Adsorption of Phosphate by Surface Precipitation on Lanthanum Carbonate Through In Situ Anion Substitution Reactions
- 1College of Geoscience and Surveying Engineering, China University of Mining and Technology, Beijing, China
- 2School of Material Science and Chemical Engineering, Anhui Jianzhu University, Hefei, China
- 3State Key Laboratory of Environmental Criteria and Risk Assessment, Chinese Research Academy of Environmental Sciences, Beijing, China
- 4Department of Veterinary Biomedical Sciences and Toxicology Centre, University of Saskatchewan, Saskatoon, SK, Canada
Efficient removal of phosphate (PO43−) is the main solution to control eutrophication. In this study, lanthanum carbonate (La2(CO3)3) was the adsorbent of choice for the removal of PO43−. Both adsorption isotherm and thermodynamic were investigated. La2(CO3)3 was an effective adsorbent for the removal of phosphate (PO43− or H2PO4−) under weak acidic conditions (pH = 2.0–6.0); the maximum adsorption amount was 106.6 mg g−1 at pH 2.9. The pHzpc of La2(CO3)3 changed from 2.1 to 6.5 prior to and after adsorption of PO43−. The adsorption of PO43− on the surfaces of La2(CO3)3 was an endothermic process. With the increase of anions, the adsorption efficiency of PO43- decreased. The presence of natural organic matter also inhibited the adsorption of PO43−, but the interference was weaker than that of anions. The mechanisms of adsorption were investigated by various techniques including Fourier transform infrared spectroscopy (FTIR), scanning electron microscopy (SEM), powder X-ray diffraction (XRD), and X-ray photoelectron spectroscopy (XPS). Based on the results obtained using XPS, during the adsorption process of PO43− on La2(CO3)3, La3+ was released and could precipitate with PO43− or H2PO4− under weak acidic conditions (pH = 2.0–4.0). Adsorption was dominated by in situ substitution reactions between CO32− and H2PO4− or HPO42−. This study provides a useful reference and potential material for the removal of PO43− in aquatic environments.
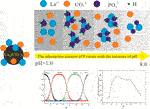
Highlights
• Lanthanum carbonate was an effective adsorbent for phosphate under weak acidic conditions, with the maximum adsorption being 106.6 mg/g at pH 2.9.
• The presence of either inorganic anions or natural organic matter would inhibit the adsorption of PO43− on La2(CO3)3.
• Because of the longer adsorption path and weaker competition in the adsorption process, the influence of natural organic matter was weaker than that of inorganic anions
• The mechanism is the combined result of physisorption and chemisorption according to the characterization, in which LaPO4 is formed when PO43− is adsorbed on the surfaces of La2(CO3)3
Introduction
Eutrophication has become one of the most pressing environmental issues that harm the quality of water. It produces undesirable color, taste, odor, and turbidity. Eutrophication reduces biodiversity, destroys aquatic habitats, and also poses significant public health risk (Wu et al., 2007; Gao et al., 2013; Su et al., 2013). Nutrient enrichment disturbs the natural ecological balance in lakes and rivers. One of the most important factors that drive the eutrophication in rivers and lakes is excess of phosphorus. Phosphate enters the environment not only through effluents of wastewater treatment plants (WWTPs) but also due to surface runoff of urban and agricultural areas. The development of a rapid and efficient method for the removal of PO43− is a highly sensitive and very interesting topic for the scientific community.
Various techniques have been developed for the removal of PO43−, including chemical precipitation (Chouyyok et al., 2010), biological processes such as harvesting biomass (Yao et al., 2011), and adsorption (Pan et al., 2014). Adsorption is a promising method because it has many advantages, including efficiency, greater speed, adaptability, easy to operate, and does not pollute the environment. In general, the adsorption, and therefore removal capacity, is directly conditioned by the physical and chemical properties of the adsorbent. In this regard, there has been a great interest in the field of environmental engineering, in advancing the development of efficient and cost-effective adsorbents. Recently, some scientific studies have reported on the removal of PO43− using different types of adsorbent, such as layered double hydroxide (Das et al., 2006; Chitrakar et al., 2010; Mandel et al., 2013), Fe–Mn binary oxide (Zhang et al., 2009), fly ashes (Chen et al., 2007), activated carbon fibers (Zhang et al., 2011; Liu et al., 2013), silica materials (Hamoudi and Belkacemi, 2013), ferrihydrite (Mallet et al., 2013), or goethite (Belelli et al., 2014). Once PO43− is adsorbed, the complex is often removed from the suspension by flocculation, facilitated either by polymeric materials (amphoteric chitosan) or by alum (Sherman et al., 2000; Agbovi and Wilson, 2018). On the other hand, flocculants such as alum can cause toxic effects, especially when it is released during flocculation. A few adsorbents can maintain a maximum adsorption capacity of PO43− under a broad range of pH, especially under acidic conditions (Lurling et al., 2014; Xie et al., 2014).
The lanthanum-based material has a great adsorption capacity and chemical stability. The lanthanum-based adsorbent contains the trivalent lanthanum ion (La3+) that has a strong affinity for PO43− even at trace levels. Once La3+ is released, it can bind with PO43− and generate an insoluble complex under acidic conditions, lanthanum-phosphate (La+3-PO43−), which is nonabsorbable (Samy et al., 2010; Yang et al., 2013). Among the new lanthanum-based adsorbents that have been developed for the removal of PO43−, NaLa(CO3)2/Fe3O4 (Hao, et al., 2019), La(OH)3 (He et al., 2015), La3+/La(OH)3 (Dong et al., 2017), and La-201 (Zhang et al., 2016) are worth noting. Using such materials, adsorption of PO43− is favored over a wide range of pH, and the adsorption mechanism involves the electrical interaction and ligand-exchange between lanthanum and PO43− (Hao et al., 2019). The main concerning of the adsorbent was related to two basic factors—how to control phosphorus in the waters and the safety of chemicals used for this processing. La2(CO3)3 was developed in recent years, and since then, its application in the pharmaceutical industry has been extensive (Persy et al., 2006). La2(CO3)3 contains La+3 and has a very strong binding capacity to PO43−. Furthermore, La2(CO3)3 does not contain aluminum or calcium and does not contaminate the environment. Despite the lanthanum-based material was considered, by many, as a potent agent for the removal of PO43− from water, few scientific articles have reported a systematic study concerning the removal of PO43− by La2(CO3)3. Therefore, the removal of phosphorus from water by La2(CO3)3 was systematically studied in this study, and it provided an effective method for the removal of PO43− in the aquatic environment.
In this study, La2(CO3)3 was synthesized and evaluated for its phosphate adsorption capacity. The effect of solution pH, adsorbent dosage, coexist inorganic ions, and natural organic matter on the removal of PO43− by La2(CO3)3 was investigated. The adsorption kinetics and isotherms were determined to compare their adsorption capacity and understand adsorption mechanisms. The X-ray photo-electron spectroscopy (XPS), X-ray diffraction (XRD), and Fourier transform infrared spectroscopy (FTIR) were used to explore the mechanisms of adsorption.
Materials and Methods
Materials and Chemicals
La2(CO3)3 was obtained from Guangfu Institute of Fine Chemicals, China. All other chemicals used (KH2PO4, Na2SiO4·9H2O, NaCl, NaNO3, Na2CO3, and Na2SO4) were of analytical grade. The measurements of pH were carried out using a PHS-3C pH-meter (Dapu instrumentation Corp., Ltd. Shanghai, China). All glassware used in experiments was carefully cleaned and rinsed with deionized water. The samples of natural organic matter (HA and FA) were collected from the soils of Jiufeng Mountain (Beijing) (Lin et al., 2011).
Batch Adsorption Experiments
La2(CO3)3 was equilibrated with a suitable amount of PO43− solution (10–100 mg L−1) using magnetic stirring for 20 h. Once the adsorbent was recovered by centrifugation, the concentration of PO43− in the supernatant was measured using the ammonium molybdate blue method. The adsorption amount of PO43− was calculated based on the difference between the balance and total amount. The influence of temperature was evaluated by setting the concentration of PO43− from 10 to 40 mg L−1 and applying a temperature which ranged from 303.15 to 323.15 K. To determine the concentration effect, the weight from 20 to 100 mg was added to 50 ml of PO43− solution (200 mg L−1), and then the suspension was agitated on a shaker for 24 h.
Interference Study
To study the influence of coexisting inorganic anions (Cl−, SO42−, NO3−, CO32−, and SiO32−), 40 mg of La2(CO3)3 was mixed with PO43− solution (100 mg L−1) and various competing ions. The effects of natural organic matter including HA and FA were also investigated, by combining 40 mg of La2(CO3)3 with 100 mg L−1 of PO43− solution and HA and FA at the concentrations of 10, 30, or 50 mg L−1. The mixture was shaken for 24 h, and the suspension was filtered through a 0.45-μm fiber membrane. The concentration of PO43− in the solution was measured using the ammonium molybdate blue method.
pHzpc Determination
The pHzpc of La2(CO3)3 was estimated according to the △pH method (Kinniburgh et al., 1975; Zhang et al., 2014), for which 50 mg of La2(CO3)3 or PO43−-saturated La2(CO3)3 was mixed with 50 ml of NaNO3 (0.01 mol L−1). These mixtures were shaken at room temperature for 20 h on an automatic shaker and then adjusted to various values of pH by additions of NaOH or HNO3. After 60 min of equilibrium, pH was measured and defined as pH(initial). Then, 1 g of NaNO3 was added to each suspension. After shaking for 1 hour, the pH(final) was measured, and the change in pH (△pH) was calculated as follows: pH(final)-pH(initial).
Characterization of the Adsorbent Before and After Adsorption
A JEOL JSM-6700F scanning electron microscope (SEM) was used to measure surface morphology. The energy dispersive spectrometer (EDS, Oxford X-MAX-20) associated with the SEM system and FTIR (Nicolet 6,700, United States) was utilized to examine chemical compounds on the surface and shape of the adsorbent before and after adsorption. Powder X-ray diffraction (XRD, Bruker D8 Advance, Germany) was also used to characterize the adsorbent. The chemical composition of La2(CO3)3 after adsorption was determined by XPS (ESCALAB250 Thermo-VG Scientific, United States). The release of CO32− and HCO3− from La2(CO3)3, during adsorption was detected by acid–base titration.
Results and Discussion
Characterization of La2(CO3)3 Prior to and After Adsorbtion of Phosphorus
The FTIR spectrums of La2(CO3)3 prior to and after adsorption of PO43− are shown in Figure 1. Compared with the spectrum of La2(CO3)3, some vibration peaks corresponding to CO32− at 1,420, 878, and 713 cm−1 almost disappeared, while other peaks at 1,054, 616, and 542 cm−1 were observed after the adsorption of PO43−. Furthermore, the peak at 1,054 cm−1 was assigned to the asymmetric stretching vibration of P-O of the PO43− group, and the peaks at 616 and 542 cm−1 were assigned to the bending vibration of O-P-O (Li et al., 2014; Wang et al., 2016), which indicated that the adsorption mechanism of PO43− on the surfaces of La2(CO3)3 included a ligand exchange process.
The typical SEM images of La2(CO3)3 prior to and after adsorption of PO43− are shown in Figure 2. La2(CO3)3 exhibited a more regular surface and better particle dispersion after the adsorption of PO43−. The adsorption of PO43− on La2(CO3)3 was confirmed by energy-dispersive spectroscopy analysis. As shown in Figure 2D, the characteristic peaks of P appeared in the spectra of La2(CO3)3 after the adsorption of PO43−, which indicated that PO43− was successfully adsorbed on the surfaces of La2(CO3)3. Meanwhile, the characteristic peaks of C decreased significantly after the adsorption of PO43− on La2(CO3)3, evidencing that CO32− was mostly replaced by PO43− on the surfaces of La2(CO3)3 (Huang et al., 2007).
The analysis of the powder X-ray diffractograms of the La2(CO3)3 prior to and after adsorption of PO43− was illustrated in Fig. S1, in which the XRD standard diffraction card was also presented. La2(CO3)3 used as an adsorbent in this study can be indexed as La2(CO3)3·8H2O (JCPS card NO.25-1,400) from the XRD patterns. The XRD pattern of La2(CO3)3 after the adsorption of PO43− was closely matched to LaPO4·0.5H2O (JCPS card NO.46-1,439). These results demonstrated that a new substance was generated after PO43− being adsorbed on the surfaces of La2(CO3)3.
Effect of Solution pH
The pH can affect not only charges on the surfaces of La2(CO3)3 but also dissociation and solubility of the adsorbent, which would influence the adsorption of PO43− on La2(CO3)3 (Yang et al., 2013). The effect of solution pH in the range of 1.0–8.0 is shown in Figure 3A. The adsorption amount increased sharply when the pH changed from 1.0 to 3.0 and then decreased slowly with an increase in pH. The maximum adsorption amount of PO43− was obtained at pH 3.0 (101.6 mg g−1), which is 15.6-fold greater than that at pH 1.1. It means that La2(CO3)3 was an efficient adsorbent for the removal of PO43− under acidic conditions. This is similar to the results of other research studies. Many researchers have studied the influence of the pH value on PO43− adsorption capacity and found that the lanthanide adsorbent has a higher PO43− removal efficiency only at a lower pH value (Lu et al., 2021). There have been many studies on the removal of PO43− by various materials, including binary and ternary compounds, for e.g., ZrO2, red-mud, La–Cu, Fe–Zr, Fe–Mn, and Fe–Al–Mn (Lǚ et al., 2013; Zhang et al., 2009; Liu et al., 2008; Huang et al., 2008; Zhao et al., 2014; Long et al., 2011). The adsorption amount of PO43− on these materials was different and significantly affected by the solution pH (Table 1). The adsorption amount of binary materials (La–Cu) and ternary materials (Fe–Al–Mn) was significantly higher than that of other materials, and the adsorption capacity of La2(CO3)3 was much greater than that of other materials. Meanwhile, the composition and synthesis method of La2(CO3)3 was simple and better when applied to removal of PO43−.
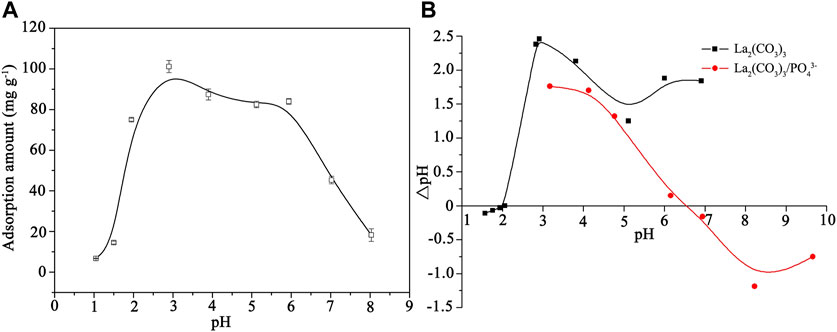
FIGURE 3. Effect of solution pH on the adsorption of PO43− on La2(CO3)3 (A). Zero-point charge (pHzpc) of La2(CO3)3 and La2(CO3)3/PO43− (B).
The isoelectric points of La2(CO3)3 prior to and after adsorption were 2.1 and 6.5, respectively (Figure 3B), indicated that PO43− neutralized the positive charge on the surface of La2(CO3)3 and caused an increase in the isoelectric point. These findings revealed that in the surface of La2(CO3)3, there was a change from carbonate to PO43− when the pH values were 2.0–6.0. The existence of PO43− species mainly in the form of H2PO4− is explained because the adsorbent gathered more positive charges on the surface under acidic conditions, which caused strong adsorption of PO43- on the surface of La2(CO3)3 by electrostatic attraction. Meanwhile, La3+ and CO32− in La2(CO3)3 were dissociated in weak acid solutions and could be replaced by H2PO4− under acidic conditions (Haghseresht et al., 2009; Xie et al., 2014). When the pH was greater than 6.0, the adsorption amount of PO43− on La2(CO3)3 continue to decrease presumably due to the competition for the adsorption sites between PO43− and other coexisting anions such as CO32− or OH−.
Effect of Adsorbent Dosage
The effect of dosage on the adsorption efficiency of PO43− is shown in Figure 4. The adsorption efficiency varied from 38.4 to 94.6% for the range of concentrations of 20–80 mg 50 ml−1, which suggested that the adsorption efficiency of PO43− by La2(CO3)3 was directly proportional to the dose of La2(CO3)3. This is because there were more adsorption sites available for PO43- as the dosage increased. When the concentration of La2(CO3)3 was higher than 80 mg 50 ml−1, it had a negligible effect on the adsorption efficiency of PO43−.
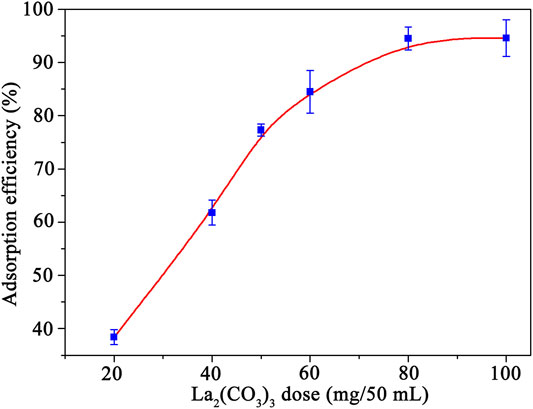
FIGURE 4. Effect of La2(CO3)3 dosage on the adsorption amount of PO43−. Initial concentration of PO43−: 200 mg L−1, temperature: 298.15 K, and pH: 6.8.
Adsorption Kinetics
Figure 5A demonstrates the effect of time on the adsorption amount of PO43− on the surfaces of La2(CO3)3. The adsorption amount of PO43− increased rapidly during the first 50 min, probably due to a fast exchange of CO32− and PO43− on the surface of La2(CO3)3 and occupancy of the sites by PO43−. After that, the adsorption amount of PO43 increased slowly over time. This implied mass transfer of CO32− and PO43− and subsequent exchange, predominant during the adsorption process. There was no significant change in the adsorption amount of PO43− on the surface of La2(CO3)3 after 24 h.
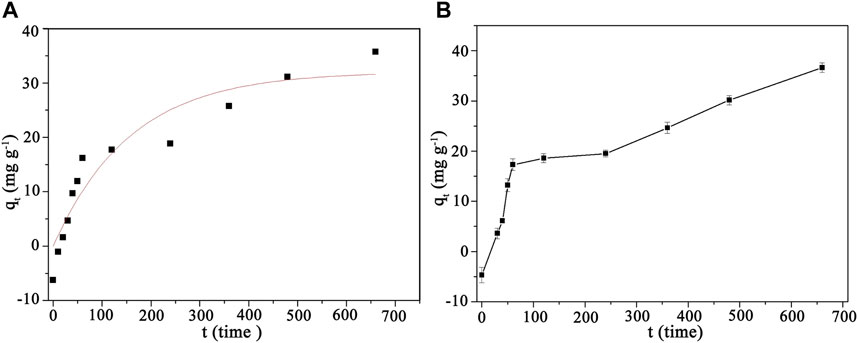
FIGURE 5. Effect of adsorption time on the adsorption amount of PO43− on La2(CO3)3 (A). Pseudo-first-order kinetic curve of adsorption of PO43− on La2(CO3)3 (B). Initial concentration of PO43−: 100 mg L−1, temperature: 298.15 K, and pH = 6.8.
A quantitative approach to determine adsorption is feasible using a kinetic model. The equation for pseudo-first-order kinetic was introduced by Lagergren (Eq. 1), which is used for the prediction of the physisorption of the adsorbate onto the adsorbent in a given system.
where qe is the amount of the adsorbate at equilibrium (mg g−1), qt is the amount of the adsorbate (mg g−1) at time t (min), and K (min−1) is the rate constant for the pseudo-first-order sorption.
This equation can also be expressed by the following alternative equation:
The kinetic curve of pseudo-first-order corresponding to the adsorption of PO43− on La2(CO3)3 is shown in Figure 5B. The qe from the nonlinear optimization was 32.08 mg g−1, the rate constant of the pseudo-first-order reaction was 6.3 × 10−3, and the correlation coefficient (r) of the formula was 0.95. These results indicated the existence of a reversible interaction between PO43− and La2(CO3)3.
Adsorption Thermodynamics
Figure 6 displays the adsorption amount of PO43− on La2(CO3)3 at 303, 313, and 323 K. The adsorption amount of PO43− increased gradually with the increase of temperature, significantly affecting the adsorption of PO43− on the surfaces of La2(CO3)3, since more carbonate ions could be dissociated from the surfaces of the adsorbent. The exchange rate of ions between PO43− and CO32− also increased, which could accelerate the adsorption reaction. The increase of the adsorption amount indicated that the adsorption of PO43− on the surfaces of La2(CO3)3 was an endothermic process (Mezenner and Bensmaili, 2009).
Effect of Coexisting Inorganic Ions
Inorganic ions such as Cl−, SO42−, NO3−, CO32−, and SiO32− are ubiquitous in environmental water. The influence of such anions on the adsorption of PO43− is shown in Figure 7A. Compared with the control, all anions had a negative effect on the adsorption efficiency of PO43−. In particular, the coexistence of CO32− and SiO32− reduced the adsorption efficiency of PO43− from 54.1 to 14.2%–9.5 and 7.6%, respectively, when the concentration of CO32− and SiO32− increased from 1.0 to 10 mmol L−1. The previous studies have also examined the effect of ions on adsorption efficiency of phosphorus of other materials, such as La-porous carbon composites (Koilraj and Sasaki, 2017). The result was consistent with this study, and the adsorption capacity was disturbed by 20 mM CO32− but not reduced in the presence 20 mM Cl− and 20 mM SO42−. To further study the mechanism involved, the change of pH was measured after adsorption in the presence of CO32− and SiO32−. The pH rose when the concentration was increased for CO32− (pH = 7.1–10.2) and SiO32− (pH = 7.7–11.9). This then entails a strong interfering effect on the adsorption of PO43− due to CO32− and SiO32−. On the one hand, the rose of pH would cause a decrease of positive charges on the surfaces of La2(CO3)3, weakening the electrostatic attraction between PO43− and La2(CO3)3. On the other hand, the increase of anions could lead to stronger competitive adsorption with PO43−, resulting in a decrease of adsorption amount of PO43−.
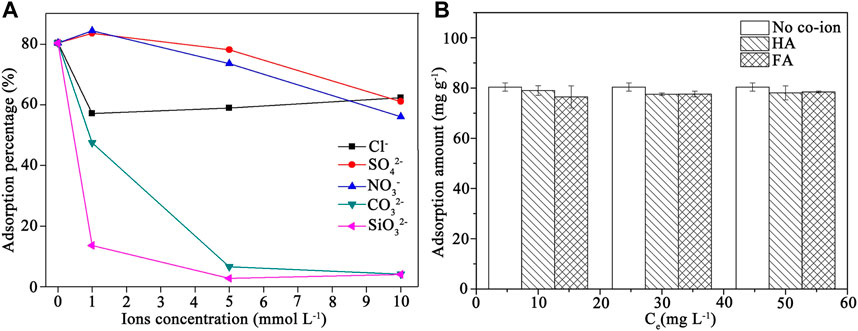
FIGURE 7. Effects of Cl−, SO42−, NO3−, CO32−, and SiO32− (A) and natural organic matter (B) on adsorption of PO43− on La2(CO3)3.
Effect of Natural Organic Matter
HA and FA are the most important components in the natural organic matter, being ubiquitous in the aquatic environment. They are complex mixtures of heterogenous compounds with a negative charge, originated from the decomposition of plant and animal residues (Valencia et al., 2012; Mcintyre and Guéguen, 2013). The existence of HA and FA in environmental waters may cause interference in the adsorption of PO43− on La2(CO3)3 through a competitive process. As shown in Figure 7B, with no coexisting ions in solution as a blank controller (no co-ion), both HA and FA had a weaker effect, as interference on the adsorption of PO43−, than that observed for the anion. This is because the molecular weight of natural organic matter was larger than that of anion and requires a longer adsorption path. HA and FA were less competitive to PO43− than inorganic anion, and FA, with a small molecular weight, had more influence on the adsorption than HA. Since HA and FA can be combined with PO43− in aqueous solution, the complex of La2(CO3)3/HA (or FA) can still be combined with PO43−, so the change in the adsorption amount of PO43− was not perceivable in the presence of HA and FA.
Analysis of the Mechanism in the Adsorption of PO43−
As shown in Figure 8, the adsorption amount of PO43− increased with the concentration of PO43−, reaching a maximum adsorption amount at 150 mg L−1. When PO43− concentration exceeded 150 mg L−1, the adsorption amount decreased. The corresponding total molar volume of bicarbonate in the solution after adsorption is also shown in Figure 8, which rose when the concentration of PO43− increased and attained equilibrium at variable concentration. In addition, the change of the adsorption amount of PO43− (the initial concentration of PO43− was 100–200 mg L−1) could be due to the coexistence of anions such as OH−. Therefore, PO43− in the solution would react with La2(CO3)3, and HCO3− would be released.
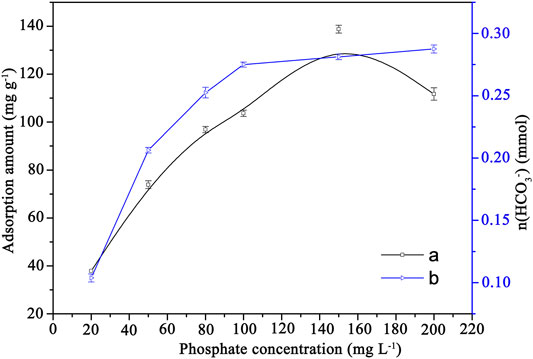
FIGURE 8. Influence of the initial concentration of PO43− on adsorption amount of PO43− (A). Total molar volume of bicarbonate in the solution after adsorption (B).
The XPS spectra of La2(CO3)3 after the adsorption of PO43− is shown in Fig. S2(a). The content of C, O, La, and P at the surface of La2(CO3)3 after adsorption were 13.7, 57.4, 18.0, and 10.8%, respectively. Fig. S2(b) and Fig. S2(c) show the fitted spectras corresponding to La3d and P2p, respectively. The peak at 835.10 eV corresponded to La3d5/2 of LaPO4 (Jorgensen et al., 2002). The binding energy of P 2p was 133.89 and 132.89 eV, which contributed to LaPO4 (Ivanova et al., 1996) and HPO42− (Kurmaev et al., 1996), respectively. The XPS spectrum indicated that the adsorption of PO43− on the surfaces of La2(CO3)3 might be the result of ion exchange between PO43− and carbonate, according to the aforementioned results. Based on a previous study, the adsorption process of PO43− on the surfaces of La2(CO3)3 could be mainly explained in terms of ion exchange (chemisorptions). A tentative adsorption mechanism is presented in Fig. S3, when HPO42− reacted with La2(CO3)3, and HCO32− was released in the solution.
Conclusion
This study has enabled the exploration of the adsorption process of PO43− on the surfaces of La2(CO3)3 and its characterization in aqueous solution. It has been evidenced that the pH had a greater impact in the adsorption of PO43− on La2(CO3)3, and the adsorbent have an excellent adsorption ability under acidic conditions (pH = 2.0–6.0). On the other hand, the presence of either inorganic anions or natural organic matter would inhibit the adsorption of PO43− on La2(CO3)3. The influences of both CO32− and Si2O32− were far higher than those of other anions. Because of the longer adsorption path and weaker competition in the adsorption process, the influence of natural organic matter was weaker than that of inorganic anions. The mechanism involved in the adsorption of PO43− on La2(CO3)3 is the combined result of physisorption and chemisorption according to the characterization, in which LaPO4 is formed when PO43− is adsorbed on the surfaces of La2(CO3)3. All these results prove that La2(CO3)3 has a large adsorption capacity and useful for the removal of PO43− from water.
Data Availability Statement
The raw data supporting the conclusion of this article will be made available by the authors, without undue reservation.
Author Contributions
SZ, ZT, and FX: conceptualization, methodology, and software. SZ and ZT: data curation and writing-original draft preparation. MF and TZ: visualization and investigation. ZT and FX: supervision. MF and TZ: software and validation. SZ, ZT, and JPG: writing-reviewing and editing.
Funding
This research was financially supported by the National Science Foundation of China (21777001, 21107001, and 42077349) and Key Research and Development Project of Anhui Province, China (202004i07020006).
Conflict of Interest
The authors declare that the research was conducted in the absence of any commercial or financial relationships that could be construed as a potential conflict of interest.
Publisher’s Note
All claims expressed in this article are solely those of the authors and do not necessarily represent those of their affiliated organizations, or those of the publisher, the editors, and the reviewers. Any product that may be evaluated in this article, or claim that may be made by its manufacturer, is not guaranteed or endorsed by the publisher.
Supplementary Material
The Supplementary Material for this article can be found online at: https://www.frontiersin.org/articles/10.3389/fenvs.2022.858258/full#supplementary-material
References
Agbovi, H. K., and Wilson, L. D. (2018). Design of Amphoteric Chitosan Flocculants for Phosphate and Turbidity Removal in Wastewater. Carbohydr. Polym. 189, 360–370. doi:10.1016/j.carbpol.2018.02.024
Belelli, P. G., Fuente, S. A., and Castellani, N. J. (2014). Phosphate Adsorption on Goethite and Al-Rich Goethite. Comput. Mater. Sci. 85, 59–66. doi:10.1016/j.commatsci.2013.12.030
Chen, J., Kong, H., Wu, D., Chen, X., Zhang, D., and Sun, Z. (2007). Phosphate Immobilization from Aqueous Solution by Fly Ashes in Relation to Their Composition. J. Hazard. Mater. 139, 293–300. doi:10.1016/j.jhazmat.2006.06.034
Chitrakar, R., Tezuka, S., Hosokawa, J., Makita, Y., Sonoda, A., Ooi, K., et al. (2010). Uptake Properties of Phosphate on a Novel Zr-Modified MgFe-LDH(CO3). J. Colloid Interf. Sci. 349, 314–320. doi:10.1016/j.jcis.2010.05.068
Chouyyok, W., Wiacek, R. J., Pattamakomsan, K., Sangvanich, T., Grudzien, R. M., Fryxell, G. E., et al. (2010). Phosphate Removal by Anion Binding on Functionalized Nanoporous Sorbents. Environ. Sci. Technol. 44, 3073–3078. doi:10.1021/es100787m
Das, J., Patra, B. S., Baliarsingh, N., and Parida, K. M. (2006). Adsorption of Phosphate by Layered Double Hydroxides in Aqueous Solutions. Appl. Clay Sci. 32, 252–260. doi:10.1016/j.clay.2006.02.005
Dong, S., Wang, Y., Zhao, Y., Zhou, X., and Zheng, H. (2017). La3+/La(OH)3 Loaded Magnetic Cationic Hydrogel Composites for Phosphate Removal: Effect of Lanthanum Species and Mechanistic Study. Water Res. 126, 433–441. doi:10.1016/j.watres.2017.09.050
Gao, Y., Chen, N., Hu, W., Feng, C., Zhang, B., Ning, Q., et al. (2013). Phosphate Removal from Aqueous Solution by an Effective clay Composite Material. J. Solution Chem. 42, 691–704. doi:10.1007/s10953-013-9985-x
Haghseresht, F., Wang, S., and Do, D. D. (2009). A Novel Lanthanum-Modified Bentonite, Phoslock, for Phosphate Removal from Wastewaters. Appl. Clay Sci. 46, 369–375. doi:10.1016/j.clay.2009.09.009
Hamoudi, S., and Belkacemi, K. (2013). Adsorption of Nitrate and Phosphate Ions from Aqueous Solutions Using Organically-Functionalized Silica Materials: Kinetic Modeling. Fuel 110, 107–113. doi:10.1016/j.fuel.2012.09.066
Hao, H., Wang, Y., and Shi, B. (2019). NaLa(CO3)2 Hybridized with Fe3O4 for Efficient Phosphate Removal: Synthesis and Adsorption Mechanistic Study. Water Res. 155, 1–11. doi:10.1016/j.watres.2019.01.049
He, J., Wang, W., Sun, F., Shi, W., Qi, D., Wang, K., et al. (2015). Highly Efficient Phosphate Scavenger Based on Well-Dispersed La(OH)3 Nanorods in Polyacrylonitrile Nanofibers for Nutrient-Starvation Antibacteria. ACS Nano 9, 9292–9302. doi:10.1021/acsnano.5b04236
Huang, X.-L., Chen, Y., and Shenker, M. (2007). Solid Phosphorus Phase in Aluminum- and Iron-Treated Biosolids. J. Environ. Qual. 36, 549–556. doi:10.2134/jeq2006.0155
Huang, W., Wang, S., Zhu, Z., Li, L., Yao, X., Rudolph, V., et al. (2008). Phosphate Removal from Wastewater Using Red Mud. J. Hazard. Mater. 158 (1), 35–42. doi:10.1016/j.jhazmat.2008.01.061
Ivanova, O., Naumkin, A., and Vasilyev, L. (1996). An XPS Study of Compositional Changes Induced by Argon Ion Bombardment of the LaPO4 Surface. Vacuum 47, 67–71. doi:10.1016/0042-207X(95)00180-8
J. J. Sherman, J. J., H. H. Van Horn, H. H., and R. A. Nordstedt, R. A. (2000). Use of Flocculants in Dairy Wastewaters to Remove Phosphorus. Appl. Eng. Agric. 16, 445–452. doi:10.13031/2013.5222
Jørgensen, S., Horst, J. A., Dyrlie, O., Larring, Y., Raeder, H., and Norby, T. (2002). XPS Surface Analyses of LaPO4ceramics Prepared by Precipitation with or without Excess of PO43−. Surf. Interf. Anal. 34, 306–310. doi:10.1002/sia.1306
Kinniburgh, D. G., Syers, J. K., and Jackson, M. L. (1975). Specific Adsorption of Trace Amounts of Calcium and Strontium by Hydrous Oxides of Iron and Aluminum. Soil Sci. Soc. America J. 39, 464–470. doi:10.2136/sssaj1975.03615995003900030027x
Koilraj, P., and Sasaki, K. (2017). Selective Removal of Phosphate Using La-Porous Carbon Composites from Aqueous Solutions: Batch and Column Studies. Chem. Eng. J. 317, 1059–1068. doi:10.1016/j.cej.2017.02.075
Kurmaev, E. Z., Fedorenko, V. V., Galakhov, V. R., Bartkowski, S., Uhlenbrock, S., Neumann, M., et al. (1996). Analysis of Oxyanion (BO 3 3? , CO 3 2? , SO 4 2? , PO 4 3? , SeO 4 4- ) Substitution in Y123 Compounds Studied by X-ray Photoelectron Spectroscopy. J. Supercond 9, 97–100. doi:10.1007/BF00728433
Li, X. L., Yang, L., Zhao, Z. C., Zhang, Z. M., Chen, L., Hu, Y., et al. (2014). Study on Comparative of Calcium Carbonate Mineral Medicines by Infrared Spectrum. Lishizhen Med. Materia Med. Res. 25, 2418–2419. doi:10.3969/j.issn.1008-0805.2014.10.044
Lin, Y., Wu, F. C., Bai, Y. C., Xie, F. Z., Cao, Z., and Su, H. L. (2011). Isolation and Characterization of Standard Fulvic Acids from Soil and Sediments in China. Res. Environ. Sci. 24, 1142–1148. doi:10.1631/jzus.A1010009
Liu, H., Sun, X., Yin, C., and Hu, C. (2008). Removal of Phosphate by Mesoporous ZrO2. J. Hazard. Mater. 151 (2), 616–622. doi:10.1016/j.jhazmat.2007.06.033
Liu, J., Zhou, Q., Chen, J., Zhang, L., and Chang, N. (2013). Phosphate Adsorption on Hydroxyl-Iron-Lanthanum Doped Activated Carbon Fiber. Chem. Eng. J. 215-216, 859–867. doi:10.1016/j.cej.2012.11.067
Long, F., Gong, J.-L., Zeng, G.-M., Chen, L., Wang, X.-Y., Deng, J.-H., et al. (2011). Removal of Phosphate from Aqueous Solution by Magnetic Fe-Zr Binary Oxide. Chem. Eng. J. 171 (2), 448–455. doi:10.1016/j.cej.2011.03.102
Lǚ, J. B., Liu, H. J., Liu, R. P., Zhao, X., Sun, L. P., and Qu, J. H. (2013). Adsorptive Removal of Phosphate by a Nanostructured Fe-Al-Mn Trimetal Oxide Adsorbent. Powder Tech. 233 (1), 146–154. doi:10.1016/j.powtec.2012.08.024
Lu, Y., Wu, H., Xia, Y., and Huang, M. (2021). Strong Adsorption of Phosphate by Amorphous Lanthanum Carbonate Nano-Adsorbents. Water Sci. Tech. 83 (8), 1605–1618. doi:10.2166/wst.2021.086
Lürling, M., Waajen, G., and van Oosterhout, F. (2014). Humic Substances Interfere with Phosphate Removal by Lanthanum Modified clay in Controlling Eutrophicationfied clay in Controlling Eutrophication. Water Res. 54, 78–88. doi:10.1016/j.watres.2014.01.059
Mallet, M., Barthélémy, K., Ruby, C., Renard, A., and Naille, S. (2013). Investigation of Phosphate Adsorption onto Ferrihydrite by X-ray Photoelectron Spectroscopy. J. Colloid Interf. Sci. 407, 95–101. doi:10.1016/j.jcis.2013.06.049
Mandel, K., Drenkova-Tuhtan, A., Hutter, F., Gellermann, C., Steinmetz, H., and Sextl, G. (2013). Layered Double Hydroxide Ion Exchangers on Superparamagnetic Microparticles for Recovery of Phosphate from Waste Water. J. Mater. Chem. A. 1, 1840–1848. doi:10.1039/C2TA00571A
Mcintyre, A. M., and Guéguen, C. (2013). Binding Interactions of Algal-Derived Dissolved Organic Matter with Metal Ions. Chemosphere 90, 620–626. doi:10.1016/j.chemosphere.2012.08.057
Mezenner, N. Y., and Bensmaili, A. (2009). Kinetics and Thermodynamic Study of Phosphate Adsorption on Iron Hydroxide-Eggshell Waste. Chem. Eng. J. 147, 87–96. doi:10.1016/j.cej.2008.06.024
Pan, B., Han, F., Nie, G., Wu, B., He, K., and Lu, L. (2014). New Strategy to Enhance Phosphate Removal from Water by Hydrous Manganese Oxide. Environ. Sci. Technol. 48, 5101–5107. doi:10.1021/es5004044
Persy, V. P., Behets, G. J., Bervoets, A. R., De Broe, M. E., and D’Haese, P. C. (2006). Lanthanum: a Safe Phosphate Binder. Semin. Dial. 19, 195–199. doi:10.1111/j.1525-139x.2006.00169.x
Samy, R., Faustino, P. J., Adams, W., Yu, L., Khan, M. A., and Yang, Y. (2010). Development and Validation of an Ion Chromatography Method for the Determination of Phosphate-Binding of Lanthanum Carbonate. J. Pharm. Biomed. Anal. 51, 1108–1112. doi:10.1016/j.jpba.2009.11.017
Su, Y., Cui, H., Li, Q., Gao, S., and Shang, J. K. (2013). Strong Adsorption of Phosphate by Amorphous Zirconium Oxide Nanoparticles. Water Res. 47, 5018–5026. doi:10.1016/j.watres.2013.05.044
Valencia, S. H., Marín, J. M., and Restrepo, G. M. (2012). Evolution of Natural Organic Matter by Size Exclusion Chromatography during Photocatalytic Degradation by Solvothermal-Synthesized Titanium Dioxide. J. Hazard. Mater. 213-214, 318–324. doi:10.1016/j.jhazmat.2012.02.003
Wang, Z., Shen, D., Shen, F., and Li, T. (2016). Phosphate Adsorption on Lanthanum Loaded Biochar. Chemosphere 150, 1–7. doi:10.1016/j.chemosphere.2016.02.004
Wu, R. S. S., Lam, K. H., Lee, J. M. N., and Lau, T. C. (2007). Removal of Phosphate from Water by a Highly Selective La(III)-chelex Resin. Chemosphere 69, 289–294. doi:10.1016/j.chemosphere.2007.04.022
Xie, J., Wang, Z., Lu, S., Wu, D., Zhang, Z., and Kong, H. (2014). Removal and Recovery of Phosphate from Water by Lanthanum Hydroxide Materials. Chem. Eng. J. 254, 163–170. doi:10.1016/j.cej.2014.05.113
Yang, Y., Shah, R. B., Yu, L. X., and Khan, M. A. (2013). In Vitro bioequivalence Approach for a Locally Acting Gastrointestinal Drug: Lanthanum Carbonate. Mol. Pharmaceutics 10, 544–550. doi:10.1021/mp300517p
Yao, Y., Gao, B., Inyang, M., Zimmerman, A. R., Cao, X., Pullammanappallil, P., et al. (2011). Removal of Phosphate from Aqueous Solution by Biochar Derived from Anaerobically Digested Sugar Beet Tailings. J. Hazard. Mater. 190, 501–507. doi:10.1016/j.jhazmat.2011.03.083
Zhang, G., Liu, H., Liu, R., and Qu, J. (2009). Removal of Phosphate from Water by a Fe-Mn Binary Oxide Adsorbent. J. Colloid Interf. Sci. 335, 168–174. doi:10.1016/j.jcis.2009.03.019
Zhang, L., Wan, L., Chang, N., Liu, J., Duan, C., Zhou, Q., et al. (2011). Removal of Phosphate from Water by Activated Carbon Fiber Loaded with Lanthanum Oxide. J. Hazard. Mater. 190, 848–855. doi:10.1016/j.jhazmat.2011.04.021
Zhang, W., Fu, J., Zhang, G., and Zhang, X. (2014). Enhanced Arsenate Removal by Novel Fe-La Composite (Hydr)oxides Synthesized via Coprecipitation. Chem. Eng. J. 251, 69–79. doi:10.1016/j.cej.2014.04.057
Zhang, Y., Pan, B., Shan, C., and Gao, X. (2016). Enhanced Phosphate Removal by Nanosized Hydrated La(III) Oxide Confined in Cross-Linked Polystyrene Networks. Environ. Sci. Technol. 50, 1447–1454. doi:10.1021/acs.est.5b04630
Keywords: lanthanum carbonate, removal, natural organic matter, anion substitution reaction, eutrophication
Citation: Zhang SY, Xie FZ, Tang Z, Zhao TH, Fang MY and Giesy JP (2022) Adsorption of Phosphate by Surface Precipitation on Lanthanum Carbonate Through In Situ Anion Substitution Reactions. Front. Environ. Sci. 10:858258. doi: 10.3389/fenvs.2022.858258
Received: 19 January 2022; Accepted: 25 February 2022;
Published: 06 April 2022.
Edited by:
Jingfu Wang, Institute of Geochemistry (CAS), ChinaReviewed by:
Kang Song, Institute of Hydrobiology (CAS), ChinaHaiquan Yang, Institute of Geochemistry (CAS), China
Copyright © 2022 Zhang, Xie, Tang, Zhao, Fang and Giesy. This is an open-access article distributed under the terms of the Creative Commons Attribution License (CC BY). The use, distribution or reproduction in other forums is permitted, provided the original author(s) and the copyright owner(s) are credited and that the original publication in this journal is cited, in accordance with accepted academic practice. No use, distribution or reproduction is permitted which does not comply with these terms.
*Correspondence: Fazhi Xie, fzxie@ahjzu.edu.cn; Zhi Tang, tzwork@hotmail.com