- 1Department of Agricultural Sciences, University of Helsinki, Helsinki, Finland
- 2Department of Forest Sciences, University of Helsinki, Helsinki, Finland
- 3Finnish Meteorological Institute, Helsinki, Finland
- 4Institute for Atmospheric and Earth System Research (INAR) / Forest Sciences, University of Helsinki, Helsinki, Finland
- 5Natural Resources Institute Finland, Jokioinen, Finland
- 6Institute for Forest Ecology and Soils, Federal Research Centre for Forests, Vienna, Austria
- 7Viikki Plant Science Center (ViPS), University of Helsinki, Helsinki, Finland
- 8Helsinki Institute of Life Science (HiLIFE), University of Helsinki, Helsinki, Finland
Biochars have potential to provide agricultural and environmental benefits such as increasing soil carbon sequestration, crop yield, and soil fertility while reducing greenhouse gas (GHG) emissions and nitrogen leaching. However, whether these effects will sustain for the long-term is still unknown. Moreover, these effects were observed mostly in highly weathered (sub-) tropical soils with low pH and soil organic carbon (SOC). The soils in northern colder boreal regions have typically higher SOC and undergo continuous freeze-thaw cycles. Therefore, effects of biochars in these regions may be different from those observed in other climates. However, only a few biochar studies have been conducted in boreal regions. We aimed to assess the long-term effects of biochars on GHG emissions, yield-normalized non-CO2 GHG emissions (GHGI), and N dynamics in boreal soils. For this, we collected data from four existing Finnish biochar field experiments during 2018 growing season. The experiments were Jokioinen (Stagnosol), Qvidja (Cambisol), Viikki-1 (Stagnosol), and Viikki-2 (Umbrisol), where biochars were applied, 2, 2, 8, and 7 years before, respectively. The GHG emissions, crop yield, soil mineral N, and microbial biomass were measured from all fields, whereas, additional measurements of plant N contents and N leaching were conducted in Qvidja. Biochars increased CO2 efflux in Qvidja and Viikki-2, whereas, there were no statistically significant effects of biochars on the fluxes of N2O or CH4, but in Qvidja, biochars tended to reduce N2O fluxes at the peak emission points. The tendency of biochars to reduce N2O emissions seemed higher in soils with higher silt content and lower initial soil carbon. We demonstrated the long-term effects of biochar on increased crop yield by 65% and reduced GHGI by 43% in Viikki-2. In Qvidja, the significant increment of plant biomass, plant N uptake, nitrogen use efficiency, and crop yield, and reduction of NO3−–N leaching by the spruce biochar is attributed to its ability to retain NO3−–N, which could be linked to its significantly higher specific surface area. The ability of the spruce biochar to retain soil NO3−–N and hence to reduce N losses, has implications for sustainable management of N fertilization.
1 Introduction
Applying stable carbon into agricultural soils in the form of biochar has been recognized as an effective tool for enhancing carbon sequestration into the soil to mitigate climate change (Lehmann et al., 2021; Roe et al., 2021). The ability of some biochars to reduce soil GHG emissions (CO2, CH4, and N2O) has further reinforced their importance in mitigating climate change. A number of recently published meta-analyses suggest that biochars generally increase soil CO2 emissions, reduce N2O emissions, and have varying effects on CH4 emissions (Song et al., 2016; He et al., 2017; Liu et al., 2018; Borchard et al., 2019; Zhang et al., 2020; Joseph et al., 2021). Nevertheless, the direction and magnitude of the effects on GHG emissions depend on the feedstock, pyrolysis condition, application rate, soils, and climatic conditions. Some of these meta-analyses indicated that most of the data included were from laboratory and greenhouse experiments rather than from field experiments (Song et al., 2016; He et al., 2017). Moreover, the biggest reductions in GHG emissions were reported mostly in short-term laboratory studies (Qi Liu et al., 2019). Drawing conclusions from such short-term laboratory studies may be unwarranted. The reliable and practical recommendation about the use of biochars to mitigate GHG emissions should be based on long-term field experiments. However, the studies reporting the biochar effects on GHG emissions after several years of application are scarce.
There are uncertainties about the long-term effects of biochars on GHG emissions. Biochars are highly resistant to decomposition and can persist in soil for thousands of years (Kuzyakov et al., 2014). Therefore, any effects on GHG emissions could be expected to pertain for years to decades. Supporting this, Zhang et al. (2021) reported that biochars reduced N2O emission by 22–48% after 2 and 7 years in two soil types. On the other hand, some studies suggest that the biochar effects are short-lived. For example, a meta-analysis by Borchard et al. (2019) reported that N2O emission reduction by biochars becomes negligible after 1 year. In addition to this, the underlying mechanism and the key influencing factors involved behind the biochar effects on GHG emissions, following the long-term application of biochar remains elusive. This points to the need for more long-term field experiments to validate the potential of biochar in suppressing GHG emissions (Song et al., 2016; He et al., 2017; Kammann et al., 2017; Zhang et al., 2020; Shakoor et al., 2021). Moreover, the effects on GHG emissions can be expected to change over time because of field-aging of biochars. The field exposure changes the properties of biochar particles by increasing the number of oxygen-containing functional groups like carboxylic and phenolic groups on their surfaces, and therefore increasing their cation exchange capacity (Cheng et al., 2006; Mukherjee et al., 2014; Singh et al., 2014). In addition, interactions between particles of biochar and soil can enhance the formation of organo-mineral layers on biochar surfaces (Hagemann et al., 2017). These changes induced by aging processes can in turn affect N retention (Hagemann et al., 2017) and soil microbial activity (Yadav et al., 2019), which can affect soil GHG emissions.
The synthesis studies summarizing the effects of biochars have shown that they can have varying agricultural and environmental effects depending on the climatic zone. Most of the studies conducted are concentrated on tropical to temperate climates (Jeffery et al., 2017; Qi Liu et al., 2019; Ye et al., 2020; Zhang et al., 2020), whereas only a few studies are available from the northern boreal region. Additionally, the positive effects of biochars on agricultural production are more common in nutrient deficit, acidic soils in the tropics than in fertile and moderate pH soils in temperate regions (Jeffery et al., 2017; Qi Liu et al., 2019). On the other hand, the N2O reduction potential of biochars has been reported to be higher in the temperate region than in the tropical and sub-tropical regions (Qi Liu et al., 2019). However, the circumstances in boreal soils are arguably different even compared to temperate soils due to significantly higher C content (Heikkinen et al., 2021) as well as periodic freeze-thaw cycles. As a result, the previously reported effects may not be applicable in boreal regions. So far, the few studies conducted in boreal climate have demonstrated that biochars had a limited effect on agricultural crop production (Tammeorg et al., 2014a; Tammeorg et al., 2014b; Soinne et al., 2020; Kalu et al., 2021a). Nevertheless, it is imperative to study whether biochar can provide long-term climatic and environmental benefits in boreal conditions as well. The greenhouse gas intensity (GHGI), which expresses GHG emissions (as CO2-equivalents) per unit of crop yield, serves as an effective indicator for quantifying the overall climatic impacts of a chosen agricultural management practice. The GHGI helps to estimate the potential climatic impacts of changing agricultural management practices. Although the short-term effects of biochar on GHGI have been analyzed, for validating biochar soil amendments as a sustainable agricultural management tool, data from long-term field trials are necessary (Xiang Liu et al., 2019; Zhang et al., 2020).
Nitrogen fertilization is crucial for sustaining global crop production. However, N fertilization can also lead to major environmental consequences through N2O emissions and leaching of mineral N into waterways. Biochars can interact with fertilizer N by retaining mineral N through surface adsorption (Yao et al., 2012), by absorbing and holding it in its internal pores, or by modifying soil physical properties (Novak et al., 2012; Yoo et al., 2014; Rasa et al., 2018; Turunen et al., 2020). Moreover, biochars may also enhance soil microbial activity (Lehmann et al., 2011) and therefore support the immobilization of mineral N to microbial biomass (Bruun et al., 2012). Such microbial immobilization of N could also protect N from leaching (Vinten et al., 2002). Through these mechanisms, biochars may reduce gaseous (especially N2O emission) and leaching losses of fertilizer N, while also improving soil N availability and plant N uptake. This helps to both maximize N use efficiency (NUE) and to minimize N losses, which are important targets in sustainable crop production. Even so, the ability of biochars to retain N in soils remains controversial due to huge variability in the properties of both soils and biochars (Ahmad et al., 2021).
Considering the above-mentioned knowledge gaps, we conducted this study to assess whether the application of biochars would provide agricultural and environmental benefits, such as reduced GHG emissions, reduced N leaching, or increased N fertilizer use efficiency, for the sustainability of boreal agriculture in the long-term. The specific objectives were i) to investigate the effects of biochar application on emissions of CO2, CH4, and N2O, and GHGI after 2–8 years of biochar field application in four different boreal soils, ii) to examine the linkage of the biochar induced change in the GHG emissions with biochar properties (pH, C content, C:N) and soil properties (sand, silt, clay, and initial soil C content), and iii) to quantify the effect of biochar on NUE and N leaching. We hypothesized that i) biochars will decrease GHG emissions and GHGI from boreal agricultural soils in the long-term, ii) the potential of biochar to reduce GHG emissions depends on the properties of soils and biochars, and iii) biochars will improve plant N use efficiency by increasing plant N uptake and reducing fertilizer N losses via N2O emission and N leaching. For this purpose, we measured GHG emissions, and soil and plant properties from four already established boreal agricultural field experiments, where soils were amended with biochars 2–8 years ago. This is the first study that reports the GHG emissions in the long-term, 7–8 years after biochar field application in boreal regions.
2 Materials and Methods
2.1 Field Experiments and Weather Conditions
This study includes data collected during the growing season of 2018 (May–September) from four previously established biochar field experiments on different types of agricultural soils in southern Finland (see Table 1). The field experiments at Jokioinen (Stagnosol) and Qvidja (Cambisol) were shorter-term experiments where biochars were applied 2 years before in the fall of 2016 in both experiments. The other two were longer-term field experiments at Helsinki-Viikki on Stagnosol and Umbrisol, where biochars were applied eight (in May 2010) and seven (in May 2011) years before this study, respectively. From here onwards, the experiments are denoted by the name of the places as Jokioinen, Qvidja, Viiki-1 (for Stagnosol experiment) and Viikki-2 (for Umbrisol experiment). The experimental design in Jokioinen was a randomized complete block design (RCBD) with only two treatments—fertilized control and biochar treatment (30 t ha−1) with five replicates (Soinne et al., 2020). The biochar applied was produced by pyrolyzing chipped forest residue at 450°C (see Table 2). The Jokioinen field was fertilized with BioFert CAN 27 at the rate of 85 kg N ha−1 plus Yara Starttilannoite (N12-P23) at the rate of 5 kg N ha−1 in 2018. The Qvidja experiment also had RCBD design with three replicates (Karhu et al., 2021). The treatments consisted of unfertilized control, fertilized control, and various organic amendment treatments but for this study, the selected treatments were two control treatments and two biochar treatments—spruce (21 t ha−1) and willow biochar (33 t ha−1), both produced by pyrolysis at 450°C. The fertilized control and both biochar treatments received Yara Mila 3 (N23-P3-K8) fertilizer at the rate of 80 kg N ha−1 in 2018. Both Viikki fields had a split-plot design with four replicates. In Viikki-1, the main-plot factor was biochar application rate (0, 5, and 10 t ha−1) and the sub-plot factor was fertilization rate (30%, 60%, and 100% recommended fertilization rate). The biochar applied in Viikki-1 was produced by pyrolyzing debarked spruce and pine chips at 500–600°C (Tammeorg et al., 2014a). The 100% recommended fertilization included Yara Mila HEVI 6 (N13-P3-K15) at the rate of 98 kg N ha−1 in 2018 and only the 100% fertilization and 10 t ha−1 biochar +100% fertilization treatments were selected at Viikki-1. In Viikki-2, the main-plot factor was biochar application rate (0, 5, 10, 20, and 30 t ha−1) and the sub-plot factor was fertilization types (no fertilizer, meat bone meal or organic fertilizer, and mineral fertilizer). The biochar applied in Viikki-2 was produced from debarked spruce chips at 500–600°C (Tammeorg et al., 2014b). The mineral fertilizer treatments received a mixture of Yara Mila HEVI 6, Starttiravinne, liquid Mantrac, Magtrac, and Bortrac contributing a total N of 56 kg N ha−1 and only the mineral fertilizer and biochar + mineral fertilizer treatments were selected at Viikki-2. In all the fields, the fertilizers were applied at the time of sowing.
The growing season in 2018 was hotter and drier compared to the long-term average (1981–2010), especially from May to July (Supplementary Table S1; Supplementary Figure S1; FMI, 2020; Heimsch et al., 2021). The mean monthly precipitation was 17–79% lower from May to August compared to the long-term average in all studied fields. Due to extremely dry weather, the field in Qvidja was irrigated with approximately 40–50 mm of water during a period of 18 h on 30 June by using 11 rotary sprinklers per replicate block whereas no other fields were irrigated.
2.2 Greenhouse Gas Flux Measurements
The fluxes of CO2, CH4, and N2O were measured during the growing season of 2018 using a static opaque aluminum chamber (60 cm × 60 cm × 75 cm) that consisted of an inbuilt fan and a HOBO temperature logger (UA-001-64, Onset, Bourne, MA, United States). Each selected experimental plot (see Section 2.1) was equipped with one collar of 60 cm × 60 cm in dimensions, inserted into the soil to a depth of 10 cm. During gas sampling, the aluminum chamber was mounted on top of the collar, then a 20 ml gas sample was withdrawn with a syringe after 0, 10, 20, 30, and 40 min and injected into a 12 ml evacuated vial (Exetainer®, Labco Ltd., United Kingdom). The large dimension of the chamber allowed us to place it over and around the growing plants and therefore measure GHG fluxes without disturbing their growth. The gas sampling was carried out approximately once every 2 weeks starting from 5, 8, 10, and 13 days after sowing in Jokioinen, Qvidja, Viikki-1, and Viikki-2, respectively. The final measurements were carried out 84, 91, 104, and 105 days after sowing in Jokioinen, Qvidja, Viikki-1, and Viiki-2, respectively. During the flux measurements in Viikki and Jokioinen, soil temperature and moisture were measured by using Testo 720 thermometer (Testo AG, Lenzkirch, Germany; probe length 7 cm) and TRIME-FM time-domain reflectometer (IMKO, Ettlingen, Germany; probe length 10 cm), respectively. In Qvidja, soil temperature was measured using HOBO temperature loggers (UA-001-64, Onset, Bourne, MA, United States) and soil moisture was measured with ML3 ThetaProbe (Delta T Devices).
The gas samples were analyzed for their CO2, CH4, and N2O concentrations using a gas chromatograph (7890A, Agilent Technologies, California, United States) equipped with a flame ionization detector (FID) and a methanizer for measuring CO2 and CH4 concentration, and an electron capture detector (ECD) for measuring N2O concentration (Pihlatie et al., 2013). The GHG flux was calculated by fitting a linear regression to the measured gas concentrations over the measurement time.
The cumulative emissions of each individual GHG were calculated using linear interpolation as follows:
where, the units of cumulative emissions of CO2, CH4, or N2O are g CO2–C m−2, mg CH4–C m−2, mg N2O–N m−2, respectively, and Fi is GHG flux (g CO2–C m−2 d−1, mg CH4–C m−2 d−1, mg N2O–N m−2 d−1) on the day i. The time interval (ti+1 – ti) is in days. The cumulative GHG emissions were calculated for the period between the first and the final measurements.
Similarly, CO2 equivalent (kg CO2–e ha−1) and GHGI (kg CO2–e t−1 grain yield) representing the sum of cumulative CH4 and N2O emissions were calculated as reported by Yang et al. (2020) as:
where
2.3 Soil Sampling and Analysis
Soil sampling was carried out three times, approximately once a month in Jokioinen (in July, August, September) and in Viikki (in June, July, and August) fields. In Viikki-1, the crops were already harvested when the final soil sampling was carried out in August. In the Qvidja field, two additional soil sampling and subsequent analyses were carried out in May and September.
The sampling consisted of taking ten soil samples (0–10 cm) from each experimental plot, which were then pooled to form one composite sample per plot. The fresh soil was then passed through a 4 mm sieve and stored at + 4°C before analyzing their mineral nitrogen and microbial biomass contents within 1–2 days after sampling. For soil mineral nitrogen, about 5 g of fresh sieved soil was extracted with 25 ml 1 M KCl, shaken for 30 min in an orbital shaker (200 rounds per minute), and filtered through SartoriusTM Grade 3-HW folded filters (diameter 150 mm) and stored frozen (–20°C) before measuring with an automated flow analyzer Lachat QuikChem 8000 (Zellweger Analytics, Milwaukee, Wisconsin, United States). Microbial biomass C (MBC) and N (MBN) were determined using the chloroform fumigation extraction (CFE) method (Vance et al., 1987) modified by Karhu et al. (2016). In short, approximately 8 g of fresh sieved soil was fumigated with chloroform inside a desiccator for 24 h in dark and then extracted with 40 ml of 0.05 M K2SO4 by shaking in an orbital shaker (30 min, 200 rounds per minute). A parallel control sample was extracted similarly, but without fumigation. The extracts were filtered using Whatman® Grade 42, ashless filter paper and subsequently through a 0.45 µm syringe filter (Sartorius, Minisart High Flow, PES) before analyzing for dissolved organic carbon (DOC) and total nitrogen (TN) using a Shimadzu TOC–V cph/cpn analyzer (Kyoto, Japan). The MBC and MBN were calculated as the difference in DOC and TN contents in chloroform fumigated and control samples, respectively. No extraction correction factors were used for calculating MBC and MBN (Christiansen et al., 2018). The MBN could not be detected with the CFE method during June from Qvidja soil samples most probably due to extremely dry conditions, hence the MBN results from June were not presented.
2.4 Distribution of Applied Fertilizer N Into Various N Pools in Qvidja
The experimental setup in Qvidja (including an unfertilized control treatment) allowed us to calculate N use efficiency (NUE) and the distribution of applied fertilizer N in different N pools. Therefore, mineral N leaching tests and plant samplings were also carried out in Qvidja. The N leaching was measured using the resin bag method (Dannenmann et al., 2018; Karhu et al., 2021). In the selected plot, a water-permeable bag containing ion-exchange resins [6 g of Amberlite, IR 120 (Na+ – ion exchanger resin), and 6 g Dowex 1 × 8 (Cl− – ion exchanger resin)] was placed approximately 20 cm deep under the intact soil column that was cored using a 10 cm diameter PVC tube. The bags were immediately placed after sowing and fertilizer application for approximately 30 days, during which they were allowed to collect leached mineral N as water percolate through them. After about every 30 days, the resin bags were replaced with new bags. Altogether, there were 3 N leaching measurement periods during the growing season: from 15 May to 7 June, from 7 June to 5 July, and from 5 July to 23 August 2018. The resin bags loaded with leached N from the soil were then extracted with 100 ml of 1 M NaCl twice, filtered with SartoriusTM Grade 3–HW folded filter paper (diameter 150 mm), and analyzed for NH4+–N and NO3−–N concentration using the method described by Hood-Nowotny et al. (2010). The extracts were analyzed at 660 and 540 nm wavelengths for measuring NH4+–N and NO3−–N concentrations, respectively using a microplate spectrophotometer (µQuant, BioTek Instruments, Bad Friedrichshall, Germany). The cumulative NH4+–N and NO3−–N leaching was calculated by summing NH4+–N and NO3−–N leached during the 3 N leaching measurement periods.
In addition, above-ground and below-ground plant samples were taken before the harvest in August 2018. Three above ground plant samples were systematically collected from each plot by cutting the plants at the height of 2 cm from the soil surface (area = 0.0314 m2), whereas five below ground root samples per plot were taken with a soil auger (diameter 5 cm) to the depth of 20 cm. The grains were separated from the above-ground biomass to calculate the grain yield. The plant samples (grains, stems-leaves, roots) were oven-dried (60 °C) for 48 h to calculate their respective biomasses. As the roots may include mineral particles even after washing, their biomasses were calculated as the loss on ignition (2 h at 550°C). The samples of grains and stems-leaves were ground and analyzed for their N contents with a dry combustion method using the Leco CN828 CN analyzer, LECO Corporation, United States (Matejovic, 1996). The root samples were not available for N analysis because of their destruction during the loss of ignition. An average N content calculated from grains and stems-leaves was used to represent root N content. Total plant N uptake was calculated by multiplying respective biomasses of grains, stems-leaves, and roots with their N contents, then summing them up. NUE (%) was calculated as follows:
where NUT is the total N uptake in a treated plot (kg ha−1), NUC is the average of total N uptake in the unfertilized control plot (kg ha−1), and NA is the amount of N fertilizer applied (kg ha−1).
The distribution of applied fertilizer N (NF¸ kg ha−1) was calculated as the difference between the N pool in the fertilized treatments (NT, kg ha−1) and the average value representing the N pool in unfertilized control (NC, kg ha−1).
2.5 Statistical Analysis
The difference in means of the measured parameters was analyzed using a linear mixed-effects model with treatment (Treat) × Measurement time (Time) as fixed factors and replicated block as a random factor in the R environment (R Core Team, 2020). Similarly, the differences in means of cumulative GHG emissions, crop yield, CO2 equivalent (CH4 and N2O), GHGI, plant N uptake, NF, and NUE among the treatments in different fields were analyzed using a linear mixed-effects model with treatment (Treat) as a fixed factor and replicated block as a random factor. The assumptions of normality and homogeneity of variances were tested using the Shapiro-Wilk test (on the residuals of the model) and Levene’s test, respectively. Whenever the assumptions were violated, the Box-Cox transformation was applied (Box and Cox, 1964). Since Box-Cox transformation is a power transformation, direct transformation of data with negative values, especially for negative CH4 and N2O emissions, was not possible. So, a minimum value was added to the data set with negative values so that all the values were positive before the transformation. Post hoc tests were computed to find the differences in means between the treatments (in each measurement time) using “emmeans” function under “emmeans” package (Lenth, 2019) with the Tukey method for p-value adjustments and a significance level of p < 0.05 specified in the “cld” function under “multcompView” package (Graves et al., 2019). Also, Pearson correlation analysis was carried out to relate cumulative emissions of gases and other measured parameters. The plot-wise average values over the growing season for the soil mineral N and microbial biomass contents and the cumulative amount of N leached were used for the correlation analysis. The cumulative GHG emissions, soil mineral N, and microbial biomass were available from all four fields for correlation analysis. In addition, cumulative N leaching and plant N uptake from Qvidja were also included in the correlation analysis.
The relative difference in emissions of CO2 (ΔCO2), CH4 (ΔCH4), and N2O (ΔN2O) between biochar and (fertilized) control treatments were calculated as in Eq. 5. The positive values indicate that biochar treatments increased the emissions, whereas the negative values indicate that biochar decreased the emissions of CO2, CH4, or N2O.
where, EBC is the cumulative emissions of CO2, CH4, or N2O in the biochar treatments and EFC is the average cumulative emissions of CO2, CH4, or N2O in the (fertilized) control treatment. The relationship between ΔCO2 or ΔN2O or ΔCH4 and inherent soil properties (such as sand, silt, clay, and initial soil C contents) and biochar properties (such as biochar pH, biochar C content, and C:N) were analyzed using redundancy analysis (RDA) and Pearson correlation analysis. All the data were analyzed at a significance level of 5%.
3 Results
3.1 Greenhouse Gas Emissions and Greenhouse Gas Intensity
Overall, the biochar treatments tended to increase CO2 fluxes while having only a moderate effect on N2O fluxes. The most notable effects on CO2 fluxes were observed in Qvidja and Viikki-2. In Qvidja, the spruce biochar significantly increased average CO2 flux compared to the unfertilized control (p = 0.043), whereas both the spruce and willow biochar treatments tended to increase average CO2 flux compared to the fertilized control (p < 0.10; Figure 1; Supplementary Tables S2, S3). Similarly, the biochar used in Viikki-2 significantly increased the average CO2 flux (p = 0.004). According to the linear mixed effect model, the treatments had a significant effect on N2O flux in Qvidja (p = 0.047). However, the post hoc test revealed that the differences in average N2O flux between the treatments were not statistically significant even though the fertilized control treatment showed notably higher average N2O flux than the spruce biochar (by 75%–76%) and the willow biochar treatments (by 88%–98%) in the final two measurements (collected on 30 July and 14 August; Figure 1F).
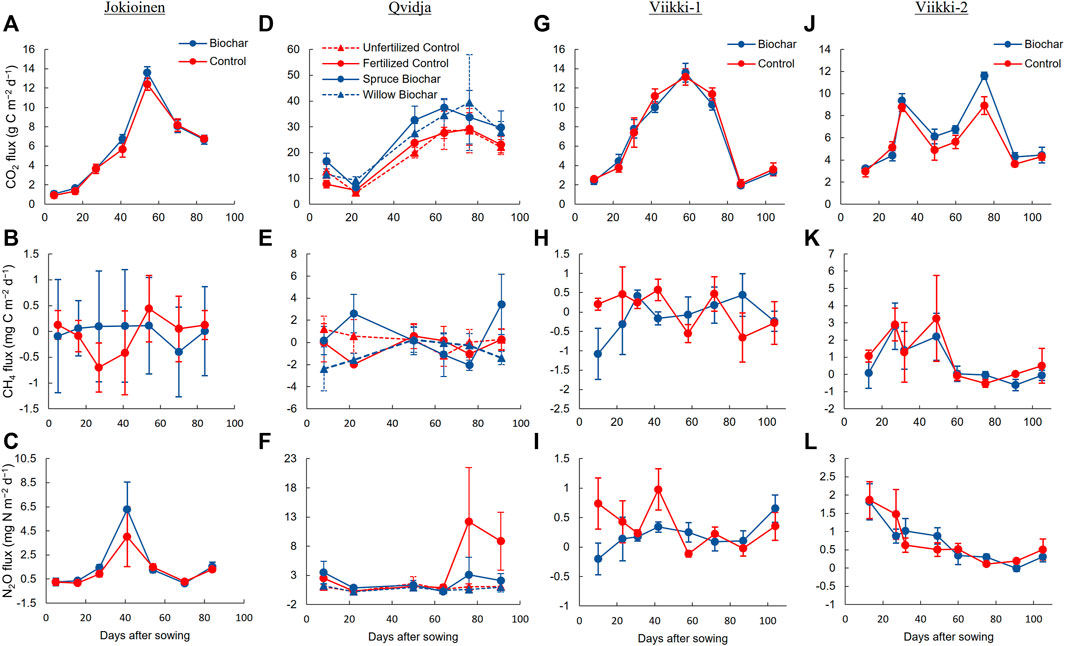
FIGURE 1. The fluxes of CO2, CH4 and N2O (average ± SE) in control and biochar treatments in Jokioinen (A–C), Qvidja (D–F), Viikki-1 (G–I) and Viikki-2 (J–L) fields over the measurement period in 2018.
Biochar had no significant effects on the cumulative GHG emissions in any of the fields (Table 3; Supplementary Table S4). The cumulative CO2 emission was relatively higher in Qvidja than in other fields. In accordance with the CO2 flux, the spruce biochar treatment tended to have higher cumulative CO2 emissions compared to both the fertilized (p = 0.067) and the unfertilized treatments (p = 0.118) in Qvidja. No significant effects of biochar were observed on GHG fluxes and cumulative GHG emissions in Jokioinen and Viikki-1.
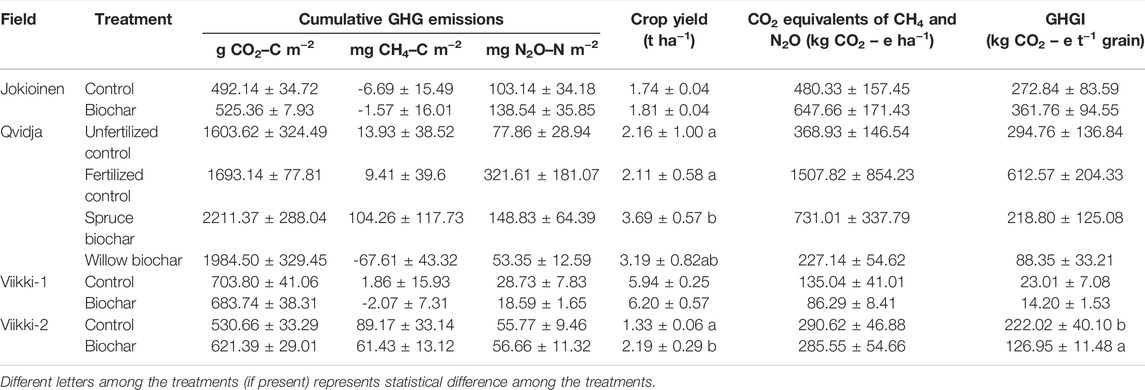
TABLE 3. Cumulative GHG emissions, crop yield, CO2 equivalents (of CH4 and N2O) and greenhouse gas intensity (GHGI) in control and biochar treatments. The numbers represent average ± SE.
In Qvidja, the spruce biochar treatment had a higher crop grain yield compared to both the fertilized (p = 0.044) and the unfertilized controls (p = 0.049). Similarly, the biochar treatment significantly increased crop grain yield in Viikki-2 by 65% (p = 0.025). However, no effects of biochar on crop yield were observed in Jokioinen or Viikki-1. The biochar treatment significantly reduced yield normalized emissions of non-CO2 GHG (GHGI) by 43% in Viikki-2 (p = 0.015). Other than that, the biochar treatments had no significant effects on CO2 equivalent (CH4 and N2O) or GHGI in any of the fields (Table 3; Supplementary Table S4). However, the average CO2 equivalent (CH4 and N2O) and GHGI values in the biochar treatments were noticeably lower than those in the (fertilized) control treatments in Qvidja and Viikki-1.
3.2 Microbial Biomass and Soil Mineral N
Overall, the biochars had only a minor effect on MBC and MBN (Figure 2; Supplementary Tables S5, S6). The significant effects and notable trends observed in each experimental field, and/or at specific sampling points are as follows. In Qvidja, the linear mixed model indicated (p = 0.049) that willow biochar had the highest average MBN followed by spruce biochar, then fertilized control, and finally unfertilized control (Supplementary Table S6). However, the post hoc test showed these differences not to be significant. In Viikki-1, the biochar treatment significantly reduced MBC (p = 0.004). The reduction in MBC was significant only in the last measurement point in August after harvesting (Figure 2I). In contrast, MBN was significantly reduced by the biochar treatments during the first measurement in June (Figure 2J).
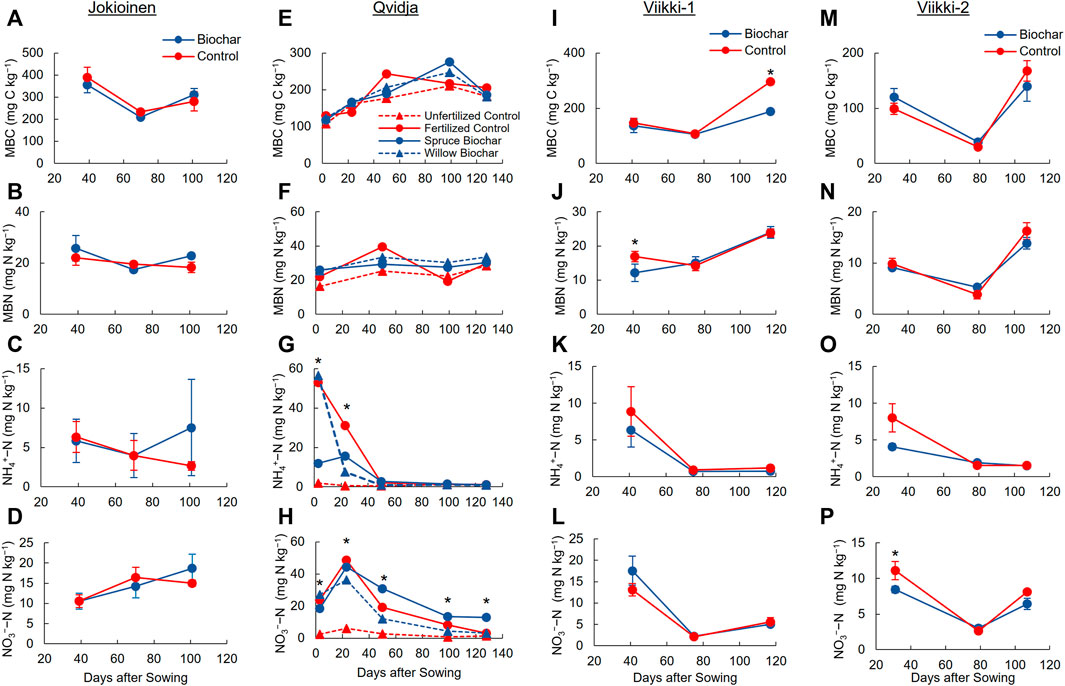
FIGURE 2. The microbial biomass C (MBC) and N (MBN), and soil mineral N contents (average ± SE) in control and biochar treatments in Jokioinen (A–D), Qvidja (E–H), Viikki-1 (I–L) and Viikki-2 (M–P) fields over the growing season of 2018. The error bars are not shown for Qvidja results to avoid noisy figure. * The effects of treatment was significant at the sampling point (p < 0.05).
In Qvidja, the spruce biochar treatment had significantly higher average soil NO3−–N content than the other treatments, but the differences in soil NO3−–N content between the fertilized control and the willow biochar treatment were not significant (Figure 2H; Supplementary Table S6). In Viikki-2, biochar significantly reduced average soil NO3−–N content, mostly during the first measurement in June (Figure 2P; Supplementary Table S6).
3.3 N Leaching, N Uptake, and NUE in Qvidja
Both biochar treatments (spruce and willow) had higher average N uptake, total plant biomass, and NUE than the fertilized control, but the differences were statistically significant only for the spruce biochar treatment (Table 4; Supplementary Table S7). Most of the N leaching occurred in the form of NO3−–N. No treatment effects were observed in NH4+–N leaching. Both biochar treatments significantly reduced average NO3−–N leaching compared to the fertilized control (Supplementary Table S6). However, the difference in cumulative NO3−–N leaching between fertilized control and biochar treatments was not statistically significant (Table 4) even though the cumulative NO3−–N leaching was 61% and 52% lower in the spruce and willow biochar treatments, respectively, compared to the fertilized control. Out of the three leaching measurement periods, the significant reduction of NO3−–N leaching was observed only during the second measurement period from 7 June 2018 to 5 July 2018 (Figure 3B), when NO3−–N leaching was highest in the fertilized control treatment, and thus biochar treatment effects were clearly observed. The NO3−–N leaching from the fertilized control was comparatively small in the beginning (from 15 May 2018–7 June 2018) and the end (5 July 2018–23 August 2018) of the growing season and the difference to the biochar treatments were smaller and statistically non-significant.

TABLE 4. Cumulative N leaching, plant N content, total plant biomass, plant N uptake and nitrogen use efficiency (NUE) in Qvidja field. The numbers represent average ± SE.
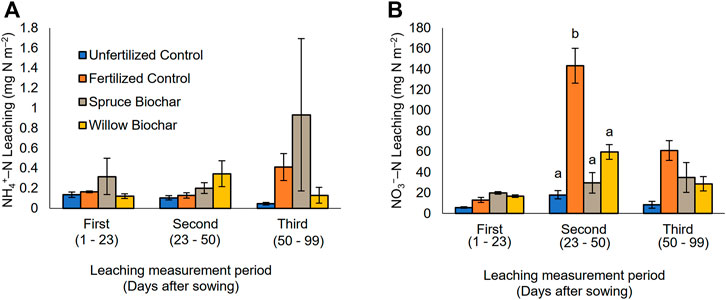
FIGURE 3. Leaching of NH4+–N (A) and NO3−–N (B) (average ± SE) measured over the growing season 2018 in Qvidja field. The first, second, and third measurement periods were from 15 May 2018 to 7 June 2018, from 7 June 2018 to 5 July 2018, and from 5 July 2018 to 23 August 2018, respectively. The different letters at a measurement time (if present) represent the statistical difference among the treatments.
We estimated the apparent distribution of the fertilizer N (80 kg N ha−1) into different N pools such as plant biomass, soil mineral N, soil MBN, N leached, and N2O emitted among the fertilized treatments and tested the differences in their means between the treatments in Qvidja field (Table 5). In general, most of the fertilizer N was recovered by plants (27–80%), while a low amount was lost through N leaching (<1–2%) and N2O emissions (<1–4%). The amounts of fertilizer N taken up by plants in both biochar treatments were higher than in the fertilized control, but statistical significance was found only for the spruce biochar treatment (p < 0.05). Also, the spruce biochar treatment had a significantly higher amount of fertilizer N retained in the soil as mineral N (17%) compared to the fertilized control (3%) and the willow biochar treatment (2%). On the other hand, the willow biochar treatment had a significantly higher amount of fertilizer N retained in microbial biomass (11%) compared to the fertilized control (3%) and the spruce biochar treatment (3%). The amount of the fertilizer N lost through N leaching and N2O emissions was higher in the fertilized control compared to the biochar treatments even though the differences were not statistically significant. The average recovery of fertilizer N, determined as the sum of all measured N pools were 31%, 77%, and 101% in the fertilized control, willow biochar, and spruce biochar treatments, respectively.

TABLE 5. Apparent distribution of applied fertilized N (80 kg N ha−1) in different measured N pools (average ± SE) among the fertilized treatments in Qvidja.
3.4 Relationship Between Greenhouse Gas Emissions and Other Measured Parameters
Most notably, the CO2 emissions correlated positively with MBN, soil mineral N, plant biomass, and plant N uptake (p < 0.01; Table 6). In addition, there were significant positive correlations between N2O emissions and soil NH4+–N and NO3−–N contents (p < 0.001). In addition, plant N uptake was positively correlated with MBN and soil NO3−–N content (p < 0.05). Whereas, no significant correlation was observed between CH4 emissions and other measured parameters. The soil temperature or moisture contents were not significantly different between the treatments in any of the fields. In addition, they were not significantly correlated with GHG fluxes (data not shown).
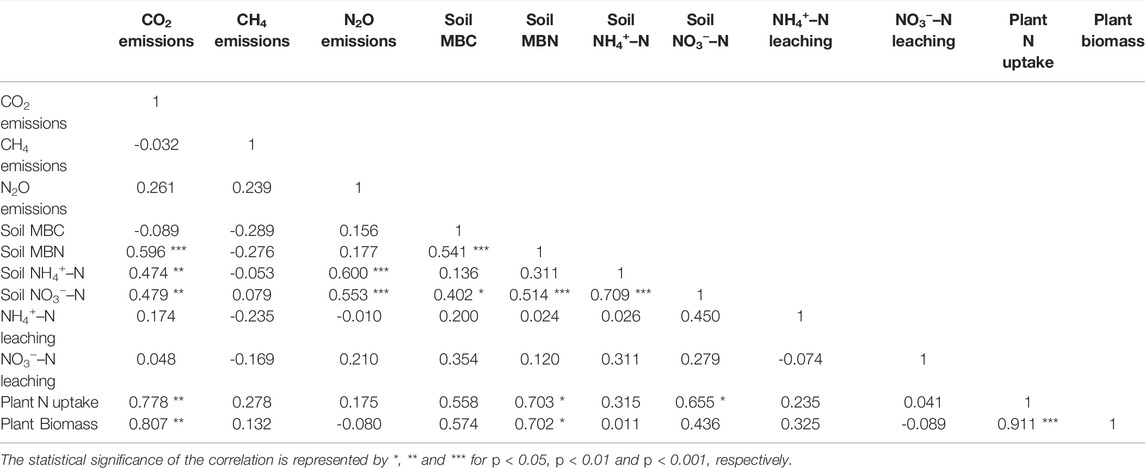
TABLE 6. Pearson correlation matrix between cumulative GHG emission and other measured parameters. The data of mineral N leaching, N uptake and plant biomass were from only Qvidja field, whereas all the data of other parameters were from all the fields combined.
Redundancy analysis showed that the relative difference in N2O emissions between biochar and fertilized control treatments (ΔN2O) was positively associated with initial soil C and negatively associated with silt content (Figure 4). Similar result was observed with the simple linear regression as ΔN2O was positively correlated with intial soil C content (p = 0.004), while negatively correlated with soil silt content (p = 0.009) (Figure 5). We found no significant relationships between ΔCO2 or ΔCH4 and soil or biochar properties (Figure 4; Supplementary Table S8).
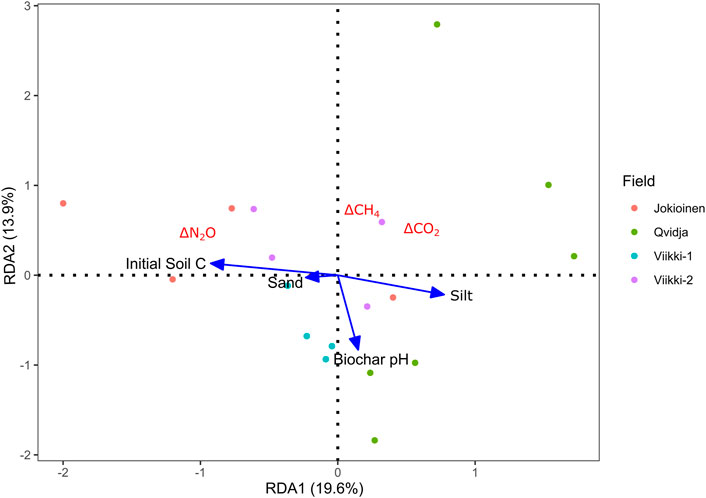
FIGURE 4. Ordination plot of redundancy analysis (RDA) conducted on the response variables (△CO2, △CH4, and △N2O) and the explanatory variables (biochar and soil characteristics).
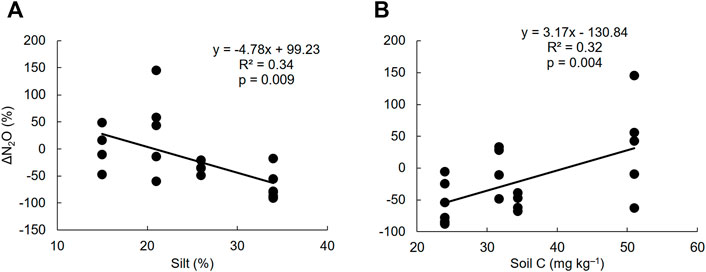
FIGURE 5. Simple linear regression between the relative difference in N2O emissions (ΔN2O) and soil silt content (A) and initial soil C content (B). The negative ΔN2O values indicate decrease in N2O emissions and positive ΔN2O values indicate increase in N2O emissions in biochar treatment compared to (fertilized) control treatment.
4 Discussion
We aimed to test the potential benefits of biochar soil amendments on reducing GHG emissions and their relation with different soil and biochar properties, as well as to investigate the potential of biochar to improve the N use efficiency of fertilizer N in Finnish agricultural soils. We did not observe clear effects of biochar on GHG emissions. Large variability in estimating the field emissions of GHGs resulting from greater soil heterogeneity within fields contributes to the lack of clear significant effects of biochar in field conditions (Hüppi et al., 2015; Kammann et al., 2017). It should be noted that the growing season of 2018, when the GHG emissions were measured, was exceptionally dry (Buras et al., 2020; Bastos et al., 2021). There was almost no rain for 30 days after the fertilization, especially in Jokioinen and Qvidja fields (Supplementary Figure S1). Therefore, our results represent a situation during drought, which is expected to become more common in the future due to climate change (Veijalainen et al., 2019). It is therefore possible that our results could underestimate the biochar effects on GHG emissions (especially N2O) as well as on N leaching during an “average” growing season.
4.1 Greenhouse Gas Emissions and Greenhouse Gas Intensity
The evidence in support of the first hypothesis is limited, as biochar had only minor effects on GHG emissions and reduced GHGI in only one out of the four long-term experimental sites. The tendency of biochar to increase CO2 efflux, observed in Qvidja and Viikki-2, was likely due to increased soil microbial activity as well as plant growth. There was a positive correlation between soil MBN and CO2 emissions (Table 6), suggesting that enhanced CO2 efflux could be the result of enhanced soil microbial biomass. Biochar usually enhances soil microbial activity by acting as a source of organic C in the short-term (Smith et al., 2010; Luo et al., 2011) and modifying the soil physical and hydrological properties, thereby providing suitable microbial habitats in the long-term (Hardy et al., 2019). We noticed slightly higher MBN in biochar treatments in Qvidja whereas biochar had no effects on MBC and MBN in Viikki-2 (Supplementary Tables S5, S6). However, the soil microbial biomass pool may not necessarily represent soil microbial activity and soil respiration, which may be determined by substrate availability (Wang et al., 2003). Apart from this, our measurement technique also captured CO2 emitted from plant above-ground and root respiration. Since biochar increased plant biomass (measured in Qvidja only) and grain yield in Qvidja and Viikki-2, the increased plant growth and thus increased plant respiration probably contributed to the higher CO2 efflux, which is also supported by the significant positive correlation between plant biomass and CO2 emissions (Table 6). Based on the data, we cannot partition the sources of CO2 for estimating the contribution of plant respiration and increased microbial activity to the increased CO2 efflux from biochar amended soils at these sites. The relatively higher cumulative CO2 emissions in Qvidja than other fields may be due to irrigation of fields in Qvidja that could have helped in increasing plant growth as well as soil microbial activity.
No significant effects of biochar on N2O emissions were observed at any of the four fields. The special dry year probably reduced N2O emissions in general (Garcia-Montiel et al., 2003; Yan et al., 2018), and thus reduced the possibility of detecting significant biochar effects on N2O emissions. However, our overall results are in line with other field studies carried out previously on boreal soils, where no significant effects of biochar soil amendments on N2O emissions have been detected (Karhu et al., 2011; Kalu et al., 2021a; Grau-Andrés et al., 2021).
In Qvidja, the N2O fluxes drastically increased in the fertilized control during the last two measurements (measured on 30 July and 14 August; Figure 1F). The reason for these high episodes of N2O fluxes could be related to weather conditions, as the latter part of the growing season was wetter than the beginning (Supplementary Table S1; Supplementary Figure S1), creating favorable conditions for N2O production (Griffis et al., 2017). The peak N2O emissions usually occur right after fertilization (Weitz et al., 2001; Harter et al., 2014) and after a rapid increase in water-filled pore space, which occurs after irrigation and high rainfall events (Trost et al., 2013; Barrat et al., 2020). Due to the extremely dry conditions at the beginning of the growing season, a significant amount of the fertilizers might have become available to plants and (denitrifying) microbes only during the latter growing season, resulting in peak N2O emissions from the fertilized control treatment late in the summer. Both the spruce and willow biochars tended to reduce N2O fluxes only during these measurements, which agrees with the result of Hüppi et al. (2016), who also observed the effectiveness of biochar to suppress N2O emissions, especially at the peak emission points. Our results are also in line with a parallel incubation study, in which soils from the same Qvidja field were incubated at different moisture levels (Peltokangas et al., Unpublished). Also in that study, it was found that both the biochar treatments significantly reduced N2O emissions mainly at the highest moisture level, i.e., 100% water holding capacity. However, in our case, there was a lack of statistical significance probably due to highly variable inherent soil properties and environmental conditions within the experimental fields (Karhu et al., 2021). There might be a possibility to detect clearer differences in the (Qvidja) N2O fluxes in a wetter growing season based on the parallel incubation study. However, a comparison to a laboratory study highlights the difficulty in catching the episodes of peak N2O emissions in field experiments, where the conditions with high enough water contents (nearly 100% water holding capacity) are transient or might only be occurring at some micro-sites or hotspots. Such highly dynamic and episodic fluxes could be difficult to catch with manual chamber measurements with the sampling frequency used in this study. Therefore, more intense measurements with online GHG flux measuring systems would be helpful to capture peak emissions and to get a better insight into the GHG reduction potential of biochar.
The significant reduction of GHGI in Viikki-2 illustrates the potential of biochar in reducing yield-normalized non-CO2 GHG emissions even in the long-term from boreal soils. This reduction seems to be mainly due to increased crop yield because the biochar significantly increased crop yield, but had no effects on the emissions of N2O or CH4. The increase in the crop yield in Viikki-2 could be due to the coarse-textured soil because the effectiveness of biochars in increasing crop yield is usually better in those types of soils (Farhangi-Abriz et al., 2021). The increased crop yield could be associated with enhanced soil water retention during the extremely dry growing season in 2018 because the biochar increased plant available water at the top soil in the first year of application in 2011 (Tammeorg et al., 2014b). With time, this effect seemed to disappear (Tammeorg et al., 2014b; Kalu et al., 2021a) probably due to the filling of internal pores of the biochar with clay and soil organic matter (Wang et al., 2019), or loss of some the biochar from the topsoil through mineralization or downward movement (Kätterer et al., 2019). However, during the long duration of the extreme dry period, biochars could enhance the formation of narrow and medium pores that enhances higher water retention and increase the rigidity of coarse-textured soils that reduces rapid water loss under drying conditions (Villagra-Mendoza and Horn, 2018).
4.2 Key Factors Influencing the Effects of Biochar on Greenhouse Gas Emissions
We observed that relative differences in N2O emissions between biochar and control treatments (ΔN2O) significantly correlated with some soil properties, supporting our second hypothesis. The ΔN2O was negatively correlated with soil silt content, while positively correlated with initial soil C content (Figures 4, 5). This indicates that biochar has a relatively higher potential to reduce N2O emissions in soils with higher silt contents and lower C contents. Although the difference was not statistically significant, the average cumulative N2O emissions of biochar treatments in Qvidja and Viikki-1 fields, where the soils had higher silt contents (Table 1), were remarkably lower compared to those of the fertilized control treatments (Table 3). Our results agree with the earlier finding by Qi Liu et al. (2019) that the reduction in soil N2O emissions by biochar tends to occur mostly in finely textured loam soils because these soils are favorable for denitrification. Similarly, Hüppi et al. (2016) also observed that the reduction in soil N2O emissions after the application of biochar was higher in silty soil than in sandy soil. A decrease in soil N2O emissions by biochar is usually common in such silty soils due to the ability of biochar to regulate the denitrification process (Qi Liu et al., 2019). On the other hand, we found the potential of biochar to increase N2O emissions is higher in soils with higher C content. This could be associated with the potential of biochar to have a positive priming effect i.e. increased mineralization of native soil organic matter (Luo et al., 2011; Singh and Cowie, 2014). The N bound in organic matter may become available for microbes during mineralization and can eventually be released as N2O via nitrification and denitrification processes (Kammann et al., 2017; Guenet et al., 2020).
4.3 Nitrogen Use Efficiency in Qvidja
We found support for our third hypothesis that biochar soil application increased plant N use efficiency. In Qvidja, the spruce biochar treatment increased soil NUE, plant biomass, and crop yield. The increased NUE and crop yield could be related to the ability of the spruce biochar to retain NO3−–N. The spruce biochar treatment resulted in better plant growth as indicated by higher plant biomass. This higher plant biomass lead to higher plant N uptake, as plant N content was not significantly different between treatments (Table 4). And, plant N uptake was positively correlated with soil NO3−–N content (Table 6), which supports the idea that at least part of the higher amount of soil NO3−–N retained in the spruce biochar treatment was available to plants. These findings are in line with previous studies, e.g., Haider et al. (2020), who reported that the biochar retained NO3−–N is available for plant uptake. Moreover, Kalu et al. (2021b) also demonstrated that biochar enhanced the plant uptake of applied fertilizer NO3−–N. We previously also found that the spruce biochar treatments reduced NO3−–N leaching in the growing season of 2017, and retained higher soil NO3−–N contents in spring before the growing season of 2018 (Karhu et al., 2021). This indicates that the retained soil NO3−–N in the spruce biochar is safe from leaching during the winter and hence accumulated over the next growing season. This also explains the reason behind more than 100% fertilizer N recovery in the measured N pools in the spruce biochar treatment. The general positive correlation between N2O emissions and soil NO3−–N content across all data (Table 6) suggests that the amount of N2O emissions is related to the amount of soil NO3−–N content (Russow et al., 2008). However, despite higher soil NO3−–N content in the spruce biochar treatment, we did not observe increased N2O emissions rather the cumulative N2O emissions were decreased (even though the differences were not statistically significant). This indicates that the soil NO3−–N retained in the spruce biochar treatment could be unavailable for the denitrifying microbes (Chintala et al., 2013; Kammann et al., 2015; Haider et al., 2016).
The willow biochar treatment increased the retention of fertilizer N in soil MBN (Table 5). It can be also noticed that soil MBN (averaged over the growing season) was relatively higher in the willow biochar treatment compared to other treatments although not statistically significant (Supplementary Table S6). Moreover, similar higher soil MBN values were observed in the willow biochar treatment in May 2018 samples (statistically insignificant; Karhu et al., 2021) and in October 2018 samples (statistically significant; Kenneth et al., Unpublished). This indicates the ability of the willow biochar to increase microbial immobilization of fertilizer N. Usually, the presence of labile C in biochar promotes N immobilization, but this effect has been reported to prevail only in the short-term (Bruun et al., 2012; Nelissen et al., 2014). However, even after 2 years, we observed an indication of increased MBN in the willow biochar treatment. The microbial N can be later re-mineralized (Vinten et al., 2002; Li et al., 2021), and become available to plants, which is why we assume it was correlated with plant N uptake and plant biomass in Qvidja (Table 6).
Similar to the results of N2O emissions, biochars did not have a significant effect on cumulative NO3−–N leaching, but biochar significantly reduced NO3−–N leaching during the second measurement period (from 7 June to 5 July). The extreme dry weather might have contributed to concealing the biochar effects on NO3−–N leaching. Usually, during an “average” growing season, high NO3−–N leaching can be expected immediately after fertilizer application before plants are able to utilize fertilizer N (Riley et al., 2001). However, because of extremely dry conditions, most of the applied fertilizer granules were not even dissolved during the first N leaching measurement period (15 May–7 June). While during the second N leaching measurement period, the fields were irrigated and the first significant rainfall of the growing season occurred during this period (Supplementary Figure S1). Therefore, NO3−–N leaching from the fertilized control treatment was high during this period and both the biochar treatments were able to significantly reduce NO3−–N leaching (Figure 3). This suggests that the potential of biochar to reduce NO3−–N leaching is high especially during conditions when high NO3−–N leaching can be expected. This potential of biochars to reduce N losses (via reduction in N2O emissions and NO3−–N leaching), especially during the time of peak N2O emissions or highest nitrogen leaching period provided more fertilizer N available for plant uptake (Table 5), thereby increasing the NUE.
5 Conclusion
No clear effects of wood-based biochars were observed on the fluxes of CH4 or N2O from boreal agricultural soils. However, biochars tended to reduce N2O emissions at one out of the four fields when the environmental conditions favored high N2O emissions suggesting that biochars are effective in reducing N2O emissions mostly at peak emission points. Such peak emissions usually occur after rainfall. However, the gas flux measurements were carried out during an extremely dry growing season, which might have underestimated the true potential of biochar to reduce N2O emissions. This point towards the necessity of continuous GHG measurements that span over multiple years. The potential of biochar to reduce N2O emissions seems to be greater in soils with higher silt content and lower soil C content. The increased crop yield and reduced yield-normalized emissions of non-CO2 GHG (CH4 and N2O) from coarse-textured soil after 7 years of biochar application indicate the potential of wood-based biochar to enhance crop production even in the long-term without increasing the emissions of CH4 or N2O to the atmosphere.
Our results may interest the farmers because in a site with more detailed measurements, the spruce biochar increased plant N uptake, NUE, crop biomass, and grain yield after 2 years of application. This agronomic benefit of the spruce biochar is related to its ability to retain and accumulate soil NO3−–N over the growing season, which is safe from leaching and denitrification. This ability of the spruce biochar suggests that it could be possible to reduce the amount of N fertilization below the recommended levels and still allow sustained crop yields, but this would need to be further verified.
Data Availability Statement
The raw data supporting the conclusion of this article will be made available by the authors, without undue reservation.
Author Contributions
SK and KK planned the study. PT, KR, and LK were responsible for the maintenance of the field experiments. JZ, LK, and SK participated in gas sampling. MP contributed in analyzing gas samples. SK and KP conducted soil mineral N and microbial biomass analysis. BK was responsible for the leaching data. SK calculated the results and wrote the first manuscript draft. KK was responsible for funding acquisition. All co-authors commented on the manuscript.
Funding
This study was funded by the University of Helsinki 3-years grant (Decision number HY/66/05.01.07/2017), Helsinki Institute of Life Science (HiLIFE), The Strategic Research Council at the Academy of Finland (decisions 327214 and 327342), Academy of Finland (Grant number 316401), and Maj and Tor Nessling Foundation.
Conflict of Interest
The authors declare that the research was conducted in the absence of any commercial or financial relationships that could be construed as a potential conflict of interest.
Publisher’s Note
All claims expressed in this article are solely those of the authors and do not necessarily represent those of their affiliated organizations, or those of the publisher, the editors and the reviewers. Any product that may be evaluated in this article, or claim that may be made by its manufacturer, is not guaranteed or endorsed by the publisher.
Acknowledgments
We would like to thank Rashmi Shrestha for helping in analyzing microbial biomass samples, Brigitte Schraufstädter for measuring mineral N concentration from resin bags, and Outi-Maaria Sietiö for helping with statistics.
Supplementary Material
The Supplementary Material for this article can be found online at: https://www.frontiersin.org/articles/10.3389/fenvs.2022.914766/full#supplementary-material
References
Ahmad, Z., Mosa, A., Zhan, L., and Gao, B. (2021). Biochar Modulates Mineral Nitrogen Dynamics in Soil and Terrestrial Ecosystems: A Critical Review. Chemosphere 278, 130378. doi:10.1016/j.chemosphere.2021.130378
Barrat, H. A., Evans, J., Chadwick, D. R., Clark, I. M., Le Cocq, K., and Cardenas, L. (2020). The Impact of Drought and Rewetting on N2O Emissions from Soil in Temperate and Mediterranean Climates. Eur. J. Soil. Sci. 72, 2504–2516. doi:10.1111/ejss.13015
Bastos, A., Orth, R., Reichstein, M., Ciais, P., Viovy, N., Zaehle, S., et al. (2021). Vulnerability of European Ecosystems to Two Compound Dry and Hot Summers in 2018 and 2019. Earth Syst. Dynam. 12, 1015–1035. doi:10.5194/esd-12-1015-2021
Borchard, N., Schirrmann, M., Cayuela, M. L., Kammann, C., Wrage-Mönnig, N., Estavillo, J. M., et al. (2019). Biochar, Soil and Land-Use Interactions that Reduce Nitrate Leaching and N2O Emissions: A Meta-Analysis. Sci. Total Environ. 651, 2354–2364. doi:10.1016/j.scitotenv.2018.10.060
Box, G. E. P., and Cox, D. R. (1964). An Analysis of Transformations. J. R. Stat. Soc. Ser. B Methodol. 26 (2), 211–243. doi:10.1111/j.2517-6161.1964.tb00553.x
Bruun, E. W., Ambus, P., Egsgaard, H., and Hauggaard-Nielsen, H. (2012). Effects of Slow and Fast Pyrolysis Biochar on Soil C and N Turnover Dynamics. Soil Biol. Biochem. 46, 73–79. doi:10.1016/j.soilbio.2011.11.019
Buras, A., Rammig, A., and Zang, C. S. (2020). Quantifying Impacts of the 2018 Drought on European Ecosystems in Comparison to 2003. Biogeosciences 17, 1655–1672. doi:10.5194/bg-17-1655-2020
Cheng, C.-H., Lehmann, J., Thies, J. E., Burton, S. D., and Engelhard, M. H. (2006). Oxidation of Black Carbon by Biotic and Abiotic Processes. Org. Geochem. 37 (11), 1477–1488. doi:10.1016/j.orggeochem.2006.06.022
Chintala, R., Mollinedo, J., Schumacher, T. E., Papiernik, S. K., Malo, D. D., Clay, D. E., et al. (2013). Nitrate Sorption and Desorption in Biochars from Fast Pyrolysis. Microporous Mesoporous Mater. 179, 250–257. doi:10.1016/j.micromeso.2013.05.023
Christiansen, C. T., Lafreniére, M. J., Henry, G. H. R., and Grogan, P. (2018). Long-term Deepened Snow Promotes Tundra Evergreen Shrub Growth and Summertime Ecosystem Net CO2 Gain but Reduces Soil Carbon and Nutrient Pools. Glob. Change Biol. 24 (8), 3508–3525. doi:10.1111/gcb.14084
Dannenmann, M., Díaz-Pinés, E., Kitzler, B., Karhu, K., Tejedor, J., Ambus, P., et al. (2018). Postfire Nitrogen Balance of Mediterranean Shrublands: Direct Combustion Losses versus Gaseous and Leaching Losses from the Postfire Soil Mineral Nitrogen Flush. Glob. Change Biol. 24 (10), 4505–4520. doi:10.1111/gcb.14388
Farhangi-Abriz, S., Torabian, S., Qin, R., Noulas, C., Lu, Y., and Gao, S. (2021). Biochar Effects on Yield of Cereal and Legume Crops Using Meta-Analysis. Sci. Total Environ. 775, 145869. doi:10.1016/j.scitotenv.2021.145869
FMI (2020). Normal Period 1981-2010. Finnish Meteorological Institute. Available at: https://en.ilmatieteenlaitos.fi/normal-period-1981-2010 (Accessed October 12, 2020).
Garcia-Montiel, D. C., Steudler, P. A., Piccolo, M., Neill, C., Melillo, J., and Cerri, C. C. (2003). Nitrogen Oxide Emissions Following Wetting of Dry Soils in Forest and Pastures in Rondônia, Brazil. Biogeochemistry 64 (3), 319–336. doi:10.1023/A:1024968802018
Grau‐Andrés, R., Pingree, M. R. A., Öquist, M. G., Wardle, D. A., Nilsson, M. C., and Gundale, M. J. (2021). Biochar Increases Tree Biomass in a Managed Boreal Forest, but Does Not Alter N2O, CH4, and CO2 Emissions. GCB Bioenergy 13 (8), 1329–1342. doi:10.1111/gcbb.12864
Graves, S., Piepho, H.-P., Selzer, L., and Dorai-Raj, S. (2019). multcompView: Visualizations of Paired Comparisons. Retrived from: https://CRAN.R-project.org/web/packages/multcompView/index.html (Accessed April 6, 2022).
Griffis, T. J., Chen, Z., Baker, J. M., Wood, J. D., Millet, D. B., Lee, X., et al. (2017). Nitrous Oxide Emissions Are Enhanced in a Warmer and Wetter World. Proc. Natl. Acad. Sci. U.S.A. 114 (45), 12081–12085. doi:10.1073/pnas.1704552114
Guenet, B., Gabrielle, B., Chenu, C., Arrouays, D., Balesdent, J., Bernoux, M., et al. (2020). Can N 2 O Emissions Offset the Benefits from Soil Organic Carbon Storage? Glob. Change Biol. 27 (2), 237–256. doi:10.1111/gcb.15342
Hagemann, N., Joseph, S., Schmidt, H.-P., Kammann, C. I., Harter, J., Borch, T., et al. (2017). Organic Coating on Biochar Explains its Nutrient Retention and Stimulation of Soil Fertility. Nat. Commun. 8 (1), 1089. doi:10.1038/s41467-017-01123-0
Haider, G., Steffens, D., Müller, C., and Kammann, C. I. (2016). Standard Extraction Methods May Underestimate Nitrate Stocks Captured by Field-Aged Biochar. J. Environ. Qual. 45 (4), 1196–1204. doi:10.2134/jeq2015.10.0529
Haider, G., Joseph, S., Steffens, D., Müller, C., Taherymoosavi, S., Mitchell, D., et al. (2020). Mineral Nitrogen Captured in Field-Aged Biochar Is Plant-Available. Sci. Rep. 10 (1), 13816. doi:10.1038/s41598-020-70586-x
Hardy, B., Sleutel, S., Dufey, J. E., and Cornelis, J.-T. (2019). The Long-Term Effect of Biochar on Soil Microbial Abundance, Activity and Community Structure Is Overwritten by Land Management. Front. Environ. Sci. 7, 110. doi:10.3389/fenvs.2019.00110
Harter, J., Krause, H.-M., Schuettler, S., Ruser, R., Fromme, M., Scholten, T., et al. (2014). Linking N2O Emissions from Biochar-Amended Soil to the Structure and Function of the N-Cycling Microbial Community. ISME J. 8 (3), 660–674. doi:10.1038/ismej.2013.160
He, Y., Zhou, X., Jiang, L., Li, M., Du, Z., Zhou, G., et al. (2017). Effects of Biochar Application on Soil Greenhouse Gas Fluxes: a Meta-Analysis. GCB Bioenergy 9 (4), 743–755. doi:10.1111/gcbb.12376
Heikkinen, J., Keskinen, R., Regina, K., Honkanen, H., and Nuutinen, V. (2021). Estimation of Carbon Stocks in Boreal Cropland Soils ‐ Methodological Considerations. Eur. J. Soil. Sci. 72 (2), 934–945. doi:10.1111/ejss.13033
Heimsch, L., Lohila, A., Tuovinen, J.-P., Vekuri, H., Heinonsalo, J., Nevalainen, O., et al. (2021). Carbon Dioxide Fluxes and Carbon Balance of an Agricultural Grassland in Southern Finland. Biogeosciences 18 (11), 3467–3483. doi:10.5194/bg-18-3467-2021
Hood-Nowotny, R., Umana, N. H.-N., Inselbacher, E., Oswald- Lachouani, P., and Wanek, W. (2010). Alternative Methods for Measuring Inorganic, Organic, and Total Dissolved Nitrogen in Soil. Soil Sci. Soc. Am. J. 74 (3), 1018–1027. doi:10.2136/sssaj2009.0389
Hüppi, R., Felber, R., Neftel, A., Six, J., and Leifeld, J. (2015). Effect of Biochar and Liming on Soil Nitrous Oxide Emissions from a Temperate Maize Cropping System. Soil 1 (2), 707–717. doi:10.5194/soil-1-707-2015
Hüppi, R., Neftel, A., Lehmann, M. F., Krauss, M., Six, J., and Leifeld, J. (2016). N Use Efficiencies and N2O Emissions in Two Contrasting, Biochar Amended Soils under Winter Wheat—Cover Crop—Sorghum Rotation. Environ. Res. Lett. 11 (8), 084013. doi:10.1088/1748-9326/11/8/084013
Jeffery, S., Abalos, D., Prodana, M., Bastos, A. C., van Groenigen, J. W., Hungate, B. A., et al. (2017). Biochar Boosts Tropical but Not Temperate Crop Yields. Environ. Res. Lett. 12 (5), 053001. doi:10.1088/1748-9326/aa67bd
Joseph, S., Cowie, A. L., Van Zwieten, L., Bolan, N., Budai, A., Buss, W., et al. (2021). How Biochar Works, and when it Doesn't: A Review of Mechanisms Controlling Soil and Plant Responses to Biochar. GCB Bioenergy 13, 1731–1764. doi:10.1111/gcbb.12885
Kätterer, T., Roobroeck, D., Andrén, O., Kimutai, G., Karltun, E., Kirchmann, H., et al. (2019). Biochar Addition Persistently Increased Soil Fertility and Yields in Maize-Soybean Rotations over 10 Years in Sub-humid Regions of Kenya. Field Crops Res. 235, 18–26. doi:10.1016/j.fcr.2019.02.015
Kalu, S., Simojoki, A., Karhu, K., and Tammeorg, P. (2021a). Long-Term Effects of Softwood Biochar on Soil Physical Properties, Greenhouse Gas Emissions and Crop Nutrient Uptake in Two Contrasting Boreal Soils. Agric. Ecosyst. Environ. 316, 107454. doi:10.1016/j.agee.2021.107454
Kalu, S., Oyekoya, G. N., Ambus, P., Tammeorg, P., Simojoki, A., Pihlatie, M., et al. (2021b). Effects of Two Wood-Based Biochars on the Fate of Added Fertilizer Nitrogen—A 15N Tracing Study. Biol. Fertil. Soils 57, 457–470. doi:10.1007/s00374-020-01534-0
Kammann, C. I., Schmidt, H.-P., Messerschmidt, N., Linsel, S., Steffens, D., Müller, C., et al. (2015). Plant Growth Improvement Mediated by Nitrate Capture in Co-composted Biochar. Sci. Rep. 5 (1), 11080. doi:10.1038/srep11080
Kammann, C., Ippolito, J., Hagemann, N., Borchard, N., Cayuela, M. L., Estavillo, J. M., et al. (2017). Biochar as a Tool to Reduce the Agricultural Greenhouse-Gas Burden - Knowns, Unknowns and Future Research Needs. J. Environ. Eng. Landsc. Manag. 25 (2), 114–139. doi:10.3846/16486897.2017.1319375
Karhu, K., Mattila, T., Bergström, I., and Regina, K. (2011). Biochar Addition to Agricultural Soil Increased CH4 Uptake and Water Holding Capacity – Results from a Short-Term Pilot Field Study. Agric. Ecosyst. Environ. 140 (1), 309–313. doi:10.1016/j.agee.2010.12.005
Karhu, K., Hilasvuori, E., Fritze, H., Biasi, C., Nykänen, H., Liski, J., et al. (2016). Priming Effect Increases with Depth in a Boreal Forest Soil. Soil Biol. Biochem. 99, 104–107. doi:10.1016/j.soilbio.2016.05.001
Karhu, K., Kalu, S., Seppänen, A., Kitzler, B., and Virtanen, E. (2021). Potential of Biochar Soil Amendments to Reduce N Leaching in Boreal Field Conditions Estimated Using the Resin Bag Method. Agric. Ecosyst. Environ. 316, 107452. doi:10.1016/j.agee.2021.107452
Kuzyakov, Y., Bogomolova, I., and Glaser, B. (2014). Biochar Stability in Soil: Decomposition during Eight Years and Transformation as Assessed by Compound-specific 14C Analysis. Soil Biol. Biochem. 70, 229–236. doi:10.1016/j.soilbio.2013.12.021
Lehmann, J., Rillig, M. C., Thies, J., Masiello, C. A., Hockaday, W. C., and Crowley, D. (2011). Biochar Effects on Soil Biota - A Review. Soil Biol. Biochem. 43 (9), 1812–1836. doi:10.1016/j.soilbio.2011.04.022
Lehmann, J., Cowie, A., Masiello, C. A., Kammann, C., Woolf, D., Amonette, J. E., et al. (2021). Biochar in Climate Change Mitigation. Nat. Geosci. 14, 883–892. doi:10.1038/s41561-021-00852-8
Lenth, R. (2019). Emmeans: Estimated Marginal Means Aka Least-Squares Means. Retrived from: https://CRAN.R-project.org/package=emmeans (Accessed April 6, 2022).
Li, Z., Zeng, Z., Song, Z., Wang, F., Tian, D., Mi, W., et al. (2021). Vital Roles of Soil Microbes in Driving Terrestrial Nitrogen Immobilization. Glob. Change Biol. 27 (9), 1848–1858. doi:10.1111/gcb.15552
Liu, Q., Zhang, Y., Liu, B., Amonette, J. E., Lin, Z., Liu, G., et al. (2018). How Does Biochar Influence Soil N Cycle? A Meta-Analysis. Plant Soil 426 (1), 211–225. doi:10.1007/s11104-018-3619-4
Luo, Y., Durenkamp, M., De Nobili, M., Lin, Q., and Brookes, P. C. (2011). Short Term Soil Priming Effects and the Mineralisation of Biochar Following its Incorporation to Soils of Different pH. Soil Biol. Biochem. 43 (11), 2304–2314. doi:10.1016/j.soilbio.2011.07.020
Matejovic, I. (1996). The Application of Dumas Method for Determination of Carbon, Nitrogen, and Sulphur in Plant Samples. Rostl. Vyroba 42 (7), 313–316.
Mukherjee, A., Zimmerman, A. R., Hamdan, R., and Cooper, W. T. (2014). Physicochemical Changes in Pyrogenic Organic Matter (Biochar) after 15 Months of Field Aging. Solid earth. 5 (2), 693–704. doi:10.5194/se-5-693-2014
Nelissen, V., Saha, B. K., Ruysschaert, G., and Boeckx, P. (2014). Effect of Different Biochar and Fertilizer Types on N2O and NO Emissions. Soil Biol. Biochem. 70, 244–255. doi:10.1016/j.soilbio.2013.12.026
Novak, J. M., Busscher, W. J., Watts, D. W., Amonette, J. E., Ippolito, J. A., Lima, I. M., et al. (2012). Biochars Impact on Soil-Moisture Storage in an Ultisol and Two Aridisols. Soil Sci. 177 (5), 310–320. doi:10.1097/SS.0b013e31824e5593
Pihlatie, M. K., Christiansen, J. R., Aaltonen, H., Korhonen, J. F. J., Nordbo, A., Rasilo, T., et al. (2013). Comparison of Static Chambers to Measure CH4 Emissions from Soils. Agric. For. Meteorol. 171-172, 124–136. doi:10.1016/j.agrformet.2012.11.008
Liu, Q., Liu, B., Zhang, Y., Hu, T., Lin, Z., Liu, G., et al. (2019). Biochar Application as a Tool to Decrease Soil Nitrogen Losses (NH3 Volatilization, N2O Emissions, and N Leaching) from Croplands: Options and Mitigation Strength in a Global Perspective. Glob. Change Biol. 25 (6), 2077–2093. doi:10.1111/gcb.14613
R Core Team (2020). R: A Language and Environment for Statistical Computing. Vienna, Austria: R Foundation for Statistical Computing. Available at: https://www.R-project.org/.
Rasa, K., Heikkinen, J., Hannula, M., Arstila, K., Kulju, S., and Hyväluoma, J. (2018). How and Why Does Willow Biochar Increase a Clay Soil Water Retention Capacity? Biomass Bioenergy 119, 346–353. doi:10.1016/j.biombioe.2018.10.004
Riley, W. J., Ortiz-Monasterio, I., and Matson, P. A. (2001). Nitrogen Leaching and Soil Nitrate, Nitrite, and Ammonium Levels under Irrigated Wheat in Northern Mexico. Nutr. Cycl. Agroecosyst. 61 (3), 223–236. doi:10.1023/A:1013758116346
Roe, S., Streck, C., Beach, R., Busch, J., Chapman, M., Daioglou, V., et al. (2021). Land-based Measures to Mitigate Climate Change: Potential and Feasibility by Country. Glob. Change Biol. 27 (23), 6025–6058. doi:10.1111/gcb.15873
Russow, R., Spott, O., and Stange, C. F. (2008). Evaluation of Nitrate and Ammonium as Sources of NO and N2O Emissions from Black Earth Soils (Haplic Chernozem) Based on 15N Field Experiments. Soil Biol. Biochem. 40 (2), 380–391. doi:10.1016/j.soilbio.2007.08.020
Shakoor, A., Arif, M. S., Shahzad, S. M., Farooq, T. H., Ashraf, F., Altaf, M. M., et al. (2021). Does Biochar Accelerate the Mitigation of Greenhouse Gaseous Emissions from Agricultural Soil? - A Global Meta-Analysis. Environ. Res. 202, 111789. doi:10.1016/j.envres.2021.111789
Singh, B. P., and Cowie, A. L. (2014). Long-term Influence of Biochar on Native Organic Carbon Mineralisation in a Low-Carbon Clayey Soil. Sci. Rep. 4 (1), 3687. doi:10.1038/srep03687
Singh, B., Fang, Y., Cowie, B. C. C., and Thomsen, L. (2014). NEXAFS and XPS Characterisation of Carbon Functional Groups of Fresh and Aged Biochars. Org. Geochem. 77, 1–10. doi:10.1016/j.orggeochem.2014.09.006
Smith, J. L., Collins, H. P., and Bailey, V. L. (2010). The Effect of Young Biochar on Soil Respiration. Soil Biol. Biochem. 42 (12), 2345–2347. doi:10.1016/j.soilbio.2010.09.013
Soinne, H., Keskinen, R., Heikkinen, J., Hyväluoma, J., Uusitalo, R., Peltoniemi, K., et al. (2020). Are There Environmental or Agricultural Benefits in Using Forest Residue Biochar in Boreal Agricultural Clay Soil? Sci. Total Environ. 731, 138955. doi:10.1016/j.scitotenv.2020.138955
Song, X., Pan, G., Zhang, C., Zhang, L., and Wang, H. (2016). Effects of Biochar Application on Fluxes of Three Biogenic Greenhouse Gases: a Meta-Analysis. Ecosyst. Health Sustain. 2 (2), e01202. doi:10.1002/ehs2.1202
Tammeorg, P., Simojoki, A., Mäkelä, P., Stoddard, F. L., Alakukku, L., and Helenius, J. (2014a). Biochar Application to a Fertile Sandy Clay Loam in Boreal Conditions: Effects on Soil Properties and Yield Formation of Wheat, Turnip Rape and Faba Bean. Plant Soil 374 (1), 89–107. doi:10.1007/s11104-013-1851-5
Tammeorg, P., Simojoki, A., Mäkelä, P., Stoddard, F. L., Alakukku, L., and Helenius, J. (2014b). Short-term Effects of Biochar on Soil Properties and Wheat Yield Formation with Meat Bone Meal and Inorganic Fertiliser on a Boreal Loamy Sand. Agric. Ecosyst. Environ. 191, 108–116. doi:10.1016/j.agee.2014.01.007
Tammeorg, P., Parviainen, T., Nuutinen, V., Simojoki, A., Vaara, E., and Helenius, J. (2014c). Effects of Biochar on Earthworms in Arable Soil: Avoidance Test and Field Trial in Boreal Loamy Sand. Agric. Ecosyst. Environ. 191, 150–157. doi:10.1016/j.agee.2014.02.023
Trost, B., Prochnow, A., Drastig, K., Meyer-Aurich, A., Ellmer, F., and Baumecker, M. (2013). Irrigation, Soil Organic Carbon and N2O Emissions. Agron. Sustain. Dev. 33 (4), 733–749. doi:10.1007/s13593-013-0134-0
Turunen, M., Hyväluoma, J., Heikkinen, J., Keskinen, R., Kaseva, J., Hannula, M., et al. (2020). Quantifying the Pore Structure of Different Biochars and Their Impacts on the Water Retention Properties of Sphagnum Moss Growing Media. Biosyst. Eng. 191, 96–106. doi:10.1016/j.biosystemseng.2020.01.006
Vance, E. D., Brookes, P. C., and Jenkinson, D. S. (1987). An Extraction Method for Measuring Soil Microbial Biomass C. Soil Biol. Biochem. 19 (6), 703–707. doi:10.1016/0038-0717(87)90052-6
Veijalainen, N., Ahopelto, L., Marttunen, M., Jääskeläinen, J., Britschgi, R., Orvomaa, M., et al. (2019). Severe Drought in Finland: Modeling Effects on Water Resources and Assessing Climate Change Impacts. Sustainability 11 (8), 2450. doi:10.3390/su11082450
Villagra-Mendoza, K., and Horn, R. (2018). Effect of Biochar Addition on Hydraulic Functions of Two Textural Soils. Geoderma 326, 88–95. doi:10.1016/j.geoderma.2018.03.021
Vinten, A., A., W., J., B., R., H., and F., W. (2002). Factors Affecting N Immobilisation/mineralisation Kinetics for Cellulose-, Glucose- and Straw-Amended Sandy Soils. Biol. Fertil. Soils 36 (3), 190–199. doi:10.1007/s00374-002-0524-y
Wang, W. J., Dalal, R. C., Moody, P. W., and Smith, C. J. (2003). Relationships of Soil Respiration to Microbial Biomass, Substrate Availability and Clay Content. Soil Biol. Biochem. 35 (2), 273–284. doi:10.1016/S0038-0717(02)00274-2
Wang, D., Li, C., Parikh, S. J., and Scow, K. M. (2019). Impact of Biochar on Water Retention of Two Agricultural Soils - A Multi-Scale Analysis. Geoderma 340, 185–191. doi:10.1016/j.geoderma.2019.01.012
Weitz, A. M., Linder, E., Frolking, S., Crill, P. M., and Keller, M. (2001). N2O Emissions from Humid Tropical Agricultural Soils: Effects of Soil Moisture, Texture and Nitrogen Availability. Soil Biol. biochem. 33 (7), 1077–1093. doi:10.1016/S0038-0717(01)00013-X
Liu, X., Mao, P., Li, L., and Ma, J. (2019). Impact of Biochar Application on Yield-Scaled Greenhouse Gas Intensity: A Meta-Analysis. Sci. Total Environ. 656, 969–976. doi:10.1016/j.scitotenv.2018.11.396
Yadav, V., Jain, S., Mishra, P., Khare, P., Shukla, A. K., Karak, T., et al. (2019). Amelioration in Nutrient Mineralization and Microbial Activities of Sandy Loam Soil by Short Term Field Aged Biochar. Appl. Soil Ecol. 138, 144–155. doi:10.1016/j.apsoil.2019.01.012
Yan, Y., Ganjurjav, H., Hu, G., Liang, Y., Li, Y., He, S., et al. (2018). Nitrogen Deposition Induced Significant Increase of N2O Emissions in an Dry Alpine Meadow on the Central Qinghai–Tibetan Plateau. Agric. Ecosyst. Environ. 265, 45–53. doi:10.1016/j.agee.2018.05.031
Yang, W., Feng, G., Miles, D., Gao, L., Jia, Y., Li, C., et al. (2020). Impact of Biochar on Greenhouse Gas Emissions and Soil Carbon Sequestration in Corn Grown under Drip Irrigation with Mulching. Sci. Total Environ. 729, 138752. doi:10.1016/j.scitotenv.2020.138752
Yao, Y., Gao, B., Zhang, M., Inyang, M., and Zimmerman, A. R. (2012). Effect of Biochar Amendment on Sorption and Leaching of Nitrate, Ammonium, and Phosphate in a Sandy Soil. Chemosphere 89 (11), 1467–1471. doi:10.1016/j.chemosphere.2012.06.002
Ye, L., Camps‐Arbestain, M., Shen, Q., Lehmann, J., Singh, B., and Sabir, M. (2020). Biochar Effects on Crop Yields with and without Fertilizer: A Meta-Analysis of Field Studies Using Separate Controls. Soil Use Manage 36 (1), 2–18. doi:10.1111/sum.12546
Yoo, G., Kim, H., Chen, J., and Kim, Y. (2014). Effects of Biochar Addition on Nitrogen Leaching and Soil Structure Following Fertilizer Application to Rice Paddy Soil. Soil Sci. Soc. Am. J. 78 (3), 852–860. doi:10.2136/sssaj2013.05.0160
Zhang, Q., Xiao, J., Xue, J., and Zhang, L. (2020). Quantifying the Effects of Biochar Application on Greenhouse Gas Emissions from Agricultural Soils: a Global Meta-Analysis. Sustainability 12 (8), 3436. doi:10.3390/su12083436
Keywords: biochar, greenhouse gas emissions, nitrate, nitrous oxide, nitrogen leaching, nitrogen uptake
Citation: Kalu S, Kulmala L, Zrim J, Peltokangas K, Tammeorg P, Rasa K, Kitzler B, Pihlatie M and Karhu K (2022) Potential of Biochar to Reduce Greenhouse Gas Emissions and Increase Nitrogen Use Efficiency in Boreal Arable Soils in the Long-Term. Front. Environ. Sci. 10:914766. doi: 10.3389/fenvs.2022.914766
Received: 07 April 2022; Accepted: 25 April 2022;
Published: 17 May 2022.
Edited by:
Lanfang Han, Guangdong University of Technology, ChinaReviewed by:
Xinhou Zhang, Nanjing Normal University, ChinaShah Fahad, The University of Haripur, Pakistan
Copyright © 2022 Kalu, Kulmala, Zrim, Peltokangas, Tammeorg, Rasa, Kitzler, Pihlatie and Karhu. This is an open-access article distributed under the terms of the Creative Commons Attribution License (CC BY). The use, distribution or reproduction in other forums is permitted, provided the original author(s) and the copyright owner(s) are credited and that the original publication in this journal is cited, in accordance with accepted academic practice. No use, distribution or reproduction is permitted which does not comply with these terms.
*Correspondence: Subin Kalu, c3ViaW4ua2FsdUBoZWxzaW5raS5maQ==