- 1Department of Soil Science, Bangladesh Agricultural University, Mymensingh, Bangladesh
- 2Centre for Sustainable Farming Systems, Future Food Institute, Murdoch University, Murdoch, WA, Australia
- 3Regional Agricultural Research Station, Bangladesh Agricultural Research Institute, Moulvibazar, Bangladesh
- 4Bangladesh Agriculture Research Council, Dhaka, Bangladesh
- 5Soil and Water Management and Crop Nutrition, Joint FAO/IAEA Division of Nuclear Techniques in Food and Agriculture, Vienna, Austria
- 6State Key Laboratory of Soil and Sustainable Agriculture, Institute of Soil Science, Chinese Academy of Sciences, Nanjing, China
- 7Institute of Plant Ecology (IFZ), Justus-Liebig University Giessen, Giessen, Germany
- 8School of Biology and Environmental Science and Earth Institute, University College Dublin, Dublin, Ireland
Wetland rice cultivation contributes significantly to global warming potential (GWP), an effect which is largely attributed to emissions of methane (CH4). Emerging technologies for wetland rice production such as conservation agriculture (CA) may mitigate greenhouse gas (GHG) emissions, but the effects are not well defined. Investigations were carried out in an irrigated rice (Boro rice) field in the fifth crop after conversion of conventional tillage (CT) to strip tillage (ST). Two crop residue levels (low versus high, LR versus HR) and three nitrogen (N) application rates (N1 = 108, N2 = 144, and N3 = 180 kg N ha−1) were laid out in a split-plot experiment with three replicates. Yield-scaled GHG emissions and GWP were estimated to evaluate the impacts of CA on mitigating CH4 and N2O emissions in the rice paddy field. There was a 55% higher N2O emission in ST with HR coupled with N3 than that in CT with LR coupled with N1. The N2O emission factors ranged from 0.43 to 0.75% in ST and 0.45 to 0.59% in CT, irrespective of the residue level and N rate. By contrast, CH4 emissions were significantly lower in CA than in the conventional practices (CT plus LR). The ST with LR in N2 reduced the GWP by 39% over the GWP in CT with HR in N1 and 16% over the conventional practices. Based on our investigation of the combination of tillage, residue, and N rate treatments, the adoption of CA with high and low residue levels reduced the GWP by 10 and 16%, respectively, because of lower CH4 and N2O emissions than the current management practices. The relatively high N2O emission factors suggest that mitigation of this GHG in wetland rice systems needs greater attention.
1 Introduction
Rice (Oryza sativa) is the staple food for more than half of the world’s population (GRSP (Global Road Safety Partnership), 2013), but its production requires at least one-seventh of the fertilizers worldwide (Heffer, 2009). In Asia, where 90% of rice is consumed, enough affordable rice is key to food security. However, rice cultivation is estimated to account for 2.5% of the present global anthropogenic warming predominantly due to emissions of CH4 (Kritee et al., 2018). In addition to being a source of greenhouse gas (GHG) emissions, rice production acts as a sink for soil carbon. However, estimates of the contribution to wetland rice to GHG emissions ignore N2O emissions because under flooding, it is assumed that N2O produced is reduced to climatically benign di-nitrogen (N2). However, a recent global policy guidance document [EPA (Environmental Protection Agency), 2013] suggests that N2O emissions from rice can contribute 25% to the GHG impact of rice cultivation on a CO2 equivalent basis (CO2e, over100 years) (Myhre et al., 2013). Yet none of the major rice-producing countries, including the two leading rice producers, China [NDRC (National Development and Reform Commission), 2012] and India (MOEFCC (Ministry of Environment, Forest and Climate Change), 2012), reported N2O emission factors in their national GHG inventories submitted to the UN (Smith, 2007). While recent scientific studies make it clear that both CH4 and N2O emissions need to be addressed (Li et al., 2011; Carlson et al., 2017; Kritee et al., 2018), there are presently very few validated estimates of the appropriate emission factors for wetland rice production.
The rates of anthropogenic CH4 emissions are increasingly studied, measured, and reported, but large uncertainties persist for wetland rice production due to the lack of regional data (Kirschke et al., 2013; Weber et al., 2019). Moreover, GHG emissions from rice cultivation vary with soil types and locations (Sun et al., 2013), growing seasons (Alam et al., 2016, 2019), and fertilizer- management practices (Gaihre et al., 2011). In Bangladesh, rice is grown in two to three seasons per year on about 11.5 million ha of land, which covers almost 80% of the agricultural land. Until now, the national GHG inventory of Bangladesh was based on the tier 1 approach proposed by the IPCC (2006) because of the lack of the local experimental data. However, understanding and quantifying the regional CH4 budget is important for assessing realistic pathways within the agricultural sector to mitigate climate change. A realistic mitigation target could not be set without having country-specific emission factors. Region-specific short- and long-term studies of rice farming practices that measure management impacts on both N2O and CH4 emissions are necessary to determine and minimize the climate impacts of rice cultivation (Kritee et al., 2018).
Rice is predominantly cultivated in flooded conditions which produce anaerobic soils, that is, suitable conditions for the anaerobic degradation of organic substances by methanogens and nitrate reduction by denitrifiers. In contrast to conventional tillage (CT) and soil puddling, both promoting the degradation of soil organic matter (SOM) and emissions of GHGs, conservation agriculture (CA) with reduced soil disturbance (e.g., strip tillage, ST) and crop residue retention within diverse crop rotations affects C–N cycling processes and can increase crop yields (Islam et al., 2014; Rashid et al., 2018; Bell et al., 2019). Reduced tillage has been reported to increase sequestration of the SOM and retention of N (Zhao et al., 2015; Alam et al., 2018; Alam and Bell, 2020), enhance soil aggregate formation and stability (Jahangir et al., 2011; Jahangir et al., 2021a), conserve soil moisture (Moraru and Rusu, 2012), and change the fungi to bacteria ratio in soil (Jahangir et al., 2011; Sun et al., 2018). However, the effect of CA practices on emissions of specific GHGs in rice paddies is still unclear because of sparse experimental results (Feng et al., 2018) and an absence of studies that considered N fertilizer or other crop management factors (Alam et al., 2016, 2019). In recent decades, rice yields have been increasing mainly because of a larger nitrogen (N) supply (Tilman et al., 2002; Erisman et al., 2008), but the effect on N2O emissions has been largely neglected (Linquist et al., 2015). Here, we examined the interplay among soil disturbance, crop residue levels, and fertilizer N management on CH4 and N2O emissions to identify suitable combinations for mitigation. We hypothesize that N fertilizer rates, increased crop residue levels, and decreased disturbance of rice paddy soils will alter the C and N biogeochemistry, resulting in changes in the CH4 and N2O production and exchange. The objectives of the present study were to 1) quantify CH4 and N2O emissions in an irrigated, wetland rice field under varying management options, and 2) evaluate different N application rates in combination with various levels of soil disturbance and residue supply for mitigating CH4 and N2O emissions in paddy rice fields.
2 Materials and Methods
2.1 Experimental Site and Soil Properties
The study was conducted on the Research Field of the Department of Soil Science at Bangladesh Agricultural University (BAU) farm, Mymensingh (24°71.60′N, 90°42.51′E), Bangladesh, that has been under CT for a long time with negligible aboveground crop residue incorporation (see also Uddin et al. (2021)). Since 2018, an annual rice–rice–rice pattern, a common cropping sequence on the floodplain soils of Bangladesh, has been followed. The farm site, in the Old Brahmaputra Floodplain, has a subtropical monsoon climate with a mean annual temperature of 26°C, average annual rainfall of 1,800 mm, and relative humidity of 85% (Weather Yard, BAU). The mean monthly rainfall and temperature during the rice cropping season were 127 mm and 25.4°C in 2019, respectively, and 171 mm and 26.8°C in 2020, respectively (Uddin et al., 2021). It is notable that the temperature and rainfall in this subtropical region has been relatively stable over the years. The field site with non-calcareous dark gray floodplain soil (Aeric Haplaquept in the U.S. Soil Taxonomy) was moderately drained with silt loam texture and had near neutral pH (6.5). At the onset of the experiment, soil organic carbon (0–15 cm depth) was 11.3 g kg−1, total N was 1.2 g kg−1, available P was 3.2 mg kg−1, exchangeable K was 0.04 g kg−1, and available S was 10.5 mg kg−1 (Uddin et al., 2021).
2.2 Crop Management
An annual sequence of three rice crops (Oryza sativa L), called hereafter Boro rice, transplanted Aus rice, and transplanted Aman rice, has been cultivated since 2018. The first crop of the sequence, Boro rice, was grown from January to May (mid-winter to pre-monsoon season), followed by transplanted Aus rice as a rain-fed crop from June to August (monsoon), and then transplanted Aman rice from August to December (late monsoon to winter). In the last week of January, land preparation began for transplanting Boro rice seedlings, followed by transplanting of Aus rice in the last week of May and transplanting of Aman rice seedlings in late August. Three seedlings were transplanted per hill with a 20 cm × 20 cm spacing. The variety for Boro rice was BRRI dhan63. The recommended dose (RD) of fertilizers for this soil was 144 kg N, 21 kg P, 60 kg K, 8 kg S, and 1.5 kg Zn ha−1 for Boro rice; 72 kg N, 7 kg P, 40 kg K, and 3 kg S ha−1 for Aus rice; and 90 kg N, 8.5 kg P, 50 kg K, 4 kg S, and 1 kg Zn ha−1 for Aman rice. N, P, K, S, and Zn were applied as urea, triple super phosphate, muriate of potash, gypsum, and zinc sulfate, respectively. Urea was applied in three equal splits at early tillering, active tillering, and panicle initiation stages on days 10, 35, and 55, respectively, after transplanting as a conventional application practice. Glyphosate (Round up®; ACI Bangladesh Ltd.), a non-selective herbicide, was sprayed over the field at a rate of 1.85 kg ha−1 3 days before the final land preparation. In addition, Pretilachlor (Superhit®, post emergence herbicide) was used at a rate of 450 g ha−1 7 days after transplanting the rice seedlings. Brifer 5G and Cidial 5G (ACI Bangladesh Ltd.) were applied as required to control rice insects. The rice fields were irrigated a day before the final land preparation and then when necessary to maintain standing water at about 3 cm above the soil surface throughout the growing season.
2.3 Experimental Design and Treatment Applications
In July 2018, the experiment was initiated with two soil disturbance levels (strip tillage, ST and conventional tillage, CT), two crop residue retention levels [low residue (LR): 15% and high residue (HR): 40%, by height], and three N fertilization rates [108 (N1), 144 (N2), and 180 (N3) kg N ha−1], with 144 kg N ha−1 being the recommended N fertilizer rate (FRG, 2018). In the ST system, soil was not puddled and left undisturbed except for a rotary tillage of 3 cm furrows for seeding or transplanting separated by 20 cm of undisturbed soil between rows using a versatile multi-crop planter (Haque et al., 2016). In the ST system, 15 and 40% residues of the previous crop were left standing, whereas in the CT system, the same amounts of residue were incorporated into the soil by repeated puddling using a rotary tiller. The 15% retention was comparable to present farmers’ practices. The experiment was laid out in a split-plot design, established with three replications for each treatment combination (Supplementary Figure S1). Rice soil is managed to develop a plough pan-limiting water percolation and increasing water retention capacity. The conversion of CT to ST was found to increase the water percolation rate due to lack of puddling. Therefore, the required irrigation interval was lower in ST than in CT to maintain a similar amount of standing water or water content in both treatments. For the Boro season, the water table depth is generally between 1 and 2 m below the ground level. Tillage treatment was assigned to the main plots, residue to the sub-plots, and fertilizer on top of the residue plots (sub-sub plot). The size of each sub-sub plot was 10 m × 4.2 m with a separating bund between the plots. The total number of plots was 36, including two tillage × two residue levels × three N rates × three replications.
2.4 Greenhouse Gas Sampling and Analysis
The GHG field measurements were conducted in Boro rice fields during the fifth crop after the initiation of the experiment in 2018 via a static chamber method (Hutchinson and Mosier, 1981). The observation period was started from the first split application of urea under continuous flooded condition and continued until the emissions declined to background levels (ambient concentration). Soda glass chambers (40 cm × 40 cm wide and 50 cm high) with stainless steel collars were placed on top of the rows, covering eight rice plants, to a depth of 10 cm (Zaman et al., 2021). Each collar had a neoprene seal which ensured an air-tight connection between the chamber lid and the frame. Urea was applied by the broadcast application method inside the pre-installed collars of the gas-collecting chambers. At each sampling event, the lids were placed on collars and gas samples were collected through the air tight rubber septa using a 20-ml polypropylene syringe equipped with a 25-gauge Luer lock needle at 0, 30, and 60 min. A 16-ml gas sample was collected from the headspace and injected into a pre-evacuated 12-ml vial (Labco Wycom Ltd.) to over pressurize the vials. The gas samples were collected on days 0, 1, 3, 5, 7, 10, 15, and 21 and repeated three times at 0, 30, and 60 min after chamber installation during the day between 10:00 a.m. and 4:00 p.m. after each split application of urea, deemed as the most representative time of a 24-h cycle (de Lima et al., 2018). The sample vials were stored for up to 7 days before analysis on a Varian 3,800 gas chromatograph (CP-3800, Varian, Inc., Switzerland) equipped with an electron capture detector (N2O) using argon (Ar) as the carrier gas, a thermal conductivity detector (CO2) using N2 as the carrier gas, and a flame ionization detector (CH4) using N2 as the carrier gas.
Calculations of Greenhouse Gas Emissions and the Emission Factors
Methane and N2O emissions at each sampling time were calculated from the change in headspace concentration based on a linear regression of measurements over 60 min Eq. 1
where dGas in ppb is the concentration change over time, dt is the difference in time, 10x is recalculation (here 10–9), Vchamber is the volume of the chamber used, p is the atmospheric pressure in Pa (100 is to convert Pa to hPa), MW is the molecular weight of CH4-C or N2O-N, R is the gas constant 8.314 J mol−1 K−1, T is the temperature in Kelvin, 10y is recalculation (here 106 (µg)), and A is the area of the chamber. Emissions for CT and ST were averaged across the residue and N-input rates and for LR and HR across CT and ST systems and N input rates. The cumulative CH4 and N2O emissions were calculated by summing up all daily fluxes for the entire experimental period by linear interpolation between the sample points (Zhang et al., 2013). Seasonal cumulative CH4 and N2O emissions were estimated following the method proposed by Mosier et al. (2006).
The N2O EFs were calculated based on the method proposed by Huang et al. (2017) Eq. 2, assuming that N2O emissions from a true 0 N control treatment was negligible.
where EF = emission factor, N2O emissions from fertilizer N treatment are in kg N ha−1 season−1, and applied fertilizer N is in kg N ha−1 season−1.
The yield-scaled GHG gas emissions (kg per t of grain) were estimated by dividing the cumulative CH4 or N2O emissions (kg CH4-C or N2O-N ha−1) by the grain yield (t ha−1). For calculating the area-based net global warming potential (GWP), the combined seasonal cumulative emissions of CH4 and N2O were converted to their CO2 equivalent (Ahmed et al., 2009; Hou et al., 2012). The GWP (over 100 years) conversion parameters used for CH4 and N2O were 34 and 298 kg ha−1 CO2 equivalents, respectively [IPCC (Intergovernmental Panel on Climate Change), 2013; Wang et al., 2015]. The yield-scaled global warming potential (GWPY), a metric that assesses the GWP per unit of yield, was calculated following the method proposed by van Groenigen et al. (2010).
2.5 Soil Sampling and Analysis
Composite soil samples were collected from each replicated plot at 0–15 cm depth with an auger at 4 days after the second split application of urea (active tillering stage) which corresponded very well to the maximum peak of N2O emissions. Soil samples were collected from several spots in a plot adjacent to each GHG gas sampling chamber and stored in sealable plastic bags in a cold room at 4°C. The soil pH was measured in the field during GHG sampling using a portable pH meter (Direct Soil pH Portable Meter, HI12923; Hanna Instruments). A portion of the field-moist soil was processed after sieving through a 2-mm mesh to remove visible plant roots and litters, and analyzed for soil ammonium (NH4+) and nitrate (NO3−) contents using the colorimetric method (Keeny and Nelson, 1982). The other portion of the soil was air-dried under shade at room temperature (∼25°C) for two weeks and processed (2- mm sieved) for the analysis of soil organic carbon (SOC) by the wet oxidation method (Walkley, 1947) and total N by the Kjeldahl method (Fawcett, 1954).
2.6 Estimation of Rice Yield
At harvesting, the grain yield in t ha−1 was determined from 4 m2 areas of each replicated plot. Grain yields were adjusted to 14% moisture.
2.7 Statistical Analysis
A split-split plot three-way analysis of variance was performed using tillage, residue level, and N application rate as fixed variables, where each of the three factors was considered as a main factor. The distribution of data for normality was checked before the analysis of variance. Data were statistically analyzed to ascertain the significant differences for the main effects and interactions among tillage, residue level, and N application rate treatments. To separate differences among the means, post hoc tests were performed using the Tukey-Kramer multiple comparison test. All statistical analyses were considered significant at p ≤ 0.05, unless otherwise mentioned, and were performed on Statistics 10 and Jamovi1.0.0.0. (R Package).
3 Results
3.1 Time Course of N2O and CH4 Emissions After N Fertilization
The N2O emission peaks increased with the growth stages of rice plants reaching the highest emission peaks at the panicle initiation stage (50–55 days after transplanting, DAT) and the lowest at the early tillering stage (15 DAT) (Figure 1). The highest peak was found on day three after urea application in all three stages of plant growth and amounted to about 10% of total N2O emissions. Surprisingly, the emission peaks were similar among the treatments at the early tillering stage but appeared to be different at latter stages. For example, the N2O emission peak was 14, 52, and 77 g N ha−1 d−1 in ST, and 14, 44, and 64 g N ha−1 d−1 in CT at the early tillering, active tillering, and panicle initiation (PI) stages, respectively (Figure 1A).
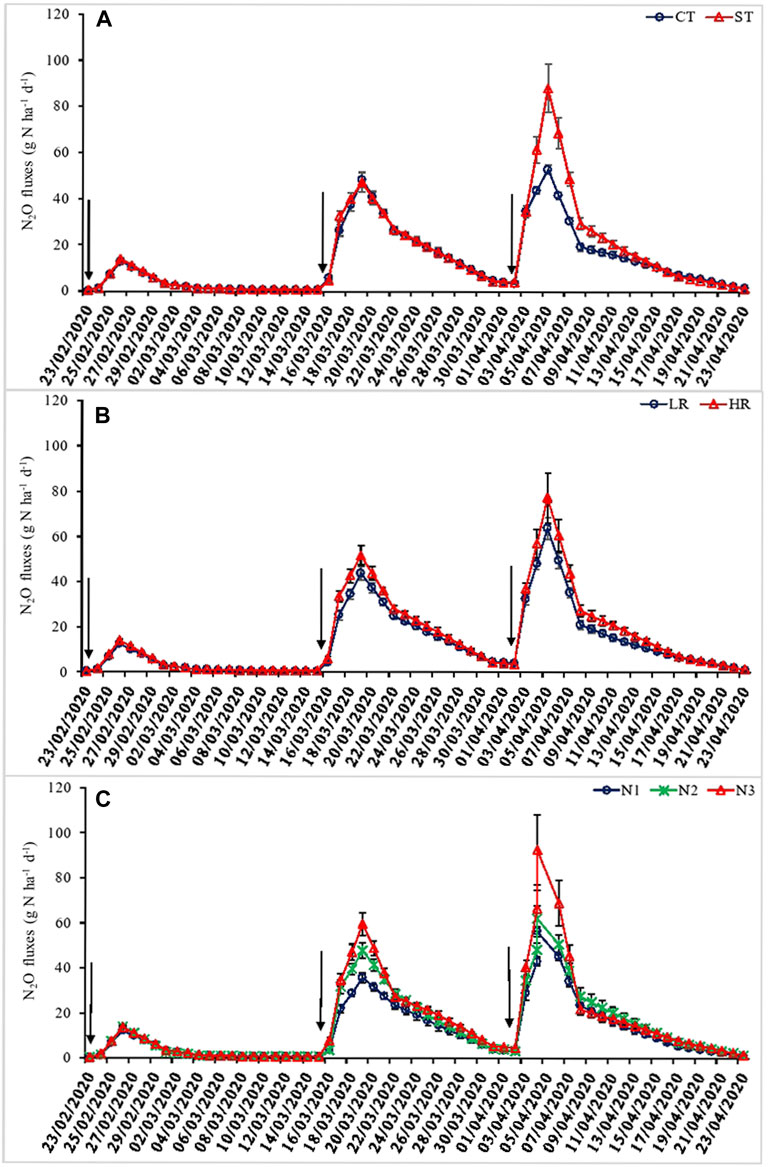
FIGURE 1. N2O emissions (mean ± SE; n = 3) in (A) two tillage systems, (B) two residue levels, and (C) three N application rates over time, days after urea application, in conventional (CT) and strip (ST) tillage systems across the residue levels and N rates in Boro rice; arrow shows the day of urea application.
Like N2O, the CH4 emission peaks were lower at the early tillering stage than at the active tillering and PI stages (Figure 2). The CH4 emission peaks were as high as 6, 15, and 12 kg C ha−1 d−1 in CT and 4, 12, and 10 kg C ha−1 d−1 in ST at early tillering, active tillering, and PI stages, respectively. The highest CH4 emission peak accounted for 10–15% of the total emissions in all treatments.
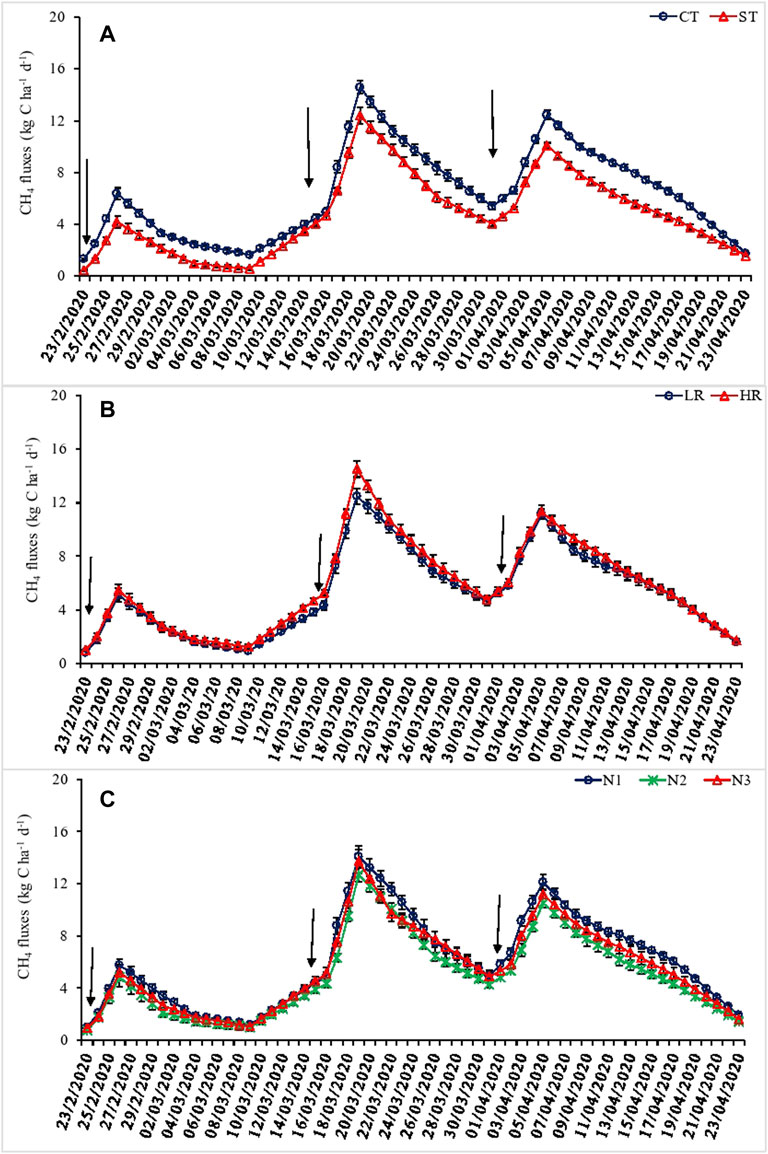
FIGURE 2. CH4 emissions (mean ± SE; n = 3) in (A) two tillage systems, (B) two residue levels, and (C) three N application rates over time, days after urea application, in conventional (CT) and strip (ST) tillage systems across the residue levels and N rates in Boro rice; arrow shows the day of urea application.
3.2 N2O Emissions Under Different Management Practices
The N2O emissions from the rice paddy fields were significantly influenced by tillage, residue levels, and N fertilization rates (Table 1). The mean (±SE) N2O emission rates were significantly higher in ST (15.7 ± 0.69 g N ha−1 d−1) than in CT (13.5 ± 0.70 g N ha−1 d−1) (Table 1). Likewise, the cumulative N2O emissions were 16% higher in ST (957 g N ha−1) than in CT (822 g N ha−1). The mean N2O emission rates were 18% higher in HR than in LR. The N2O emission rates linearly increased with the fertilizer N rate, from 12.7 ± 0.61 to 14.6 ± 0.62 and 16.9 ± 0.91 mg N ha−1 d−1 in N1, N2, and N3, respectively, irrespective of the tillage and residue level. The mean N2O emission rates increased significantly by about 15% with each increase in the N rate. The interaction effects of tillage × residue × N rate were significant, resulting in the highest N2O emissions in ST with HR coupled with N3 (21.3 g N ha−1 d−1), whereas the lowest N2O emissions were found in the combination of CT, LR, and N1 (9.63 g N ha−1 d−1) (Table 1). The highest cumulative N2O emissions were also observed in ST coupled with HR and N3 which were 117% higher than the lowest emissions in CT with LR and N1 and 80% higher than the conventional management practices (CT with LR in N2). The cumulative N2O emissions were 30% higher in ST with HR coupled with N2 than the conventional management practices (CT with LR in N2).
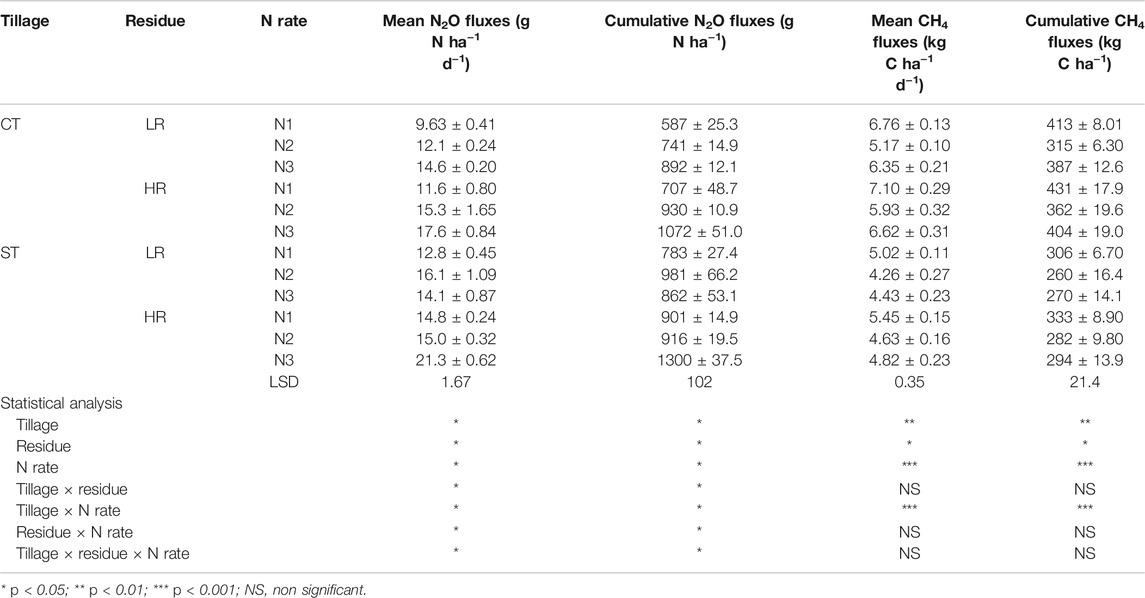
TABLE 1. Effect of tillage systems (conventional tillage, CT, and strip tillage, ST), residue levels (low residue, LR, and high residue HR), and N application rates (108, 144, and 180 kg ha−1) on mean and cumulative N2O and CH4 emissions in rice paddy (Boro rice) (n = 3; mean ± SE).
3.3 CH4 Emissions Under Different Management Practices
The mean CH4 emission rates were significantly influenced by tillage systems, residue levels, and N fertilization rates (Table 1). The mean CH4 emission rates were significantly higher in CT than in ST by 32%. The cumulative CH4 emissions during the measurement period were also higher in CT than in ST (Table 1). High crop residue levels significantly increased the mean CH4 emission rates, which were 5.76 and 5.33 kg C ha−1 d−1 in HR and LR, respectively. Similarly, cumulative CH4 emissions were higher in HR than in LR. Mean CH4 emission rates were 21 and 11% higher in N1 and N3 than in N2, respectively (Table 1). The recommended N application rate (N2) in ST plus LR or HR reduced the cumulative CH4 emissions by 10–18% over the current tillage and residue management practices (i.e., N2 in CT with LR).
3.4 Management Effects on Yield-Scaled N2O Emissions and N2O Emission Factors
The total yield-scaled N2O emissions over the experimental duration were not significantly different in CT and ST, with a mean value of 0.27 and 0.29 kg N t−1, respectively (Table 2). Like tillage, the crop residue levels had no significant effect on yield-scaled N2O emissions. However, the yield-scaled N2O emissions were significantly lower in N3 than in N1 but equal to N2 since the latter two were similar to each other. The interaction effects of tillage, residue level, and N fertilization rate were non-significant.
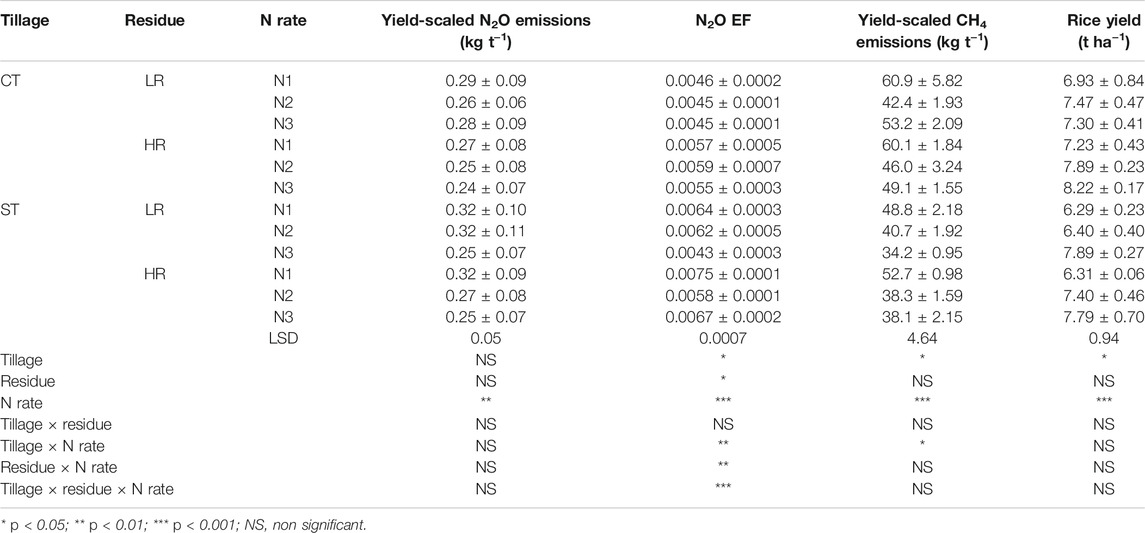
TABLE 2. Effect of tillage systems (conventional tillage, CT and strip tillage, ST), residue levels (low residue, LR and high residue HR), and N application rates (108, 144, and 180 kg ha−1) on Boro rice yield, yield-scaled CH4, and N2O emissions, and N2O emission factor (n = 3; mean ± SE).
The mean N2O EFs were significantly higher in ST (0.0062 ± 0.0003) than in CT (0.0051 ± 0.0002) (Table 2). Similar to the tillage effect, the N2O EF was significantly higher in HR (0.0062 ± 0.0002) than in LR (0.0051 ± 0.0002). The N2O EF was significantly higher in N1 than in N2 and N3 where the latter two were alike (Table 2). The interaction effects of tillage x N fertilization rate, residue level × N fertilization rate, and tillage × residue level × N fertilization rate were significant. The highest N2O EF was found in ST with HR coupled with N1, while the lowest was found in CT with LR in all N fertilization rates, which is equal to ST with LR in N3.
3.5 Management Impacts on Yield-Scaled CH4 Emissions
The ST significantly reduced yield-scaled CH4 emissions by 23% over the CT (Table 2). Yield-scaled CH4 emissions between high and low residue levels were similar to each other, with the mean values of 46.7 ± 2.35, and 47.4 ± 2.01 kg C t−1, respectively. The lowest rate of N fertilizer (N1) increased yield-scaled CH4 emissions by 33 and 27% over N2 and N3, but the higher N rates were similar to each other. The interaction effects of tillage × N rate were significant, resulting in the highest yield-scaled CH4 emissions in CT coupled with N1 in either residue level. The lowest yield-scaled CH4 emissions were in ST coupled with N3 in both residue levels which was equal to the emissions in ST with N2 at both residue levels.
3.6 Global Warming Potential of CH4 and N2O Emissions in Rice
The conversion of CT to ST significantly reduced the seasonal GWP from the combined emissions of CH4 and N2O from the wetland rice field. The mean GWP was 24% higher in CT (293 kg CO2-e ha−1) than in ST (224 kg CO2-e ha−1) (Table 3; Figure 3). Crop residue levels significantly increased the GWP of combined CH4 and N2O emissions in rice by 21%, with a mean GWP of 269 and 248 kg CO2-e ha−1 in HR and LR, respectively. Interestingly, the optimum fertilizer N rate had a significantly lower GWP (N2; 233 kg CO2-e ha−1) than the higher (N3; 282 kg CO2-e ha−1) and the lower N rates (N1; 260 kg CO2-e ha−1) (Table 3). The interaction effects of tillage × residue level × fertilizer N rate were significant so that the highest GWP from combined CH4 and N2O emissions was in CT with HR coupled with N1 and lowest in ST with either residue level coupled with N2 (Table 3). The ST with LR in N2 reduced the GWP from combined CH4 and N2O emissions by 39% over the GWP in CT with HR in N1. The yield-scaled GWP was significantly higher in CT with N1 in either residue level than in ST with N1and ST with N2 where the latter two were similar to each other in either residue level (Table 3).
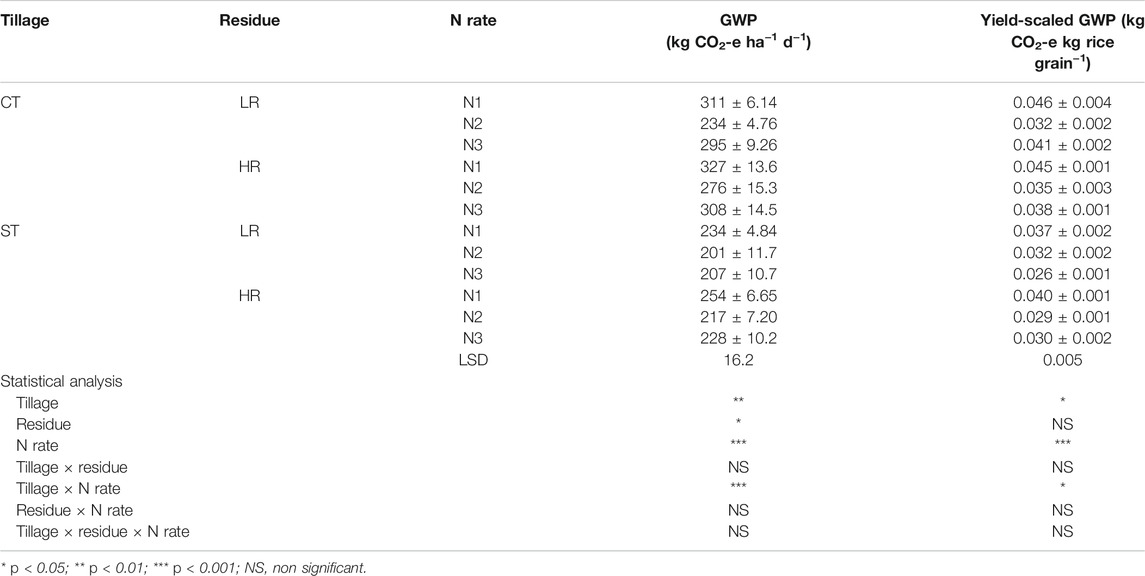
TABLE 3. Effect of tillage systems (conventional tillage, CT and strip tillage, ST), residue levels (low residue, LR and high residue, HR), and N application rates (108, 144, and 180 kg ha−1) on global warming potential (GWP) and yield-scaled GWP of CH4 and N2O emissions in Boro rice (n = 3; mean ± SE).
3.7 Rice Yield
After four crops of continued CA practices, the Boro rice yield was significantly higher in CT than in ST at a mean yield of 7.51 ± 0.19 and 7.01 ± 0.22 t ha−1, respectively (Table 2). The mean rice yields at the two residue levels were not significantly different. With each increment in the fertilizer N rate, the rice yield increased significantly. The interaction effects of tillage, residue level, and N fertilizer rate were non-significant.
3.8 Soil Properties
After 2 years of CA practices, the soil pH was slightly higher in ST (6.66 ± 0.03) than in CT (6.61 ± 0.02) but not significantly different between HR and LR (Table 4). The N fertilization rate significantly increased the soil pH, from 6.52 in N1 to 6.75 in N3, independent of tillage and residue levels (Table 4). The interaction effects of the tillage and N fertilization rate caused a significantly higher pH in ST with N3 in either residue. The tillage × residue level × N fertilization interaction showed a significantly higher TN in ST with either residue level in combination with N2 or N3 than any other treatment combinations that included N1. The soil NH4+ content was significantly higher in ST than in CT (ca. 9.9 ± 0.46 and 8.4 ± 0.41 mg N kg−1 in ST and CT, irrespective of residue and fertilizer rate), but the values were similar in HR to LR (Table 4). The N fertilization rate significantly increased the NH4+ content in N3 compared to N1 and N2. By contrast, the soil NO3− —N content was similar in ST to CT and in HR to LR. The NO3−–N content was lower in N1 than N2 and N3 where the latter two were similar to each other. After five crops, the SOC was unchanged by tillage and residue levels, but the suboptimal N fertilization rate (N1) caused a lower SOC than under N2 and N3.
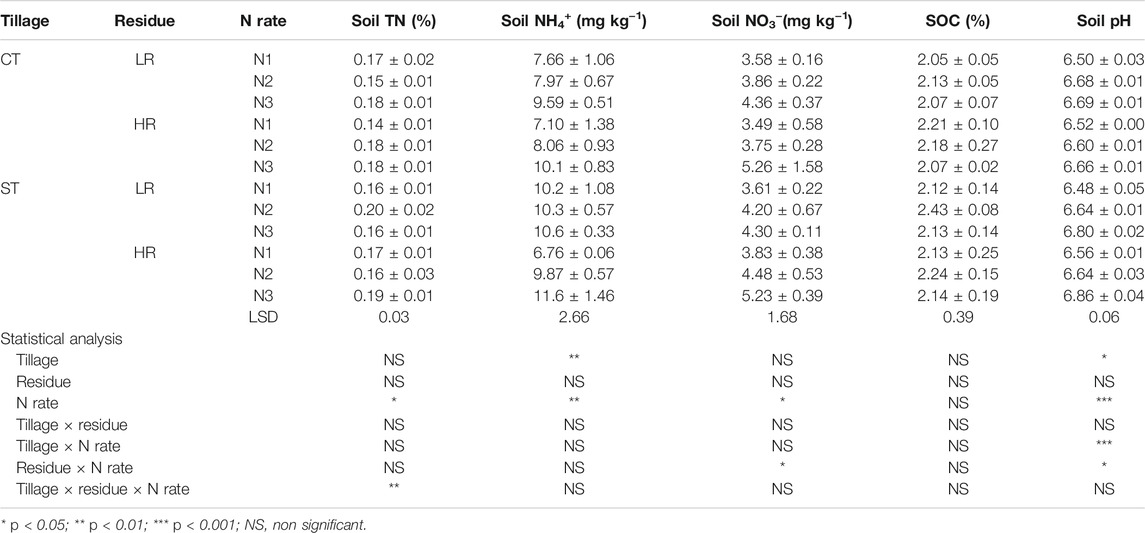
TABLE 4. Effect of tillage systems (conventional tillage, CT and strip tillage, ST), residue levels (low residue, LR and high residue, HR) and N application rates (108, 144, and 180 kg ha−1) on soil organic carbon (SOC), total and mineral N, and pH (n = 3; mean ± SE).
4 Discussion
4.1 Effect of Tillage, Crop Residue, and N Fertilization Rate on the GWP of Rice
The adoption of minimum soil disturbance (ST) and HR retention with the recommended fertilizer N rate reduced the GWP from CH4 and N2O emissions by 10% relative to the present farmers’ practice (CT with LR) at the same N fertilizer rate. However, if ST is combined with LR and the recommended fertilizer rate (N2), it reduced the GWP by 39% relative to LR in combination with the recommended fertilizer rate under CT. Equally, if we consider yield-scaled GWP for the recommended N rate, ST with HR, which represents a CA cropping system, reduced the GWP from combined CH4 and N2O emissions by 9% relative to present farmers’ practices (CT with LR). Overall, the GWP was lower for ST with either LR or HR than CT with LR under the recommended N fertilizer rate.
While CA had opposite effects on CH4 and N2O emissions, the net effect was to decrease the GWP since CH4 emissions dominate the total GWP in paddy rice, accounting for around 96% of the total GWP. Since rice is usually grown under flooded conditions, CH4 emissions make a major contribution to the GWP (Linquist B. et al., 2012). Contributions of N2O emissions to the GWP of GHGs in rice were reported in previous studies to be low compared to CH4 emissions (Ly et al., 2013; Vu et al., 2015; Alam et al., 2016, 2019a), but emissions increased after N fertilizer application (Zou et al., 2005; Pandey et al., 2014).
If we consider the total seasonal N2O emissions, they were relatively large, ranging from 0.59 kg N ha−1 to 1.30 kg N ha−1. These results were comparable with seasonal N2O emissions from wheat (ranged 0.32 kg N ha−1–1.20 kg N ha−1) under the same management practices and the same agroclimatic conditions (Jahangir et al., 2021b). This suggests that paddy rice is also a presently insufficiently recognized source of N2O even though it is relatively less important than CH4 under these conditions. Hence, management interventions such as optimum N fertilizer rates that decrease the seasonal N2O emissions from rice paddy fields also need further attention as a means to lower the GWP of rice paddy.
4.2 Management Impacts on Greenhouse Gas Emissions
4.2.1 Management Impacts on N2O Emissions
The N2O emissions from the Boro rice crop increased because of the conversion of CT to ST, especially when coupled with higher residue levels and higher N application rates. In ST, the accumulation of soil C and N close to the surface may accelerate the loss of N2O from soil (Kay and VandenBygaart, 2002). The increase in N2O emissions under ST points to less oxygen being available (= lower redox) due to minimum soil disturbance. Uddin et al. (2021) reported that the redox potential of soil was lower under CA, and suggested that this would increase the rate of N2O formation via denitrification. Lower N2O emissions in rice paddy in CT were in agreement with other studies (Steinbach and Alvarez, 2006; Ahmed et al., 2009; Zhang et al., 2013). The increase in N2O emissions with higher residue levels and N application rates can be attributed to the greater availability of organic and mineral N for nitrifying or denitrifying microbes. Higher residue levels may have increased the abundance of electron donors (SOC) for denitrifiers (Chen et al., 2013). Higher residue levels can lead to more reducing soil conditions by consuming more O2 when the decomposition of residues occurs and thus enhance microbial denitrification processes, that is, an imbalance between NO and N2O reduction stimulating N2O emissions. Along with the direct effects, ST with higher residue levels, mulched on the soil surface, can indirectly increase N2O emissions by increasing the soil moisture conservation, especially when followed by intermittent wetting and drying (Sharma and Acharya, 2000) and by an increased soil aggregation which increases N2O diffusion (Jahangir et al., 2011). Yield-scaled N2O emissions were lower in the higher N application rate (N3) than the optimal (N2) or sub-optimal (N1) rates because yield did not increase in proportion to N2O emissions under varied fertilizer N rates.
The N2O EF was estimated based on the assumption that N2O emissions from the zero N control were negligible which was in line with previous studies, for example, Bronson et al. (1997) who reported that N2O emissions were rarely detected during the rice season except directly after fertilization. Similarly, Islam et al. (2018) and Zou et al. (2005) observed significant N2O emission peaks only after N fertilizer application. We observed that N2O emission peaks appeared only after N fertilizer application and they reduced to the detection limit within 12–18 days after urea application (Jahangir et al., 2021b).
The N2O EF for rice paddy under the present recommended N rate in both CA (ST with HR) and conventional (CT with LR) management practices (ca. 0.58 and 0.45%, respectively) was higher than the Intergovernmental Panel on Climate Change (IPCC) default value of 0.30% for rice [IPCC, 2006]. In ST, the water percolation rate may have been higher due to the lack of puddling which resulted in shorter irrigation intervals (data unpublished). Despite a higher number of irrigation events, water was applied to maintain similar levels of water in both ST and CT. The higher rates of percolation in ST may have enhanced oxygen diffusion in the surface soil, resulting in higher N2O emissions and thus higher N2O EF. Across all treatments, the N2O EF in our study ranged from 0.43 to 0.75%. It suggests that there is a higher N2O EF for rice paddy than the IPCC default value for the determination of the GWP of wetland paddy rice; however, these values need to be confirmed in subsequent studies that cover the entire growing season and a wider diversity of wetland rice production zones in the Eastern Gangetic Plain.
4.2.2 Management Impacts on CH4 Emissions
The conversion of CT to ST significantly reduced CH4 emissions. Zhang et al. (2013) compared conventional tillage, rotary tillage (RT), and no tillage (NT) for CH4 emissions in rice paddy and observed that no tillage (NT) significantly reduced the CH4 emissions (by 16–18%) during rice growing seasons. Similarly, Harada et al. (2007) showed that NT depressed CH4 emissions by 43% from a rice field compared with that of CT. Repeated ploughing and puddling in CT, which was absent in ST, can increase the mineralization of organic matter by its disturbance in soil and by disrupting soil aggregates. The decomposition of crop residues consumed O2 in soil pores which may inhibit CH4 oxidation and promote anaerobic conditions, resulting in higher net emissions (Hütsch, 2001). The tillage also influenced crop residue distribution in the soil where CT, through ploughing, can mix crop residues to a greater soil depth (Yamulki et al., 2002; Mangalassery et al., 2014). Reduced tillage enhanced soil organic matter accumulation through reduced mineralization (Chen et al., 2013), as well as physical protection of organic matter in soil aggregates (Jahangir et al., 2021a), but it tends to stratify organic matter closer to the soil surface. Gregorich et al. (2006) attributed the differences in gas fluxes between CT and NT to the differences in the physical conditions of soil. The NT can increase CH4 oxidation by improving the soil structure and decreasing the disturbance which maintains higher methanotrophic activity (Li et al., 2011). Undisturbed systems such as pristine ecosystems are generally the highest methanotrophic sink systems in the world (Price et al., 2004). Tellez-Rio et al. (2015) reported that reduced soil disturbance could reduce the disturbance to methanotrophic microbes and enhance the CH4 uptake. Reduced tillage or NT increases soil porosity and in turn increases aeration, thus increasing the CH4 oxidation potential (Zhang et al., 2013). From a meta-analysis, Feng et al. (2018) concluded that lower CH4 emissions in NT were possibly due to CH4 oxidation to CO2. The difference in CH4 emissions between LR and HR treatments in the CT and ST was similar, suggesting that the main effect of ST was reduced residue mixing and distribution which may have caused lower CH4 emissions than CT (Wang et al., 1998).
Higher CH4 emissions under higher crop residue levels are attributed to the increased substrate C supply for methanogenic activity (Ma et al., 2010). In addition, the decomposition of crop residues consumes soil-dissolved oxygen, which lowers the redox potential and further enhances CH4 production (Zhang et al., 2013). In ST, crop residues were not incorporated into the soil but surface mulched, which may have reduced the soil temperature (Zhang et al., 2013), leading to lower CH4 emissions (Whalen and Reeburgh, 1996; Zhu et al., 2007).
In this study, CH4 emissions were higher at the higher N application rate than at the recommended N rate (N2). The nitrogen fertilization rate increases CH4 emission because NH4+ production in flooded conditions after urea application inhibits CH4 consumption with a net effect toward CH4 emissions. The similarity in the size and structure of NH4+ and CH4 allows the CH4 monooxygenase enzyme to bind and react with NH4+ instead of CH4 (Gulledge and Schimel, 1998). Higher N application rates increase plant tillers and growth which can enhance CH4 emissions (Wang et al., 2015) as plant-mediated emissions are higher than those directly from the soil (Wassmann and Aulakh, 2000). Plants provide a conduit for CH4 loss directly from the soil where it is produced avoiding its oxidation in the thin surface layer on top of the paddy soil. By contrast, CH4 emissions were highest in the sub-optimal N application rate (N1). This may have been triggered by greater root exudations in response to the nutrient stress conditions in the soil that accelerated plant–soil–microbe interactions and CH4 production (Chen et al., 2014). The combination of ST and the optimum N rate had the lowest CH4 emissions which can be attributed to the soil conditions conducive for CH4 oxidation. Yield-scaled CH4 emissions were the lowest in ST coupled with the recommended N rate or the 25% higher N rate. Yield-scaled CH4 emissions under 25% higher N rates were lower because higher yield compensates for higher cumulative CH4 emissions. With less than the optimum N supply, there is a lower yield but more CH4 production than with the recommended N rate. Our results suggested that failure to optimize the N fertilizer rate can increase CH4 emissions under ST.
4.2.3 Pattern of CH4 and N2O Emissions After N Fertilization
Both CH4 and N2O emissions peaked at the active tillering to the PI stage of rice growth. Both GHGs showed clear peaks after the fertilizer N application that was followed by irrigation water supply. Linquist B. A. et al. (2012); Linquist et al. (2012 B) postulated that N fertilization boosts plant growth, which both increases the C supply for methanogens and provides a larger root aerenchyma pathway for the CH4 movement from the soil to the atmosphere. However, the higher CH4 and N2O emissions at later stages may be attributed to the higher vegetative growth of plants along with a vigorous root growth which enhances the availability of labile C through excretion of root exudates and increases the activities and community of methanogens or other heterotrophic microbes (Islam et al., 2020). In addition, GHGs produced in soil can exchange faster between the soil and the atmosphere through diffusion from soil through the aerenchyma tissue in rice roots. Moreover, the photosynthetic capacity of plants increases with plant growth and canopy development which also enhances the root mass of the plant, providing the substrate for CH4 production (Conrad, 1993).
The highest N2O emissions occurred on day 3 after urea application which is in line with the highest hydrolysis activity of the applied N resulting in an increased availability of NH4+ for nitrification and NO3− for denitrification, which is dependent on the oxidation–reduction state of bulk and rhizosphere soil. Uddin et al. (2021) also reported a higher NH4+ content in rice soil and standing water, as well as higher N loss via volatilization between 2 and 5 days after urea application in the rice fields. The highest peak of N2O emissions is comparable to the N2O emission peaks observed in past rice (Zou et al., 2005; Pandey et al., 2014) and wheat studies (Jahangir et al., 2021b). After maximum vegetative growth and panicle initiation, the CH4 emissions decreased gradually to a background level when the rice field was drained before harvesting (end of April). This result was in line with previous findings (Zhang et al., 2013; Islam et al., 2020).
4.3 Co-Benefits of CA on Soil Properties and on Yield
In our study, the effect of tillage, residue level, and N rate on mineral N concentrations was non-significant, whereas both ST and higher N rates resulted in higher NH4+ concentrations. Overall, the CA (ST with HR) increased soil TN, nitrate, and pH, whereas ST alone increased NH4+ availability. In this study, in the fifth crop of the rotation after conversion of CT to ST, the irrigated rice yield was 6.7% higher in CT than in ST. The rice yield in the first few years after the conversion of CT to ST was higher in CT than in ST (Li et al., 2015) or equal between CT and ST (Haque et al., 2016). The decrease in the yield due to conversion of CT to ST in the present study can be attributed to the transitional effect on soil physicochemical and biological properties. The ST can increase the soil microbial growth that can stimulate the mineralization–immobilization turnover (MIT) in later years. The mechanism for the temporal yield recovery under ST can be attributed to an increased SOM and nutrient availability, biological efficiency, and improved soil fertility properties (Islam and Weil, 2000; Samal et al., 2017). An increase in the rice yield with the increase in the N application rate indicates that the soil was deficient in N content and thus responded to the added N fertilizer. However, ST with HR and the recommended fertilizer N rate significantly increased soil TN over the conventional management (e.g., CT with LR) at the same N rate. The SOC was similar for all treatment combinations, which could be because of the short time period (<2 years) after the switch from CT to ST. In addition, SOC consumption by denitrification in some treatments or by CH4 production in other treatments can minimize the differences as higher N2O production corresponded to a lower CH4 production. Nitrate was easily denitrified under anaerobic conditions and the processes of denitrification consume electrons and H2, competing with CH4 producers (Kluber and Conrad, 1998).
5 Conclusion
Adoption of CA in a triple-rice cropping system increased N2O emissions but reduced CH4 emissions during the irrigation of wetland rice (Boro rice) crop, leading to a net reduction of the GWP (ca. 16%). The higher N2O emissions were off-set by the lower CH4 emissions in ST because CH4 contributed around 96% of the GWP in irrigated wetland rice. The current recommended N fertilization rate reduced CH4 emissions by 25% relative to lower or higher rates of N application, suggesting that under CA, the optimum rate of N is a suitable management decision for mitigating GHGs. The N2O emissions were higher in CA when higher residue levels were coupled with any N rate than conventional management practices (CT with LR) with the same N rate. The N2O EF was surprisingly high for wetland rice, ranging from 0.43 to 0.75%. With the recommended N application rate, the N2O EF was higher in ST plus LR/HR than in conventional management practices (CT plus LR). The study shows that the complex interactions among pH, SOC, TN, and mineral N, which increased under CA compared to conventional management practices, were associated with the mitigation of the GWP from N2O and CH4 emissions within five crop cycles after the transition to CA.
Data Availability Statement
The raw data supporting the conclusion of this article will be made available by the authors, without undue reservation.
Author Contributions
MJ: conceptualization, methodology, funding acquisition, supervision, formal analysis, writing—original draft, and writing—review and editing; RB: conceptualization, funding acquisition, and writing—review and editing; SU: methodology, data analysis, and writing—review and editing; JF: methodology and writing—review and editing. SN: methodology and writing—review and editing; MH: funding acquisition and writing—review and editing; MS: writing—review and editing. MZ: conceptualization and writing—reviewing and editing; WD: formal analysis and writing—reviewing and editing; MJ: funding acquisition, supervision, and writing—reviewing and editing; CM: conceptualization and writing—reviewing and editing.
Funding
The research was funded by a Krishi Goveshona Foundation (KGF) project administered by the Bangladesh Agriculture Research Council (BARC) in association with the Australian Centre for International Agricultural Research (ACIAR: Project LWR 2016/136), Soil and Water Management Division of IAEA (RAS5083 and CRP D1.50.20), and State Key laboratory of Soil and Sustainable Agriculture, Institute of Soil Science, Chinese Academy of Sciences.
Conflict of Interest
The authors declare that the research was conducted in the absence of any commercial or financial relationships that could be construed as a potential conflict of interest.
Publisher’s Note
All claims expressed in this article are solely those of the authors and do not necessarily represent those of their affiliated organizations, or those of the publisher, the editors, and the reviewers. Any product that may be evaluated in this article, or claim that may be made by its manufacturer, is not guaranteed or endorsed by the publisher.
Supplementary Material
The Supplementary Material for this article can be found online at: https://www.frontiersin.org/articles/10.3389/fenvs.2022.853655/full#supplementary-material
Supplementary Data S1 | Field layout showing the experimental design.
References
Ahmed, S. A., Diffenbaugh, N. S., and Hertel, T. W. (2009). Climate Volatility Deepens Poverty Vulnerability in Developing Countries. Environ. Res. Lett. 4, 034004. doi:10.1088/1748-9326/4/3/034004
Alam, M. K., Bell, R. W., and Biswas, W. K. (2019). Decreasing the Carbon Footprint of an Intensive rice-based Cropping System Using Conservation Agriculture on the Eastern Gangetic Plains. J. Clean. Prod. 218, 259–272. doi:10.1016/j.jclepro.2019.01.328
Alam, M. K., Bell, R. W., Haque, M. E., and Kader, M. A. (2018). Minimal Soil Disturbance and Increased Residue Retention Increase Soil Carbon in rice-based Cropping Systems on the Eastern Gangetic Plain. Soil Tillage Res. 183, 28–41. doi:10.1016/j.still.2018.05.009
Alam, M. K., and Bell, R. W. (2020). Soil Nitrogen Storage and Availability to Crops Are Increased by Conservation Agriculture Practices in rice–based Cropping Systems in the Eastern Gangetic Plains. Field Crops Res. 250, 107764. doi:10.1016/j.fcr.2020.107764
Alam, M. K., Biswas, W. K., and Bell, R. W. (2016). Greenhouse Gas Implications of Novel and Conventional rice Production Technologies in the Eastern-Gangetic plains. J. Clean. Prod. 112, 3977–3987. doi:10.1016/j.jclepro.2015.09.071
Bell, R., Haque, M., Jahiruddin, M., Rahman, M., Begum, M., Miah, M., et al. (2019). Conservation Agriculture for rice-based Intensive Cropping by Smallholders in the Eastern Gangetic Plain. Agriculture 9, 5. doi:10.3390/agriculture9010005
Bronson, K. F., Neue, H.-U., Abao, E. B., and Singh, U. (1997). Automated Chamber Measurements of Methane and Nitrous Oxide Flux in a Flooded Rice Soil: I. Residue, Nitrogen, and Water Management. Soil Sci. Soc. America J. 61, 981–987. doi:10.2136/sssaj1997.03615995006100030038x
Carlson, K. M., Gerber, J. S., Mueller, N. D., Herrero, M., MacDonald, G. K., Brauman, K. A., et al. (2017). Greenhouse Gas Emissions Intensity of Global Croplands. Nat. Clim Change 7, 63–68. doi:10.1038/nclimate3158
Chen, C., Chen, D., Pan, J., and Lam, S. K. (2013). Application of the Denitrification-Decomposition Model to Predict Carbon Dioxide Emissions under Alternative Straw Retention Methods. ScientificWorldJournal 2013, 851901. doi:10.1155/2013/851901
Chen, R., Senbayram, M., Blagodatsky, S., Myachina, O., Dittert, K., Lin, X., et al. (2014). Soil C and N Availability Determine the Priming Effect: Microbial N Mining and Stoichiometric Decomposition Theories. Glob. Change Biol. 20, 2356–2367. doi:10.1111/gcb.12475
Conrad, R. (1993). “Microbial Metabolism of Nitric Oxide in Soil,” in Trends in Microbial Ecology. Editors R. Guerrero, and C. Pedros-Alio (Barcelona: Spanish Soc. Microbiol), 67–70.
de Lima, M. A., Pazianotto, R. A. A., Villela, O. V., and Paraíba, L. C. (2018). Diurnal Variation of Methane Emission from a Paddy Field in Brazilian Southeast. Ciênc. Rural 48 (04), e20170054. doi:10.1590/0103-8478cr20170054
EPA (2013). Global Mitigation of Non-CO2 Greenhouse Gases: 2010–2030. Washington, DC): United States Environmental Protection Agency, Office of Atmospheric Programs, 19–42.
Erisman, J. W., Sutton, M. A., Galloway, J., Klimont, Z., and Winiwarter, W. (2008). How a century of Ammonia Synthesis Changed the World. Nat. Geosci 1, 636–639. doi:10.1038/ngeo325
Fawcett, J. K. (1954). The Semi-micro Kjeldahl Method for the Determination of Nitrogen. J. Med. Lab. Technol. 12, 1–22.
Feng, J., Li, F., Zhou, X., Xu, C., Ji, L., Chen, Z., et al. (2018). Impact of Agronomy Practices on the Effects of Reduced Tillage Systems on CH4 and N2O Emissions from Agricultural fields: A Global Meta-Analysis. PLoS One 13 (5), e0196703. doi:10.1371/journal.pone.0196703
FRG (2018). Fertilizer Recommendation Guide. Farmgate: Bangladesh Agricultural Research Council. Khamarbari road, Dhaka-1215.
Gaihre, Y. K., Tirol-Padre, A., Wassmann, R., Aquino, E., Pangga, G. V., and Sta-Cruz, P. C. (2011). Spatial and Temporal Variations in Methane Fluxes from Irrigated lowland rice fields. Philipp. Agric. Sci. 94, 335–342.
Gregorich, E., Rochette, P., Hopkins, D., McKim, U., and Stgeorges, P. (2006). Tillage-induced Environmental Conditions in Soil and Substrate Limitation Determine Biogenic Gas Production. Soil Biol. Biochem. 38, 2614–2628. doi:10.1016/j.soilbio.2006.03.017
Gulledge, J., and Schimel, J. P. (1998). Moisture Control over Atmospheric CH4 Consumption and CO2 Production in Diverse Alaskan Soils. Soil Biol. Biochem. 30, 1127–1132. doi:10.1016/s0038-0717(97)00209-5
Haque, M. E., Bell, R. W., Islam, M. A., and Rahman, M. A. (2016). Minimum Tillage Unpuddled Transplanting: An Alternative Crop Establishment Strategy for rice in Conservation Agriculture Cropping Systems. Field Crops Res. 185, 31–39. doi:10.1016/j.fcr.2015.10.018
Harada, H., Kobayashi, H., and Shindo, H. (2007). Reduction in Greenhouse Gas Emissions by No-Tilling rice Cultivation in Hachirogata Polder, Northern Japan: Life-Cycle Inventory Analysis. Soil Sci. Plant Nutr. 53, 668–677. doi:10.1111/j.1747-0765.2007.00174.x
Heffer, P. (2009). Assessment of Fertilizer Use by Crop at the Global Level 2006–2007. Paris: International Fertilizer Industry Association.
Hou, H., Peng, S., Xu, J., Yang, S., and Mao, Z. (2012). Seasonal Variations of CH4 and N2O Emissions in Response to Water Management of Paddy fields Located in Southeast China. Chemosphere 89, 884–892. doi:10.1016/j.chemosphere.2012.04.066
IPCC (2006). in 2006 IPCC Guidelines for National Greenhouse Gas Inventories, Prepared by the National Greenhouse Gas Inventories Programme. Editors H. S. Eggleston, L. Buendia, K. Miwa, T. Ngara, and K. Tanabe (PublishedJapan: IGES).
Huang, T., Yang, H., Huang, C., and Ju, X. (2017). Effect of Fertilizer N Rates and Straw Management on Yield-Scaled Nitrous Oxide Emissions in a maize-wheat Double Cropping System. Field Crops Res. 204, 1–11. doi:10.1016/j.fcr.2017.01.004
Hutchinson, G. L., and Mosier, A. R. (1981). Improved Soil Cover Method for Field Measurement of Nitrous Oxide Fluxes. Soil Sci. Soc. America J. 45, 311–316. doi:10.2136/sssaj1981.03615995004500020017x
Hütsch, B. W. (2001). Methane Oxidation, Nitrification, and Counts of Methanotrophic Bacteria in Soils from a Long-Term Fertilization experiment. J. Plant Nutr. Soil Sci. 164 (1), 21–28. doi:10.1002/1522-2624(200102)164:1<21::aid-jpln21>3.0.co;2-b
Islam, A. K. M. S., Hossain, M. M., and Saleque, M. A. (2014). Effect of Non-puddled Transplanting on the Growth and Yield of Dry Season rice (Oryza Sativa L.) in High Barind Tract. Agriculturist 12, 91–97. doi:10.3329/agric.v12i2.21736
Islam, K. R., and Weil, R. R. (2000). Land Use Effects on Soil Quality in a Tropical forest Ecosystem of Bangladesh. Agric. Ecosyst. Environ. 79, 9–16. doi:10.1016/s0167-8809(99)00145-0
Islam, S. F.-u., Sander, B. O., Quilty, J. R., de Neergaard, A., van Groenigen, J. W., and Jensen, L. S. (2020). Mitigation of Greenhouse Gas Emissions and Reduced Irrigation Water Use in rice Production through Water-Saving Irrigation Scheduling, Reduced Tillage and Fertiliser Application Strategies. Sci. Total Environ. 739, 140215. doi:10.1016/j.scitotenv.2020.140215
Islam, S. F.-u., van Groenigen, J. W., Jensen, L. S., Sander, B. O., and de Neergaard, A. (2018). The Effective Mitigation of Greenhouse Gas Emissions from rice Paddies without Compromising Yield by Early-Season Drainage. Sci. Total Environ. 612, 1329–1339. doi:10.1016/j.scitotenv.2017.09.022
Jahangir, M. M. R., Begum, R., Jahiruddin, M., Dawar, K., Zaman, M., Bell, R. W., et al. (2021b). Reduced Tillage with Residue Retention and Nitrogen Application Rate Increase N2O Fluxes from Irrigated Wheat in a Subtropical Floodplain Soil. Agric. Ecosyst. Environ. 306, 107194. doi:10.1016/j.agee.2020.107194
Jahangir, M. M. R., Jahiruddin, M., Akter, H., Pervin, R., and Islam, K. R. (2021a). Cropping Diversity with rice Influences Soil Aggregate Formation and Nutrient Storage under Different Tillage Systems. J. Plant Nutr. Soil Sci. 184, 150–162. doi:10.1002/jpln.202000310
Jahangir, M. M. R., Roobroeck, D., Van Cleemput, O., and Boeckx, P. (2011). Spatial Variability and Biophysicochemical Controls on N2O Emissions from Differently Tilled Arable Soils. Biol. Fertil. Soils 47 (7), 753–766. doi:10.1007/s00374-011-0580-2
Kay, B. D., and VandenBygaart, A. J. (2002). Conservation Tillage and Depth Stratification of Porosity and Soil Organic Matter. Soil Tillage Res. 66, 107–118. doi:10.1016/s0167-1987(02)00019-3
Keeney, D. R., and Nelson, D. W. (19821982). “Nitrogen-Inorganic Forms,” in Methods of Soil Analysis. 2nd ed. (Madison, WI, USA: ASA and SSSA), Part 2, 643. Agronomy 9.
Kirschke, S., Bousquet, P., Ciais, P., Saunois, M., Canadell, J. G., Dlugokencky, E. J., et al. (2013). Three Decades of Global Methane Sources and Sinks. Nat. Geosci 6, 813–823. doi:10.1038/ngeo1955
Klüber, H., and Conrad, R. (1998). Effects of Nitrate, Nitrite, NO and N2O on Methanogenesis and Other Redox Processes in Anoxic rice Field Soil. FEMS Microbiol. Ecol. 25 (3), 301–319. doi:10.1016/s0168-6496(98)00011-7
Kritee, K., Nair, D., Zavala-Araiza, D., Proville, J., Rudek, J., Adhya, T. K., et al. (2018). High Nitrous Oxide Fluxes from rice Indicate the Need to Manage Water for Both Long- and Short-Term Climate Impacts. Proc. Natl. Acad. Sci. U.S.A. 115 (39), 9720–9725. doi:10.1073/pnas.1809276115
Li, H., He, J., Gao, H., Chen, Y., and Zhang, Z. (2015). The Effect of Conservation Tillage on Crop Yield in China. Front. Agr. Sci. Eng. 2 (2), 179–185. doi:10.15302/j-fase-2015058
Li, X., Yuan, W., Xu, H., Cai, Z., and Yagi, K. (2011). Effect of Timing and Duration of Midseason Aeration on CH4 and N2O Emissions from Irrigated lowland rice Paddies in China. Nutr. Cycl. Agroecosyst. 91, 293–305. doi:10.1007/s10705-011-9462-0
Linquist, B. A., Adviento-Borbe, M. A., Pittelkow, C. M., van Kessel, C., and van Groenigen, K. J. (2012a). Fertilizer Management Practices and Greenhouse Gas Emissions from rice Systems: A Quantitative Review and Analysis. Field Crops Res. 135, 10–21. doi:10.1016/j.fcr.2012.06.007
Linquist, B. A., Anders, M. M., Adviento‐Borbe, M. A. A., Chaney, R. L., Nalley, L. L., Da Rosa, E. F. F., et al. (2015). Reducing Greenhouse Gas Emissions, Water Use, and Grain Arsenic Levels in rice Systems. Glob. Change Biol. 21, 407–417. doi:10.1111/gcb.12701
Linquist, B., Groenigen, K. J., Adviento-Borbe, M. A., Pittelkow, C., and Kessel, C. (2012b). An Agronomic Assessment of Greenhouse Gas Emissions from Major Cereal Crops. Glob. Change Biol. 18, 194–209. doi:10.1111/j.1365-2486.2011.02502.x
Ly, P., Jensen, L. S., Bruun, T. B., and de Neergaard, A. (2013). Methane (CH4) and Nitrous Oxide (N2O) Emissions from the System of rice Intensification (SRI) under a Rain-Fed lowland rice Ecosystem in Cambodia. Nutr. Cycl. Agroecosyst. 97, 13–27. doi:10.1007/s10705-013-9588-3
Ma, X., Chen, L., Chen, Z., Wu, Z., Zhang, L., and Zhang, Y. (2010). Soil Glycosidase Activities and Water-Soluble Organic Carbon under Different Land Use Types. Rev. Cienc. Suelo Nutr. Veg. 10, 93–101. doi:10.4067/s0718-27912010000200001
Mangalassery, S., Dayal, D., Meena, S. L., and Ram, R. (2014). Carbon Sequestration in Agroforestry and Pasture Systems in Arid Northwestern India. Curr. Sci. 107 (8), 1290–1293.
MOEFCC (2012). Government of India’s Second National Communication to the United Nations Framework Convention on Climate Change. New Delhi): Ministry of Environment, Forest and Climate Change.
Moraru, P. I., and Rusu, T. (2012). Effect of Tillage Systems on Soil Moisture, Soil Temperature, Soil Respiration and Production of Wheat, maize and Soybean Crops. J. Food Agric. Environ. 10 (2), 445–448.
Mosier, A. R., Halvorson, A. D., Reule, C. A., and Liu, X. J. (2006). Net Global Warming Potential and Greenhouse Gas Intensity in Irrigated Cropping Systems in Northeastern Colorado. J. Environ. Qual. 35, 1584–1598. doi:10.2134/jeq2005.0232
Myhre, G., Shindell, D., Bréon, F. M., Collins, W., Fuglestvedt, J., and Huang, J. (2013). “Anthropogenic and Natural Radiative Forcing,” in Climate Change 2013: The Physical Science Basis. Contribution of Working Group I to the Fifth Assessment Report of the Intergovernmental Panel on Climate Change. Editor T. F. Stocker. (Cambridge, UK): Cambridge Univ. Press).
NDRC (2012). Second National Communication on Climate Change of the People’s Republic of China. Beijing): National Development and Reform Commission.
Pandey, A., Mai, V. T., Vu, D. Q., Bui, T. P. L., Mai, T. L. A., Jensen, L. S., et al. (2014). Organic Matter and Water Management Strategies to Reduce Methane and Nitrous Oxide Emissions from rice Paddies in Vietnam. Agric. Ecosyst. Environ. 196, 137–146. doi:10.1016/j.agee.2014.06.010
Price, S. J., Sherlock, R. R., Kelliher, F. M., McSeveny, T. M., Tate, K. R., and Condron, L. M. (2004). Pristine New Zealand forest Soil Is a strong Methane Sink. Glob. Change Biol. 10 (1), 16–26. doi:10.1046/j.1529-8817.2003.00710x
Rashid, M. H., Goswami, P. C., Hossain, M. F., Mahalder, D., Rony, M. K. I., Shirazy, B. J., et al. (2018). Mechanised Non-puddled Transplanting of Boro rice Following Mustard Conserves Resources and Enhances Productivity. Field Crops Res. 225, 83–91. doi:10.1016/j.fcr.2018.06.006
Samal, S. K., Rao, K. K., Poonia, S. P., Kumar, R., Mishra, J. S., Prakash, V., et al. (2017). Evaluation of Long-Term Conservation Agriculture and Crop Intensification in rice-wheat Rotation of Indo-Gangetic Plains of South Asia: Carbon Dynamics and Productivity. Eur. J. Agron. 90, 198–208. doi:10.1016/j.eja.2017.08.006
Sharma, P. K., and Acharya, C. L. (2000). Carry-over of Residual Soil Moisture with Mulching and Conservation Tillage Practices for Sowing of Rainfed Wheat (Triticum aestivum L.) in north-west India. Soil Tillage Res. 57, 43–52. doi:10.1016/s0167-1987(00)00141-0
Smith, P. (2007). in Agriculture in Climate Change 2007: Mitigation. Contribution of Working Group III to the Fourth Assessment Report of the Intergovernmental Panel on Climate Change. Editors B. Metz, O. R. Davidson, P. R. Bosch, R. Dave, and L. A. Meyer (Cambridge, UK): Cambridge Univ. Press).
Steinbach, H. S., and Alvarez, R. (2006). Changes in Soil Organic Carbon Contents and Nitrous Oxide Emissions after Introduction of No-Till in Pampean Agroecosystems. J. Environ. Qual. 35, 3–13. doi:10.2134/jeq2005.0050
Sun, L., Song, C., Miao, Y., Qiao, T., and Gong, C. (2013). Temporal and Spatial Variability of Methane Emissions in a Northern Temperate Marsh. Atmos. Environ. 81, 356–363. doi:10.1016/j.atmosenv.2013.09.033
Sun, R., Li, W., Dong, W., Tian, Y., Hu, C., and Liu, B. (2018). Tillage Changes Vertical Distribution of Soil Bacterial and Fungal Communities. Front. Microbiol. 9, 699. doi:10.3389/fmicb.2018.00699
Tellez-Rio, A., García-Marco, S., Navas, M., López-Solanilla, E., Rees, R. M., Tenorio, J. L., et al. (2015). Nitrous Oxide and Methane Emissions from a Vetch Cropping Season Are Changed by Long-Term Tillage Practices in a Mediterranean Agroecosystem. Biol. Fertil. Soils 51 (1), 77–88. doi:10.1007/s00374-014-0952-5
IPCC (2013). “Summary for Policymakers,” in Climate Change 2013: The Physical Science Basis. Contribution of Working Group I to the Fifth Assessment Report of the Intergovernmental Panel on Climate Change. Editors T. F. Stocker, D. Qin, G.-K. Plattner, M. Tignor, S. K. Allen, J. Boschunget al. (Cambridge, United Kingdom and New York, NY, USA: Cambridge University Press).
Tilman, D., Cassman, K. G., Matson, P. A., Naylor, R., and Polasky, S. (2002). Agricultural Sustainability and Intensive Production Practices. Nature 418, 671–677. doi:10.1038/nature01014
Uddin, S., Nitu, T. T., Milu, U. M., Nasreen, S. S., Hossenuzzaman, M., Haque, M. E., et al. (2021). Ammonia Fluxes and Emission Factors under an Intensively Managed Wetland rice Ecosystem. Environ. Sci. Process. Impacts 23 (1), 132–143. doi:10.1039/d0em00374c
van Groenigen, J. W., Velthof, G. L., Oenema, O., van Groenigen, K. J., and van Kessel, C. (2010). Towards an Agronomic Assessment of N2O Emissions: a Case Study for Arable Crops. Eur. J. Soil Sci. 61, 903–913. doi:10.1111/j.1365-2389.2009.01217.x
Vu, Q. D., de Neergaard, A., Tran, T. D., Hoang, Q. Q., Ly, P., Tran, T. M., et al. (2015). Manure, Biogas Digestate and Crop Residue Management Affects Methane Gas Emissions from rice Paddy fields on Vietnamese Smallholder Livestock Farms. Nutr. Cycl. Agroecosyst. 103, 329–346. doi:10.1007/s10705-015-9746-x
Walkley, A. (1947). A Critical Examination of a Rapid Method for Determining Organic Carbon in Soils-Effect of Variations in Digestion Conditions and of Inorganic Soil Constituents. Soil Sci. 63, 251–264. doi:10.1097/00010694-194704000-00001
Wang, M., Li, J., and Zhen, X. (1998). Methane Emission and Mechanisms of Methane Production, Oxidation, Transportation in the Rice Fields. Sci. Atmos. Sin. 22, 600–612. (In Chinese).
Wang, W., Lai, D. Y. F., Sardans, J., Wang, C., Datta, A., Pan, T., et al. (2015). Rice Straw Incorporation Affects Global Warming Potential Differently in Early vs. Late Cropping Seasons in Southeastern China. Field Crops Res. 181, 42–51. doi:10.1016/j.fcr.2015.07.007
Wassmann, R., and Aulakh, M. S. (2000). The Role of rice Plants in Regulating Mechanisms of Methane Missions. Biol. Fertil. Soils 31, 20–29. doi:10.1007/s003740050619
Weber, T., Wiseman, N. A., and Kock, A. (2019). Global Ocean Methane Emissions Dominated by Shallow Coastal Waters. Nat. Commun. 10, 4584. doi:10.1038/s41467-019-12541-7
Whalen, S. C., and Reeburgh, W. S. (1996). Moisture and Temperature Sensitivity of CH4 Oxidation in Boreal Soils. Soil Biol. Biochem. 28, 1271–1281. doi:10.1016/s0038-0717(96)00139-3
Yamulki, S., Anderson, R., Peace, A., and Morison, J. I. L. (2002). Soil CO2, CH4 and N2O Fluxes from an Afforested lowland Raised Peat Bog in Scotland: Implications for Drainage and Restoration. Biogeosci 10, 1051–1065. doi:10.5194/bg-10-1051-2013
Zaman, K., Kleineidam, K., Bakken, L., Berendt, L., Bracken, C., Butterbach-Bahl, K., et al. (2021). “Methodology for Measuring Greenhouse Gas Emissions from Agricultural Soils Using Non-isotopic Techniques,” in Measuring Emission of Agricultural Greenhouse Gases and Developing Mitigation Options Using Nuclear and Related Techniques. Editors M. Zaman, L. Heng, and C. Müller (Cham: Springer), 82–89.
Zhang, H.-L., Bai, X.-L., Xue, J.-F., Chen, Z.-D., Tang, H.-M., and Chen, F. (2013). Emissions of CH4 and N2O under Different Tillage Systems from Double-Cropped Paddy Fields in Southern China. PLoS ONE 8 (6), e65277. doi:10.1371/journal.pone.0065277
Zhao, X., Liu, S. L., Pu, C., Zhang, X. Q., Xue, J. F., Zhang, R., et al. (2015). Methane and Nitrous Oxide Emissions under No-Till Farming in China: a Meta-Analysis. Glob. Chang Biol. 22, 1372–1384. doi:10.1111/gcb.13185
Zhu, R., Liu, Y., Sun, L., and Xu, H. (2007). Methane Emissions from Two Tundra Wetlands in Eastern Antarctica. Atmos. Environ. 41, 4711–4722. doi:10.1016/j.atmosenv.2007.03.030
Keywords: emission factor, GHG emissions, GWP, N rate, residue, rice yield, tillage, yield-scaled emissions
Citation: Jahangir MMR, Bell RW, Uddin S, Ferdous J, Nasreen SS, Haque ME, Satter MA, Zaman M, Ding W, Jahiruddin M and Müller C (2022) Conservation Agriculture With Optimum Fertilizer Nitrogen Rate Reduces GWP for Rice Cultivation in Floodplain Soils. Front. Environ. Sci. 10:853655. doi: 10.3389/fenvs.2022.853655
Received: 12 January 2022; Accepted: 07 March 2022;
Published: 29 March 2022.
Edited by:
David Widory, Université du Québec à Montréal, CanadaReviewed by:
Zhenwei Song, Institute of Crop Sciences (CAAS), ChinaSangeeta Lenka, Indian Institute of Soil Science (ICAR), India
Copyright © 2022 Jahangir, Bell, Uddin, Ferdous, Nasreen, Haque, Satter, Zaman, Ding, Jahiruddin and Müller. This is an open-access article distributed under the terms of the Creative Commons Attribution License (CC BY). The use, distribution or reproduction in other forums is permitted, provided the original author(s) and the copyright owner(s) are credited and that the original publication in this journal is cited, in accordance with accepted academic practice. No use, distribution or reproduction is permitted which does not comply with these terms.
*Correspondence: M. M. R. Jahangir, bW1yamFoYW5naXJAYmF1LmVkdS5iZA==