 586, 143–154. doi:10.1007/s10750-006-0570-9
Lemaire, B. J., Noss, C., and Lorke, A. (2017). Toward Relaxed Eddy Accumulation Measurements of Sediment-Water Exchange in Aquatic Ecosystems. Geophys. Res. Lett. 44, 8901–8909. doi:10.1002/2017GL074625
Lettmann, K. A., Riedinger, N., Ramlau, R., Knab, N., Böttcher, M. E., Khalili, A., et al. (2012). Estimation of Biogeochemical Rates from Concentration Profiles: A Novel Inverse Method. Estuarine, Coastal Shelf Sci. 100, 26–37. doi:10.1016/j.ecss.2011.01.012
Li, B., Gu, Q., Miao, Y., Luo, W., Xing, P., and Wu, Q. L. (2020). Methane Distribution Patterns along a Transect of Lake Fuxian, a Deep Oligotrophic lake in China. Environ. Sci. Pollut. Res. 27, 25848–25860. doi:10.1007/s11356-019-06098-7
Li, L., Fuchs, A., Ortega, S. H., Xue, B., and Casper, P. (2021). Spatial Methane Pattern in a Deep Freshwater lake: Relation to Water Depth and Topography. Sci. Total Environ. 764, 142829. doi:10.1016/j.scitotenv.2020.142829
Li, L., Xue, B., Yao, S., Tao, Y., and Yan, R. (2018). Spatial-temporal Patterns of Methane Dynamics in Lake Taihu. Hydrobiologia 822, 143–156. doi:10.1007/s10750-018-3670-4
Liikanen, A., Flöjt, L., and Martikainen, P. (2002a). Gas Dynamics in Eutrophic Lake Sediments Affected by Oxygen, Nitrate, and Sulfate. J. Environ. Qual. 31, 338–349. doi:10.2134/jeq2002.3380
Liikanen, A., Huttunen, J. T., Murtoniemi, T., Tanskanen, H., Väisänen, T., Silvola, J., et al. (2003). Spatial and Seasonal Variation in Greenhouse Gas and Nutrient Dynamics and Their Interactions in the Sediments of a Boreal Eutrophic lake. Biogeochemistry 65, 83–103. doi:10.1023/A:1026070209387
Liikanen, A., Huttunen, J. T., Valli, K., and Martikainen, P. J. (2002b). Methane Cycling in the Sediment and Water Column of Mid-boreal Hyper-Eutrophic Lake Kevätön, Finland. fal 154, 585–603. doi:10.1127/archiv-hydrobiol/154/2002/585
Liikanen, A., and Martikainen, P. J. (2003). Effect of Ammonium and Oxygen on Methane and Nitrous Oxide Fluxes across Sediment-Water Interface in a Eutrophic lake. Chemosphere 52, 1287–1293. doi:10.1016/S0045-6535(03)00224-8
Liikanen, A., Murtoniemi, T., Tanskanen, H., Väisänen, T., and Martikainen, P. J. (2002c). Effects of Temperature and Oxygen Availability on Greenhouse Gas and Nutrient Dynamics in Sediment of a Eutrophic Mid-boreal lake. Biogeochemistry 59, 269–286. doi:10.1023/A:1016015526712
Liikanen, A., Tanskanen, H., Murtoniemi, T., and Martikainen, P. J. (2002d). A Laboratory Microcosm for Simultaneous Gas and Nutrient Flux Measurements in Sediments. Boreal Environ. Res. 7, 151–160.
Liu, L., Sotiri, K., Dück, Y., Hilgert, S., Ostrovsky, I., Uzhansky, E., et al. (2019). The Control of Sediment Gas Accumulation on Spatial Distribution of Ebullition in Lake Kinneret. Geo-mar Lett. 40, 453–466. doi:10.1007/s00367-019-00612-z
Lofton, D. D., Whalen, S. C., and Hershey, A. E. (2015). Vertical Sediment Distribution of Methanogenic Pathways in Two Shallow Arctic Alaskan Lakes. Polar Biol. 38, 815–827. doi:10.1007/s00300-014-1641-4
Lorke, A., Müller, B., Maerki, M., and Wüest, A. (2003). Breathing Sediments: The Control of Diffusive Transport across the Sediment-Water Interface by Periodic Boundary-Layer Turbulence. Limnol. Oceanogr. 48, 2077–2085. doi:10.4319/lo.2003.48.6.2077
Maeck, A., DelSontro, T., McGinnis, D. F., Fischer, H., Flury, S., Schmidt, M., et al. (2013). Sediment Trapping by Dams Creates Methane Emission Hot Spots. Environ. Sci. Technol. 47, 8130–8137. doi:10.1021/es4003907
Maeck, A., Hofmann, H., and Lorke, A. (2014). Pumping Methane Out of Aquatic Sediments - Ebullition Forcing Mechanisms in an Impounded River. Biogeosciences 11, 2925–2938. doi:10.5194/bg-11-2925-2014
Maerki, M., Müller, B., Dinkel, C., and Wehrli, B. (2009). Mineralization Pathways in lake Sediments with Different Oxygen and Organic Carbon Supply. Limnol. Oceanogr. 54, 428–438. doi:10.4319/lo.2009.54.2.0428
Magen, C., Lapham, L. L., Pohlman, J. W., Marshall, K., Bosman, S., Casso, M., et al. (2014). A Simple Headspace Equilibration Method for Measuring Dissolved Methane. Limnol. Oceanogr. 12, 637–650. doi:10.4319/lom.2014.12.637
Marcon, L., Bleninger, T., Männich, M., and Hilgert, S. (2019). High-frequency Measurements of Gas Ebullition in a Brazilian Subtropical Reservoir-Identification of Relevant Triggers and Seasonal Patterns. Environ. Monit. Assess. 191. doi:10.1007/s10661-019-7498-9
Martinez-Cruz, K., Leewis, M.-C., Herriott, I. C., Sepulveda-Jauregui, A., Anthony, K. W., Thalasso, F., et al. (2017). Anaerobic Oxidation of Methane by Aerobic Methanotrophs in Sub-arctic lake Sediments. Sci. Total Environ. 607-608, 23–31. 608. doi:10.1016/j.scitotenv.2017.06.187
Martinez-Cruz, K., Sepulveda-Jauregui, A., Casper, P., Anthony, K. W., Smemo, K. A., and Thalasso, F. (2018). Ubiquitous and Significant Anaerobic Oxidation of Methane in Freshwater lake Sediments. Water Res. 144, 332–340. doi:10.1016/j.watres.2018.07.053
Matthews, D. A., Effler, S. W., and Matthews, C. M. (2005). Long-term Trends in Methane Flux from the Sediments of Onondaga Lake, NY: Sediment Diagenesis and Impacts on Dissolved Oxygen Resources. archiv_hydrobiologie 163, 435–462. doi:10.1127/0003-9136/2005/0163-0435
Mayr, M. J., Zimmermann, M., Dey, J., Brand, A., Wehrli, B., and Bürgmann, H. (2020a). Growth and Rapid Succession of Methanotrophs Effectively Limit Methane Release during lake Overturn. Commun. Biol. 3. doi:10.1101/707836
Mayr, M. J., Zimmermann, M., Dey, J., Wehrli, B., and Bürgmann, H. (2020b). Lake Mixing Regime Selects Apparent Methane Oxidation Kinetics of the Methanotroph Assemblage. Biogeosciences 17, 4247–4259. doi:10.5194/bg-17-4247-2020
McAullife, C. (1971). GC Determination of Solutes by Multiple Phase Equilibration. Chem. Tech. 1, 46–51.
McGillis, W. R., Langdon, C., Loose, B., Yates, K. K., and Corredor, J. (2011). Productivity of a Coral Reef Using Boundary Layer and Enclosure Methods. Geophys. Res. Lett. 38, a–n. doi:10.1029/2010GL046179
Mudroch, A., and MacKnight, S. D. (1991). “Bottom Sediment Sampling,” in Handbook of Techniques for Aquatic Sediments Sampling, 29–95.
Müller, B., Wang, Y., Dittrich, M., and Wehrli, B. (2003). Influence of Organic Carbon Decomposition on Calcite Dissolution in Surficial Sediments of a Freshwater lake. Water Res. 37, 4524–4532. doi:10.1016/S0043-1354(03)00381-6
Müller, B., Bryant, L. D., Matzinger, A., and Wüest, A. (2012). Hypolimnetic Oxygen Depletion in Eutrophic Lakes. Environ. Sci. Technol. 46, 9964–9971. doi:10.1021/es301422r
Murase, J., and Sugimoto, A. (2002). Seasonal and Spatial Variations of Methane Production in Mesotrophic lake Sediments (Lake Biwa, Japan). SIL Proc. 1922-2010 28, 971–974. doi:10.1080/03680770.2001.11901861
Norði, K. à., Thamdrup, B., and Schubert, C. J. (2013). Anaerobic Oxidation of Methane in an Iron-Rich Danish Freshwater lake Sediment. Limnol. Oceanogr. 58, 546–554. doi:10.4319/lo.2013.58.2.0546
Nozhevnikova, A. N., Nekrasova, V., Ammann, A., Zehnder, A. J. B., Wehrli, B., and Holliger, C. (2007). Influence of Temperature and High Acetate Concentrations on Methanogenensis in lake Sediment Slurries. FEMS Microbiol. Ecol. 62, 336–344. doi:10.1111/j.1574-6941.2007.00389.x
Nusslein, B., Chin, K.-J., Eckert, W., and Conrad, R. (2001). Evidence for Anaerobic Syntrophic Acetate Oxidation during Methane Production in the Profundal Sediment of Subtropical Lake Kinneret (Israel). Environ. Microbiol. 3, 460–470. doi:10.1046/j.1462-2920.2001.00215.x
Nüsslein, B., and Conrad, R. (2000). Methane Production in Eutrophic Lake Plußsee: Seasonal Change, Temperature Effect and Metabolic Processes in the Profundal Sediment. fal 149, 597–623. doi:10.1127/archiv-hydrobiol/149/2000/597
Ostrovsky, I. (2000). The Upper-Most Layer of Bottom Sediments: Sampling and Artifacts. Fundam. Appl. Limnol. (Archiv Hydrobiol. 55, 243–255.
Pasche, N., Schmid, M., Vazquez, F., Schubert, C. J., Wüest, A., Kessler, J. D., et al. (2011). Methane Sources and Sinks in Lake Kivu. J. Geophys. Res. 116, G03006. doi:10.1029/2011JG001690
Paull, C. K., Lorenson, T. D., Dickens, G., Borowski, W. S., Ussler, W., and Kvenvolden, K. (2000). Comparisons of In Situ and Core Gas Measurements in ODP Leg 164 Bore Holes. Ann. N. Y. Acad. Sci. 912, 23–31. doi:10.1111/j.1749-6632.2000.tb06756.x
Peeters, F., Encinas Fernandez, J., and Hofmann, H. (2019). Sediment Fluxes rather Than Oxic Methanogenesis Explain Diffusive CH4 Emissions from Lakes and Reservoirs. Sci. Rep. 9. doi:10.1038/s41598-018-36530-w
Pimenov, N. V., Kallistova, A. Y., Rusanov, I. I., Yusupov, S. K., Montonen, L., Jurgens, G., et al. (2010). Methane Formation and Oxidation in the Meromictic Oligotrophic Lake Gek-Gel (Azerbaijan). Microbiology 79, 247–252. doi:10.1134/S0026261710020177
Podgrajsek, E., Sahlée, E., Bastviken, D., Holst, J., Lindroth, A., Tranvik, L., et al. (2014). Comparison of Floating Chamber and Eddy Covariance Measurements of lake Greenhouse Gas Fluxes. Biogeosciences 11, 4225–4233. doi:10.5194/bg-11-4225-2014
Powell, T., and Jassby, A. (1974). The Estimation of Vertical Eddy Diffusivities below the Thermocline in Lakes. Water Resour. Res. 10, 191–198. doi:10.1029/WR010i002p00191
Praetzel, L. S. E., Plenter, N., Schilling, S., Schmiedeskamp, M., Broll, G., and Knorr, K.-H. (2020). Organic Matter and Sediment Properties Determine in-lake Variability of Sediment CO2 and CH4 Production and Emissions of a Small and Shallow lake. Biogeosciences 17, 5057–5078. doi:10.5194/bg-17-5057-2020
Rahalkar, M., Deutzmann, J., Schink, B., and Bussmann, I. (2009). Abundance and Activity of Methanotrophic Bacteria in Littoral and Profundal Sediments of Lake Constance (Germany). Appl. Environ. Microbiol. 75, 119–126. doi:10.1128/AEM.01350-08
Reeburgh, W. S. (1967). An Improved Interstitial Water Sampler1. Limnol. Oceanogr. 12, 163–165. doi:10.4319/lo.1967.12.1.0163
Reidenbach, M. A., Monismith, S. G., Koseff, J. R., Yahel, G., and Genin, A. (2006). Boundary Layer Turbulence and Flow Structure over a Fringing Coral Reef. Limnol. Oceanogr. 51, 1956–1968. doi:10.4319/lo.2006.51.5.1956
Rissanen, A. J., Karvinen, A., Nykänen, H., Peura, S., Tiirola, M., Mäki, A., et al. (2017). Effects of Alternative Electron Acceptors on the Activity and Community Structure of Methane-Producing and Consuming Microbes in the Sediments of Two Shallow Boreal Lakes. FEMS Microbiol. Ecol. 93, 1–16. doi:10.1093/femsec/fix078
Rodriguez, M., Gonsiorczyk, T., and Casper, P. (2018). Methane Production Increases with Warming and Carbon Additions to Incubated Sediments from a Semiarid Reservoir. Inland Waters 8, 109–121. doi:10.1080/20442041.2018.1429986
Rolletschek, H. (1997). Temporal and Spatial Variations in Methane Cycling in Lake Müggelsee. fal 140, 195–206. doi:10.1127/archiv-hydrobiol/140/1997/195
Rosentreter, J. A., Borges, A. V., Deemer, B. R., Holgerson, M. A., Liu, S., Song, C., et al. (2021). Half of Global Methane Emissions Come from Highly Variable Aquatic Ecosystem Sources. Nat. Geosci. 14, 225–230. doi:10.1038/s41561-021-00715-2
Rothfuss, F., Bender, M., and Conrad, R. (1997). Survival and Activity of Bacteria in a Deep, Aged lake Sediment (Lake Constance). Microb. Ecol. 33, 69–77. doi:10.1007/s002489900009
Rudd, J. W. M., Hamilton, R. D., and Campbell, N. E. R. (1974). Measurement of Microbial Oxidation of Methane in lake Water. Limnol. Oceanogr. 19, 519–524. doi:10.4319/lo.1974.19.3.0519
Rudd, J. W. M., and Hamilton, R. D. (1978). Methane Cycling in a Eutrophic Shield lake and its Effects on Whole lake Metabolism 1. Limnol. Oceanogr. 23, 337–348. doi:10.4319/lo.1978.23.2.0337
Sabrekov, A. F., Runkle, B. R. K., Glagolev, M. V., Terentieva, I. E., Stepanenko, V. M., Kotsyurbenko, O. R., et al. (2017). Variability in Methane Emissions from West Siberia's Shallow Boreal Lakes on a Regional Scale and its Environmental Controls. Biogeosciences 14, 3715–3742. doi:10.5194/bg-14-3715-2017
Sanches, L. F., Guenet, B., Marinho, C. C., Barros, N., and de Assis Esteves, F. (2019). Global Regulation of Methane Emission from Natural Lakes. Sci. Rep. 9, 1–10. doi:10.1038/s41598-018-36519-5
Schmid, M., Batist, M. D., Granin, N. G., Kapitanov, V. A., McGinnis, D. F., Mizandrontsev, I. B., et al. (2007). Sources and Sinks of Methane in Lake Baikal: A Synthesis of Measurements and Modeling. Limnol. Oceanogr. 52, 1824–1837. doi:10.4319/lo.2007.52.5.1824
Schmid, M., Ostrovsky, I., and McGinnis, D. F. (2017). Role of Gas Ebullition in the Methane Budget of a Deep Subtropical lake: What Can We Learn from Process-Based Modeling? Limnol. Oceanogr. 62, 2674–2698. doi:10.1002/lno.10598
Schubert, C. J., Diem, T., and Eugster, W. (2012). Methane Emissions from a Small Wind Shielded Lake Determined by Eddy Covariance, Flux Chambers, Anchored Funnels, and Boundary Model Calculations: A Comparison. Environ. Sci. Technol. 46, 4515–4522. doi:10.1021/es203465x
Schubert, C. J., Vazquez, F., Lösekann-Behrens, T., Knittel, K., Tonolla, M., and Boetius, A. (2011). Evidence for anaerobic oxidation of methane in sediments of a freshwater system (Lago di Cadagno). FEMS Microbiol. Ecol. 76, 26–38. doi:10.1111/j.1574-6941.2010.01036.x
Seeberg-Elverfeldt, J., Schlüter, M., Feseker, T., and Kölling, M. (2005). Rhizon Sampling of Porewaters Near the Sediment-Water Interface of Aquatic Systems. Limnol. Oceanogr. Methods 3, 361–371. doi:10.4319/lom.2005.3.361
Shirayama, Y., and Fukushima, T. (1995). Comparisons of Deep-Sea Sediments and Overlying Water Collected Using Multiple Corer and Box Corer. J. Oceanogr. 51, 75–82. doi:10.1007/bf02235937
Simpson, J. H., Wiles, P. J., and Lincoln, B. J. (2011). Internal Seiche Modes and Bottom Boundary-Layer Dissipation in a Temperate lake from Acoustic Measurements. Limnol. Oceanogr. 56, 1893–1906. doi:10.4319/lo.2011.56.5.1893
Sinke, A. J. C., Cornelese, A. A., Cappenberg, T. E., and Zehnder, A. J. B. (1992). Seasonal Variation in Sulfate Reduction and Methanogenesis in Peaty Sediments of Eutrophic Lake Loosdrecht, The Netherlands. Biogeochemistry 16, 43–61. doi:10.1007/bf02402262
Stadmark, J., and Leonardson, L. (2007). Greenhouse Gas Production in a Pond Sediment: Effects of Temperature, Nitrate, Acetate and Season. Sci. Total Environ. 387, 194–205. doi:10.1016/j.scitotenv.2007.07.039
Steinsberger, T., Müller, B., Gerber, C., Shafei, B., and Schmid, M. (2019). Modeling Sediment Oxygen Demand in a Highly Productive lake under Various Trophic Scenarios. PLoS One 14, e0222318–23. doi:10.1371/journal.pone.0222318
Steinsberger, T., Schmid, M., Wüest, A., Schwefel, R., Wehrli, B., and Müller, B. (2017). Organic Carbon Mass Accumulation Rate Regulates the Flux of Reduced Substances from the Sediments of Deep Lakes. Biogeosciences 14, 3275–3285. doi:10.5194/bg-14-3275-2017
Stepanenko, V., Mammarella, I., Ojala, A., Miettinen, H., Lykosov, V., and Vesala, T. (2016). LAKE 2.0: A Model for Temperature, Methane, Carbon Dioxide and Oxygen Dynamics in Lakes. Geosci. Model. Dev. 9, 1977–2006. doi:10.5194/gmd-9-1977-2016
Stepanenko, V. M., Machul’skaya, E. E., Glagolev, M. V., and Lykossov, V. N. (2011). Numerical Modeling of Methane Emissions from Lakes in the Permafrost Zone. Izv. Atmos. Ocean. Phys. 47, 252–264. doi:10.1134/S0001433811020113
St. Louis, V. L. V. L., Kelly, C. a., Duchemin, É., Rudd, J. W. M., and Rosenberg, D. M. (2000). Reservoir Surfaces as Sources of Greenhouse Gases to the Atmosphere: A Global Estimate. Bioscience 50, 766. doi:10.1641/0006-3568(2000)050[0766:rsasog]2.0.co;2
Strayer, R. F., and Tiedje, J. M. (1978). In Situ methane Production in a Small, Hypereutrophic, Hard-Water lake: Loss of Methane from Sediments by Vertical Diffusion and Ebullition 1. Limnol. Oceanogr. 23, 1201–1206. doi:10.4319/lo.1978.23.6.1201
Sturm, K., Yuan, Z., Gibbes, B., Werner, U., and Grinham, A. (2014). Methane and Nitrous Oxide Sources and Emissions in a Subtropical Freshwater Reservoir, South East Queensland, Australia. Biogeosciences 11, 5245–5258. doi:10.5194/bg-11-5245-2014
Su, G., Niemann, H., Steinle, L., Zopfi, J., and Lehmann, M. F. (2019). Evaluating Radioisotope‐based Approaches to Measure Anaerobic Methane Oxidation Rates in Lacustrine Sediments. Limnol. Oceanogr. Methods 17, 429–438. doi:10.1002/lom3.10323
Sweerts, J.-P. R. A., Bär-Gilissen, M.-J., Cornelese, A. A., and Cappenberg, T. E. (1991). Oxygen-consuming Processes at the Profundal and Littoral Sediment-Water Interface of a Small Meso-Eutrophic lake (Lake Vechten, The Netherlands). Limnol. Oceanogr. 36, 1124–1133. doi:10.4319/lo.1991.36.6.1124
Takeshita, Y., McGillis, W., Briggs, E. M., Carter, A. L., Donham, E. M., Martz, T. R., et al. (2016). Assessment of Net Community Production and Calcification of a Coral Reef Using a Boundary Layer Approach. J. Geophys. Res. Oceans 121, 5655–5671. doi:10.1002/2016JC011886
Tan, Z., Zhuang, Q., and Walter Anthony, K. (2015). Modeling Methane Emissions from Arctic Lakes: Model Development and Site‐level Study. J. Adv. Model. Earth Syst. 7, 459–483. doi:10.1002/2014MS000344
Thebrath, B., Rothfuss, F., Whiticar, M. J., and Conrad, R. (1993). Methane Production in Littoral Sediment of Lake Constance. FEMS Microbiol. 102, 279–289. doi:10.1111/j.1574-6968.1993.tb05819.x
Thottathil, S. D., Reis, P. C. J., and Prairie, Y. T. (2019). Methane Oxidation Kinetics in Northern Freshwater Lakes. Biogeochemistry 143, 105–116. doi:10.1007/s10533-019-00552-x
Torres, I. C., Inglett, K. S., and Reddy, K. R. (2011). Heterotrophic Microbial Activity in lake Sediments: Effects of Organic Electron Donors. Biogeochemistry 104, 165–181. doi:10.1007/s10533-010-9494-6
Turk, D., Yates, K., Vega-Rodriguez, M., Toro-Farmer, G., L’Esperance, C., Melo, N., et al. (2015). Community Metabolism in Shallow Coral Reef and Seagrass Ecosystems, Lower Florida Keys. Mar. Ecol. Prog. Ser. 538, 35–52. doi:10.3354/meps11385
Tyroller, L., Tomonaga, Y., Brennwald, M. S., Ndayisaba, C., Naeher, S., Schubert, C., et al. (2016). Improved Method for the Quantification of Methane Concentrations in Unconsolidated Lake Sediments. Environ. Sci. Technol. 50, 7047–7055. doi:10.1021/acs.est.5b05292
Urban, N. R., Dinkel, C., and Wehrli, B. (1997). Solute Transfer across the Sediment Surface of a Eutrophic lake: I. Porewater Profiles from Dialysis Samplers. Aquat. Sci. 59, 1–25. doi:10.1007/pl0000130210.1007/bf02522546
Vachon, D., Langenegger, T., Donis, D., and McGinnis, D. F. (2019). Influence of Water Column Stratification and Mixing Patterns on the Fate of Methane Produced in Deep Sediments of a Small Eutrophic lake. Limnol. Oceanogr. 64, 2114–2128. doi:10.1002/lno.11172
Valle, J., Gonsior, M., Harir, M., Enrich-Prast, A., Schmitt-Kopplin, P., Bastviken, D., et al. (2018). Extensive Processing of Sediment Pore Water Dissolved Organic Matter during Anoxic Incubation as Observed by High-Field Mass Spectrometry (FTICR-MS). Water Res. 129, 252–263. doi:10.1016/j.watres.2017.11.015
van Grinsven, S., Sinninghe Damsté, J. S., Harrison, J., and Villanueva, L. (2020). Impact of Electron Acceptor Availability on Methane-Influenced Microorganisms in an Enrichment Culture Obtained from a Stratified Lake. Front. Microbiol. 11. doi:10.3389/fmicb.2020.00715
Viollier, E., Rabouille, C., Apitz, S. E., Breuer, E., Chaillou, G., Dedieu, K., et al. (2003). Benthic Biogeochemistry: State of the Art Technologies and Guidelines for the Future of In Situ Survey. J. Exp. Mar. Biol. Ecol. 285-286, 5–31. –286. doi:10.1016/S0022-0981(02)00517-8
Wagener, S., Schulz, S., and Hanselmann, K. (1990). Abundance and Distribution of Anaerobic Protozoa and Their Contribution to Methane Production in Lake Cadagno (Switzerland). FEMS Microbiol. Lett. 74, 39–48. doi:10.1111/j.1574-6968.1990.tb04050.x
Webb, J. R., Maher, D. T., and Santos, I. R. (2016). Automated, In Situ Measurements of Dissolved CO2, CH4, and δ13 C Values Using Cavity Enhanced Laser Absorption Spectrometry: Comparing Response Times of Air-Water Equilibrators. Limnol. Oceanogr. Methods 14, 323–337. doi:10.1002/lom3.10092
West, W. E., Coloso, J. J., and Jones, S. E. (2012). Effects of Algal and Terrestrial Carbon on Methane Production Rates and Methanogen Community Structure in a Temperate lake Sediment. Freshw. Biol. 57, 949–955. doi:10.1111/j.1365-2427.2012.02755.x
West, W. E., Creamer, K. P., and Jones, S. E. (2016). Productivity and Depth Regulate lake Contributions to Atmospheric Methane. Limnol. Oceanogr. 61, S51–S61. doi:10.1002/lno.10247
Wik, M., Thornton, B. F., Bastviken, D., Uhlbäck, J., and Crill, P. M. (2016). Biased Sampling of Methane Release from Northern Lakes: A Problem for Extrapolation. Geophys. Res. Lett. 43, 1256–1262. doi:10.1002/2015GL066501
Winfrey, M. R., and Zeikus, J. G. (1979). Microbial Methanogenesis and Acetate Metabolism in a Meromictic lake. Appl. Environ. Microbiol. 37, 213–221. doi:10.1128/aem.37.2.213-221.1979
Wüest, A., and Lorke, A. (2003). Small-scale Hydrodynamics in Lakes. Annu. Rev. Fluid Mech. 35, 373–412. doi:10.1146/annurev.fluid.35.101101.161220
Xiao, S., Liu, L., Wang, W., Lorke, A., Woodhouse, J., and Grossart, H.-P. (2020). A Fast-Response Automated Gas Equilibrator (FaRAGE) for Continuous In Situ Measurement of Methane Dissolved in Water. Hydrol. Earth Syst. Sci. Discuss., 1–28. doi:10.5194/hess-2020-67
Yang, Y., Chen, J., Li, B., Liu, Y., and Xie, S. (2018). Anaerobic Methane Oxidation Potential and Bacteria in Freshwater Lakes: Seasonal Changes and the Influence of Trophic Status. Syst. Appl. Microbiol. 41, 650–657. doi:10.1016/j.syapm.2018.08.002
Yang, Y., Li, N., Wang, W., Li, B., Xie, S., and Liu, Y. (2017). Vertical Profiles of Sediment Methanogenic Potential and Communities in Two Plateau Freshwater Lakes. Biogeosciences 14, 341–351. doi:10.5194/bg-14-341-2017
Yavitt, J. B., Angell, L. L., Fahey, T. J., Cirmo, C. P., and Driscoll, C. T. (1992). Methane Fluxes, Concentrations, and Production in Two Adirondack beaver Impoundments. Limnol. Oceanogr. 37, 1057–1066. doi:10.4319/lo.1992.37.5.1057
Zeikus, J. G., and Winfrey, M. R. (1976). Temperature Limitation of Methanogenesis in Aquatic Sediments. Appl. Environ. Microbiol. 31, 99–107. doi:10.1128/aem.31.1.99-107.1976
Keywords: methane, sediment methane flux, sediment methanogenesis, CH4 flux, flux measurement
Citation: D’Ambrosio SL and Harrison JA (2022) Measuring CH4 Fluxes From Lake and Reservoir Sediments: Methodologies and Needs. Front. Environ. Sci. 10:850070. doi: 10.3389/fenvs.2022.850070
Received: 07 January 2022; Accepted: 09 March 2022;
Published: 29 March 2022.
Edited by:
Daniel F. McGinnis, Université de Genève, SwitzerlandCopyright © 2022 D’Ambrosio and Harrison. This is an open-access article distributed under the terms of the Creative Commons Attribution License (CC BY). The use, distribution or reproduction in other forums is permitted, provided the original author(s) and the copyright owner(s) are credited and that the original publication in this journal is cited, in accordance with accepted academic practice. No use, distribution or reproduction is permitted which does not comply with these terms.
*Correspondence: Sofia L. D’Ambrosio, c29maWEuZGFtYnJvc2lvQHdzdS5lZHU=