- 1Community School, Naples, FL, United States
- 2Everglades Research and Education Center, University of Florida, Belle Glade, FL, United States
- 3Department of Soil, Water & Ecosystem Sciences, University of Florida—Department of Soil, Water & Ecosystem Sciences, Gainesville, FL, United States
- 4Department of Soil, Water & Ecosystem Sciences, University of Florida, Immokalee, FL, United States
- 5Department of Soil, Water & Ecosystem Sciences, University of Florida, Belle Glade, FL, United States
Excess nutrient loading from agriculture and urban runoff into limnetic and marine ecosystems is associated with harmful algal blooms that result in eutrophication. Sequestration of nutrients such as phosphorus (P) and nitrogen (N) from agricultural outflows and recycling them as soil amendments would be an environmentally and economically sustainable strategy to alleviate this problem. This study explored the use of biobeads constructed with phytoplankton, Chlorella vulgaris, alginate and glomalin as a possible medium for a cyclic culture-harvest-reapply (CHR) system to address the problem of eutrophication. These “biobeads” were constructed from different concentrations of sodium alginate, C. vulgaris, and glomalin. Bead vitality was evaluated by introducing C. vulgaris to both eutrophic (phosphate ∼1.5 ppm) and hypereutrophic (phosphate ∼4.0 ppm) solutions and measuring phosphate removal. After 9 days in the eutrophic solution, biologically active groups reduced orthophosphate concentrations by an average of 1.35 ppm (80%). In the hypereutrophic solution, an average of 1.52 ppm total phosphate removal (38%) was observed over 5 weeks. The addition of glomalin in high concentrations increased the structural cohesivity of the hydrogel matrix, while low concentrations had an inverse effect. Reapplication of these biobeads to topsoil did not reduce plant growth or plant health parameters. These data suggest that glomalin, in appropriate proportions, is a suitable secondary scaffolding for a sodium alginate hydrogel immobilization medium. The alginate beads of immobilized C. vulgaris could be a promising treatment technique for phosphorus-containing urban wastewater. Further research is warranted to assess long-term impacts on nutrient dispersal and soil quality upon reapplication.
Introduction
The eutrophication of limnetic and marine ecosystems has garnered global concern because of the ramifications on ecological, economic, and human health (Sinha et al., 2019). The introduction of excess nutrients into surface water often occurs through the leaching of phosphorus (P) and nitrogen (N)-based fertilizers applied to ensure profitable crop yields, maintain recreational facilities, and grow residential lawns (Jamwal, 2017). The continued addition of N and P through agricultural and urban runoff has been associated with the formation of dead zones, toxic algal blooms, and fish kills in numerous watersheds around the world (Kirkpatrick et al., 2004). Nitrogen and P loading is not solely dependent on the surface application of these elements as fertilizers but also on the management of water flow in urban and agricultural systems. The anthropogenic propensity to shunt water rapidly away from managed lands has created a gridded series of flow-ways that act as a highway for nutrients from amended sites to surface waters. Although anthropogenic influences on hydrology in these systems are associated with eutrophication (Chuai et al., 2012), the inherent integration of man-made convergence points from drainage systems are also ideal sites for nutrient filtration. Use of photosynthetic organisms to metabolically remove metals, toxins, and a host of other chemicals is well documented (Bhat et al., 2022). However, filtration of nutrients for disposal lacks cyclic sustainability. A sustainable strategy would be the use of phyto-filtration units to remove nutrients from water and redistribute them into the soil. This could reduce eutrophication and promote sustainable agriculture.
Using algae to bioremediate excess nutrients from urban and agricultural effluents is an established and efficacious practice (Delrue et al., 2016). Some algae utilize the nutrients as part of their metabolic processes, accumulating biomass and creating a biological “micro-sink” for carbon, P, N, and micronutrients. Algal cultures are relatively inexpensive, easy to maintain, and can be harvested for a multitude of uses including biofuel generation, biochar production, and reapplication as a soil amendment (Vidyashankar and Ravishankar, 2016). Although these characteristics make algae a prime remediator, harvesting planktonic cells or desorbing them from biofilter substrates can be complicated and add an expense to an otherwise economically feasible system. A truly efficacious and sustainable biofiltration system must effectively remediate nutrients from eutrophic effluent, keep nutrient-loaded microbes at the point source until harvesting, remain harvestable in a form suitable for immediate reapplication to the soil, and be cost-effective.
To meet the above parameters, immobilizing bio-remedial microbes within a hydrogel could be an option (Hu et al., 2020). Hydrogels consist of three-dimensional polymeric networks characterized by a large ratio of hydrophilic functional groups which form flexible and fluid associations within the polymer (Khan and Lo, 2016). Exposing the hydrogel fluid to ion solutions causes an electrostatic repulsion, inducing the formation of linkages between the gel molecules at the gel/solution interface and causing spherization of the gel within the ion solution. The resulting gel-sphere is water permeable yet maintains its structural integrity (Ching et al., 2017). Hydrogels become biologically active once an organism, a consortium of organisms, or functional biomolecules are immobilized within the matrix. Although the gel “beads” are relatively stable, they tend to lose cohesion over time. However, combining a hydrogel with a stabilizing agent and a bio-remediating algae could result in a stable system to both capture and harvest excess nutrients.
Chlorella vulgaris is a unicellular, planktonic algae used in a wide range of remediation applications from surface water treatment to the remediation of contaminated groundwater (Delrue et al., 2016). Salgueiro et al. (2016) reported C. vulgaris reduced phosphate concentrations by up to 99%. Furthermore, C. vulgaris has been successfully used for bioremediation of different wastewater sources due to its robustness, mixotrophic culturing conditions, high growth rate under harsh conditions, and tolerance to high levels of heavy metals (Ge et al., 2013). However, work by Kube et al. (2020) suggests that certain environmental conditions may play an inhibitory role in nutrient accumulation. Further, it is possible the immobilization medium could be destabilized in some aquatic conditions (Mohseni et al., 2021). The vitality of C. vulgaris, indicated by accumulated biomass, is directly correlated with the rate of orthophosphate and total phosphate removal from a nutrient loaded solution (Znad et al., 2018). This suggests that if the hydrogel beads were biologically activated with C. vulgaris, the resulting “biobeads” should remediate P from the solution, as C. vulgaris will incorporate the P into its biomass which would be stable within the biobead matrix.
Remediation of excess nutrients in aquatic systems is expensive yet necessary for ecosystem health (Nakarmi et al., 2022). Using materials that can synergistically utilize the phytoremedial properties of known hyperaccumulators to create a technique to remove nutrients that can be reapplied as fertilizer would be more cost effective. Current water management systems of storm drains with point source outflows are inherently primed for the application of filtration methods. In addition, increasing the stability of immobilized algae filtration mediums could lead to applications in a variety of industries including wastewater treatment (Mohseni et al., 2021).
Therefore, the objectives of this study were to: 1) determine the scaffolding effect of a stabilizing agent to maintain the strength and durability of the hydrogel matrix by measuring the amount of pressure hydrogel beads withstand before the structural collapse; 2) determine the ability of the hydrogel medium to maintain viable C. vulgaris cultures within a eutrophic solution by measuring the decline in P concentrations due to metabolic activity and bioaccumulation; and 3) observe the impact of reapplying the P-enriched hydrogel beads to the soil by measuring seed germination, plant height, and dry biomass in a growth chamber.
Methods
Experimental overview
Sodium alginate was selected as a hydrogel medium because it is biologically non-reactive, non-toxic, sustainably sourced, and inexpensive (Tummino et al., 2020). Glomalin, a mixture of proteins and polysaccharides referred to as GRSFs (glomalin-related soil fractions), was selected as a potential stabilizing agent. It is exuded by arbuscular mycorrhizal fungi and is associated with soil aggregate formation enhancing the stability of soil structure (Rillig, 2004). In-vitro experiments were performed to test the impact of added glomalin on the cohesivity of the generated immobilization beads (termed biobeads), the total remediation of orthophosphate (1.5 ppm PO43-) and total phosphate (4 ppm PO43-), and crop health at a BSL-1 laboratory (Canterbury School, Fort Myers, FL). Biobeads were constructed from a colloidal gel suspension consisting of a 2% sodium alginate solution complex, different concentrations of glomalin, and a culture of C. vulgaris.
Preparation of beads
Glomalin was extracted from unamended garden soil covered with a thick layer of accumulated leaf litter. Such an environment is conducive for high glomalin production. A soil solution of 1.2 g soil in 10 ml of 50 mM sodium citrate was prepared following the methods of Wright and Upadhyaya (1996) to extract glomalin from the soil. The solution was autoclaved for 75 min at 121°C then centrifuged at 3500 RPM for 15 min. The supernatant was extracted, autoclaved, and centrifuged eight times until the solution changed from opaque to transparent brown. Ten samples were simultaneously processed at each iteration to generate 50 ml of glomalin solution. The C. vulgaris live culture (Suncoast Marine Aquaculture) was agitated to homogenize the C. vulgaris sample before extraction. A diluted solution of food-grade sodium alginate (The Seaweed Solution) was prepared by adding 5 ml of alginate to 100 ml distilled water. The solution was stirred slowly for 30 min, to avoid gas bubbles that destabilize the final structure. The solution was stored for 24 h at 4°C. Secondary solutions were made for the inoculated beads in 20 ml batches. Six groups were determined based on a range of proportional concentrations of sodium alginate, glomalin, and Chlorella vulgaris: 100A-0G-0C, 50A-0G-50C, 50A-10G-40C, 50A-25G-25C, 50A-40G-10C 0A-0G-0C (Table 1).
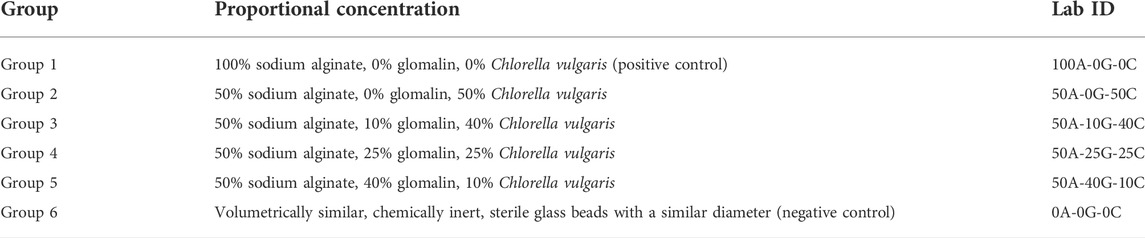
TABLE 1. Six treatment groups with proportional concentration of sodium alginate, glomalin, and Chlorella vulgaris.
Approximately 0.2 ml of the secondary solution was added dropwise to 3% CaCl2 solution kept on ice to induce spherization. The generated beads averaged between 4 and 6 mm. Beads were left in the CaCl2 solution for 30 min to mature, drained from the CaCl2 solution using a nylon mesh, rinsed with distilled water, counted, and placed in the nutrient solution (Gonzalez and Bashan, 2000). During the spherization process, bead formation results from both intra-molecular interactions (between the polysaccharide and organic acid matrix) and the sodium/cation exchange generated in the solution. The resulting bead is temporarily insoluble yet permeable in water and can be inoculated with a variety of microorganisms or enzymes that maintain their biological functionality within the medium (Aydelotte et al., 1998; Jin et al., 2011). Although the alginate beads are water insoluble, they are pH and cation sensitive and can lose their cohesivity over time (Velings and Mestdagh, 1995). The impact of the glomalin/alginate matrix on the vitality of C. vulgaris was assessed in eutrophic concentrations (∼1.5 ppm PO43-) and hypereutrophic concentrations (∼4 ppm PO43-) to determine if excessive eutrophication would destabilize the biobeads or inhibit the functionality of C. vulgaris in the remediation of phosphate. The bioavailable form of P, orthophosphate was measured under both conditions to determine the viability of the system. Total P was measured in the second set of experiments to ensure that the drop in orthophosphate concentration was associated with metabolic uptake, not P transformations.
Determining eutrophic orthophosphate uptake and bead vitality
A P solution (Carolina Biological) was diluted with distilled water at 10 ml L−1. Twenty-five biobeads were added into 200 ml of solution per flask: 100A-0G-0C, 50A-0G-50C, 50A-10G-40C, 50A-25G-25C, 50A-40G-10C 0A-0G-0C. A low range phosphate colorimeter (Hanna Instruments HI 713–0; 0.01 ppm resolution and+/−0.03 ppm accuracy) was used to determine the concentration of orthophosphate in the nutrient solution. The colorimeter was used in conjunction with pre-packaged reagents in accordance with the 4500-PE ascorbic acid method which can determine a range in orthophosphate concentrations from 0.1 to 6 ppm. 10 ml water samples were collected every 3 days after start of the experiment up to 9 days (3 DAT, 6 DAT, and 9 DAT).
Determining orthophosphate hypereutrophic uptake, total P and bead vitality
A hypereutrophic P solution was used to determine whether an increase in P destabilize the biobeads or if the system would be inhibited by P toxicity. A 1000 ppm TP solution (Fischer Scientific) was diluted to ∼4 ppm. 400 ml of nutrient solution was added to thirty 500 ml beakers designated as: 100A-0G-0C, 50A-0G-50C, 50A-10G-40C, 50A-25G-25C, 50A-40G-10C, and 0A-0G-0C. Fifty beads were added to each beaker and two 10 ml samples were extracted every 7 days for 5 weeks. One 10 ml sample was analyzed immediately for orthophosphate using the heteropoly molybdenum blue method (Holman, 1943) with a high range phosphate colorimeter (Hannah Instruments HI 717; 0.1 ppm resolution and accuracy of+/-1 ppm). The second 10 ml sample was stored at 4°C for analysis. Total P was analyzed using an inductively coupled plasma-optical emission spectrometer (5110 ICP-OES, Agilent Technologies Inc, CA) (U.S. EPA. 1994) at the University of Florida Soil, Water, and Nutrient Management Laboratory in Belle Glade, FL.
Cohesivity analysis
Throughout both experiments, the internal cohesivity of the beads was assessed by determining the pressure the bead could withstand before complete structural collapse using a penetrometer. Every 7 days, three beads were removed from the system and placed within the wells of a microwell plate. The pressure necessary to structurally destabilize the biobeads was measured by a penetrometer in kg cm−2 (105 Pa). A penetrometer (sclerometer, model GY-1), with a range of 2–15 kg cm−2 (x 105 Pa) and a resolution of+/-0.01, was used to determine the force necessary to crush the beads in kg cm−2 (105 Pa).
Plant growth experiment
Germination, plant growth, leaf number, and dry biomass were assessed to determine if the application of the beads impacted crop growth. The P loaded biobeads were applied to the surface of sterile, hydrated soil pods approximately 4 cm in diameter and 6 cm high (Barefoot Farmer organic farm, TN) and planted with Brassica rapa (subspecies Kosaitai raab) seeds. A moisture wick was run through the pods which were then rehydrated using DI water. Three B. rapa seeds were placed in each soil pod. Five beads from each concentration group were placed in 10 pods and 10 pods received no beads as a control. The soil was mixed with a scoopula to 1 cm depth with the beads intact. The pods were separated into five hydration trays and distributed in the trays in a randomized block design. The hydration trays were placed in a plant growth cart equipped with 2 grand lux tubes and 2 incandescent grow bulbs (60 W) adjusted for a 10 h photoperiod (Carolina Biological, NC). Seed germination was assessed after 10 days. Growth from the soil surface to the base of the longest terminal leaf was measured at 10 and 34 days. At 34 days, the stems were cut at the surface of the soil and the number of leaves was counted per plant. The plants were grouped according to treatment, placed on a drying surface, and dried in an oven for 4 days at 40°C. Dry biomass was measured for each group.
Statistical analyses
The effects of added glomalin and incubation time on the cohesivity of biobeads, the total remediation of orthophosphate and total phosphate, and crop health was analyzed using randomized complete design analysis with the generalized linear model (GLIMMIX) procedure in SAS (Version 9.4; SAS Institute Inc. Cary, NC). The treatment × date effect was significant; thus, data were analyzed separately for each date. Differences between treatments at each incubation time were further evaluated for significance using the Fisher LSD post hoc test. All tests were considered significant at p < 0.05.
Results and discussion
Low range orthophosphate—eutrophic solution
An initial experiment to determine if the glomalin alginate matrix would inhibit the bioremedial abilities of C. vulgaris was conducted using a phosphate solution of 1.68 ppm. Both time and treatment had a highly significant effect on the reduction of orthophosphate from the solution (Table 2). The positive control group (alginate-only beads) significantly lowered orthophosphate concentrations compared to the negative control group (glass beads) at 6 DAT and 9 DAT (Table 3). However, there was no difference between two control groups at 3 DAT. At 3 DAT, each glomalin/Chlorella bead had lower mean concentrations of orthophosphate compared to the alginate-only beads and glass beads in each measured date except group containing 50% sodium alginate, 0% glomalin, 50% C. vulgaris. All alginate bead types reduced orthophosphate concentration over time. At 9 DAT, alginate-only beads extracted 43% of the orthophosphate from the system. Alginate beads with glomalin and Chlorella reduced the orthophosphate concentration more efficiently than alginate-only beads and no-alginate beads. The most significant drop in orthophosphate was observed in the 50A-10G-40C groups which had an average concentration reduction from 1.68 to 0.17 ppm, or 90%, over the 9 days of observation. Significant lower orthophosphate concentration in positive control group compared to negative control group at 6 DAT and 9 DAT suggests that the alginate bead itself absorbs the solution and sequesters orthophosphate even in the absence of a bioremedial organisms. A lower mean concentration of orthophosphate in the solution between each of the glomalin/C. vulgaris beads compared to the alginate-only beads and no-alginate beads indicates that the beads with C. vulgaris had additional effects in the remediation of orthophosphate. Lau et al. (2010) also reported alginate immobilized C. vulgaris had higher nutrient removal efficiency from wastewater than free cells.
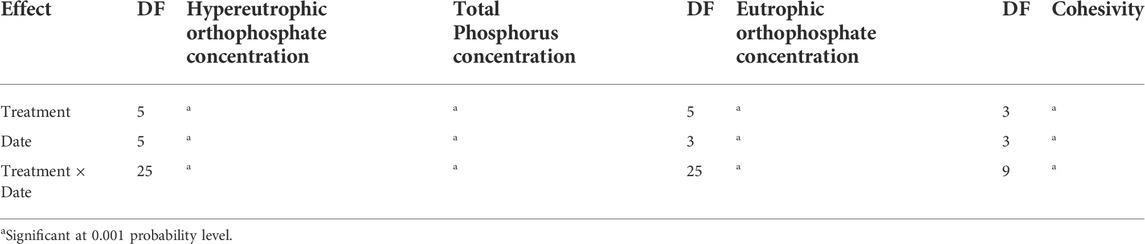
TABLE 2. Analysis of variance for the effect of treatment and time on hypereutrophic phosphate concentration, total phosphate concentration and eutrophic phosphate concentration and cohesivity.
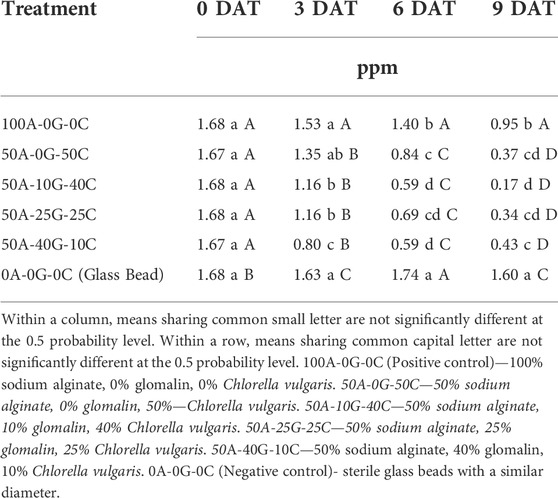
TABLE 3. Effect of treatment group on orthophosphate concentration at eutrophic solution at 0, 3, 6, and 9 days after treatment (DAT).
High range orthophosphate—hypereutrophic solution
The change in orthophosphate concentration was analyzed over time to determine if the removal of orthophosphate was constant or if an assimilation lag would be observed. No significant change in orthophosphate concentration was observed for negative control over time. No significant change in orthophosphate concentration was observed for all alginate beads (Table 4) up to 9 DAT. However, there was a significant reduction in orthophosphate concentration at 30 and 35 DAT for all alginate beads groups compared to the initial concentration. The most significant drop in orthophosphate was observed in the groups containing 50% sodium alginate, 25% glomalin, 25% C. vulgaris which had an average concentration reduction of 51% over the 35 days of observation. At 0 DAT, a significant difference in orthophosphate concentration was observed between treatments. At 9, 16, 23, 30, and 35 DAT, the negative control group had higher orthophosphate concentrations than positive control group and rest of the alginate group with glomalin/C. vulgaris except positive control, group containing 50A-10G-40C, and group containing 50A-40G-10C at 23 DAT (Table 4). No significant difference was measured in the mean reduction of orthophosphate between most of the biologically active groups, reinforcing the finding that the addition of glomalin did not inhibit the bioremedial ability of C. vulgaris. There was no significant difference between the mean orthophosphate concentrations up to 9 DAT, but there was a significant reduction at 30 and 35 DAT, indicating higher levels of eutrophication may increase the time needed for microorganisms to digest the P, thus causing a lag in nutrient remediation. Rasoul-Amini et al. (2014) investigated the influence of three microalgae strains (Chlamydomonas sp, Chlorella sp, and Oocystis sp.) on N and phosphate removal and found all microalgal strains achieved their best removal efficacies for PO43- at 14 DAT, though that was also the last day of testing for the study. Therefore, a longer culture time appears to increase microalgae nutrient absorption. The lack of significant differences in the mean reduction of orthophosphate between most of the biologically active groups indicates the addition of glomalin did not inhibit the bioremedial ability of C. vulgaris.
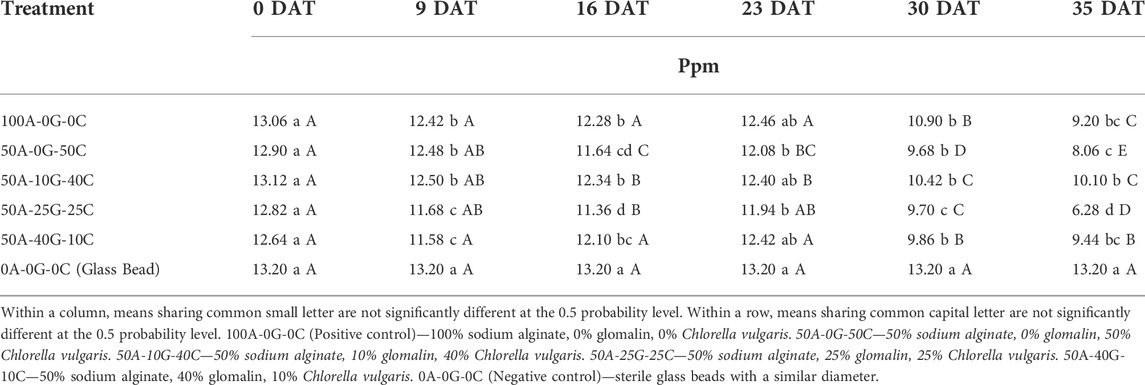
TABLE 4. Effect of treatment group on orthophosphate concentration at hypereutrophic solution at 0, 9, 16, 23, 30, and 35 days after treatment (DAT).
Total phosphorus—hypereutrophic solution
A significant reduction in the TP concentrations was measured between sampling dates (0 DAT to 35 DAT) except for negative control (Table 5). There were no significant differences in TP concentration between the glomalin/Chlorella beads and the positive control except group containing 50A-25G-25C at 16 DAT, group containing 50A-25G-25C at 23 DAT, and group containing 50A-0G-50C at 30 DAT. Although the binding of phosphate in the positive control was observed, the drop in TP in the solution was not statistically significant between the positive and negative control except at 9 DAT and 30 DAT. The glomalin/C. vulgaris groups, regardless of glomalin concentration, significantly reduced the TP in the solution compared to the negative control. AT 30 DAT, the negative control had significantly higher TP compared to other alginate groups. No significant differences in TP concentration between the glomalin/C. vulgaris groups and the positive control confirms that the sodium alginate matrix is capable of absorbing phosphate without the introduction of bio-remedial organisms. Mohseni et al. (2021) reported alginate beads of C. vulgaris were sufficiently stable and were capable of remediation of TP up to 100% over a 10-day period. The lack of significant variance between the means of the biologically active groups indicates that the introduction of glomalin did not inhibit the ability of C. vulgaris to remove P from the solution. In addition, although a greater loss of TP was observed in biologically active groups, this difference was not statistically significant.
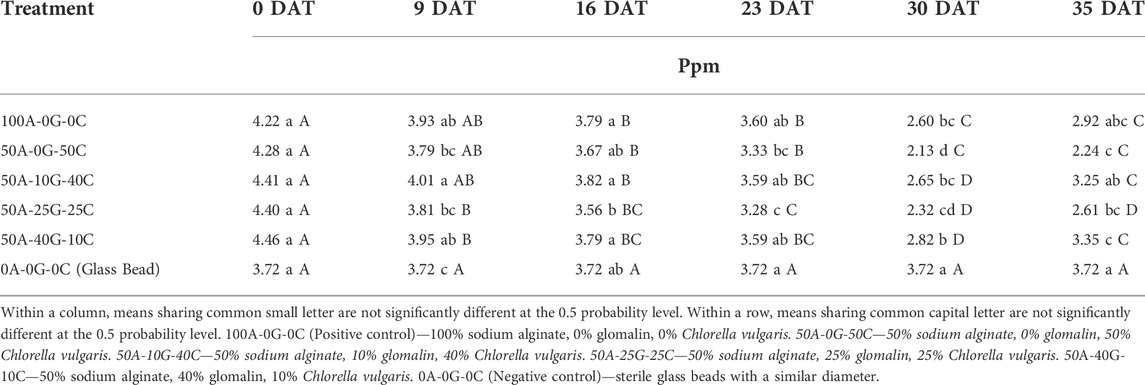
TABLE 5. Effect of treatment group on total phosphorus concentration at 0, 9, 16, 23, 30, and 35 days after treatment (DAT).
Cohesivity experiment
All glomalin/C. vulgaris groups displayed increased structural cohesivity over the experimental period. In all groups, there was a significant increase in the bead resilience to pressure over time (Table 6). A significant increase in cohesivity was observed over the first 14 days but not between days 14–28 for positive control group and groups containing 50% sodium alginate, 40% glomalin, 10% C. vulgaris whereas groups containing 50% sodium alginate, 10% glomalin, 40% C. vulgaris and 50% sodium alginate, 25% glomalin, 25% C. vulgaris no significant difference in cohesivity was observed over the first 14 days (Table 6). The group with the greatest relative concentration of glomalin exhibited a statistically significant increase (208%) in cohesive stability. However, while relationship between glomalin concentration and structural integrity was linear, the relationship between glomalin concentration and biobead strength was parabolic. This parabolic relationship between glomalin concentration and biobead strength suggests that an increase in glomalin may initially inhibit the cross-linkages that increase gelation and cohesivity. However, at higher concentrations interaction with the glomalin may increase intra-bead molecular interactions, increasing structural strength. The lack of significant differences in cohesivity over the first 14 days suggests there is a maturation period of 2 weeks during which the alginate beads continue to experience gelation processes.
Growth experiment
Seed germination was assessed by counting the number of sprouted seeds at day 10. Although on average there was a greater percentage of seed germination between the groups containing glomalin and Chlorella, there was no significant difference in germination between treatments (Figure 1). There was no significant difference in growth at 34 days or biomass between the treatment groups (Figure 2, Figure 3). The leaf number was significantly higher in the high concentration glomalin groups (40% glomalin and 25% glomalin) than in the low concentration group (10% glomalin) and controls. The lack of significant differences between treatment groups for the seed germination, biomass, and plant growth suggests the application of the biobeads did not confer a growth advantage to the B. rapa seeds. However, the higher leaf numbers in the high concentration glomalin groups (40% glomalin and 25% glomalin) compared to the low concentration group (10% glomalin) and the control groups suggests increased concentrations of glomalin related soil fractions (GRSFs) may improve leaf growth.
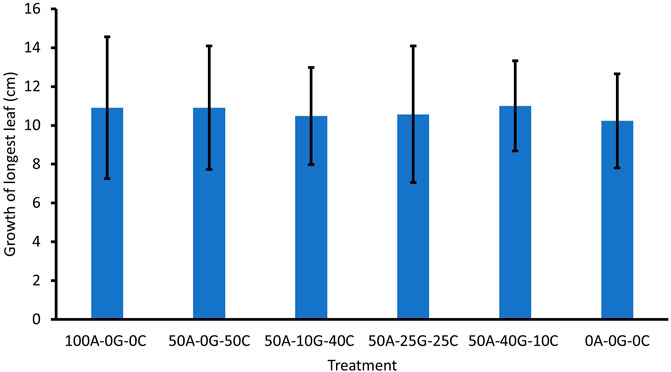
FIGURE 1. Effect of treatment group on mean seed germination. Error bars correspond to standard deviation. 100A-0G-0C (Positive control)—100% sodium alginate, 0% glomalin, 0% Chlorella vulgaris 50A-0G-50C—50% sodium alginate, 0% glomalin, 50% Chlorella vulgaris 50A-10G-40C—50% sodium alginate, 10% glomalin, 40% Chlorella vulgaris 50A-25G-25C—50% sodium alginate, 25% glomalin, 25% Chlorella vulgaris 50A-40G-10C—50% sodium alginate, 40% glomalin, 10% Chlorella vulgaris 0A-0G-0C (Negative control)- sterile glass beads with a similar diameter.
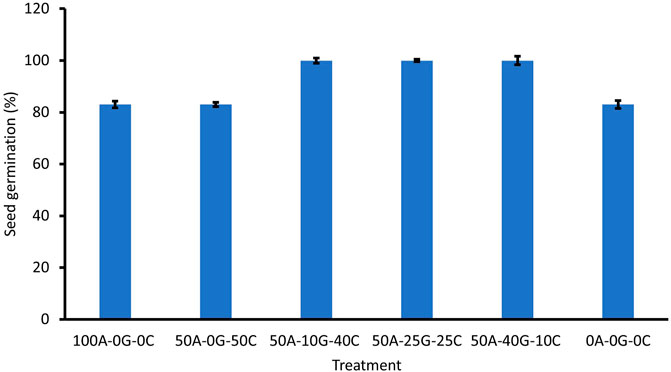
FIGURE 2. Effect of treatment group on growth at 34 days. Error bars correspond to standard deviation. 100A-0G-0C (Positive control)—100% sodium alginate, 0% glomalin, 0% Chlorella vulgaris 50A-0G-50C—50% sodium alginate, 0% glomalin, 50% Chlorella vulgaris 50A-10G-40C—50% sodium alginate, 10% glomalin, 40% Chlorella vulgaris 50A-25G-25C—50% sodium alginate, 25% glomalin, 25% Chlorella vulgaris 50A-40G-10C—50% sodium alginate, 40% glomalin, 10% Chlorella vulgaris 0A-0G-0C (Negative control)- sterile glass beads with a similar diameter.
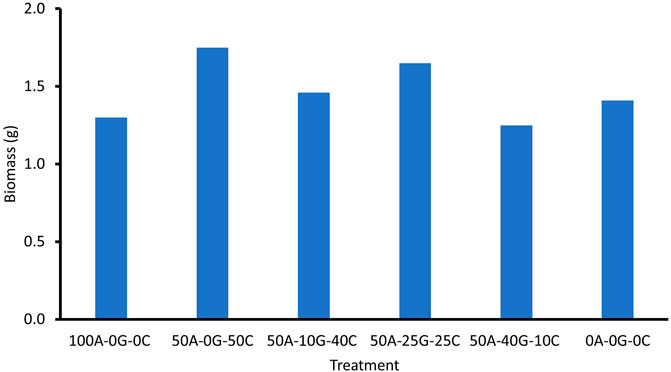
FIGURE 3. Effect of treatment group on biomass. 100A-0G-0C (Positive control)—100% sodium alginate, 0% glomalin, 0% Chlorella vulgaris 50A-0G-50C—50% sodium alginate, 0% glomalin, 50% Chlorella vulgaris 50A-10G-40C—50% sodium alginate, 10% glomalin, 40% Chlorella vulgaris 50A-25G-25C—50% sodium alginate, 25% glomalin, 25% Chlorella vulgaris 50A-40G-10C—50% sodium alginate, 40% glomalin, 10% Chlorella vulgaris 0A-0G-0C (Negative control)- sterile glass beads with a similar diameter.
Conclusion
This study demonstrates that a sodium alginate matrix can sequester phosphate even without the inclusion of C. vulgaris active groups. Immobilized C. vulgaris also showed a substantial potential for nutrient remediation in a short period of time. Overall, the sodium alginate/glomalin matrix with immobilized C. vulgaris was stable and capable of P remediation. However, the stability and nutrient removal of these biobeads could be significantly affected by environmental factors such as pH, salinity, temperature, and light. High concentrations of glomalin-related soil fractions helped stabilize the medium and did not impact the vitality of the C. vulgaris culture. Further explorations of the ability of the biobeads to redistribute nutrients post-application are warranted to determine if these biofiltration units are truly capable of being integrated into a sustainably cyclic culture-harvest-reapply (CHR) system. To employ microalgae in wastewater treatment, several limitations must first be addressed, including the creation of wastewater adapting microalgal species, as well as the enhancement of cultivation systems that will allow for improved algal biomass growth, harvesting, and conversion. Future research should focus on the development of pollutant specific hydrogels so that target pollutants can be selectively removed. To be economically practical and sustainable, a hydrogel must be durable and reusable in water and wastewater treatment, as well as having superior adsorption and separation properties.
Data Availability Statement
The raw data supporting the conclusions of this article will be made available by the authors, without undue reservation.
Author Contributions
KP: Conceptualization, Data curator, Formal analysis, Investigation, Methodology, Writing-original draft preparation, Visualization. NRA: Writing—reviewing and editing. KI: Supervision, Writing–review and editing. SLS: Supervision, Writing—review and editing. JHB: Conceptualization, Funding acquisition, Methodology, Project administration, Resources, Supervision, Writing—review and editing.
Funding
The study was provided by the USDA Hatch Funds FLAERC-005552, ‘Soil, Water, and Nutrient Management: A Systems Approach to Sustainable Agriculture in South Florida’
Conflict of Interest
The authors declare that the research was conducted in the absence of any commercial or financial relationships that could be construed as a potential conflict of interest.
Publisher’s Note
All claims expressed in this article are solely those of the authors and do not necessarily represent those of their affiliated organizations, or those of the publisher, the editors and the reviewers. Any product that may be evaluated in this article, or claim that may be made by its manufacturer, is not guaranteed or endorsed by the publisher.
Acknowledgments
The study was inspired by NSF-STEPS Center CBET-2019435. The authors would like to thank Abul Rabbany in the Soil, Water, and Nutrient Management Laboratory for assisting with phosphorus analyses.
Supplementary Material
The Supplementary Material for this article can be found online at: https://www.frontiersin.org/articles/10.3389/fenvs.2022.889940/full#supplementary-material
Abbreviation
N, nitrogen; P, phosphorous; PO43-, phosphate; CHR, culture harvest reapply; GRSF, glomalin related soil fractions; BSL 1, biosafety level 1; RPM, repetitions per minute; TP, total phosphorous; TN, total nitrogen.
References
Aydelotte, M. B., Thonar, E. J., Mollenhauer, J., and Flechtenmacher, J. (1998). Culture of chondrocytes in alginate gel: Variations in conditions of gelation influence the structure of the alginate gel, and the arrangement and morphology of proliferating chondrocytes. Vitro Cell. Dev. Biol. -Animal. 34 (2), 123–130. doi:10.1007/s11626-998-0094-x
Bhat, S. A., Bashir, O., Haq, S. A. U., Amin, T., Rafiq, A., Ali, M., et al. (2022). Phytoremediation of heavy metals in soil and water: An eco-friendly, sustainable and multidisciplinary approach. Chemosphere 303, 134788. doi:10.1016/j.chemosphere.2022.134788
Ching, S. H., Bansal, N., and Bhandari, B. (2017). Alginate gel particles–A review of production techniques and physical properties. Crit. Rev. food Sci. Nutr. 57 (6), 1133–1152. doi:10.1080/10408398.2014.965773
Chuai, X. M., Chen, X., Yang, L., Zeng, J., Miao, A., Zhao, H., et al. (2012). Effects of climatic changes and anthropogenic activities on lake eutrophication in different ecoregions. Int. J. Environ. Sci. Technol. (Tehran). 9 (3), 503–514. doi:10.1007/s13762-012-0066-2
Delrue, F., Álvarez-Díaz, P., Fon-Sing, S., Fleury, G., and Sassi, J. F. (2016). The environmental biorefinery: Using microalgae to remediate wastewater, a win-win paradigm. Energies 9 (3), 132. doi:10.3390/en9030132
Ge, Z., Zhang, H., Zhang, Y., Yan, C., and Zhao, Y. (2013). Purifying synthetic high-strength wastewater by microalgae chlorella vulgaris under various light emitting diode wavelengths and intensities. J. Environ. Health Sci. Eng. 11 (1), 8. doi:10.1186/2052-336X-11-8
Gonzalez, L. E., and Bashan, Y. (2000). Increased growth of the microalga Chlorella vulgaris when coimmobilized and cocultured in alginate beads with the plant-growth-promoting bacterium Azospirillum brasilense. Appl. Environ. Microbiol. 66 (4), 1527–1531. doi:10.1128/aem.66.4.1527-1531.2000
Holman, W. I. (1943). A new technique for the determination of phosphorus by the molybdenum blue method. Biochem. J. 37 (2), 256–259. doi:10.1042/bj0370256
Hu, J., Liu, H., Shukla, P., Lin, W., and Luo, J. (2020). Nitrogen and phosphorus removals by the agar-immobilized Chlorella sacchrarophila with long-term preservation at room temperature. Chemosphere 251, 126406. doi:10.1016/j.chemosphere.2020.126406
Jamwal, P. (2017). Fate of nutrients in human dominated ecosystems. Reson. 22 (3), 279–290. doi:10.1007/s12045-017-0460-7
Jin, J., Yang, L., Chan, S. M. N., Luan, T., Li, Y., Tam, N. F. Y., et al. (2011). Effect of nutrients on the biodegradation of tributyltin (TBT) by alginate immobilized microalga, Chlorella vulgaris, in natural river water. J. Hazard. Mat. 185 (2-3), 1582–1586. doi:10.1016/j.jhazmat.2010.09.075
Khan, M., and Lo, I. M. C. (2016). A holistic review of hydrogel applications in the adsorptive removal of aqueous pollutants: Recent progress, challenges, and perspectives. Water Res. 106, 259–271. doi:10.1016/j.watres.2016.10.008
Kirkpatrick, B., Fleming, L. E., Squicciarini, D., Backer, L. C., Clark, R., Abraham, W., et al. (2004). Literature review of Florida red tide: Implications for human health effects. Harmful algae. 3 (2), 99–115. doi:10.1016/j.hal.2003.08.005
Kube, M., Spedding, B., Gao, L., Fan, L., and Roddick, F. (2020). Nutrient removal by alginate‐immobilized Chlorella vulgaris : Response to different wastewater matrices. J. Chem. Technol. Biotechnol. 95 (6), 1790–1799. doi:10.1002/jctb.6377
Lau, P. S., Tam, N. F. Y., and Wong, Y. S. (2010). Wastewater nutrients (N and P) removal by carrageenan and alginate immobilized Chlorella vulgaris. Environ. Technol. 18 (9), 945–951. doi:10.1080/09593331808616614
Mohseni, A., Kube, M., Fan, L., and Roddick, F. A. (2021). Treatment of wastewater reverse osmosis concentrate using alginate-immobilised microalgae: Integrated impact of solution conditions on algal bead performance. Chemosphere 276, 130028. doi:10.1016/j.chemosphere.2021.130028
Nakarmi, A., Kanel, S., Nadagouda, M. N., and Viswanathan, T. (2022). “Applications of conventional and advanced technologies for phosphorus remediation from contaminated water,” in Green functionalized nanomaterials for environmental applications (Amsterdam: Elsevier), 181–213. doi:10.1016/b978-0-12-823137-1.00007-5
Rasoul-Amini, S., Montazeri-Najafabady, N., Shaker, S., Safari, A., Kazemi, A., Mousavi, P., et al. (2014). Removal of nitrogen and phosphorus from wastewater using microalgae free cells in bath culture system. Biocatal. Agric. Biotechnol. 3 (2), 126–131. doi:10.1016/j.bcab.2013.09.003
Rillig, M. C., Wright, S. F., and Eviner, V. T. (2004). Arbuscular mycorrhizae, glomalin, and soil aggregation. Plant Soil 238 (2), 325–333. doi:10.1023/a:1014483303813
Salgueiro, J. L., Perez, L., Maceiras, R., Sanchez, A., and Cancela, A. (2016). Bioremediation of wastewater using Chlorella vulgaris microalgae: Phosphorus and organic matter. Int. J. Environ. Res. 10 (3), 465–470. doi:10.22059/ijer.2016.58766
Sinha, E., Michalak, A. M., Calvin, K. V., and Lawrence, P. J. (2019). Societal decisions about climate mitigation will have dramatic impacts on eutrophication in the 21st century. Nat. Commun. 10 (1), 939. doi:10.1038/s41467-019-08884-w
Tummino, M. L., Magnacca, G., Cimino, D., Laurenti, E., and Nisticò, R. (2020). The innovation comes from The Sea: Chitosan and alginate hybrid gels and films as sustainable materials for wastewater remediation. Int. J. Mol. Sci. 21 (2), 550. doi:10.3390/ijms21020550
U.S. EPA (1994). Method 200.7: Determination of metals and trace elements in water and wastes by inductively coupled plasma-atomic emission spectrometry, 4.4. Cincinnati, OH, Revision. Available at: https://www.epa.gov/sites/production/files/2015-08/documents/method_200-7_rev_4-4_1994.pdf.
Velings, N. M., and Mestdagh, M. M. (1995). Physico-chemical properties of alginate gel beads. Polym. Gels Netw. 3 (3), 311–330. doi:10.1016/0966-7822(94)00043-7
Vidyashankar, S., and Ravishankar, G. A. (2016). Algae-based bioremediation. Bioremediation Bioeconomy, 457–493. doi:10.1016/b978-0-12-802830-8.00018-6
Wright, S. F, and Upadhyaya, A (1996). Glomalin extraction. Available at: https://www.ars.usda.gov/ARSUserFiles/12650400/glomalin/extract.pdf (Accessed July 6, 2017).
Keywords: Chlorella vulgaris, glomalin, bioremediation, immobilized algae, sodium alginate, phosphorus
Citation: Percivall K, Amgain NR, Inglett K, Strauss SL and Bhadha JH (2022) Phosphorous remediation using alginate/glomalin biobeads: Examining structural cohesivity, nutrient retention, and reapplication viability. Front. Environ. Sci. 10:889940. doi: 10.3389/fenvs.2022.889940
Received: 04 March 2022; Accepted: 27 June 2022;
Published: 15 July 2022.
Edited by:
Farooq Sher, Nottingham Trent University, United KingdomReviewed by:
Yang Li, Anhui University of Science and Technology, ChinaShakeel Ahmad Bhat, Sher-e-Kashmir University of Agricultural Sciences and Technology, India
Copyright © 2022 Percivall, Amgain, Inglett, Strauss and Bhadha. This is an open-access article distributed under the terms of the Creative Commons Attribution License (CC BY). The use, distribution or reproduction in other forums is permitted, provided the original author(s) and the copyright owner(s) are credited and that the original publication in this journal is cited, in accordance with accepted academic practice. No use, distribution or reproduction is permitted which does not comply with these terms.
*Correspondence: Jehangir H. Bhadha, amFuZ29AdWZsLmVkdQ==