- 1Scottish Association for Marine Science, Scottish Marine Institute, Oban, United Kingdom
- 2Marine Scotland Science, Aberdeen, United Kingdom
Various field methods have been used globally in an attempt to understand and quantify plastic pollution. However, in regions, such as the west coast of Scotland, sparse populations, combined with complex coastlines of numerous islands, sea lochs and headlands, has resulted in limited field data. The Clyde Sea is the most populated and industrialised region on the west coast of Scotland and therefore a potential source of land-based plastic litter to the less populated coast to the north. This study first presents an analysis of Marine Conservation Society (MCS) citizen-science beach-clean data, from 1994 to 2019, revealing spatial patterns between beach-clean sites. Plastic litter was categorised into land, marine and unknown sources, with the most common items in these three categories being crisp packets, fishing rope and fragments, respectively. On the west coast of Scotland there is on average 380.3 ± 419.9 plastic items per 100 m of coast, with the site average number of items recovered ranging from 1–2,355 per 100 m of coast. To simulate marine plastic litter movement from the Clyde Sea to a defined northwest model sub-area, an unstructured-grid hydrodynamic model was coupled with a particle tracking model subject to currents, diffusion, and wind. Three coastal boundary conditions were used to compare transport paths with or without particle beach resuspension, and for the resuspending cases, with or without a distinction between coastal type (retentive beaches versus reflective rocky coasts). Of the total released particles, the percentage that beached within the model sub-area, after a 1-year model run, ranged between 45.7% and 88.3% depending on the coastal boundary condition. The Clyde Sea was found to be a potential source of beached land-based plastic litter to the north, as on average, 6.8% (range: 2.9%–11.7%) of particles exited the Clyde Sea, crossed a defined northern boundary, and beached on the northwest coast. Both hydrodynamic and particle tracking models were tested, and the varying boundary conditions were compared to investigate holistic methodologies to better understand plastic pollution.
1 Introduction
The problem of plastic litter has been exacerbated by economic growth, and in particular a sharp-rise in single-use plastics due to modern ‘throw-away’ cultures (Jambeck et al., 2015; Lau, 2020), and increasingly “on-the-go” lifestyles (Williams and Rangel-Buitrago, 2019). Plastic is the most used manufacturing material (Topcu et al., 2013), with approximately 60%–80% of world litter being plastic (Derraik, 2002). As a result of this, and its persistence, plastic is now widely abundant in the marine environment (Derraik, 2002; Thompson et al., 2009; Turrell, 2018). It has been estimated that 4.8–12.7 million tons of plastic waste enters the ocean globally each year, contributing to at least 5.25 trillion plastic particles floating on the ocean’s surface (Jambeck et al., 2015).
Plastic has a slow rate of degradation due to its durability, which permits these synthetic polymers to withstand the harsh pressures of the marine environment for years, decades or even longer (Law et al., 2010). Many plastic items are highly buoyant, and are easily dispersed across vast distances throughout the ocean by hydrodynamic and meteorological conditions (Ryan, 2015).
In the past decade, the attention paid to plastic pollution has rapidly increased with numerous studies investigating the occurrence of plastic litter in the marine environment (e.g., Law et al., 2010; Isobe et al., 2014; Ryan, 2015; Woodall et al., 2015; Courtene-Jones et al., 2017; Siegfried et al., 2017; Courtene-Jones et al., 2019; Jalon-Rojas et al., 2019; Jamieson et al., 2019). Estimates of plastic mass based on surface sampling have been reported to differ by multiple orders of magnitude from what is predicted from input estimates, indicating large quantities of missing plastic that are not present at the surface. It has recently been proposed that missing plastic is predominantly nearshore or beached (Daily et al., 2021). Various field methods have been developed to quantify and document the abundance and composition of plastic litter in the ocean and on foreshores. For example, the use of visual or trawl surveys (e.g., Suaria et al., 2015; Ruiz-Orejon et al., 2016; Lebreton et al., 2018), or citizen-science beach-clean survey methods (e.g., Williams et al., 2016; Nelms et al., 2017; Watts et al., 2017), or unmanned aerial vehicles (UAV’s) (e.g., Martin et al., 2017; Andriolo et al., 2021; Cocking et al., 2022). However, while beach monitoring methods are effective, they do not provide information on the variability of the flux into and out of a region, or the transport trajectories (Turrell, 2020a). This knowledge is essential for clean-up strategies to target areas highly impacted by plastic litter.
Originating from sources both on land and at sea, beached litter can comprise a wide range of materials, including plastic, metal, wood, rubber, glass, and paper (Nelms et al., 2017; Turrell, 2019; Morales-Caselles et al., 2021). Many studies (e.g., Derraik, 2002; Topcu et al., 2013; Nelms et al., 2017; Lebreton and Andrady, 2019; Turrell, 2019; Morales-Caselles et al., 2021; Smith and Turrell, 2021; Damian et al., 2022) have shown that litter composition is dominated by plastic, because of its buoyancy and persistence (Topcu et al., 2013). According to the OSPAR commission (OSPAR, Commission, 2019), up to 90% of the items found on beaches in the OSPAR Maritime Area were plastic. On assessment of 12 million litter items, collected from seven major environments globally, Morales-Caselles et al. (2021), found that 80% of items were made of plastic, with 95% found in surface waters, and 83% on shorelines. Turrell (2019) also reports that in terms of litter composition, foreshores on the west coast of Scotland, a region with predominant onshore winds, had the greatest percentage of plastic (84% in embayments and 72% for open coast foreshores). Likewise, Nelms et al. (2017) found that plastic represented 66% of beached litter found on the UK coastline.
A recent study by Meijer et al. (2021) estimated that between 0.8 and 2.7 million tons of plastic waste currently enters the ocean per year from rivers, with the top 20 polluting rivers located in Asia, accounting for 67% of the total global river input. Following on from this, Turrell (2020a), modelled the influx of land-based plastics scaled by population size and catchment area for Scotland. The results suggest that >90% of macroplastic in Scottish seas is from littering and enters the sea through riverine input. This also suggests that population density, proximity to the source, industrialisation, and rainfall rates are all substantial factors in river-based inputs to the ocean.
The quantification of beached plastic litter is complex. Once a particle enters the ocean it is exposed to horizontal and vertical advection, diffusion, (e.g., Critchell and Lambrechts, 2016) beaching, and deposition in coastal and benthic sediments (e.g., Zhang, 2017; Turrell, 2018). The variability of deposition is influenced by fluctuating hydrodynamic factors, e.g., the prevalence of offshore/onshore winds, the suspension and resuspension by rising and falling tides (e.g., Turrell, 2018), as well as the impact of in-situ fragmentation (Smith and Turrell, 2021), driven by physical processes such as, currents, wind, Ekman transport and wave-induced Stokes’ drift (e.g., Dobler et al., 2019; Onink et al., 2019; van Sebille et al., 2020). Wind, in particular, could have a significant impact on floating litter transport, and is represented by the intensity of wind drag on the exposed part of a floating object (Onink et al., 2019). As the wind drag is dependent on surface exposure, it is extremely variable and would evidently be greater for highly buoyant objects (Critchell et al., 2015; Chubarenko et al., 2016).
The Atlantic coast of Scotland is a region of complex hydrography due to its prevailing onshore westerly winds (Turrell, 2019), a coastal current (Simpson and Hill, 1986), a variety of tidal ranges, and intense weather conditions throughout a typical year (Sabatino et al., 2016). The Scottish Coastal Current (SCC) receives contributions from rivers and sea-lochs, notably the Firth of Clyde and the Firth of Lorn (Jones et al., 2019), with on average, a net rate movement to the north of ∼5 kmd−1 (Simpson and Hill, 1986). On entering the Little Minch, part of the flow turns westward, and continues southwards along the east coast of the Outer Hebrides before proceeding northwards again up the west coast of the Outer Hebrides (Simpson and Hill, 1986). In the North Channel, the Islay Front marks the transition from the coastal region, an area of strong tides and relatively shallow water, to stratified oceanic water to the west (MacKay and Baxter, 1986).
A key tool that has been widely explored in scientific literature to investigate plastic litter transport is hydrodynamic modelling coupled with particle tracking models (e.g., Lebreton et al., 2012; Critchell et al., 2015; Critchell and Lambrechts, 2016; Hardesty et al., 2017; Siegfried et al., 2017; Gutow et al., 2018; Jalon-Rojas et al., 2019; van der Mheen et al., 2019; van der Mheen et al., 2020). This method provides an opportunity to both understand the dynamics of floating plastic litter and predict beach loadings (Critchell and Lambrechts, 2016; Turrell, 2018). Particle tracking models use simulated current velocities to advect particles around a model grid. The rate of change of the particle’s position can be dependent on surface current velocity (advection), a stochastic term representing horizontal turbulent diffusion, wind, and wave effects.
Previous studies, for example, Onink et al. (2021), have found through modelling that 77% of floating marine plastic litter released from land-based sources was either beached or floating in coastal waters within 5 years of entering the ocean. In addition, Lebreton and Andrady (2019) estimated that 67% of global plastic waste ends up on beaches. A simulation of floating marine plastic litter by Liubartseva et al. (2016), found that the coastline of the River Delta in Italy, receives an onshore flux of approximately 70 kg/km/day−1.
An original hypothesis-driven model, Varying Wind and Water Levels (VaWWL), was developed by Turrell (2018) to simulate how wind-blown marine litter ends up on foreshores. The model demonstrated that persistent strandlines of beached litter can form high up on the beach after onshore winds, high tides, and intense storms and persist for months (Turrell, 2018). Even though some marine litter items were able to “beach” and “de-beach” over the course of one tide, persistent strandlines remained under the influence of variable winds and water levels (Turrell, 2018).
Critchell and Lambrechts (2016), introduced a settling and resuspension rate of 0.2 day−1, a wind coefficient of 2%, and a wind shadow length of 2,500 m to their particle tracking model. The model results demonstrated that the physical characteristics of the source location can have a large effect on the beaching patterns of plastic litter.
Therefore, in order to determine if deposition on the coastline explains the whereabouts of missing plastics from the surface of the ocean, it is important to apply reliable beaching conditions, in particle tracking model simulations to estimate the variability of beach-loadings (Maximenko et al., 2012; Lebreton et al., 2019; van der Mheen et al., 2019). Hence, the specific objectives of this paper are to: 1) quantify citizen-science beach-clean data to highlight the spatial variability of beached plastic litter items in northwest Scotland; 2) investigate the trajectories and distribution of plastic litter from a potential main source using a particle tracking model and 3) investigate the effect of varying model coastal boundary conditions.
2 Materials and methods
2.1 Citizen-science data collection
2.1.1 Survey locations
Data on discarded litter are routinely collected from many UK beaches by volunteers as part of the Marine Conservation Society (MCS) citizen-science beach-clean and Great British Beach Clean (GBBC) project. This study focussed on 127 beach survey locations across the west coast of Scotland (Figure 1A). A total of 666 surveys were conducted in the survey region between 1994 and 2019 (inclusive), with the frequency varying depending on location. Plastic litter items were recorded and counted during all surveys yielding a total of 338,494 items from 163 km of cumulative surveyed coastline.
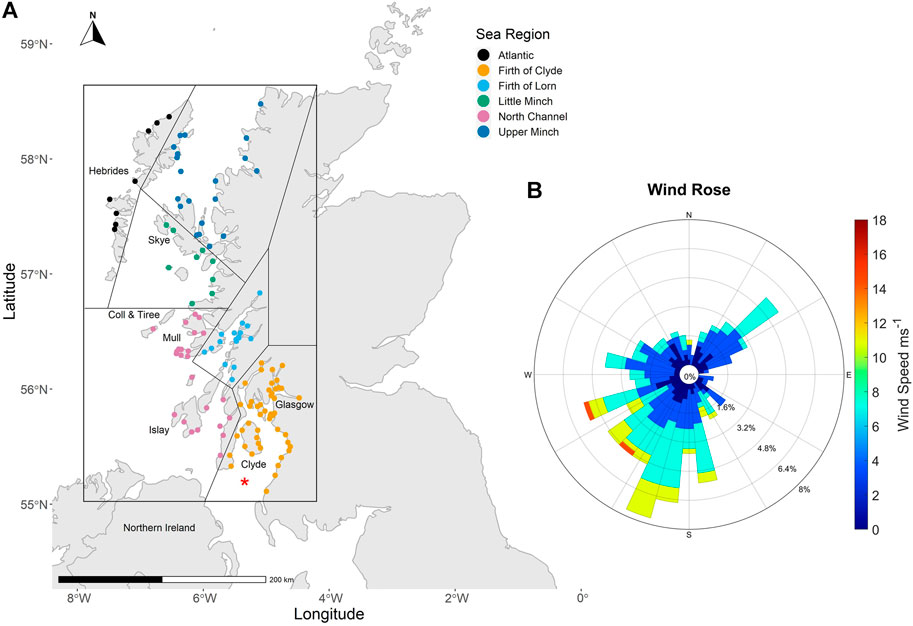
FIGURE 1. (A): Beach survey locations (n = 127) on the west coast of Scotland conducted by the Marine Conservation Society citizen-science beach-clean programme, between 1994 and 2019 (inclusive), colour coded by sea-region. The specific boundaries are provided by the inner boundaries, and the model sub-area is defined by the outer boundary. The survey locations are divided in relation to the Scottish Coastal Current (SCC), starting in the Firth of Clyde, and heading northwards through the North Channel, the Firth of Lorn, and into the Little Minch where the flow divides and turns westwards to the Atlantic or heads north through the Upper Minch. (B) Wind Rose of the daily-averaged wind speed (ms−1) and direction (from which the wind blows), for the year 2020. Wind speed was interpolated from the local high-resolution weather forecast model (WRF), at coordinates to 5.34°W, 55.20°N, in the Clyde Sea, marked by the red asterix in (A). The main islands are labelled to provide reference to their geographical location.
2.1.2 Marine Conservation Society beach-clean protocol
MCS volunteers surveyed a standard 100 m transect horizontally marked out along the most recent high tide line, known as the strandline, which is identified by a horizontal line of seaweed, or marine debris. To search for and collect litter items, volunteers walked between the transect and the back of the beach.
A recent study of MCS beach-clean data by Nelms et al. (2020) clarified that upon survey completion all litter items were divided and counted into defined categories using a field guide. All data were validated by the survey coordinator and subjected to further checks by MCS.
2.1.3 Data analysis
2.1.3.1 Data preparation
The long-term datasets were combined to produce a complete dataset from 1994 to 2019 (inclusive). The data were cleaned and sorted using the computer programming language R (R Core Team, 2020). Local beach names and corresponding GPS coordinates were assessed and refined to account for discrepancy in naming between data recorders. One set of GPS coordinates (latitude and longitude) were then given to each local survey location identified to be the same beach (i.e., along the same segment of coastline; Supplementary Table S1). The survey locations were categorised to one of six defined sea-regions, within the northwest sub-area, depending on which section of coast it lies on (Figure 1A). This was done relative to the northward coastal flow following the methods of Turrell (2019): Firth of Clyde, North Channel, Firth of Lorn, Minches (Little and Upper), and the Atlantic (Figure 1A).
The next stage was to explore the 118 litter categories utilised by MCS and defined by the OSPAR commission (OSPAR Agreement 2020-02, Supplementary Table S2). As this study was focussed on plastic items, the data was filtered to only include categories defined as plastic (n = 56). Finally, the total number of plastic items (NP) collected per survey were calculated and a cumulative total was computed for each survey location. The total number of plastic items were then generated for the six defined sea-regions. The MCS protocol indicated it was standard practice that a 100 m survey should be undertaken, although, given the nature of the project, it was common that surveys exceeded this. To facilitate analysis of the data, all surveys were standardised to 100 m (e.g., Nelms et al., 2017; Watts et al., 2017; Turrell, 2019; Nelms et al., 2020).
2.1.3.2 Survey effort
Descriptive statistics were provided for each of three variables related to survey effort: beach transect length, number of volunteers and the duration of the search, to describe the variation within the dataset. Overviews of plastic abundance in the six sea-regions were then given to collectively illustrate the volume of plastics on the west coast of Scotland.
As outliers or extreme values were present in the data, the variability was also summarised using the interquartile range (IQR). This statistic is the difference between the first quartile, denoted as Q1, and the third quartile, denoted as Q3, where Q1 is the value in the data set that holds 25% of the values below it and Q3 is the value in the data set that holds 25% of the values above it.
2.1.3.3 Spatial distribution of plastic litter items
To examine for spatial patterns, the average NP/100 m survey was calculated for each survey location (n = 127) across all surveys conducted at each location. The average NP/100 m was then calculated collectively for each sea-region, to investigate variation in plastic distribution relative to the northward coastal flow. Furthermore, the database, which comprised 56 plastic litter categories, was refined into three source-categories: land-based (n = 32, e.g., plastic bottles, plastic food packaging, household items, toys, plastic bags, and plastic toiletries), marine-based (n = 18, e.g., fishing nets, fishing boxes, oyster catches, octopus catches, oil cans and rope) and unknown (n = 6, e.g., plastic fragments, sponge), to explore the composition of plastic litter at each survey location.
2.2 Numerical models
2.2.1 Hydrodynamic model
Ocean surface current data were derived from the West Scotland Coastal Ocean Modelling System (WeStCOMS; Aleynik et al. (2016), an implementation of the finite volume community ocean model (FVCOM; Chen et al., 2013). The unstructured WeStCOMS grid consists of a 0.1–2.3 km resolution horizontal mesh composed of triangular elements (Figure 2) that are smaller in complex coastline regions. Output has been used at a temporal resolution of 1 h. Wind data was derived from a high-resolution weather forecast (WRF) model (Skamarock et al., 2008) for the west coast of Scotland (Aleynik et al., 2016). The WeStCOMS model was upgraded to version 2 in 2019 and its domain extended north, south, and westward to include the Atlantic coasts of the Hebrides (Davidson et al., 2021).
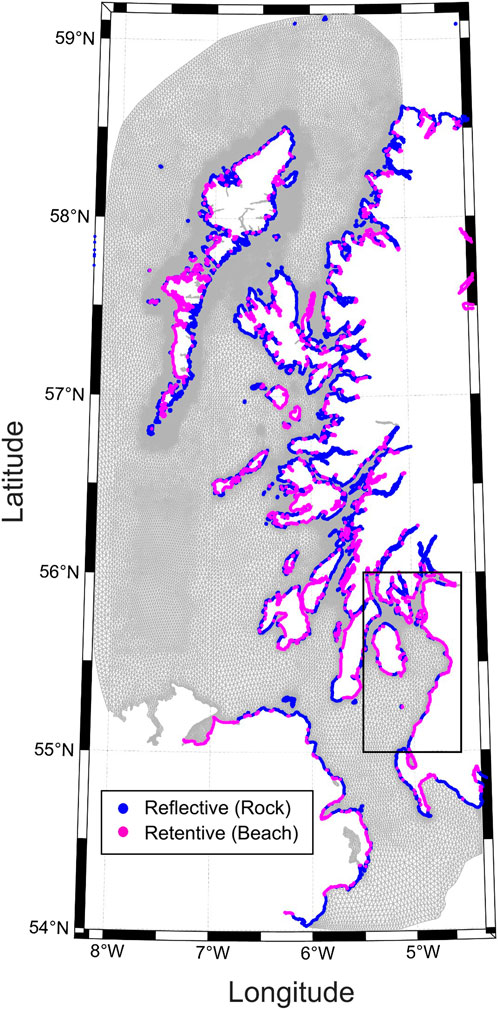
FIGURE 2. A map of the unstructured model domain composed of triangular elements and coastal classification of the model coastline. Reflective segments (rock) are highlighted in blue, and retentive segments (beach) are highlighted in pink. The source area is shown by the black box, with the particles starting locations chosen randomly in space within this area.
WeStCOMS has been tested and validated using multiple oceanic observations (Aleynik et al., 2016). The WRF model output achieved excellent model-observation agreement. The long-term mean wind direction derived from Met Office (2019) was 219°, while the average value from the WRF model was 214° (Aleynik et al., 2016). The average residual currents of WeStCOMS gave transport times which agreed with the spreading estimates of the radionuclide tracers of Sellafield origin (McKinley et al., 1981; MacKay and Baxter, 1986; Aleynik et al., 2016).
2.2.2 Particle tracking model
To model the spatial distribution of plastic litter, a particle tracking model was developed using MATLAB. The particle tracking model was designed to simulate the horizontal transport at the surface (i.e., 0 m depth) of virtual floating particles that move with ocean currents and respond to the wind effect. The change in the position of a particle (
where u is the eastward surface current component, v is the northward surface current component, uw is the eastward wind component and vw is the northward wind component. A particle’s trajectory in space and time was determined by linearly interpolating the hydrodynamic model output between fixed grid points and using a 4th order Runge-Kutta method (Waziri et al., 2010) to step forward in time with a timestep
Adapted from Turrell (2020b), where
Each modelled coastal boundary condition (see next section) was simulated using downwind drift of 0%, 1%, 3% and 5% (e.g., Duhec et al., 2015), to test the effect of varying windage on the distribution of beach loadings. The position of each particle was saved daily throughout the simulation period for plotting and post-run analysis purposes. The model boundary was refined for the purpose of analysis into a focal sub-area of the northwest coast only, shown by the outer boundary in Figure 1A. The sub-area covers the North Channel to the Upper Minch and Atlantic sub-region, excluding the southern regions of the Solway Coast and the Irish coast (4.23–7.90°W, 55.06–58.64°N).
2.3 Coastal boundary conditions
2.3.1 Sticky coast
The particle tracking model initially adopted a “sticky coast,” meaning the model did not allow for resuspension of particles following beaching. A particle is assumed “beached” if it is advected outside the model domain, i.e., no longer located within a mesh triangle.
2.3.2 Resuspending coast–unclassified
A resuspending coast was then introduced by giving each “beached” particle the probability to re-float. This was done by assigning a random number to each beached particle every timestep and if the random number was less than the probability of re-floating, the particle could resuspend and was reflected into the nearest mesh triangle within the model domain. The probability that a particle could re-float in a single timestep was described by:
where
2.3.3 Resuspending coast–classified
The morphology of the coastline can influence the suspension and resuspension of beached plastic litter. On the northwest coast of Scotland there are two predominant types of coasts; retentive sloping beaches; and non-retentive rocky coasts and cliffs. The model coastline was therefore classified based on the EUROSION dataset available from the European Environment Agency (Lenotre et al., 2004). The dataset originally consisted of 20 coastal types which was refined for the west coast of Scotland and further simplified into two sub-categories: reflective or retentive coastlines (Supplementary Table S3). Using these two sub-categories, the WeStCOMS model coastline was classified by identifying and categorising model coastal segments (Figure 2). In total, 15,890 coastal boundary segments were classified as reflective (representing 69.3% of the total 22,921 coastline segments) and 7,031 as retentive (representing 30.7% of the coastline). For the reflective coastal boundary condition, the value of T was fixed at 1 day, and it was set to 20 days again for retentive (beach) segments (Turrell, 2018).
2.4 Litter source
The input source of plastic litter was the Clyde Sea (Figure 1A), Scotland’s largest fjord (Midgley et al., 2001). This region is an area of considerable urbanisation, human interaction, fisheries, recreational activities and sewage disposal (Baxter et al., 1979), thus an important supplier of oceanic pollution. Turrell (2020a) suggested that 93% of plastic litter originating from land-based sources on the west coast of Scotland originates from the river Clyde.
Virtual particles were simulated from within the Clyde Sea for 1-year; 1st January 2020–31st December 2020, for each coastal boundary condition. A simulation length of 1 year was chosen to balance long term model behaviour vs. computational cost. A 1-year simulation allowed for the model to reach the quasi-steady state that exists between a constant source supplying floating litter and the processes which control beaching and de-beaching. After an equilibrium has been reached, the beach loadings represent those that will be experienced because of the constant litter source, coupled with varying winds and tides. An average of 50 active (floating) particles were released at the source (Figure 2), with their locations chosen randomly in space at 6-hourly intervals.
2.5 Particle conversion
To present the model results in a real-life context, modelled particles were converted into numbers of plastic litter items using the method described by (Turrell, 2020a). The total number of litter items entering the Clyde Sea in one year (Ninput) from urban litter sources is given by:
where Minput is the estimated annual input of 403 tonnes of plastic waste from modelled values of the Clyde Sea and weight is the average weight of a plastic item (g). The number of plastic items which each model particle represents (Nitems) is calculated as:
where Nmodel is the total number of model particles released into the model in one-year. If the average weight of a litter item is assumed to be 5 g (Turrell, 2020a), this gives a value of Ninput of 8.06 × 107. Approximately 50 model particles are released every six hours, giving a value of Nmodel to be roughly 73,000 for 1-year. Hence each model particle represents, on average, 1,104 litter items.
2.6 Model diagnostics
During each model run, a daily output was saved recording every particle’s position in space and time, and status, i.e., active (“floating”) or ashore (“beached”). To investigate beaching behaviour over time, the net total (i.e., the net change of beaching and resuspending particles after 24 h) of particles on a given day were computed ‘offline’ using the daily particle positions and calculated as follows:
and further converted into plastic litter items,
where Tbeached (t) is the net total number of model particles beached at the end of day t, Nbeacheddaily (t) is the net total of model particles beaching during day t, and Nitemsdaily (t) is the net total number of plastic litter items beaching during day t.
To further understand beaching behaviour, a relationship was explored between the modelled daily beaching events, wind speed, and direction. A set of coordinates within the Clyde Sea were selected (Figure 1B), and components u and v of wind speed (ms−1) were interpolated from WeStCOMS. The vector averaged daily wind speed (ms−1) was calculated and visualised to display the prevailing wind speed and direction.
2.7 Particle distribution
The model domain was divided into six sea-regions within the sub-area defined in Figure 1A, and the percentage of modelled particles in each region were calculated at the end of each model simulation. This was done to assess the spatial distribution induced from the Clyde Sea, influenced by varying wind drift coefficients. Furthermore, to visualise the areas of high deposition, the number of beached modelled particles within a 0.0045° (500 m) radius were counted, converted to number of plastic litter items, and standardised to determine NP/100 m.
3 Results
3.1 Observational data
3.1.1 Wind forcing
Figure 1B presents a summary of the daily-averaged wind forcing in the Clyde Sea for the year 2020. The predominant wind direction is from the south and southwest. Aleynik et al. (2016), reports similar observations on the Atlantic coast of Scotland in which the prevailing wind direction was from the southwest. Winds from the other sectors are generally weak (Figure 1B), although events such as storms can result in episodic periods of strong winds from any direction, but these are generally short lived (days).
3.1.2 Survey effort
The survey length in the MCS beach surveys varied between 30–4,500 m (mean = 245.68, SD = 417.23, median = 100, IQR = 100–150). The number of survey participants differed with a range between 1–141 volunteers per survey (mean = 12.86, SD = 14.94, median = 9, IQR = 3–18). The survey time ranged from 5 to 840 min (mean = 99.64, SD = 81.06, median = 90, IQR = 60–120).
Plastic items collected varied between 0 and 6,447 items per standard 100 m transect across the full dataset (mean = 380.31, SD = 5944.40, median = 171, IQR = 73.0–443.0). The number of surveys per any given location ranged from 1 to 50 (mean = 15.25, SD = 13.87, median = 10, IQR = 5–25).
Using the standardised counts across all surveys from 1995 to 2019, the total NP was 253,286 with an average of 380.31 ± 419.92 NP/100 m found on the west coast of Scotland’s beaches. Figure 3 summarises the average number of plastic items per 100 m for each survey location.
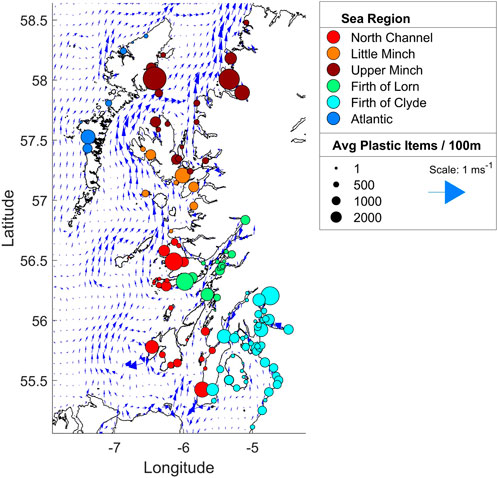
FIGURE 3. Spatial distribution of the average NP/100 m on the west coast of Scotland between 1994 and 2019 (inclusive), at all survey locations (n = 127), derived from MCS citizen-science data collection. A vector plot of the yearly averaged surface currents (ms−1) are shown across the model sub-area.
3.1.3 Spatial distribution of plastic litter
Overall, the Upper Minch had the greatest average NP/100 m, 476.1 ± 612.8 (Table 1; Figure 3). However, it is important to interpret this result with caution as there were only 65 surveys conducted within this sea-region over the time-period. The Firth of Clyde (367 surveys) had 421.4 ± 672.7 NP per 100 m respectively, with the Firth of Clyde hosting the greatest average NP/100 m of land-plastics (216.5 ± 333.3 NP/100 m; Table 1). The Upper Minch, Atlantic and the Little Minch have the greatest average NP/100 m of marine litter (245.5 ± 375.1, 204.3 ± 302.7, 198.7 ± 211.1 respectively). In the case of plastic litter of unknown origin, the Firth of Clyde has the greatest average (148.3 ± 322.1 NP/100 m) followed by the Upper Minch (146.7 ± 194.8 NP/100 m). The sea-region with the greatest survey effort was the Firth of Clyde; 367 surveys and the Firth of Lorn; 103 surveys (Table 1).
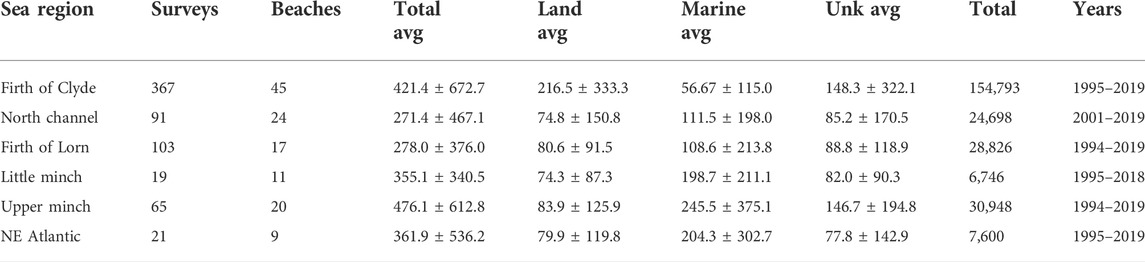
TABLE 1. A descriptive table of the average (avg) ± standard deviation, of plastic items per 100 m transect in each of the following sea-regions, within the sub-area. Sea-regions are presented following the flow of the Scottish Coastal Current (SCC), starting from the Firth of Clyde, and going northwards.
With respect to plastic litter composition, land-based sources represented 40% of the total, yielding 103,087 plastic items, with an average 154.8 ± 269.0 per 100 m. Marine-based sources represented 26% with a total of 66,161 plastic items, with an average 99.34 ± 202.1 NP/100 m. Plastic items that were unidentifiable represented 34% with a total of 84,038 plastic items, and an average of 126.2 ± 261.9 NP/100 m. The most common land-based items found were crisp packets (33%) followed by bottle caps (18%), and plastic bags (8%). String represented 47% of marine litter, with small fishing nets <50 cm representing 27% and the unknown sub-category consisted of small and large fragments at 43 and 41% of the total (Supplementary Table S2).
3.2 Particle tracking model
3.2.1 Model diagnostics
In total, 73,000 modelled particles were released in the Clyde Sea and after a 1-year model run at 3% downwind drift. On day 365, 86.7%, 80.2% and 46.3% were beached in the model sub-area for the sticky, resuspending (unclassified and classified) coastal boundary conditions, respectively (Supplementary Table S5). Figure 4B shows the total beached particles over time at 3% downwind drift, with the resuspending classified coastal boundary condition reaching an equilibrium state (i.e., beaching balances de-beaching) from day 300. It should be noted that prior to day 300 there is a “ramp-up” stage due to the number of particles released within the model increasing (Figures 4B,C). The sticky coastal boundary condition never reaches an equilibrium as beached loads continually increase (Figure 4B). The resuspending unclassified coast does not reach equilibrium within the 1-year model run, as the beached concentration needed for de-beaching to equal beaching is not reached (Figure 4B).
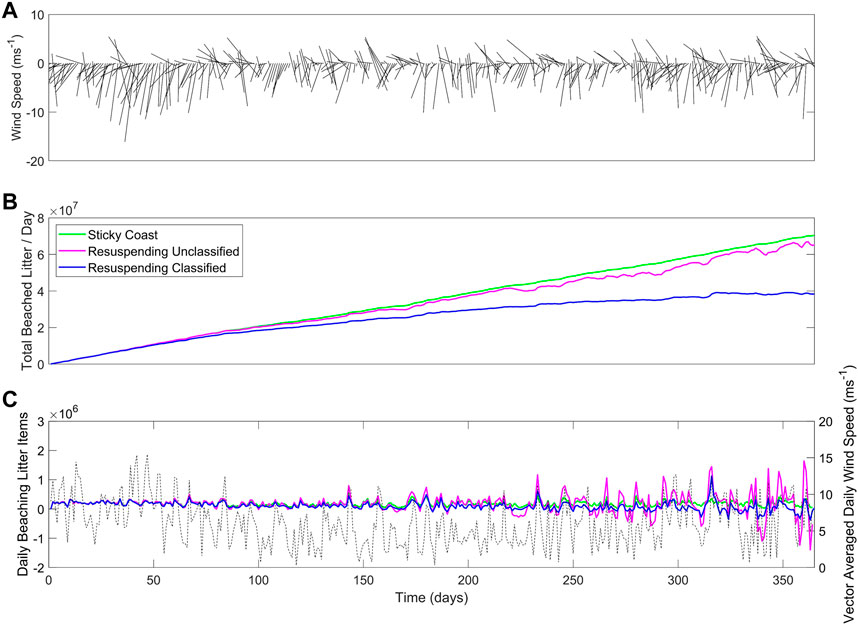
FIGURE 4. (A) A vector timeseries of the daily average wind speed (ms−1) and direction (from which the wind blows) across the model domain. (B) Total daily beaching plastic litter items at 3% wind drift and (C) the number of daily beaching plastic litter items at 3% wind drift for the sticky coastline (solid green line) the resuspending unclassified coastline (solid black line) and the resuspending classified coastline (solid pink line). The vector averaged daily wind speed (ms−1), is represented by the dotted black line. The model simulation ran from 1st January to 31st December 2020.
The number of daily beaching particles throughout each model run, at 1%, 3%, 5% downwind drift, are variable and fluctuate due to changing wind conditions, with less fluctuation observed at 0% downwind drift (Supplementary Figure S2). For example, on day 312, the vector averaged wind speed is 3.1 ms−1 (Figure 4C), with generally weak easterly winds (Figure 4A), to which there was a net resuspension of plastic items, −491,280 and −98,256 off the full model coastline for the resuspending unclassified and classified coast respectively (Figure 4C). In comparison, on day 316, the vector averaged wind speed increases to 12.4 ms−1 (Figure 4C), with generally stronger northerly winds (Figure 4A), to which there was a net influx of plastic items onto the full model coastline, 1,446,240 and 1,127,184 for the resuspending unclassified and classified coast respectively (Figure 4C).
The relationship between daily beaching events and wind forcing is further explored during the steady-state period (day 300–365) in Figure 5. For the sticky coast (Figure 5A), high numbers of particles beach for winds from the south and southwest (yellow/red symbols), while low numbers beach for winds from the north and northeast (blue symbols). For the resuspending coasts (Figures 5B,C), winds from the south and southwest generally result in net beaching events (yellow/red symbols), while winds from the north and northeast generally result in net un-beaching events (blue/green symbols).
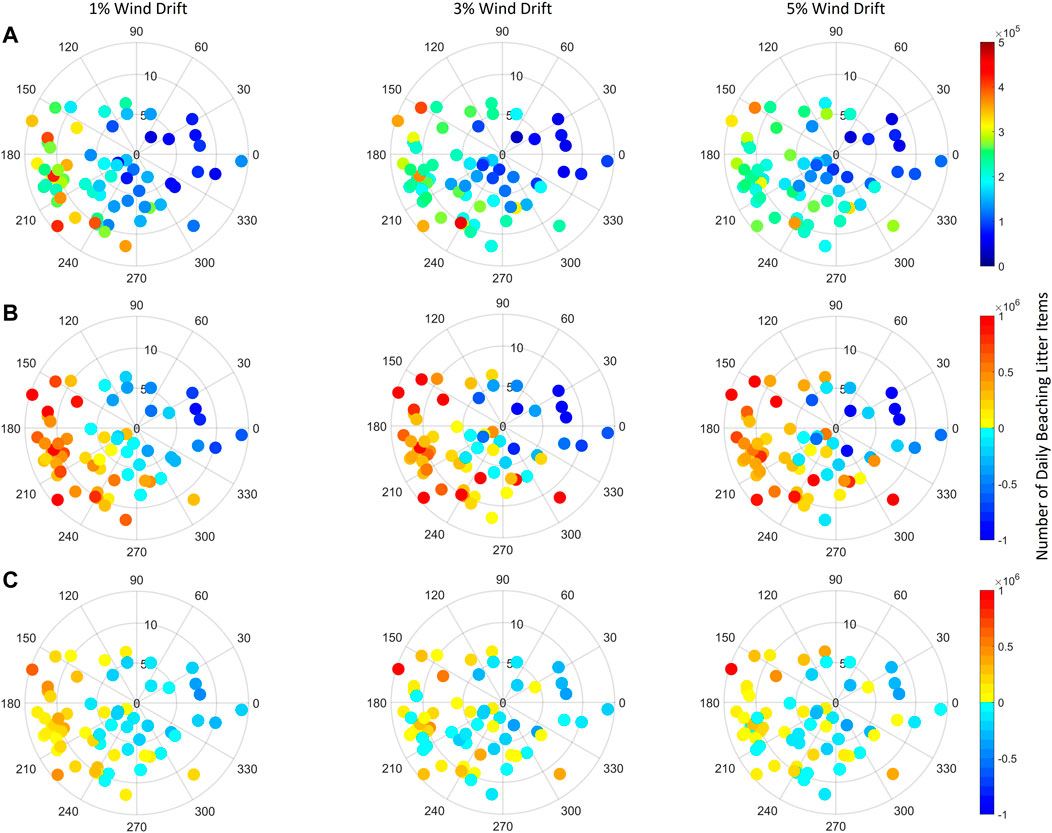
FIGURE 5. Wind Scatter plot of the number of daily beaching plastic litter items within the model sub-area during the steady-state period (day 300–365) for the (A) sticky coast boundary condition, (B) resuspending unclassified coastal boundary condition and (C) resuspending classified coastal boundary condition. A comparison of the downwind drift as a percentage of the wind speed is displayed for 1%, 3% and 5%.
3.2.2 Particle spatial distributions
After a 1-year model run at 3% downwind drift, 4.84%, 9.8% and 3.1%, of modelled particles travelled across a northern boundary and beached along the northwest coast for the sticky, resuspending (unclassified and classified) coastal boundary conditions, respectively (Supplementary Table S5). Figure 6 presents the distribution of beached particles, on day 365, after 1-year of simulated dispersion from the Clyde litter source. Results are presented here for three windage values (1%, 3% and 5%), with results of 0% windage provided in Supplementary Figure S4 for the three coastal boundary conditions (sticky, resuspending unclassified, resuspending classified). For a visual representation of the geographical areas discussed in this section, see Figure 1.
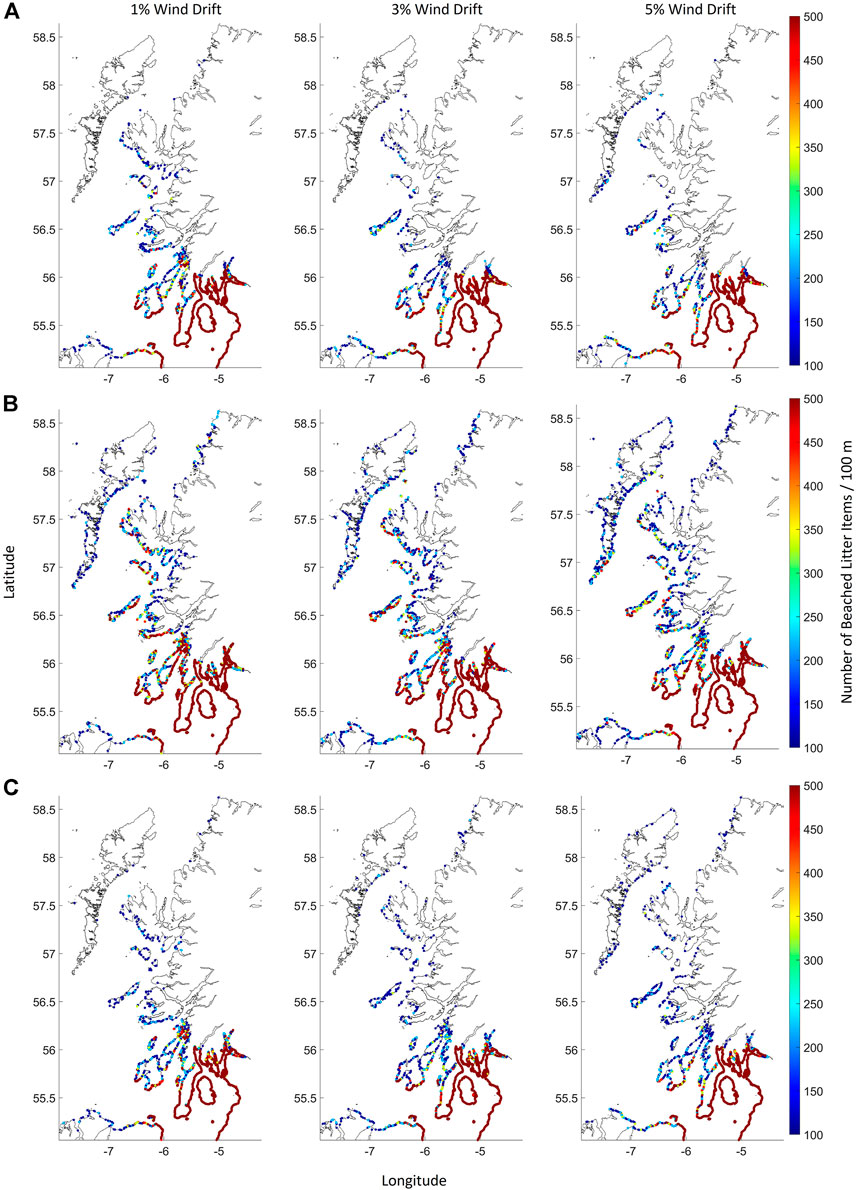
FIGURE 6. Beaching distribution of NP/100 m within the model sub-area. Results are derived from the particle tracking model at the end of a 1-year model run (i.e., a count of the final position of beached particles on day 365) for (A) sticky coastal boundary condition, (B) resuspending unclassified coastal boundary condition and (C) resuspending classified coastal boundary condition. It is important to note here the minimum value on the scale is 100 and the maximum value 6 × 104. The scale has been adjusted to allow representation of lower values along the coastline.
Litter from the Clyde clearly impacts coasts north of the Clyde Sea. However, the most heavily affected coasts are within the Clyde itself, and along the coast of Northern Ireland, opposite the entrance to the Clyde. For example, at 3% downwind drift, on day 365, 81.9%, 70.4% and 43.2% of modelled particles were deposited on coasts of the Clyde Sea for the sticky, resuspending (unclassified and classified) coastal boundary conditions, respectively (Supplementary Table S5). The residual circulation in the North Channel consists of two main flows (MacKay and Baxter, 1985; MacKay and Baxter, 1986), a minor flow going southward along the Irish coast, and the major outflow is northward along its eastern Scottish coast (Figure 3; Supplementary Figure S1). Along the Scottish coast, beached litter is evident (Figures 6A–C), with the particle densities decreasing northwards from the Clyde (Table 2).
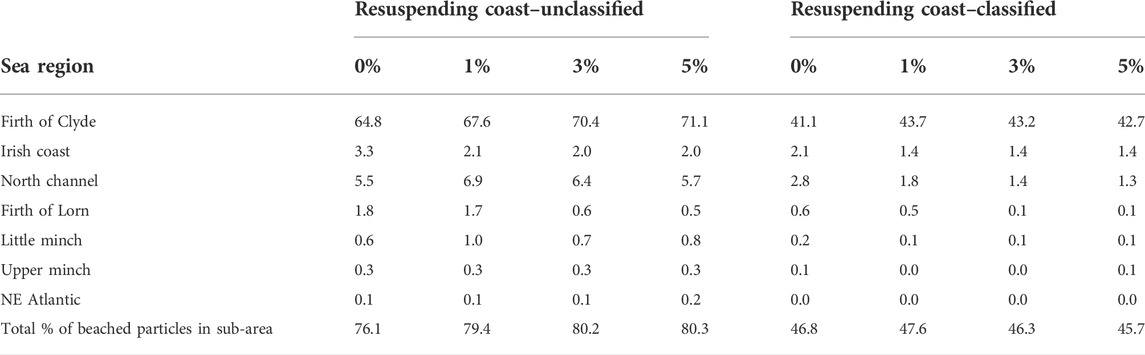
TABLE 2. The percentage of plastic litter items, of the total released particles, that ‘beached’ at the end of each model run (day 365) in the defined sea-regions within the model sub-area. Sea-regions are presented following the flow of the Scottish Coastal Current (SCC), starting from the Firth of Clyde, and going northwards. Details of the SCC are provided in Supplementary Figure S1. Values have been calculated for 0%, 1%, 3% and 5% wind drift for the resuspending unclassified and the resuspending classified coastline to compare wind effect on beached particle distribution.
For the sticky coast (Figure 6A), most litter is trapped by outer coastlines and southerly facing coasts of islands. Very little litter enters the sea lochs along the coast. The effect of increasing windage from 1% to 5% seems to be to drive litter further offshore, with more litter accumulating on the Outer Hebrides. For this coastal condition, little litter reaches north of about 58°N.
When resuspension is introduced along all the coastline (Figure 6B), litter reaches the northern tip of the Scottish west coast (i.e., >58.5°N). Much more reaches island coasts (e.g., Tiree, Skye, Outer Hebrides), and more litter enters sea lochs (e.g., Loch Linnhe). This is to be expected, as resuspension allows the litter to be dispersed by a wider range of wind strengths and directions.
Introducing the more realistic coastal boundary condition of a classified resuspending coast (i.e., with pockets of retaining beaches separated by resuspending rocks and cliffs. See Figure 2), results in an intermediate dispersion of litter, between the extremes of a sticky coast and a fully resuspending coast (Figure 6C). The classified resuspending boundary condition seems to emphasise the difference between the three windage values. For the 5% windage model run, there is considerable differentiation between the east and west coastlines within the Clyde itself, and far less litter reaches the coast of Northern Ireland.
4 Discussion
This study investigated the spatial variability of beached plastic litter on the west coast of Scotland, using holistic methodologies to explore the need to utilise both field and modelling methods to better understand plastic litter distribution. First, the long-term, beach-clean data set provided by the Marine Conservation Society (MCS), which is the only data available for the sparsely populated west coast of Scotland, was explored to provide an understanding of, and detect spatial patterns in, beached plastic litter in the study region.
On average, it was calculated that there are 383.6 ± 589.4 NP/100 m on the Scottish west coast. To gain a broad understanding of the source location, items were divided into either land, marine or unknown, based on their identified purpose. We found that the Clyde Sea had the largest NP/100 m of land-based plastics, most likely local litter from urban centres (Turrell, 2019). In contrast, the Upper Minch and North-East Atlantic regions had the largest NP/100 m of marine plastics (Table 1). Similarly, Nelms et al. (2017) found that in the North Atlantic, the greatest proportion of litter was attributed to marine-based sources. They suggest this is likely caused by exposure to inputs from marine sources, mainly fisheries, due to the remoteness of the region and low population density.
Even though engagement of citizen-scientists in beach-clean surveys has proven to produce high-quality data (e.g., Nelms et al., 2017; Paradinas et al., 2021; Damian et al., 2022), data are subject to volunteer availability and site accessibility. For the west coast of Scotland, gaps were evident in the analysis of the spatial distribution and quantification of beached plastic litter, most probably due to rural areas of sparse populations. In total, across the 25-year dataset, there were 367 beach-clean surveys conducted within the Clyde Sea alone on 45 beaches, whereas, within the remote northeast Atlantic there were only 21 beach-clean surveys conducted on nine beaches (Table 1). This reflects that citizen-science data collection is likely to favour densely populated and easily accessible areas. Here, while the purpose of the hydrodynamic modelling was not to quantify beach loadings, we effectively show that hydrodynamic modelling is a key tool to fill these notable gaps, and together with field methods can provide a more robust understanding of plastic litter distribution and dynamics.
The first stage in the analysis of the particle tracking model output, was to record the accumulation of beached particles over time. The results showed that 86.6%, 80.2% and 46.3%, of particles were beached after a 1-year model run at 3% downwind drift, for the sticky, resuspending (unclassified and classified) coastal boundary conditions, respectively. Onink et al. (2021), stated that depending on parameter values, beached particles were in the range of 31%–95% at the end of a 5-year simulation. Notably, they also suggested that high beaching probabilities combined with small resuspension probabilities, would result in substantial amounts of beach loadings.
Initially when implementing the classified model coastline, it was hypothesised that coast type (reflective; rock, or retentive; beach) would be a significant factor in impacting plastic litter distribution. While it does have a definite impact, especially in retentive regions with a high probability of beaching (Figure 6C), beaching mechanisms also depend on local coastal dynamics, such as, tidal ranges and the prevalence of onshore and offshore winds, as well as morphology, e.g., cliffs, therefore beaching probabilities are likely to vary from location to location.
Here, we used a simple method by defining the resuspension probability based on a random number, but a better understanding of the spatial variation of resuspending particles could account for local tidal elevation, wind direction, and the frequency of storms (Turrell, 2018; Bastesen et al., 2021). Implementing these mechanisms would likely improve the spatial distribution of beaching plastics (van der Mheen et al., 2020). Furthermore, Turrell (2019) proposed that for macro-tidal beaches (>4 m), which are typical on the west coast of Scotland, areas of high-density plastics are washed back into the ocean by wind-driven events at times of high water levels, thus not accumulating onshore. Comparable patterns have been observed in other geographical regions, for example, Damian et al. (2022) found that on the Caribbean coast of Costa Rica, beached plastic litter loadings were higher during the dry season (January–September) and lower during the rainy season (November–January), with peak accumulation rates observed during the spring months (March–May). They suggest this was an effect of rising water levels e.g., heavy rainfall, flooding, and increased intensity of storms, effectively washing beached plastic litter into the ocean.
To assess such local dynamics within the study region, in particular the wind effect, the daily beaching events were plotted in relation to wind speed and direction at 1%, 3% and 5% downwind drift (Figures 5A–C). As the sticky coast did not allow for resuspension, the resuspending coastlines are only applicable here. Figures 5B,C demonstrates that there is a positive relationship between the prevailing westerly winds (Figure 1B) and daily beaching events. It can be observed that positive values, which represent deposition, are most prominent in the southwest direction (onshore wind), whereas negative values, which represent resuspension, are more prominent in the northeast (offshore wind).
These results correspond with Turrell (2018), where the VaWWL model found that during offshore wind events litter is removed from the beach, and during onshore wind events more litter is deposited. Veerasingam et al. (2016) found that on the Goa coast of India, microplastics were deposited during the southwest monsoon (July), with the driving forces for their transportation winds and surface currents. During the southwest monsoon, the currents and winds are to the east direction (i.e., towards the coast), concluding that prevailing strong west/south westerly winds combined with wind driven surface currents cause the transport of microplastics towards the coast.
Finally, an analysis of the model output was conducted to assess particle distribution from the source input and identify spatial patterns along the coastline. Table 2 demonstrates that for each coastal boundary condition, particles exited the Clyde Sea, and traversed to the northwest coast, therefore the Clyde Sea is considered a source of domestic plastic litter to this region. For the sticky coast boundary condition, 81.9% of particles remained in the Clyde Sea, which suggests beaching quickly after entry. When introducing the resuspending (unclassified) coastal boundary conditions this value is reduced to 70.4% and finally, for the resuspending (classified) coastline, the value is 43.2% as more particles exit the region.
Spatial patterns in beach plastic litter are evident on the northwest coast, more so in both resuspending models, which highlights the importance of this coastal boundary condition in modelling beached litter distribution. Figure 6B shows spatial patterns along the northwest coast, which are not visible in Figure 6A. These patterns coincide with Turrell (2018) in which they state such removal from the beach would characteristically result in patches of high-density litter entering the SCC (Supplementary Figure S1), consequently influencing the flux onto the next beach in the northwards direction of the coastal flow. The major outflow in the North Channel is northwards along the eastern Scottish coast (Figure 3; Supplementary Figure S1), enabling the transportation of particles from the Clyde Sea in this direction.
Higher densities are more prominent on the exposed Atlantic coast of the outer islands, for example, on the southwest coast of the Isle of Islay, Isle of Mull, and the Isle of Skye (Figures 6B,C). The Islay Front is a strong horizontal salinity gradient, with northward current speeds of 0.3–0.5 ms−1 (Figure 3; Supplementary Figure S1), which marks the transition from the coastal region, an area of strong tides and relatively shallow water, to stratified oceanic water to the west of the front (MacKay and Baxter, 1986), continuing a particles’ journey northwards.
The observed distribution of particles on the exposed Atlantic coast could also be influenced by the prevailing westerly wind direction (Figure 1A), and the complex topography of the region, consisting of islands, embayment’s, sea lochs, and cliffs, shadowing the mainland. Accumulation of plastic litter in exposed areas were also described by Critchell and Lambrechts (2016), in which they modelled plastic litter distributions around complex regions. They highlighted the importance of local winds, and that wind shadowing caused by such features can regulate plastic litter distribution. These results are also similar to those of Turrell (2019), in which foreshores facing into the prevailing westerly winds, had greater plastic litter loadings than foreshores within sheltered embayment’s. When comparing the influence of downwind drift on the spatial distribution, it is noticeable that in the model run with 5%, particles reach the northwest Atlantic coast (Figures 6A–C), whereas in the model run with 1% they are absent in this region. This suggests that windage exerted on the surface area of positively buoyant (floating) particles can influence their trajectory and distribution ultimately covering large distances. Additionally, particles subject to 0% wind drag, i.e., solely currents, had less fluctuation in daily beaching events, compared with higher wind drag coefficients (Supplementary Figures S2A–C). Likewise, Gutow et al. (2018), observed through oceanographic modelling that the probability of a particles being deposited onshore was influenced by the wind drag coefficient. They report that particles subject to strong winds were more quickly pushed ashore by the prevailing westerly winds in the region.
While it is more robust to use the resuspending (classified) coastal boundary condition, as this has been shown to further influence spatial patterns, it is important to consider that there are similarities between accumulation patterns for both the resuspending (unclassified and classified) models. These similarities could be explained by parameters that are constant through both models, such as current flow, windage or beach residence times. Similar observations were observed by Daily et al. (2021) when incorporating terrain into particle tracking models.
In the context of evaluating the robustness of the model, it is worth noting the number of beached particles (NP/100 m), for each model run was an overestimation compared to the observed values in the observational data (Table 1) by at least an order of magnitude (Figures 6A–C). At 3% downwind drift, the sticky coast had a range of 100–50 × 103 NP/100 m (Figure 6A), and the resuspending coast (unclassified and classified) had a range of 100–58 × 103 NP/100 m (Figure 6B) and 100–28 × 103 NP/100 m respectively (Figure 6C), compared to a range of 1–2,355 NP/100 m for the observational data. This may be explained by loss mechanisms occurring on short timescales (Onink et al., 2021), such as biofouling, which can increase the density of a particle and cause it to sink over time (Fazey and Ryan, 2016), burial in sediments, or ingestion (Onink et al., 2021). This is proposed based on evidence from previous studies that floating plastics were present in samples collected from both nearshore and deep-sea sediment (e.g., Ye and Andrady, 1991; Lobelle and Cunliffe, 2011; Lobelle et al., 2021). Furthermore, Cozar et al. (2014) suggested that 60%–64% of land-based plastic entering the ocean via rivers traverses the coastal ocean and enters the open ocean. Hence between 36% and 40% sinks to the seabed on first entry to sea or soon thereafter.
The particle tracking model developed for the purpose of this study therefore may be limited in the sense that it simulates positively buoyant plastic (floating plastic), that can only ever be active, “floating” or ashore, “beached,” i.e., it does not account for mechanisms that can remove floating plastics from the surface, and only considers one input source. Ideally, for future predictions, the model could be improved by incorporating such mechanisms which would likely have an increasing effect on sediment deposition and thus reduce the amount of beached plastic. Further simulations could be conducted to consider alternative sources of oceanic plastic pollution. Understanding the factors that influence the deposition of plastic litter in coastal regions is vital to inform governing agencies of management strategies that could be used to prevent plastic litter inputs and reduce marine plastic pollution. The model presented here will be further developed and used to provide advice to marine managers. It will also be used to develop a more targeted monitoring programme of both macro and micro plastics along the Scottish Atlantic coast.
Data availability statement
The original contributions presented in the study are included in the article/Supplementary Material, further inquiries can be directed to the corresponding author.
Author contributions
NA is a PhD student supervised by BN, AD and WT, and all contributed to, conceived, and designed the study. DA developed and run WeStCOMS operational hydrodynamic forecasting system. NA and AD developed the particle tracking model. NA conducted the data analysis and drafted the first version of the manuscript. All authors read and critically revised the final version of the manuscript.
Funding
This research was funded by Marine Scotland as part of the Scottish Universities Partnership for Environmental Research (SUPER) DTP PhD studentship. Expansion of the WeStCOMS hydrodynamic model was carried out in the “Collaborative Oceanography and Monitoring for Protected Areas and Species” (COMPASS) project, supported by the EU’s INTERREG VA Programme and managed by the Special EU Programmes Body, the EU Atlantic Area INTERREG project “Predicting Risk and Impact of Harmful Events on the Aquaculture Sector” (PRIMROSE), and UKRI grants: “Combining Autonomous observations and Models for Predicting and Understanding Shelf seas” (CAMPUS NE/R00675X/1) and “Evaluating the Environmental Conditions Required for the Development of Offshore Aquaculture” (OFF-AQUA, BB/S004246/1).
Acknowledgments
The authors would like to thank the Marine Conservation Society for providing access to their long-term monitoring dataset, and to all volunteers who participated and made it possible to generate such a dataset. The dedication and hard work required during such beach-clean surveys is greatly acknowledged and appreciated. We also give thanks to the editor and two reviewers for their invaluable feedback and support in improving this manuscript.
Conflict of interest
The authors declare that the research was conducted in the absence of any commercial or financial relationships that could be construed as a potential conflict of interest.
Publisher’s note
All claims expressed in this article are solely those of the authors and do not necessarily represent those of their affiliated organizations, or those of the publisher, the editors and the reviewers. Any product that may be evaluated in this article, or claim that may be made by its manufacturer, is not guaranteed or endorsed by the publisher.
Supplementary material
The Supplementary Material for this article can be found online at: https://www.frontiersin.org/articles/10.3389/fenvs.2022.940892/full#supplementary-material
References
Aleynik, D., Dale, A. C., Porter, M., and Davidson, K. (2016). A high resolution hydrodynamic model system suitable for novel harmful algal bloom modelling in areas of complex coastline and topography. Harmful Algae 53, 102–117. doi:10.1016/j.hal.2015.11.012
Andriolo, U., Goncalves, G., Rangel-Buitrago, N., Paterni, M., Bessa, F., Goncalves, L. M. S., et al. (2021). Drones for litter mapping: An inter-operator concordance test in marking beached items on aerial images. Mar. Pollut. Bull. 169, 112542. doi:10.1016/j.marpolbul.2021.112542
Bastesen, E., Haave, M., Andersen, G. L., Velle, G., Bødtker, G., and Krafft, C. G. (2021). Rapid landscape changes in plastic bays along the Norwegian coastline. Front. Mar. Sci. 8. doi:10.3389/fmars.2021.579913
Baxter, M. S., McKinley, I. G., Mackenzie, A. B., and Jack, W. (1979). Windscale radiocaesium in the Clyde Sea area. Mar. Pollut. Bull. 10, 116–120. doi:10.1016/0025-326x(79)90104-8
Chen, C., Beardsley, R. C., Cowles, G., Qi, J., Lai, Z., Gao, GaoGuoping., et al. (2013). An unstructured grid, finite-volume community ocean model FVCOM user manual.
Chubarenko, I., Bagaev, A., Zobkov, M., and Esiukova, E. (2016). On some physical and dynamical properties of microplastic particles in marine environment. Mar. Pollut. Bull. 108 (1-2), 105–112. doi:10.1016/j.marpolbul.2016.04.048
Cocking, J., Narayanaswamy, B. E., Waluda, C. M., and Williamson, B. J. (2022). Aerial detection of beached marine plastic using a novel, hyperspectral short-wave infrared (SWIR) camera. ICES J. Mar. Sci. 0, 648–660. doi:10.1093/icesjms/fsac006
Courtene-Jones, W., Quinn, B., Ewins, C., Gary, S. F., and Narayanaswamy, B. E. (2019). Consistent microplastic ingestion by deep-sea invertebrates over the last four decades (1976-2015), a study from the North East Atlantic. Environ. Pollut. 244, 503–512. doi:10.1016/j.envpol.2018.10.090
Courtene-Jones, W., Quinn, B., Gary, S. F., Mogg, A. O. M., and Narayanaswamy, B. E. (2017). Microplastic pollution identified in deep-sea water and ingested by benthic invertebrates in the Rockall Trough, North Atlantic Ocean. Environ. Pollut. 231 (1), 271–280. doi:10.1016/j.envpol.2017.08.026
Cozar, A., Echevarria, F., Gonzalez-Gordillo, J. I., Irigoien, X., Ubeda, B., Hernandez-Leon, S., et al. (2014). Plastic debris in the open ocean. Proc. Natl. Acad. Sci. U. S. A. 111 (28), 10239–10244. doi:10.1073/pnas.1314705111
Critchell, K., Grech, A., Schlaefer, J., Andutta, F. P., Lambrechts, J., Wolanski, E., et al. (2015). Modelling the fate of marine debris along a complex shoreline: Lessons from the Great Barrier Reef. Estuar. Coast. Shelf Sci. 167, 414–426. doi:10.1016/j.ecss.2015.10.018
Critchell, K., and Lambrechts, J. (2016). Modelling accumulation of marine plastics in the coastal zone; what are the dominant physical processes? Estuar. Coast. Shelf Sci. 171, 111–122. doi:10.1016/j.ecss.2016.01.036
Daily, J., Onink, V., Jongedijk, C. E., Laufkotter, C., and Hoffman, M. J. (2021). Incorporating terrain specific beaching within a Lagrangian transport plastics model for Lake Erie. Microplast. Nanoplast. 1 (1), 19. doi:10.1186/s43591-021-00019-7
Damian, M., Harris, A., Aussage, J., and Fraser, G. S. (2022). Seasonal deposition of marine debris on an important marine turtle nesting beach in Costa Rica. Mar. Pollut. Bull. 177, 113525. doi:10.1016/j.marpolbul.2022.113525
Davidson, K., Whyte, C., Aleynik, D., Dale, A., Gontarek, S., Kurekin, A. A., et al. (2021). HABreports: Online early warning of harmful algal and biotoxin Risk for the scottish shellfish and finfish aquaculture industries. Front. Mar. Sci. 8. doi:10.3389/fmars.2021.631732
Derraik, J. G. B. (2002). The pollution of the marine environment by plastic debris: A review. Mar. Pollut. Bull. 44, 842–852. doi:10.1016/s0025-326x(02)00220-5
Dobler, D., Huck, T., Maes, C., Grima, N., Blanke, B., Martinez, E., et al. (2019). Large impact of Stokes drift on the fate of surface floating debris in the South Indian Basin. Mar. Pollut. Bull. 148, 202–209. doi:10.1016/j.marpolbul.2019.07.057
Duhec, A. V., Jeanne, R. F., Maximenko, N., and Hafner, J. (2015). Composition and potential origin of marine debris stranded in the Western Indian Ocean on remote Alphonse Island, Seychelles. Mar. Pollut. Bull. 96 (1-2), 76–86. doi:10.1016/j.marpolbul.2015.05.042
Fazey, F. M. C., and Ryan, P. G. (2016). Debris size and buoyancy influence the dispersal distance of stranded litter. Mar. Pollut. Bull. 110 (1), 371–377. doi:10.1016/j.marpolbul.2016.06.039
Gutow, L., Ricker, M., Holstein, J. M., Dannheim, J., Stanev, E. V., and Wolff, J. O. (2018). Distribution and trajectories of floating and benthic marine macrolitter in the south-eastern North Sea. Mar. Pollut. Bull. 131, 763–772. doi:10.1016/j.marpolbul.2018.05.003
Hardesty, B. D., Harari, J., Isobe, A., Lebreton, L., Maximenko, N., Potemra, J., et al. (2017). Using numerical model simulations to improve the understanding of micro-plastic distribution and pathways in the marine environment. Front. Mar. Sci. 4. doi:10.3389/fmars.2017.00030
Isobe, A., Kubo, K., Tamura, Y., Kako, S., Nakashima, E., and Fujii, N. (2014). Selective transport of microplastics and mesoplastics by drifting in coastal waters. Mar. Pollut. Bull. 89 (1-2), 324–330. doi:10.1016/j.marpolbul.2014.09.041
Jalon-Rojas, I., Wang, X. H., and Fredj, E. (2019). A 3D numerical model to Track Marine Plastic Debris (TrackMPD): Sensitivity of microplastic trajectories and fates to particle dynamical properties and physical processes. Mar. Pollut. Bull. 141, 256–272. doi:10.1016/j.marpolbul.2019.02.052
Jambeck, J. R., Geyer, R., Wilcox, C., Siegler, R. T., Perryman, M., Andrady, A., et al. (2015). Plastic waste inputs from land into the ocean. Science 347 (6223), 768–771. doi:10.1126/science.1260352
Jamieson, A. J., Brooks, L. S. R., Reid, W. D. K., Piertney, S. B., Narayanaswamy, B. E., and Linley, T. D. (2019). Microplastics and synthetic particles ingested by deep-sea amphipods in six of the deepest marine ecosystems on Earth. R. Soc. open Sci. 6 (2), 180667. doi:10.1098/rsos.180667
Jones, S., Inall, M., Porter, M., Graham, J., and Cottier, F. (2019). Strom-driven across-shelf oceanic flows into coastal waters. Ocean Sci. doi:10.5194/os-2019-115
Law, K. L., Ferguson, M. S., Maximenko, A. N., Proskurowski, G., Peacock, E. E., Hafner, J., et al. (2010). Plastic accumulation in the north atlantic subtropical gyre. Science 329, 1185–1188. doi:10.1126/science.1192321
Lebreton, L., and Andrady, A. (2019). Future scenarios of global plastic waste generation and disposal. Palgrave Commun. 5 (1), 6. doi:10.1057/s41599-018-0212-7
Lebreton, L. C., Greer, S. D., and Borrero, J. C. (2012). Numerical modelling of floating debris in the world's oceans. Mar. Pollut. Bull. 64 (3), 653–661. doi:10.1016/j.marpolbul.2011.10.027
Lebreton, L., Egger, M., and Slat, B. (2019). A global mass budget for positively buoyant macroplastic debris in the ocean. Sci. Rep. 9 (1), 12922. doi:10.1038/s41598-019-49413-5
Lebreton, L., Slat, B., Ferrari, F., Sainte-Rose, B., Aitken, J., Marthouse, R., et al. (2018). Evidence that the Great pacific garbage patch is rapidly accumulating plastic. Sci. Rep. 8 (1), 4666. doi:10.1038/s41598-018-22939-w
Lenotre, N., Thierry, P., Batkowski, D., and Vermeersch, F. (2004). EUROSION PROJECT the coastal erosion layer WP 2.6.
Liubartseva, S., Coppini, G., Lecci, R., and Creti, S. (2016). Regional approach to modeling the transport of floating plastic debris in the Adriatic Sea. Mar. Pollut. Bull. 103 (1-2), 115–127. doi:10.1016/j.marpolbul.2015.12.031
Lobelle, D., and Cunliffe, M. (2011). Early microbial biofilm formation on marine plastic debris. Mar. Pollut. Bull. 62 (1), 197–200. doi:10.1016/j.marpolbul.2010.10.013
Lobelle, D., Kooi, M., Koelmans, A. A., Laufkotter, C., Jongedijk, C. E., Kehl, C., et al. (2021). Global modeled sinking characteristics of biofouled microplastic. J. Geophys. Res. Oceans 126 (4), e2020JC017098. doi:10.1029/2020JC017098
MacKay, W. A., Baxter, M. S., Ellett, D., and Meldrum, D. (1986). Radiocaesium and circulation patterns west of Scotland. J. Environ. Radioact. 4, 205–232. doi:10.1016/0265-931x(86)90011-1
MacKay, W. A., and Baxter, M. S. (1985). Water transport from the north-east Irish Sea to western scottish coastal waters: Further observations from time-trend matching of Sellafield radiocaesium. Estuar. Coast. Shelf Sci. 21, 471–480. doi:10.1016/0272-7714(85)90051-4
Martin, C., Parkes, S., Zhang, Q., Zhang, X., McCabe, M. F., and Duarte, C. M. (2017). Use of unmanned aerial vehicles for efficient beach litter monitoring. doi:10.31230/osf.io/zgtwn
Maximenko, N., Hafner, J., and Niiler, P. (2012). Pathways of marine debris derived from trajectories of Lagrangian drifters. Mar. Pollut. Bull. 65 (1-3), 51–62. doi:10.1016/j.marpolbul.2011.04.016
McKinley, I. G., Baxter, M. S., and Jack, W. (1981). A simple model of radiocaesium transport from windscale to the clyde sea area. Estuar. Coast. Shelf Sci. 13, 59–67. doi:10.1016/s0302-3524(81)80105-3
Meijer, L. J. J., van Emmerik, T., van der Ent, R., Schmidt, C., and Lebreton, L. (2021). More than 1000 rivers account for 80% of global riverine plastic emissions into the ocean. Sci. Adv. 7 (18), eaaz5803. doi:10.1126/sciadv.aaz5803
Met Office (2019). Met Office integrated data archive system (MIDAS) open: UK land surface stations data (1853 - current). (CEDA), Oxford, UK. Centre for Environmental Data Analysis. Available at: http://catalogue.ceda.ac.uk/uuid/dbd451271eb04662beade68da43546e1 (Accessed: 23 06, 2022).
Midgley, R. P., Simpson, J. H., Hyder, P., and Rippeth, T. P. (2001). Seasonal cycle of vertical structure and deep water renewal in the Clyde Sea. Estuar. Coast. Shelf Sci. 53 (6), 813–823. doi:10.1006/ecss.2001.0823
Morales-Caselles, C., Viejo, J., Martí, E., González-Fernández, D., Pragnell-Raasch, H., González-Gordillo, J. I., et al. (2021). An inshore–offshore sorting system revealed from global classification of ocean litter. Nat. Sustain. 4 (6), 484–493. doi:10.1038/s41893-021-00720-8
Nelms, S. E., Coombes, C., Foster, L. C., Galloway, T. S., Godley, B. J., Lindeque, P. K., et al. (2017). Marine anthropogenic litter on British beaches: A 10-year nationwide assessment using citizen science data. Sci. Total Environ. 579, 1399–1409. doi:10.1016/j.scitotenv.2016.11.137
Nelms, S. E., Eyles, L., Godley, B. J., Richardson, P. B., Selley, H., Solandt, J. L., et al. (2020). Investigating the distribution and regional occurrence of anthropogenic litter in English marine protected areas using 25 years of citizen-science beach clean data. Environ. Pollut. 263, 114365. doi:10.1016/j.envpol.2020.114365
Onink, V., Jongedijk, C. E., Hoffman, M. J., van Sebille, E., and Laufkötter, C. (2021). Global simulations of marine plastic transport show plastic trapping in coastal zones. Environ. Res. Lett. 16 (6), 064053. doi:10.1088/1748-9326/abecbd
Onink, V., Wichmann, D., Delandmeter, P., and van Sebille, E. (2019). The role of ekman currents, geostrophy, and Stokes drift in the accumulation of floating microplastic. J. Geophys. Res. Oceans 124 (3), 1474–1490. doi:10.1029/2018JC014547
OSPAR Commission (2019). Beach litter monitoring - characteristics of lotter in the marine and coastal environment OSPAR assessment. Available at: https://oap.ospar.org/en/ospar-assessments/committee-assessments/eiha882thematic-assessments/marine-litter/beach-litter-monitoring/.
OSPAR Commission (2020). CEMP Guidelines for marine monitoring and assessment of beach litter OSPAR agreement 2020-02. doi:10.25607/OBP-1728
Paradinas, L. M., James, N. A., Quinn, B., Dale, A., and Narayanaswamy, B. E. (2021). A new collection tool-kit to sample microplastics from the marine environment (sediment, seawater, and biota) using citizen science. Front. Mar. Sci. 8. doi:10.3389/fmars.2021.657709
R Core Team (2020). R: The R project for statistical computing. Vienna, Austria. Available at: https://www.r-project.org/.
Ruiz-Orejon, L. F., Sarda, R., and Ramis-Pujol, J. (2016). Floating plastic debris in the central and western mediterranean sea. Mar. Environ. Res. 120, 136–144. doi:10.1016/j.marenvres.2016.08.001
Ryan, P. G. (2015). Does size and buoyancy affect the long-distance transport of floating debris? Environ. Res. Lett. 10 (8), 084019. doi:10.1088/1748-9326/10/8/084019
Sabatino, A. D., O’Hara Murray, R. B., Hills, A., Speirs, D. C., and Heath, M. R. (2016). Modelling sea level surges in the Firth of Clyde, a fjordic embayment in south-west Scotland. Nat. Hazards (Dordr). 84 (3), 1601–1623. doi:10.1007/s11069-016-2506-7
Siegfried, M., Koelmans, A. A., Besseling, E., and Kroeze, C. (2017). Export of microplastics from land to sea. A modelling approach. Water Res. 127, 249–257. doi:10.1016/j.watres.2017.10.011
Simpson, J. H., and Hill, A. E. (1986). “The scottish coastal current,” in The role of freshwater outflow in coastal marine ecosystems, 295–308.
Skamarock, W. C., Klemp, J. B., Dushia, J., Gill, D. O., Barker, D. M., Duda, M. G., et al. (2008). A description of the advanced research WRF version 3. NCAR Tech. Note 475, 125. doi:10.5065/D68S4MVH
Smagorinsky, J. (1963). General circulation experiements with the primitive equations monthly weather review 91 (3), 99–164. doi:10.1175/1520-0493(1963)091<0099:gcewtp>2.3.co;2
Smith, L., and Turrell, W. R. (2021). Monitoring plastic beach litter by number or by weight: The implications of fragmentation. Front. Mar. Sci. 8, 2. doi:10.3389/fmars.2021.702570
Suaria, G., Melinte-Dobrinescu, M. C., Ion, G., and Aliani, S. (2015). First observations on the abundance and composition of floating debris in the North-Western Black Sea. Mar. Environ. Res. 107, 45–49. doi:10.1016/j.marenvres.2015.03.011
Thompson, R. C., Swan, S. H., Moore, C. J., and vom Saal, F. S. (2009). Our plastic age. Phil. Trans. R. Soc. B 364 (1526), 1973–1976. doi:10.1098/rstb.2009.0054
Topcu, E. N., Tonay, A. M., Dede, A., Ozturk, A. A., and Ozturk, B. (2013). Origin and abundance of marine litter along sandy beaches of the Turkish Western Black Sea Coast. Mar. Environ. Res. 85, 21–28. doi:10.1016/j.marenvres.2012.12.006
Turrell, W. R. (2018). A simple model of wind-blown tidal strandlines: How marine litter is deposited on a mid-latitude, macro-tidal shelf sea beach. Mar. Pollut. Bull. 137, 315–330. doi:10.1016/j.marpolbul.2018.10.024
Turrell, W. R. (2020a). Estimating a regional budget of marine plastic litter in order to advise on marine management measures. Mar. Pollut. Bull. 150, 110725. doi:10.1016/j.marpolbul.2019.110725
Turrell, W. R. (2020b). How litter moves along a macro tidal mid-latitude coast exposed to a coastal current. Mar. Pollut. Bull. 160, 111600. doi:10.1016/j.marpolbul.2020.111600
Turrell, W. R. (2019). Spatial distribution of foreshore litter on the northwest European continental shelf. Mar. Pollut. Bull. 142, 583–594. doi:10.1016/j.marpolbul.2019.04.009
van der Mheen, M., Pattiaratchi, C., and Sebille, E. (2019). Role of Indian ocean dynamics on accumulation of buoyant debris. J. Geophys. Res. Oceans 124 (4), 2571–2590. doi:10.1029/2018jc014806
van der Mheen, M., van Sebille, E., and Pattiaratchi, C. (2020). Beaching patterns of plastic debris along the Indian Ocean rim. Ocean. Sci. 16 (5), 1317–1336. doi:10.5194/os-16-1317-2020
van Sebille, E., Aliani, S., Law, K. L., Maximenko, N., Alsina, J., Bagaev, A., et al. (2020). The physical oceanography of the transport of floating marine debris. Environ. Res. Lett. 15, 023003. doi:10.1088/1748-9326/ab6d7d
Veerasingam, S., Saha, M., Suneel, V., Vethamony, P., Rodrigues, A. C., Bhattacharyya, S., et al. (2016). Characteristics, seasonal distribution and surface degradation features of microplastic pellets along the Goa coast, India. Chemosphere 159, 496–505. doi:10.1016/j.chemosphere.2016.06.056
Watts, A. J. R., Porter, A., Hembrow, N., Sharpe, J., Galloway, T. S., and Lewis, C. (2017). Through the sands of time: Beach litter trends from nine cleaned north Cornish beaches. Environ. Pollut. 228, 416–424. doi:10.1016/j.envpol.2017.05.016
Waziri, M. Y., Saidu, I., and Musa, H. (2010). A simplified derivation and analysis of fourth order Runge Kutta method. Int. J. Comput. Appl. 9 (8), 51–55. doi:10.5120/1402-1891
Williams, A. T., Randerson, P., Di Giacomo, C., Anfuso, G., Macias, A., and Perales, J. A. (2016). Distribution of beach litter along the coastline of Cadiz, Spain. Mar. Pollut. Bull. 107 (1), 77–87. doi:10.1016/j.marpolbul.2016.04.015
Williams, A. T., and Rangel-Buitrago, N. (2019). Marine litter: Solutions for a major environmental problem. J. Coast. Res. 35 (3), 648. doi:10.2112/jcoastres-d-18-00096.1
Woodall, L. C., Robinson, L. F., Rogers, A. D., Narayanaswamy, B. E., and Paterson, G. L. J. (2015). deep-sea litter: A comparison of seamounts, banks and a ridge in the atlantic and Indian oceans reveals both environmental and anthropogenic factors impact accumulation and composition. Front. Mar. Sci. 2. doi:10.3389/fmars.2015.00003
Ye, S., and Andrady, A. L. (1991). Fouling of floating plastic debris under biscayne bay exposure conditions. Mar. Pollut. Bull. 22 (12), 608–613. doi:10.1016/0025-326x(91)90249-r
Keywords: plastic transport, marine litter, hydrodynamic modelling, particle tracking, citizen-science, Clyde Sea, Scotland
Citation: Allison NL, Dale A, Turrell WR, Aleynik D and Narayanaswamy BE (2022) Simulating the distribution of beached litter on the northwest coast of Scotland. Front. Environ. Sci. 10:940892. doi: 10.3389/fenvs.2022.940892
Received: 10 May 2022; Accepted: 20 September 2022;
Published: 03 October 2022.
Edited by:
Andrei Bagaev, Marine Hydrophysical Institute (RAS), RussiaReviewed by:
Erik Van Sebille, Utrecht University, NetherlandsMarcel Ricker, Helmholtz Zentrum Hereon, Germany
Copyright © 2022 Allison, Dale, Turrell, Aleynik and Narayanaswamy. This is an open-access article distributed under the terms of the Creative Commons Attribution License (CC BY). The use, distribution or reproduction in other forums is permitted, provided the original author(s) and the copyright owner(s) are credited and that the original publication in this journal is cited, in accordance with accepted academic practice. No use, distribution or reproduction is permitted which does not comply with these terms.
*Correspondence: Nicole L. Allison, Tmljb2xlLkFsbGlzb25Ac2Ftcy5hYy51aw==