- 1Departamento de Ecología, Genética y Evolución, Facultad de Ciencias Exactas y Naturales, Universidad de Buenos Aires. Instituto de Ecología, Genética y Evolución de Buenos Aires (IEGEBA - CONICET/UBA), Ciudad Autónoma de Buenos Aires, Argentina
- 2Département des science biologiques, Université du Québec à Montréal, Montréal, QC, Canada
Submerged macrophytes play a key role in maintaining clear vegetated states in shallow lakes, but their role on methane (CH4) dynamics is less explored. They might enhance methanogenesis by providing organic matter but they can also supply oxygen to the sediments increasing methanotrophy. They may also affect gas exchange by diminishing wind turbulence in the water column. We previously measured seasonal CO2 and CH4 partial pressure (pCO2 and pCH4) and diffusive fluxes from two clear vegetated and two turbid algal shallow lakes of the Pampean Plain, Argentina, and we reported that clear lakes had higher mean annual pCH4 despite states having similar mean annual CH4 diffusive flux. In this study we explore the contribution of physical and biological factors regulating surface pCH4. Mean annual CH4 diffusive fluxes and CH4 fraction of oxidation (Fox) were similar between states, implying a comparable mean annual CH4 input. kCH4 was significantly higher than kCO2, suggesting occurrence of CH4 microbubbles, yet kCH4 was higher in turbid lakes than in clear lakes, implying a higher microbubble formation in turbid lakes. Furthermore, in turbid lakes there were positive relationships between k and wind speed, and between k and pCH4, yet in clear lakes these relations were absent. Results suggest that submerged vegetation suppresses wind induced turbulence in clear vegetated lakes, decoupling kCH4 from wind and reducing microbubble formation, therefore augmenting pCH4 in their surface waters. Overall, physical rather than biological factors appear to control the observed differences in pCH4 between states.
Introduction
Freshwater systems are a significant component of the global carbon cycle (Tranvik et al., 2018) and they emit substantial amounts of methane (CH4) to the atmosphere (Bastviken et al., 2011). Within freshwater systems, shallow lakes are considered to be biogeochemical hot spots and are distributed worldwide (Downing 2010; Holgerson and Raymond 2016). In some regions, shallow lakes can present two contrasting states: a clear water state dominated by submerged macrophytes, with low turbidity and low phytoplankton biomass, and a turbid water state dominated by phytoplankton, with high turbidity and no submerged vegetation (Scheffer et al., 1993; Sánchez et al., 2015). Submerged vegetation plays a key role in maintaining clear vegetated states by preventing sediment resuspension, by taking up nutrients from the water column, and by providing refuge for zooplankton, among others (Scheffer 2001; Hilt 2015). Their presence can also affect other processes, such as primary production, carbon burial rates and greenhouse gas (GHG) emissions (Hilt et al., 2017). In particular, the effect of submerged vegetation or phytoplankton on CH4 dynamics is not well understood (Hilt et al., 2017). Submerged vegetation can enhance methanogenesis by providing macrophyte-derived carbon to the sediments (Emilson et al., 2018; Grasset et al., 2019), but phytoplankton-derived carbon has also been reported to enhance methanogenesis in sediments (Schwarz et al., 2008; West et al., 2012). Similarly, alternative states could have a differential effect on methane oxidation (MOX). The activity of methane oxidizing bacteria (MOB) depends mainly on, O2 concentration and light penetration, where lower O2 concentrations in combination with a reduction of light favors methanotrophs (Thottathil et al., 2018). Both submerged vegetation and phytoplankton can generate high oxygen concentrations and they can also diminish light penetration in the water column (Torremorell et al., 2009; Andersen et al., 2017). At the same time, it has been shown that submerged vegetation diminishes wind induced turbulence in the water column (Herb and Stefan 2005; Andersen et al., 2017), which could have a physical effect on gas exchange with the atmosphere, and this effect is not present in turbid phytoplanktonic lakes. Thus, it is not straightforward to predict potential biological—methanogenesis and methanotrophy—and physical - gas exchange with the atmosphere and also vertical and horizontal transport - differences in CH4 dynamics between clear vegetated and turbid algal shallow lakes.
In a previous study we reported that clear vegetated shallow lakes from the Pampean Plain of Argentina had higher mean annual surface water CH4 partial pressure (pCH4) in comparison with turbid algal lakes (Baliña et al. under revision). However, we also reported that clear and turbid lakes presented similar mean annual CH4 diffusive fluxes. The average CH4 concentration in the water is the net balance between the rates of input to the water column, oxidation within the water column, and outflux to the atmosphere (Vachon et al., 2019; Noyce and Megonigal, 2021). Given that the average fluxes to the atmosphere were similar between states (Baliña et al. under revision), the differences in average pCH4 could be the result of a physical effect due to differences in gas exchange, potentially combined with a biological effect, due to differences in the net balance between input and oxidation in the water column between the two states. CH4 can be emitted from surface waters by diffusive flux, a strictly fickian process which depends on the concentration gradient at the water-air interface and the gas exchange velocity (k) and, in some cases, CH4 can also be emitted in the form of microbubbles (Bastviken et al., 2004; Beaulieu et al., 2012). If CH4 microbubbles are present, they can generate an additional flux of CH4 to the atmosphere which will lead to increased measured k that is difficult to distinguish from that of purely diffusive k, and to a decoupling between CH4 and CO2 exchange velocities (Beaulieu et al., 2012; Prairie and del Giorgio 2013; McGinnis et al., 2015). This is a CH4 emission pathway that is thought to relate positively to both water column turbulence and surface water CH4 concentration (Prairie and del Giorgio 2013; McGinnis et al., 2015; Tang et al., 2016), yet it is currently difficult to predict whether these two contrasting lake states may be associated with an increased incidence of microbubbles.
In this study we assess both biological and physical factors influencing ambient surface pCH4 in these shallow lakes: as biological factors, we explored potential differences in overall CH4 oxidation between clear vegetated and turbid algal lakes, and by combining the patterns of oxidation and diffusive flux we infer potential differences in CH4 input between states. As physical factors, we explored potential differences in kCH4 between clear vegetated and turbid algal shallow lakes and also possible differences in the relationship between kCH4 and wind, and between kCH4 and pCH4. In addition, we compared the patterns of kCO2 and kCH4 to infer differences in CH4 microbubble dynamics between the two states.
Materials and Methods
Study Area and Design
This study was carried out in the Pampean Plain (Buenos Aires, Argentina), a region with an exceptionally flat landscape that has a mean annual precipitation of 935 mm and mean annual temperature of 15.3°C (Allende et al., 2009). This region is characterized by the presence of hundreds of thousands of shallow lakes (Geraldi et al., 2011) that are eutrophic to hypereutrophic, polymictic (Diovisalvi et al., 2015), and that can be mostly found under two alternative states: a clear vegetated state dominated by submerged macrophytes, and a turbid algal state dominated by phytoplankton (Allende et al., 2009). For this study, we used data collected from four shallow lakes of the Pampean Plain: two in a clear vegetated state dominated by submerged macrophytes—La Segunda (SG) and Kakel Huincul (KH)—and other two in a turbid algal state, dominated by phytoplankton and with no submerged macrophytes—El Burro (BU) and La Salada Monasterio (SA) - (Figure 1). Lakes were sampled seasonally between 2018 and 2019, in winter (11–25 June 2018), spring (16–23 October 2019), summer (3–7 February 2019) and autumn (22–30 April 2019). In each field campaign physical, chemical, and biogeochemical parameters were measured (see specific parameters and related details below).
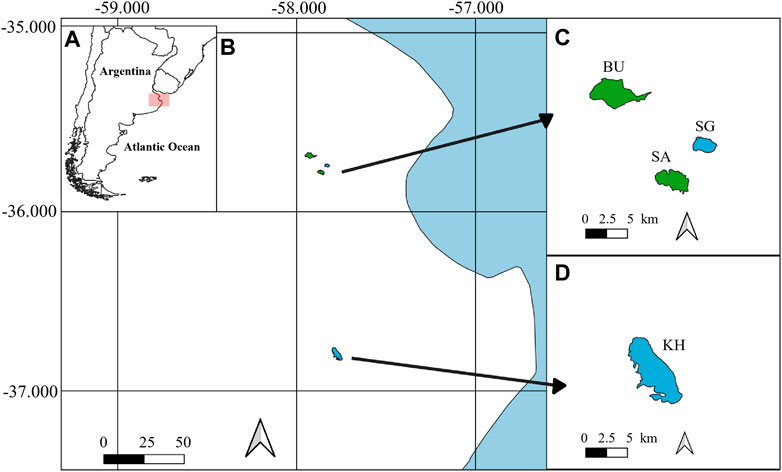
FIGURE 1. (A) Map of Argentina, in red the study area. (B) Study area and the four studied shallow lakes. (C) La Segunda (SG), La Salada Monasterio (SA), El Burro (BU) and (D) Kakel Huincul (KH). Lakes in green correspond to the turbid algal lakes, whereas lakes in blue correspond to the clear vegetated lakes.
Baliña et al. (under revision) presented the environmental background data, pCH4 and pCO2, and flux data obtained for these lakes during the above-mentioned campaigns. Here we focus on the patterns of kCH4 and kCO2 derived from those diffusive fluxes and surface water pCH4 and pCO2, as well as on the isotopic signature of the surface water CH4, which was used to derive CH4 oxidation extent. Below we provide a summary of the methods used to obtain the surface water gas concentrations and diffusive fluxes (further details can be found in Baliña et al. (under revision)) and also the methods used to obtain the gas exchange velocities, the isotopic signature of surface water CH4 and fresh CH4 bubbles, and the calculation of CH4 fraction of oxidation. Background information of the studied lakes can be found in Supplementary Table S1.
Environmental Parameters
In each shallow lake we measured surface water temperature and dissolved oxygen using a multi parameter HACH HQ30D portable sensor (HACH, United States). We also measured air temperature, atmospheric pressure, and wind speed using a Kestrel (4,000 Pocket Weather Tracker, Nielsen-Kellerman).
CH4 and CO2 Dissolved in the Water and Their Isotopic Signature
We took samples of surface waters to determine CH4 and CO2 partial pressure (p) in the water using the headspace technique, as described in Baliña et al. (under revision). Briefly, we filled two 60 ml syringes with 30 ml of water and 30 ml of atmospheric air, creating a 1:1 ratio of water: air. The syringes were then shaken for 2 min to ensure equilibration of the gas between the two phases. After equilibration, the 30 ml of air in the syringe were injected into a 30 ml glass pre-evacuated vial equipped with a crimped rubber stopper (Exetainer, Labco) for subsequent analysis in a cavity ringdown spectrometer (CRDS, Picarro G2201-i) (Maher et al., 2013) that determines pCH4 and pCO2 along with δ13C- CH4 isotopic signature. To obtain the original p and isotopic signature of the CH4 in the water, these data were subsequently corrected for the ambient air pCO2 and pCH4, the headspace ratio, in situ temperature of the water, water temperature after equilibration of the gas, the atmospheric pressure and the isotopic fractionation in the liquid:gas interface (Soued and Prairie 2020). pCH4 and pCO2 are reported as parts per million in volume (ppmv) and CH4 isotopic data are reported in the standard delta notation (δ) expressed in per mil (‰) relative to the Vienna Pee Dee Belemnite standard (Whiticar 1999).
Diffusive Flux
Diffusive fluxes of CH4 and CO2 at the water-air interface were measured using a floating chamber (volume = 18.8 L, area = 0.1 m2) equipped with a valve that allows to sample the chamber headspace, and also with an internal thermometer to track headspace temperature. We took samples from the chamber headspace at intervals of 5 min for 15 min, obtaining four time points. At each time point, we took two samples of air that were injected into 30 ml glass pre-evacuated vials equipped with crimped rubber stoppers (Exetainer, Labco), for subsequent analysis in a cavity ringdown spectrometer (CRDS, Picarro G2201-i). At each sampling time we also registered temperature. The diffusive flux of each gas (Flux gas) was determined in mmol m−2 d−1 following Eq. 1:
Where s is the accumulation rate of gas in the chamber (ppmv min−1), V is the volume of the chamber (L), mV is the molar volume of the gas (L mmol−1) - which is corrected for the temperature in the chamber -, A is the chamber surface area (m2), and t is a factor that converts minutes to days (1 day = 1,440 min) (DelSontro et al., 2016).
Isotopic Signature of Fresh Methane Bubbles
We captured fresh CH4 bubbles to estimate the isotopic signature of fresh CH4 that we subsequently used as a source endmember in the oxidation mass balance. We used an inverted funnel (area = 0.3 m2) tied to a floating device that was deployed in the water. A glass bottle was filled with water from the lake and screwed to the neck of the funnel. After this, sediments were stirred using an oar, causing the liberation of fresh CH4 bubbles from the sediment through the water column and into the bottle (Supplementry Figure S1). We took two 30 ml air samples from the headspace that was generated in bottle and injected this air into 30 ml glass pre-evacuated vials equipped with crimped rubber stoppers (Exetainer, Labco), for subsequent analysis in a cavity ringdown spectrometer (CRDS, Picarro G2201-i). We determined δ13C-CH4 signature, as described for the gas dissolved in the water.
Estimation of the Extent of Water Column CH4 Oxidation (Fox)
To explore possible differences in CH4 oxidation between clear vegetated and turbid algal shallow lakes, we performed isotopic mass balances using two different models: a steady state open model (Eq. 2; Happell et al., 1994) and a non-steady state closed model (Eq. 3; Liptay et al., 1998). These models estimate a fraction of oxidation (Fox) based on different assumptions. The first model assumes a steady state system whereas the second considers that the water body may be in a dynamic, not at steady state condition (Thottathil et al., 2018).
δ13Csource is the isotopic signature of the source of methane, in this case the isotopic signature of the fresh CH4 bubbles; δ13CWT is the isotopic signature of the CH4 within the water column; and α is the isotopic fractionation factor related to microbial CH4 oxidation. We used a value of α = 1.021 following Coleman et al. (1981) and Thottathil et al. (2018). Additionally, we also calculated Fox using values of α = 1.005 and α = 1.031 (Alperin et al., 1988; Whiticar 1999; Clayer et al., 2018) to better qualify the uncertainty around the selected α value, which is an intermediate value within the mentioned range.
Exchange Velocities Derived From Flux Measurements
The gas exchange velocity (k) is a rate equivalent to the depth of the water column that is equilibrated with the atmosphere per unit time (Prairie and del Giorgio 2013). This parameter was obtained using the measurements of diffusive flux and of gas dissolved in the surface waters, following Eq. 4:
Where Flux gas is the diffusive flux for CH4 or CO2 determined using Eq. 1 (mmol m−2 d−1), Kh is the Henry’s constant correspondent to each gas corrected for atmospheric pressure and water temperature, and ∆pGas is the difference between the partial pressure of the respective gas in the water (Pw) and the partial pressure of the gas in equilibrium with the atmosphere (Peq), i.e.,
In order to allow comparison between gas exchange velocities, individual kCH4 and kCO2 were standardized to a Schmidt number of 600, following Eq. 5:
Where Sc is the Schmidt number of a given gas at a given temperature (Wanninkhof 1992), and n is a value that depends on wind speed. We used a value of n = 2/3 for ambient wind speeds <3.7 m s−1 and of n = 1/2 for ambient wind speeds >3.7 m s−1 (Guérin et al., 2007).
Statistical Analyses
To explore differences in mean annual CH4 Fox between states, we used a mixed generalized linear model with one fixed factor (state) and two random factors (season and lake). Since FOX is a fraction we used the Beta distribution, which has a fixed domain between 0 and 1. We tested differences between k600 CO2 and k600 CH4 using a mixed general linear model with one fixed factor (gas, CO2 or CH4) and two random factors (season and lake). To test differences between states in k600 derived from CH4 and k600 derived from CO2, we used a mixed general linear model with one fixed factor (state) and two random factors (season and lake). To explore relations between k600 CH4 vs. wind, k600 CH4 vs. pCH4, and k600 CO2 vs. wind, we performed simple linear regressions for each state, including in all cases two random factors (season and lake).
We tested the assumptions for each model: for the Fox model we analyzed the distribution of standardized residuals vs. predicted values to explore homogeneity of variances. For the rest of the models, residuals were tested to fit the assumptions of normality and homogeneity of variances. The Fox model was carried out using the package “glmmTMB” (Brooks et al., 2017). The rest of the models were done using package “lmerTest 3.1-2” (Kuznetsova et al., 2017). Normality was checked with package “Stats 3.6.2” (Royston 1982) and homogeneity of variances was checked by exploring the relation between standardized residuals vs. predicted values and also with package “Car 3.0-8” (Fox and Sanford., 2018). If homogeneity of variances was not fulfilled, we modeled heteroscedasticity by means of three different functions: varIdent, varPower and varExp (Zuur et al., 2009) using package nlme 3.1-142 (Jose et al., 2019). All tests were performed at the 95% significance level using R version 3.6.2 in the RStudio environment version 1.2.5019 (R Core Team 2019). Figures were plotted with the package “ggplot2 3.3.2” (Hadley, 2016).
Results
Clear vegetated lakes had three-fold higher mean annual pCH4 than turbid algal lakes (1,181.7 ± 1,375.8 ppmv and 358.9 ± 390.4 ppmv, respectively) (p = 0.002, df = 1, F = 10.6; Figure 2A; data from Baliña et al. (under revision)). Nonetheless, clear vegetated and turbid algal lakes had similar mean annual CH4 diffusive fluxes (14.4 ± 24.2 and 19.9 ± 33.5 mmol m−2 d−1, respectively) (Figure 2B; data from Baliña et al. (under revision)). Mean annual δ13C-CH4 was similar between clear vegetated and turbid algal shallow lakes and in both states and the isotopic signatures corresponded to enriched values related to fresh sediment CH4 (Figure 2C and Supplementary Table S2). Our estimates of mean annual CH4 fraction of oxidation (Fox), obtained using the non-steady state closed model and assuming an average fractionation (α) of 1.021, were also very similar between clear vegetated and turbid algal lakes (average 0.57 ± 0.09 and 0.58 ± 0.11, respectively; Figure 2C). The steady state open model was not appropriate for these systems, since in a significant number of cases it yielded Fox values higher than one (Supplementary Figure S2), as has been observed in other studies (Bastviken et al., 2002; Barbosa et al., 2018; Thottathil et al., 2018). Using α = 1.005 we obtained nonsensical Fox values for both closed and open models, whereas using α = 1.031 we obtained logical Fox values for both closed and open models that were in the range of the results obtained with α of 1.021 (Supplementary Figure S3).
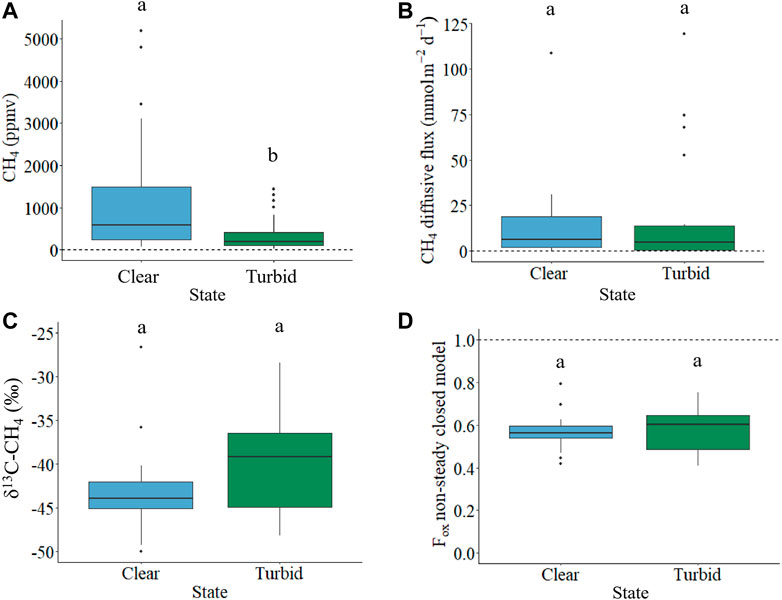
FIGURE 2. (A) Mean annual surface water pCH4 dissolved (ppmv), (B) mean annual CH4 diffusive flux (mmol m−2 d−1), (C) mean annual CH4 isotopic signature (‰) and (D) mean annual Fraction of oxidation (Fox) obtained with the non-steady closed model, of clear vegetated (blue) and turbid algal (green) shallow lakes, respectively. Different letters (a and b) imply significant differences between states within the respective panels. The dashed line in panel (A) corresponds to the mean annual atmospheric CH4 partial pressure (1.71 ppmv), in panel (B) corresponds to a zero CH4 diffusive flux and in panel (D) corresponds to an oxidation of 100%.
There was a large range in measured k600 values based on either CO2 or CH4 across lakes (from 0.3 to 59.8 m d−1), and although both agreed well for approximately 32% of observations, there was a high proportion of points that deviated significantly from the expected 1:1 relationship, and most of these points corresponded to turbid shallow lakes (Figure 3). The overall mean annual k600 CH4 (12.7 ± 15.1 m d−1) was significantly (6.4 times) higher than mean annual k600 CO2 (1.98 ± 1.43 m d−1), (p < 0.0001, df = 1, F = 19.7; Figure 4A). There were also differences between states in the patterns of gas exchange: mean annual k600 CH4 was 3 times higher in turbid lakes (19.3 ± 18.9 m d−1) than in clear lakes (6.7 ± 7.0 m d−1) (p = 0.0092, df = 1, F = 7.5, Figure 4B), whereas mean annual k600 CO2 was similar between clear and turbid lakes (2.0 ± 1.5 m d−1 and 2.0 ± 1.4 m d−1, respectively, Figure 4C).
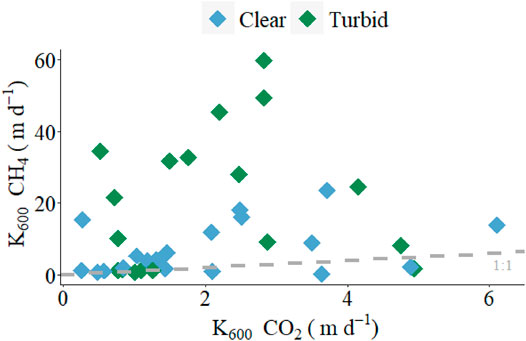
FIGURE 3. Linear regression between k600 CH4 (m d−1) and k600 CO2 (m d−1). The dashed line represents the expected 1:1 relation between standardized exchange velocities. Blue diamonds correspond to clear vegetated lakes and green diamonds correspond to turbid algal lakes.
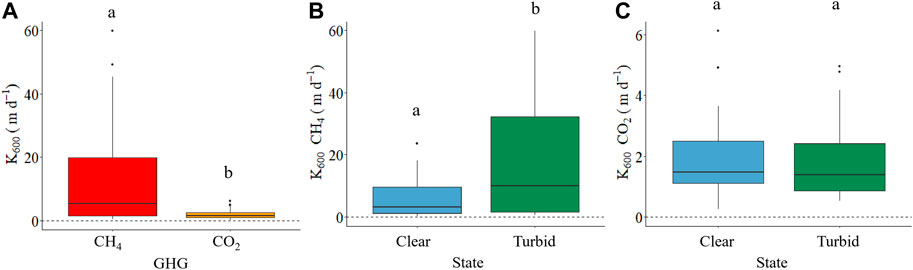
FIGURE 4. Overall k600 of CH4 and CO2: (m d-1) (A), k600 CH4 (m d-1) for clear vegetated and turbid algal lakes (B) and k600 CO2 (m d-1) for clear and turbid lakes (C). Different letters (a and b) imply significant differences. The dotted line in the three panels corresponds to a zero-exchange velocity.
We explored the relationships between K600 and wind speed and also between K600 and CH4 and CO2 partial pressure in the water, separately for clear vegetated and turbid algal lakes. Mean annual wind speed was similar between clear vegetated and turbid algal lakes (Supplementary Figure S4). There was a significant positive relationship between k600 CO2 and wind speed (Figure 5A) and also between k600 CH4 and wind speed (Figure 5B), but in both cases these relationships were present only for turbid algal lakes and were not significant for clear vegetated lakes. These two relationships with wind in turbid lakes, however, are strikingly different: the slope of the k600 CH4 vs. wind relationship (4.9 ± 1.9) is one order of magnitude higher than that of k600 CO2 (0.4 ± 0.1), and the intercepts are significantly different as well (6 ± 7.7 vs. 1 ± 0.5, respectively). Regarding dissolved GHG, pCO2 was weakly and negatively related to k600 CO2 in both clear vegetated and turbid algal shallow lakes, and the relationship was similar for both states (Figure 5C). Likewise, there was a weak (but not significant) negative relationship between ambient pCH4 and k600 CH4 in clear vegetated lakes yet, interestingly, there was a strong significant positive relationship between pCH4 and k600 CH4 in turbid algal lakes (Figure 5D).
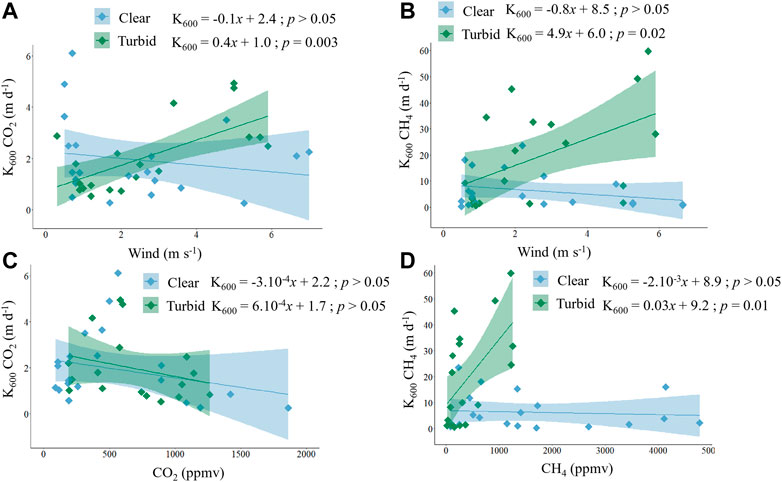
FIGURE 5. Linear regressions between (A) k600 CH4 and wind, (B) k600 CO2 and wind, (C)k600 CH4 and pCH4, and (D) k600 CO2 and pCO2. Blue diamonds correspond to clear vegetated lakes while green diamonds correspond to turbid algal lakes. The shade corresponds to the standard error of the respective linear models and p values correspond to the slope of the regression model.
Discussion
Surface water δ13C-CH4 corresponded to enriched methane in both clear vegetated and turbid algal shallow lakes (between −50‰ and −25‰), followed by also similar percentages of CH4 oxidation (mean of 57%) and, therefore, implying a high methanotrophic activity in both states. A comparable range of δ13C-CH4 was reported for a subtropical shallow wetland from Australia (−53 and −39‰, Jeffrey et al., 2019) and a shallow Boreal lakes (−60 and −35‰, Desrosiers et al., 2021). A wider range of Fox, also obtained using a non-steady state model, was reported for different habitat types within a shallow boreal lake (Desrosiers et al., 2021), with values ranging from 34% to 56% in Brasenia and Typha dominated habitats and down to 31% in open water areas. A wide range has also been reported for subtropical (15%–36%, Jeffrey et al., 2019), tropical (34%–100%; Barbosa et al., 2018), boreal (57%–75%; Bastviken et al., 2002) and temperate lakes (2%–97%; Thottathil et al., 2018) of varying size. This broad range of Fox both within and across aquatic systems, highlights the complexity in the regulation of CH4 oxidation. In this regard, the convergence in annual average Fox between clear vegetated and turbid algal lakes despite their contrasting environmental conditions is remarkable.
Although there was significant seasonal variability in pCH4 in both clear vegetated and turbid algal lakes (Baliña et al. (under revision)), this variation was centered around very different mean annual pCH4 values for each state. Assuming that these annual means reflect an average steady state partial pressure of each lake state and are not varying greatly, then the amount of CH4 exchanged at the water-air interface on an annual basis reflects the total CH4 input to the water column (which includes CH4 production in the sediments and water column, as well as lateral input) minus the CH4 oxidized. Considering that annually the fraction of oxidation and the rates of diffusive flux were similar between states, we can infer that on an annual basis CH4 input would be rather comparable between clear vegetated and turbid algal shallow lakes. CH4 production in the sediments depends mainly on temperature, oxygen concentration and on the amount and type of organic matter (Megonigal et al., 2003; Duc et al., 2010). Both macrophyte and phytoplankton derived organic matter are known to favor methanogenesis (Emilson et al., 2018; Yan et al., 2019), but the extent to which the dominance of these different sources of organic matter may condition carbon cycling and methanogenesis at the ecosystem level is not well understood, with few studies having assessed this impact (Brothers et al., 2013). Although in this study we do not explicitly address CH4 production, these results imply a comparable mean annual CH4 input between clear vegetated and turbid algal states, which could be plausible since the characteristics of both states seem to favor sediment methanogenesis. Independent of sediment production, several authors have demonstrated that there is also a significant potential for CH4 production in the water column (Grossart et al., 2011; Bogard et al., 2014; Günthel et al., 2019). Whereas there are studies that have explored possible isotopic signatures for this CH4 (Günthel et al., 2020; Hartmann et al., 2020), there is to date no clear information of what the isotopic signature of this fresh pelagic CH4 could be. Therefore, we could not include this source of CH4 in the isotopic mass balance. Nonetheless, we consider that for the purpose of the present study it is sufficient to include a sedimentary methane source to derive a first order estimate of oxidation extent.
In our study, k600 CH4 was 6.4 times higher than k600 CO2. Previous studies have reported k600 CH4 to be on average 1.8-fold higher than k600 CO2 in two boreal lakes (Rantakari et al., 2015), 2.3-fold higher in a Canadian hydroelectric reservoir and boreal lakes (Prairie and del Giorgio 2013), 2.5-fold times higher in oligotrophic Lake Stechlin (McGinnis et al., 2015), and 2.5-fold higher in a tropical reservoir from Brazil (Paranaíba et al., 2018). In all cases, the differences between CH4 and CO2 exchange velocities were explained by the presence of CH4 microbubbles, which result in a k600 CH4 that is higher than that from diffusive exchange alone (Beaulieu et al., 2012; Prairie and del Giorgio 2013) and, therefore, generates a decoupling between k600 CH4 and k600 CO2 (Prairie and del Giorgio 2013; McGinnis et al., 2015). Moreover, we observed that turbid lakes had higher k600 CH4 than clear lakes, which would suggest a differential CH4 microbubble formation between states. CH4 microbubbles are thought to be produced as a combination of CH4 supersaturation and turbulence (Vagle et al., 2010; Prairie and del Giorgio 2013; McGinnis et al., 2015). Although clear vegetated lakes had a higher mean annual pCH4 than turbid algal lakes, overall pCH4 was high in both states, and even higher in comparison with other studies that reported CH4 microbubble formation (Prairie and del Giorgio 2013; McGinnis et al., 2015; Tang et al., 2016). Therefore, both clear vegetated and turbid algal lakes could potentially harbor the production of CH4 microbubbles in terms of the amount of CH4 present in the water column. On the other hand, water column turbulence is substantially different between states, as evidenced by the different relationship that exists between k600 CO2 and wind speed and as has been reported in previous studies (Herb and Stefan 2005; Andersen et al., 2017): in clear vegetated lakes submerged vegetation tends to suppress wind induced turbulence in the water column, whereas in turbid algal lakes the absence of submerged vegetation allows a higher wind induced turbulence. This might explain the observed apparent higher CH4 microbubble formation in turbid algal lakes, leading to higher k600 CH4 in comparison with clear vegetated lakes, in spite of average lower pCH4.
Most models of diffusive gas exchange in lakes have positively linked k600 to wind speed (Sebacher et al., 1983; Raymond and Cole 2001; Guérin et al., 2007) and, if CH4 microbubbles are present, k600 CH4 is also expected to have a positive correlation with pCH4 (Prairie and del Giorgio 2013). Our results suggest a fundamentally different response of CH4 and CO2 to wind forcing in turbid algal lakes, evidenced by the slope of the regressions, which also point towards CH4 microbubble formation. In clear vegetated lakes, in contrast, neither k600 CH4 nor k600 CO2 were significantly related to wind, yet k600 CH4 was nevertheless consistently higher than k600 CO2, also implying CH4 microbubble formation but with a different behavior towards wind turbulence. The positive and expected relationship between k600 CH4 and pCH4 was found only for turbid algal lakes and is consistent with a pattern of wind induced microbubble formation that is enhanced by increasing supersaturation of CH4. This was not the outcome for clear vegetated lakes, implying that this wind vs. pCH4 interaction is largely suppressed in vegetated habitats. On the other hand, ambient pCO2 was weakly and negatively related to k600 CO2 in both states in a very similar manner, which is coherent with the expected role of gas exchange as a modulator of ambient gas concentrations in surface waters, as has been previously reported for lakes (Lapierre et al., 2013) and rivers (Rocher-Ros et al., 2019). Therefore, in turbid algal lakes the combination of wind and pCH4 determine k600 CH4, yet in clear vegetated lakes, gas exchange is largely decoupled from wind, and under this circumstance a reciprocal relationship is established between pCH4 and gas exchange: the wind-independent and relatively constant k in clear vegetated lakes leads to high pCH4 because it acts as a lid, yet pCH4 also appears to influence k600 CH4, because the high pCH4 leads to higher k600 CH4 relative to CO2 through microbubble formation. Submerged vegetation therefore influences gas dynamics two-fold in these clear vegetated lakes: directly by modulating the effect of wind on water column turbulence and therefore on gas exchange velocity, as is the case on CO2 exchange, but also indirectly, by altering the dynamics of microbubble formation and therefore of CH4 exchange.
Previous studies have also reported a strong impact of aquatic vegetation on gas exchange in shallow lakes. Kosten et al. (2016) reported a lower CH4 exchange velocity in the presence of free-floating vegetation in comparison with open water sites, where the higher pCH4 detected below the floating vegetation was partly explained by the lower k. Likewise, Barbosa et al. (2020) reported that vegetated and open water habitats from a tropical floodplain lake had similar CH4 diffusive fluxes but that vegetated habitats had higher pCH4, which was linked to a higher k in open water sites. Martinsen et al. (2020) also reported a lower CO2 exchange velocity in a small shallow lake when submerged macrophytes were more abundant and related this observation with a negative effect of vegetation on the mixing of the water column. In our case, k600 CO2 did not differ in average magnitude between lake states, but as pointed out above, the relationship between k600 CO2 and wind differed markedly between states. An almost complete decoupling between exchange velocity and wind has been reported in previous studies carried out in small lakes (Heiskanen et al., 2014; Tedford et al., 2014; Tan et al., 2021), where wind-based models (Cole and Caraco 1998; Crusius and Wanninkhof 2003; MacIntyre et al., 2010) did not adequately explain the observed patterns in gas exchange, and where other factors, such as convection, had a stronger influence on gas exchange velocities. In our study, wind speed was a good predictor for k600 CO2 and k600 CH4 but only in turbid algal lakes. In clear vegetated lakes, submerged vegetation seems to decouple this relationship, therefore the use of wind speed would not be a good predictor for exchange velocities in these systems.
Overall, our results imply a roughly comparable mean annual CH4 input to the water column between lakes in turbid algal and clear vegetated states, the latter inferred from similar mean annual CH4 diffusive fluxes and mean annual CH4 fraction of oxidation. We also observed that mean annual pCH4 in clear lakes was 3 times higher than in turbid lakes, while mean annual k600 CH4 in turbid lakes was 3 times higher than in clear lakes. Furthermore, the higher k600 CH4 in turbid lakes was associated with a positive relationship with wind and pCH4, and these relationships were absent in clear vegetated shallow lakes. Therefore, the higher pCH4 in clear vegetated lakes could be explained by their lower average k600 CH4 and also by the absence of a relation between k600 CH4 and wind, which further suggests a lower CH4 microbubble formation. These patterns seem to be driven by a physical effect produced by the submerged vegetation over the mixing of the water column: a reduction of water column turbulence apparently leads to both a lower exchange velocity and also to a reduction of CH4 microbubble formation, consequently leading to higher surface water pCH4 in clear vegetated lakes (Figure 6). Furthermore, whereas pCO2 is at least in part controlled by k600 CO2, as expected, pCH4 seems to be differentially controlled depending on the lake state: on one hand, in clear lakes pCH4 is primarily controlled by gas exchange, yet k600 CH4 is to some degree also influenced by pCH4, since there is some degree of microbubble formation and k600 CH4 is still systematically higher than k600 CO2. In turbid algal lakes, on the other hand, pCH4 appears to influence the apparent k600 CH4 through wind-driven CH4 microbubble formation, but there is also a control of k600 CH4 over pCH4 (Figure 6). Therefore, physical rather than biological processes - ie. methanogenesis and methanotrophy-seem to be controlling the differences observed in surface water mean annual pCH4 between clear vegetated and turbid algal shallow lakes from the Pampean plain.
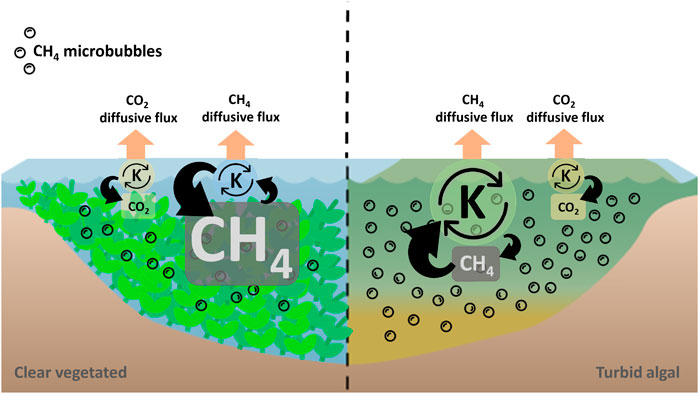
FIGURE 6. Conceptual scheme of a plausible explanation for the observed results: states had similar mean annual CO2 diffusive flux, pCO2 and k600 CO2. States also had similar mean annual CH4 diffusive flux, but clear vegetated lakes had higher mean annual pCH4 than turbid algal lakes. This difference in mean annual pCH4 between states was explained by a lower mean annual k600 CH4 in clear vegetated lakes, where submerged vegetation reduced wind induced turbulence in the water column, therefore diminishing CH4 microbubbles formation. Overall, pCO2 is controlled by k600 CO2 in both states, as expected, but regulation of CH4 is more complex: in clear lakes pCH4 is mainly controlled by gas exchange (k600 CH4) but there is also a minor control of pCH4 over k600 CH4, reflected in a consistently elevated k600 CH4 relative to k600 CO2 but that is independent of pCH4 and wind. In turbid lakes, apparent k600 CH4 is mainly controlled by wind and secondarily by pCH4 through wind-driven CH4 microbubble formation, whereas average pCH4 is itself secondarily influenced by k600 CH4.
The observation that k600 CH4 is systematically higher than k600 CO2 is consistent with the formation and subsequent emission of CH4 microbubbles, and similar observations have been reported for rivers (Beaulieu et al., 2012; Campeau et al., 2014), lakes (McGinnis et al., 2015; Rantakari et al., 2015; Jansen et al., 2020), and reservoirs (Prairie and del Giorgio 2013; Paranaíba et al., 2018), with enhancement values ranging from 1.8 to 2.5. Although this appears to be a widespread phenomenon, the data are still sparse because there are surprisingly few studies that have measured CH4 air-water exchange in parallel to that of another gas that can be used as a reference. As a result, the CH4 microbubble dynamics in inland waters remains poorly constrained (Jansen et al., 2020), and this adds a large degree of uncertainty to current models and budgets of freshwater CH4 emissions that already include ebullitive, diffusive and plant mediated fluxes. Here we have shown that there is indeed an interaction between wind velocity and surface water CH4 concentration in determining the relative enhancement of k600 CH4 but that this combined effect is only present in turbid shallow lakes. In vegetated clear lakes, the presence of submerged macrophytes seems to greatly dampen wind-induced water column turbulence, and under this scenario, k600 CH4 responds to neither wind speed nor to pCH4. Had we sampled lakes in only one state, we may have perhaps concluded that pCH4 regulates k, or that k regulates pCH4, and yet both are occurring but under different habitat and climatic combinations. It is clear that the regulation of water-air CH4 exchange is complex, and an improved understanding of this process will require parallel CH4 and CO2 - or another reference gas - measurements carried out in a wide range of habitat and climatic conditions.
Data Availability Statement
The raw data supporting the conclusion of this article will be made available by the authors, without undue reservation.
Author Contributions
SB contributed substantially to the data acquisition, laboratory, and statistical analyses, as well as to the writing of the manuscript. MLS contributed substantially to the data acquisition and to the drafting of the manuscript. PdG contributed substantially to the study’s conception, to the analyses of the results, and the drafting of the manuscript.
Funding
This work was supported by the NSERC/HQ CarBBAS Industrial Research Chair, by Préstamo BID PICT RAICES 2017-2498 and by Préstamo BID PICT 2015-1509.
Conflict of Interest
The authors declare that the research was conducted in the absence of any commercial or financial relationships that could be construed as a potential conflict of interest.
Publisher’s Note
All claims expressed in this article are solely those of the authors and do not necessarily represent those of their affiliated organizations, or those of the publisher, the editors and the reviewers. Any product that may be evaluated in this article, or claim that may be made by its manufacturer, is not guaranteed or endorsed by the publisher.
Acknowledgments
We would like to thank several colleagues that were essential to carry out field work: F. Zolezzi, V. Rago, G. Chaparro, M. Saraceno, C. Miranda, S. Porcel and F. Rego. We also thank I. Izaguirre for academic and logistical support and L. Babino for statistical advice. We thank A. Parkes for the laboratory and logistics help through this entire project, P. Bodmer and P. Barbosa for their constructive comments on this manuscript and all the members of the CarBBAS laboratory for their helpful insights and discussions.
Supplementary Material
The Supplementary Material for this article can be found online at: https://www.frontiersin.org/articles/10.3389/fenvs.2022.892339/full#supplementary-material
References
Allende, L., Tell, G., Zagarese, H., Torremorell, A., Pérez, G., Bustingorry, J., et al. (2009). Phytoplankton and Primary Production in Clear-Vegetated, Inorganic-Turbid, and Algal-Turbid Shallow Lakes from the Pampa Plain (Argentina). Hydrobiologia 624 (1), 45–60. doi:10.1007/s10750-008-9665-9
Alperin, M. J., Reeburgh, W. S., and Whiticar, M. J. (1988). Carbon and Hydrogen Isotope Fractionation Resulting from Anaerobic Methane Oxidation. Glob. Biogeochem. Cycles 2 (3), 279–288. doi:10.1029/gb002i003p00279
Andersen, M. R., Kragh, T., and Sand-Jensen., K. (20171862). Extreme Diel Dissolved Oxygen and Carbon Cycles in Shallow Vegetated Lakes. Proc. R. Soc. B 284, 20171427. doi:10.1098/rspb.2017.1427
Barbosa, P. M., Farjalla, V. F., Melack, J. M., Amaral, J. H. F., da Silva, J. S., and Forsberg, B. R. (2018). High Rates of Methane Oxidation in an Amazon Floodplain Lake. Biogeochemistry 137 (3), 351–365. doi:10.1007/s10533-018-0425-2
Barbosa, P. M., Melack, J. M., Amaral, J. H. F., MacIntyre, S., Kasper, D., Cortés, A., et al. (2020). Dissolved Methane Concentrations and Fluxes to the Atmosphere from a Tropical Floodplain Lake. Biogeochemistry 148 (2), 129–151. doi:10.1007/s10533-020-00650-1
Bastviken, D., Tranvik, L. J., Downing, J. A., Crill, P. M., and Enrich-Prast, A. (2011). Freshwater Methane Emissions Offset the Continental Carbon Sink. Science 331 (Table 1), 50. doi:10.1126/science.1196808
Bastviken, D., Cole, J., Pace, M., and Tranvik, L. (2004). Methane Emissions from Lakes: Dependence of Lake Characteristics, Two Regional Assessments, and a Global Estimate. Glob. Biogeochem. Cycles 18 (4), a–n. doi:10.1029/2004GB002238
Bastviken, D., Ejlertsson, J., and Tranvik, L. (2002). Measurement of Methane Oxidation in Lakes: A Comparison of Methods. Environ. Sci. Technol. 36 (15), 3354–3361. doi:10.1021/es010311p
Beaulieu, J. J., Shuster, W. D., and Rebholz, J. A. (2012). Controls on Gas Transfer Velocities in a Large River. J. Geophys. Res. 117 (2), a–n. doi:10.1029/2011JG001794
Bogard, M. J., Del Giorgio, P. A., Boutet, L., Chaves, M. C. G., Prairie, Y. T., Merante, A., et al. Maria Carolina Garcia Chaves (2014). Oxic Water Column Methanogenesis as a Major Component of Aquatic CH4 Fluxes. Nat. Commun. 5 (May). doi:10.1038/ncomms6350
Brooks, M. E., Kristensen, K., Benthem, K. J. v., Magnusson, A., Berg, C. W., Nielsen, A., et al. (2017). GlmmTMB Balances Speed and Flexibility Among Packages for Zero-Inflated Generalized Linear Mixed Modeling. R J. 9 (2), 378–400. doi:10.32614/rj-2017-066
Brothers, S. M., Hilt, S., Attermeyer, K., Grossart, H. P., Kosten, S., Lischke, B., et al. (2013). A Regime Shift from Macrophyte to Phytoplankton Dominance Enhances Carbon Burial in a Shallow, Eutrophic Lake. Ecosphere 4 (11), art137. doi:10.1890/ES13-00247.1
Campeau, A., Lapierre, J.-f., Vachon, D., and Paul, G. (2014). Global Biogeochemical Cycles Boreal Landscape of Québec. Glob. Biogeochem. Cycles 28, 57–69. doi:10.1002/2013GB004685
Clayer, F., Moritz, A., Gélinas, Y., Tessier, A., and Gobeil, C. (2018). Modeling the Carbon Isotope Signatures of Methane and Dissolved Inorganic Carbon to Unravel Mineralization Pathways in Boreal Lake Sediments. Geochimica Cosmochimica Acta 229, 36–52. doi:10.1016/j.gca.2018.02.012
Cole, J. J., and Caraco, N. F. (1998). Atmospheric Exchange of Carbon Dioxide in a Low-Wind Oligotrophic Lake Measured by the Addition of SF6. Limnol. Oceanogr. 43 (4), 647–656. doi:10.4319/lo.1998.43.4.0647
Coleman, D. D., Risatti, J. B., and Schoell, M. (1981). Fractionation of Carbon and Hydrogen Isotopes by Methane-Oxidizing Bacteria. Geochimica Cosmochimica Acta 45 (7), 1033–1037. doi:10.1016/0016-7037(81)90129-0
Crusius, J., and Wanninkhof, R. (2003). Gas Transfer Velocities Measured at Low Wind Speed over a Lake. Limnol. Oceanogr. 48 (3), 1010–1017. doi:10.4319/lo.2003.48.3.1010
DelSontro, T., Boutet, L., St-Pierre, A., del Giorgio, P. A., and Prairie, Y. T. (2016). Methane Ebullition and Diffusion from Northern Ponds and Lakes Regulated by the Interaction between Temperature and System Productivity. Limnol. Oceanogr. 61, S62–S77. doi:10.1002/lno.10335
Desrosiers, K., DelSontro, T., and del Giorgio, P. A. (2021). Disproportionate Contribution of Vegetated Habitats to the CH4 and CO2 Budgets of a Boreal Lake. Ecosystems. doi:10.1007/s10021-021-00730-9
Diovisalvi, N., Bohn, V. Y., Piccolo, M. C., Perillo, G. M. E., Baigún, C., and Zagarese, H. E. (2015). Shallow Lakes from the Central Plains of Argentina: An Overview and Worldwide Comparative Analysis of Their Basic Limnological Features. Hydrobiologia 752 (1), 5–20. doi:10.1007/s10750-014-1946-x
Downing, J. A. (2010). Emerging Global Role of Small Lakes and Ponds: Little Things Mean a Lot. Limnetica 29 (1), 9–24. doi:10.23818/limn.29.02
Duc, N. T., Crill, P., and Bastviken, D. (2010). Implications of Temperature and Sediment Characteristics on Methane Formation and Oxidation in Lake Sediments. Biogeochemistry 100 (1), 185–196. doi:10.1007/s10533-010-9415-8
Emilson, E. J. S., Carson, M. A., Yakimovich, K. M., Osterholz, H., Dittmar, T., Gunn, J. M., et al. (2018). Climate-Driven Shifts in Sediment Chemistry Enhance Methane Production in Northern Lakes. Nat. Commun. 9 (1), 1801–1806. doi:10.1038/s41467-018-04236-2
Fox, J., and Sanford., W. (2018). Visualizing Fit and Lack of Fit in Complex Regression Models with Predictor Effect Plots and Partial Residuals. J. Stat. Softw. 87, 1–27. doi:10.18637/jss.v087.i09
Geraldi, Alejandra. M., Piccolo Maria, C., and Perillo, Gerardo. E. (2011). Lagunas Bonaerenses En El Paisaje. Cienc. Hoy 21 (123), 16–22.
Grasset, C., Abril, G., Mendonça, R., Roland, F., and Sobek, S. (2019). The Transformation of Macrophyte‐derived Organic Matter to Methane Relates to Plant Water and Nutrient Contents. Limnol. Oceanogr. 64 (4), 1737–1749. doi:10.1002/lno.11148
Grossart, H.-P., Frindte, K., Dziallas, C., Eckert, W., and Tang, K. W. (2011). Microbial Methane Production in Oxygenated Water Column of an Oligotrophic Lake. Proc. Natl. Acad. Sci. U.S.A. 108 (49), 19657–19661. doi:10.1073/pnas.1110716108
Guérin, F., Abril, G., Serça, D., Delon, C., Richard, S., Delmas, R., et al. (2007). Gas Transfer Velocities of CO2 and CH4 in a Tropical Reservoir and its River Downstream. J. Mar. Syst. 66 (1–4), 161–172. doi:10.1016/j.jmarsys.2006.03.019
Günthel, M., Donis, D., Kirillin, G., Ionescu, D., Bizic, M., McGinnis, D. F., et al. (2019). Contribution of Oxic Methane Production to Surface Methane Emission in Lakes and its Global Importance. Nat. Commun. 10 (1), 1–10. doi:10.1038/s41467-019-13320-0
Günthel, M., Klawonn, I., Woodhouse, J., Bižić, M., Ionescu, D., Ganzert, L., et al. (2020). Photosynthesis‐driven Methane Production in Oxic Lake Water as an Important Contributor to Methane Emission. Limnol. Oceanogr. 65 (12), 2853–2865. doi:10.1002/lno.11557
Happell, J. D., Chanton, J. P., and Showers, W. S. (1994). The Influence of Methane Oxidation on the Stable Isotopic Composition of Methane Emitted from Florida Swamp Forests. Geochimica Cosmochimica Acta 58 (20), 4377–4388. doi:10.1016/0016-7037(94)90341-7
Hartmann, J. F., Günthel, M., Klintzsch, T., Kirillin, G., Grossart, H.-P., Keppler, F., et al. (2020). High Spatiotemporal Dynamics of Methane Production and Emission in Oxic Surface Water. Environ. Sci. Technol. 54 (3), 1451–1463. doi:10.1021/acs.est.9b03182
Heiskanen, J. J., Mammarella, I., Haapanala, S., Pumpanen, J., Vesala, T., Macintyre, S., et al. (2014). Effects of Cooling and Internal Wave Motions on Gas Transfer Coefficients in a Boreal Lake. Tellus B Chem. Phys. Meteorology 66 (1), 22827. doi:10.3402/tellusb.v66.22827
Herb, W. R., and Stefan, H. G. (2005). Dynamics of Vertical Mixing in a Shallow Lake with Submersed Macrophytes. Water Resour. Res. 41 (2), 1–14. doi:10.1029/2003WR002613
Hilt, S., Brothers, S., Jeppesen, E., Veraart, A. J., and Kosten, S. (2017). Soren Brothers, Erik Jeppesen, Annelies J. Veraart, and Sarian KostenTranslating Regime Shifts in Shallow Lakes into Changes in Ecosystem Functions and Services. BioScience 67 (10), 928–936. doi:10.1093/biosci/bix106
Hilt, S. (2015). Regime Shifts between Macrophytes and Phytoplankton - Concepts beyond Shallow Lakes, Unravelling Stabilizing Mechanisms and Practical Consequences. Limnetica 34 (2), 467–480. doi:10.23818/limn.34.35
Holgerson, M. A., and Raymond, P. A. (2016). Large Contribution to Inland Water CO2 and CH4 Emissions from Very Small Ponds. Nat. Geosci. 9 (3), 222–226. doi:10.1038/ngeo2654
Jansen, J., Thornton, B. F., Cortés, A., Snöälv, J., Wik, M., MacIntyre, S., et al. (2020). Drivers of Diffusive CH<sub>4</sub> Emissions from Shallow Subarctic Lakes on Daily to Multi-Year Timescales. Biogeosciences 17 (7), 1911–1932. doi:10.5194/bg-17-1911-2020
Jeffrey, L. C., Maher, D. T., Johnston, S. G., Kelaher, B. P., Steven, A., and Tait, D. R. (2019). Wetland Methane Emissions Dominated by Plant‐mediated Fluxes: Contrasting Emissions Pathways and Seasons within a Shallow Freshwater Subtropical Wetland. Limnol. Oceanogr. 64 (5), 1895–1912. doi:10.1002/lno.11158
Jose, Pinheiro., Douglas, Bates., Saikat., Deb. Roy., and Sarkar, Deepayan.the R Core Team (2019). Linear and Nonlinear Mixed Effects Models. Available at https://cran.rproject.org/web/packages/nlme/index.html.
Kosten, S., Piñeiro, M., de Goede, E., de Klein, J., Lamers, L. P. M., and Ettwig, K. (2016). Fate of Methane in Aquatic Systems Dominated by Free-Floating Plants. Water Res. 104, 200–207. doi:10.1016/j.watres.2016.07.054
Kuznetsova, A., Brockhoff, P. B., and Christensen, R. H. B. (2017). LmerTest Package: Tests in Linear Mixed Effects Models. J. Stat. Soft. 82 (13). doi:10.18637/jss.v082.i13
Lapierre, J.-F., Guillemette, F., Berggren, M., del Giorgio, P. A., and Del Giorgio, P. A. (2013). Increases in Terrestrially Derived Carbon Stimulate Organic Carbon Processing and CO2 Emissions in Boreal Aquatic Ecosystems. Nat. Commun. 4. doi:10.1038/ncomms3972
Liptay, K., Chanton, J., Czepiel, P., and Mosher, B. (1998). Use of Stable Isotopes to Determine Methane Oxidation in Landfill Cover Soils. J. Geophys. Res. 103 (97), 8243–8250. doi:10.1029/97jd02630
MacIntyre, S., Jonsson, A., Jansson, M., Aberg, J., Turney, D. E., and Miller, S. D. (2010). Buoyancy Flux, Turbulence, and the Gas Transfer Coefficient in a Stratified Lake. Geophys. Res. Lett. 37 (24), a–n. doi:10.1029/2010GL044164
Maher, D. T., Santos, I. R., Oakes, J. M., Erler, D. V., Carvalho, M. C., and Eyre, B. D. (2013). Novel Use of Cavity Ring-Down Spectroscopy to Investigate Aquatic Carbon Cycling from Microbial to Ecosystem Scales. Environ. Sci. Technol. 47 (22), 12938–12945. doi:10.1021/es4027776
Martinsen, K. T., Kragh, T., and Sand-Jensen, K. (2020). Carbon Dioxide Efflux and Ecosystem Metabolism of Small Forest Lakes. Aquat. Sci. 82 (1). doi:10.1007/s00027-019-0682-8
McGinnis, D. F., Kirillin, G., Tang, K. W., Flury, S., Bodmer, P., Engelhardt, C., et al. (2015). Enhancing Surface Methane Fluxes from an Oligotrophic Lake: Exploring the Microbubble Hypothesis. Environ. Sci. Technol. 49 (2), 873–880. doi:10.1021/es503385d
Megonigal, J. P., Hines, M. E., and Visscher, P. T. (2003). Anaerobic Metabolism: Linkages to Trace Gases and Aerobic Processes. Treatise Geochem. 8 (9), 317–424. doi:10.1016/B0-08-043751-6/08132-9
Noyce, G. L., and Megonigal, J. P. (2021). Biogeochemical and Plant Trait Mechanisms Drive Enhanced Methane Emissions in Response to Whole-Ecosystem Warming. Biogeosciences 18 (8), 2449–2463. doi:10.5194/bg-18-2449-2021
Paranaíba, José. R., Barros, Nathan., Mendonça, Raquel., Linkhorst, Annika., Isidorova, Anastasija., Roland, Fábio., et al. (2018). Spatially Resolved Measurements of CO2 and CH4 Concentration and Gas-Exchange Velocity Highly Influence Carbon-Emission Estimates of Reservoirs. Environ. Sci. Technol. 52 (2), 607–615. doi:10.1021/acs.est.7b05138
Prairie, Y., and del Giorgio, P. (2013). A New Pathway of Freshwater Methane Emissions and the Putative Importance of Microbubbles. Iw 3 (3), 311–320. doi:10.5268/IW-3.3.542
R Core Team. (2019). R: A Language and Environment for Statistical Computing. Vienna, Austria: R Foundation for Statistical Computing.
Rantakari, M., Heiskanen, J., Mammarella, I., Tulonen, T., Linnaluoma, J., Kankaala, P., et al. (2015). Different Apparent Gas Exchange Coefficients for CO2 and CH4: Comparing a Brown-Water and a Clear-Water Lake in the Boreal Zone during the Whole Growing Season. Environ. Sci. Technol. 49 (19), 11388–11394. doi:10.1021/acs.est.5b01261
Raymond, P. A., and Cole, J. J. (2001). Gas Exchange in Rivers and Estuaries: Choosing a Gas Transfer Velocity. Estuaries 24 (2), 312–317. doi:10.2307/1352954
Rocher‐Ros, G., Sponseller, R. A., Lidberg, W., Mörth, C. M., and Giesler, R. (2019). Landscape Process Domains Drive Patterns of CO 2 Evasion from River Networks. Limnol. Oceanogr. Lett. 4 (4), 87–95. doi:10.1002/lol2.10108
Royston, J. P. (1982). An Extension of Shapiro and Wilk’s W Test for Normality to Large Samples. Source J. R. Stat. Soc. Ser. C Appl. Statistics) 31, 115–124. doi:10.2307/2347973
Sánchez, M. L., Lagomarsino, L., Allende, L., and Izaguirre, I. (2015). Changes in the Phytoplankton Structure in a Pampean Shallow Lake in the Transition from a Clear to a Turbid Regime. Hydrobiologia 752 (1), 65–76. doi:10.1007/s10750-014-2010-6
Scheffer, M., Hosper, S. H., Meijer, M. L., Moss, B., and Jeppesen, E. (1993). Alternative Equilibria in Shallow Lakes. Trends Ecol. Evol. 8 (8), 275–279. doi:10.1016/0169-5347(93)90254-M
Scheffer, M. (2001). Alternative Attractors of Shallow Lakes. Sci. World J. 1, 254–263. doi:10.1100/tsw.2001.62
Schwarz, J. I. K., Eckert, W., and Conrad., R. (2008). Response of the Methanogenic Microbial Community of a Profundal Lake Sediment (Lake Kinneret, Israel) to Algal Deposition. Limnol. Oceanogr. 53 (1), 113–121. doi:10.4319/lo.2008.53.1.0113
Sebacher, D. I., Harriss, R. C., and Bartlett, K. B. (1983). Methane Flux across the Air-Water Interface: Air Velocity Effects. Tellus 35B, 103–109. doi:10.1111/j.1600-0889.1983.tb00014.x
Soued, C., and Prairie, Y. T. (2020). The Carbon Footprint of a Malaysian Tropical Reservoir: Measured versus Modelled Estimates Highlight the Underestimated Key Role of Downstream Processes. Biogeosciences 17 (2), 515–527. doi:10.5194/bg-17-515-2020
Tan, D., Li, Q., Wang, S., Yeager, K. M., Guo, M., Liu, K., et al. (2021). Diel Variation of CH4 Emission Fluxes in a Small Artificial Lake: Toward More Accurate Methods of Observation. Sci. Total Environ. 784, 147146. doi:10.1016/j.scitotenv.2021.147146
Tang, K. W., McGinnis, D. F., Ionescu, D., and Grossart, H.-P. (2016). Methane Production in Oxic Lake Waters Potentially Increases Aquatic Methane Flux to Air. Environ. Sci. Technol. Lett. 3 (6), 227–233. doi:10.1021/acs.estlett.6b00150
Tedford, E. W., MacIntyre, S., Miller, S. D., and Czikowsky, M. J. (2014). Similarity Scaling of Turbulence in a Temperate Lake during Fall Cooling. J. Geophys. Res. Oceans 119 (8), 4689–4713. doi:10.1002/2014JC010135
Thottathil, S. D., Reis, P. C. J., del Giorgio, P. A., and Prairie, Y. T. (2018). The Extent and Regulation of Summer Methane Oxidation in Northern Lakes. J. Geophys. Res. Biogeosci. 123 (10), 3216–3230. doi:10.1029/2018JG004464
Torremorell, A., Llames, M. E., Pérez, G. L., Escaray, R., Bustingorry, J., and Zagarese, H. (2009). Annual Patterns of Phytoplankton Density and Primary Production in a Large, Shallow Lake: The Central Role of Light. Freshw. Biol. 54 (3), 437–449. doi:10.1111/j.1365-2427.2008.02119.x
Tranvik, L. J., Cole, J. J., and Prairie, Y. T. (2018). The Study of Carbon in Inland Waters-From Isolated Ecosystems to Players in the Global Carbon Cycle. Limnol. Oceanogr. Lett. 3 (3), 41–48. doi:10.1002/lol2.10068
Vachon, D., Langenegger, T., Donis, D., McGinnis, D. F., and McGinnis, D. F. (2019). Influence of Water Column Stratification and Mixing Patterns on the Fate of Methane Produced in Deep Sediments of a Small Eutrophic Lake. Limnol. Oceanogr. 64 (5), 2114–2128. doi:10.1002/lno.11172
Vagle, S., McNeil, C., and Steiner, N. (2010). Upper Ocean Bubble Measurements from the NE Pacific and Estimates of Their Role in Air-Sea Gas Transfer of the Weakly Soluble Gases Nitrogen and Oxygen. J. Geophys. Res. 115 (12), 1–16. doi:10.1029/2009JC005990
Wanninkhof, R. (2014). Relationship between Wind Speed and Gas Exchange over the Ocean Revisited. Limnol. Oceanogr. Methods 12 (JUN), 351–362. doi:10.4319/lom.2014.12.351
West, W. E., Coloso, J. J., and Jones, S. E. (2012). Effects of Algal and Terrestrial Carbon on Methane Production Rates and Methanogen Community Structure in a Temperate Lake Sediment. Freshw. Biol. 57 (5), 949–955. doi:10.1111/j.1365-2427.2012.02755.x
Whiticar, Michael. J. (1999). Carbon and Hydrogen Isotope Systematics of Bacterial Formation and Oxidation of Methane. Chem. Geol. 161 (1), 291–314. doi:10.1016/s0009-2541(99)00092-3
Yan, X., Xu, X., Ji, M., Zhang, Z., Wang, M., Wu, S., et al. (2019). Cyanobacteria Blooms: A Neglected Facilitator of CH4 Production in Eutrophic Lakes. Sci. Total Environ. 651, 466–474. doi:10.1016/j.scitotenv.2018.09.197
Keywords: methane, submerged macrophytes, gas exchange, microbubbles, turbulence, methane oxidation
Citation: Baliña S, Sánchez ML and del Giorgio PA (2022) Physical Factors and Microbubble Formation Explain Differences in CH4 Dynamics Between Shallow Lakes Under Alternative States. Front. Environ. Sci. 10:892339. doi: 10.3389/fenvs.2022.892339
Received: 08 March 2022; Accepted: 30 May 2022;
Published: 23 June 2022.
Edited by:
Daniel F. McGinnis, Université de Genève, SwitzerlandReviewed by:
Baoli Wang, Tianjin University, ChinaFrancois Clayer, Norwegian Institute for Water Research (NIVA), Norway
Copyright © 2022 Baliña, Sánchez and del Giorgio. This is an open-access article distributed under the terms of the Creative Commons Attribution License (CC BY). The use, distribution or reproduction in other forums is permitted, provided the original author(s) and the copyright owner(s) are credited and that the original publication in this journal is cited, in accordance with accepted academic practice. No use, distribution or reproduction is permitted which does not comply with these terms.
*Correspondence: Sofia Baliña, c29maWFiYWxpbmlhQGdtYWlsLmNvbQ==