- 1Sustainability Studies Program, School of Marine and Atmospheric Sciences, Stony Brook University, Stony Brook, NY, United States
- 2Département d'Informatique et de Génie Logiciel, Université Laval, Ville de Québec, QC, Canada
Glyphosate, the herbicidal ingredient in Roundup products, can persist in soil for months or years, allowing soil invertebrates ample time to encounter and respond to contamination. While Roundup products can negatively impact earthworm (Eisenia fetida) health, they may also provide a direct or indirect food source. In a set of three experiments, we aimed to determine if Roundup Ready-to-Use III provides a nutritional benefit, damages earthworm health, or both. We used cameras and ant-farm-style enclosures to measure how exposure to a commonly used Roundup formulation impacted earthworm foraging speed as measured by the amount of soil displaced per minute. We also assessed whether contamination drove changes in earthworm body mass and stress test survival time. We found that earthworms living in contaminated soil decreased body mass and displaced more soil per minute relative to earthworms living in non-contaminated soil, suggesting that contamination offered no nutritional benefit. Exposure to contamination did not significantly impact earthworm survival time during a stress test, suggesting weak direct toxicity. Exposure to this contaminant drove a decrease in body mass and increase in movement, which outside of the lab might increase the speed of tunnel formation and microbial dispersal, at a cost to the earthworms. The results of these experiments highlight the need to understand the relationship between Roundup formulations, earthworm behavior and health, and the interplay between earthworm behavior and soil health.
Introduction
Humans depend heavily on the hundreds of glyphosate-based herbicides marketed under the Roundup name. Because of their effectiveness, price, and safety, Roundup herbicides are currently the most frequently used pesticide in the agricultural sector, and the second most frequently used pesticide in urban settings (Battaglin et al., 2014; Myers et al., 2016; Benbrook 2018). They are also commonly used in forestry and in horticulture (Sihtmae et al., 2013; Botten et al., 2021). They have been considered safe because under laboratory conditions, glyphosate can degrade quickly (Moore et al., 1983; Balthazor and Hallas, 1986; Jacob et al., 1988; McAuliffe et al., 1990; Barrett and McBride, 2005; Paudel et al., 2015; Li et al., 2016; la Cecilia et al., 2018), thus posing a low risk for persistence. Glyphosate strongly adsorbs onto soil particles (Al-Rajab and Hakami, 2014; Sidoli et al., 2016), lowering the likelihood of leaching, and glyphosate is less toxic than other herbicides (Gill et al., 2018).
Despite its presumed lack of persistence, Roundup formulations, glyphosate itself, and AMPA (aminomethylphosphonic acid), its major metabolite, occur ubiquitously in our environment. Researchers find glyphosate in our precipitates, streams, and groundwater (Battaglin et al., 2014; Lefrancq et al., 2017; Alonso et al., 2018; la Cecilia et al., 2018; Andrade et al., 2021), our soil (Lane et al., 2012; Aparicio et al., 2013; Battaglin et al., 2014; Alonso et al., 2018; Niemeyer et al., 2018; Silva et al., 2018; Schogl et al., 2022) and our food (Mesnage and Antoniou, 2017; Conrad et al., 2017; Mertens et al., 2018; Gillezeau et al., 2019). Glyphosate remains in forest plant tissues for a decade or more (Botten et al., 2021), glyphosate can persist in soil for months or years, and AMPA degrades even slower (Laitinen et al., 2009; Mertens et al., 2018; Nguyen et al., 2018). Plants translocate glyphosate and its associated surfactants into soil via their root systems where it adsorbs to minerals and becomes available to soil invertebrates (Kremer et al., 2005; Lane et al., 2012; Maqueda et al., 2017; Niemeyer et al., 2018; Silva et al., 2018).
Understanding the impact of Roundup formulations, glyphosate, and AMPA contamination on earthworms and on soil health is critically important since soil ecosystem services are estimated at 11.4 trillion dollars globally, or an average of $867 per hectare across all land uses and soil types (Schon and Dominati, 2020). The contribution of earthworms to soil quality is widely documented in the literature (Edwards and Bohlen, 1996; Brown et al., 2004). They play a critical role in soil formation and nutrient cycling (Van Groenigen et al., 2014), decomposition (Schimel and Schaeffer 2012; Creamer et al., 2015), the recovery of soil carbon pools after natural and anthropogenic disturbance (Angst et al., 2019), maintaining soil microbial diversity (Liu et al., 2019; Liu et al., 2020), controlling plant pathogens (Li et al., 2016; Euteneuer et al., 2019; Plaas et al., 2019), and maintenance of soil porosity (Edwards and Bohlen, 1996; Brown et al., 2004). Earthworms increase the value of land by improving pasture production, increasing the value by $222–1,265 per hectare per year depending on whether dairy cows or sheep were using the pasture. Removing dung from soil improved pasture production, increasing the value by an average of $28- $29 per hectare per year, depending on whether dairy cows or sheep were using the land. Earthworms improve net carbon storage and increase drainage, thereby decreasing flood risks and increasing land value by up to $329 per hectare per year (Schon and Dominati, 2020). One of the biggest threats to soil biological activity as grasslands intensify is the physical degradation of soil which can make it a difficult enclosure for the soil biology to function and contribute to ecosystem services (Greenwood and McKenzie, 2001; Schon et al., 2012). This is why Bender et al. (1984) acknowledged the need to maintain or enhance soil biodiversity to enable proper ecosystem functioning.
The ubiquity of this suite of contaminants—Roundup formulations, glyphosate, and AMPA—may impair the ability of soil invertebrates to deliver their ecosystem services. Earthworms, common soil invertebrates, play an important role in temperate ecosystems, influencing nutrient cycling and ecosystem functioning (Edwards and Bohlen, 1996; Zaller et al., 2014). They shred and redistribute organic material in soil, increase soil penetrability for roots, and improve soil health (Zaller et al., 2014; Gaupp-Berghausen et al., 2015). They also help convert plant-derived lipids and ligands into sugar-based microbial necromass; this conversion increases soil stability, making it denser and gluier in texture (Angst et al., 2019). Earthworms’ contributions to soil health are so critical that the European Union (EU), the Organization for Economic Co-operation and Development (OECD), the International Organization for Standards (ISO), and the Food and Agriculture Organization of the United Nations (FAO) all use compost worms (Eisenia fetida) as an indicator organism for ecotoxicological testing (Piola et al., 2013; Santadino et al., 2014).
Because earthworms possess both highly permeable skin and a highly permeable alimentary tract, they are permanently in close contact with soil contaminants (Jager et al., 2003; Drake and Horn, 2007; Roubalova et al., 2015), and therefore, soil pollutants can significantly impair their health (Jager et al., 2003; Drake and Horn, 2007; Roubalova et al., 2015). Despite a robust and complex immune system (Ghosh 2018), exposure to glyphosate-based herbicides causes tissue damage, lysosomal damage, and cell death in earthworms (Morowati, 2000; Casabé et al., 2007; Correia and Moreira, 2010; Piola et al., 2013; Muangphra et al., 2014; Stanley and Joy, 2014). However, exposure to Roundup formulations, glyphosate and AMPA have a less predictable impact on enzymatic activity, fertility, body mass, and mortality (reviewed in Pochron et al., 2019; Zaller et al., 2021), suggesting that the relationship between exposure to contamination and earthworm health is not always linear or predictable.
Some researchers have suggested that rather than serving as contaminants, Roundup formulations, glyphosate, and/or AMPA may act as a food source for earthworms, either indirectly via the added micronutrients or directly through the addition of fungi or dead plant material to the soil. In an experiment where Roundup exposure led to heavier earthworms, researchers suggested that Roundup application killed soil fungus, providing increased food abundance for the earthworms (Zaller et al., 2014). Gaupp-Berghausen et al. (2015) reported that exposure to a Roundup contaminant initially stimulated earthworm activity, likely as the consequence of an increased availability of dead leaf material caused by the herbicide. That same study reported a substantial increase in soil nitrate and phosphate concentration with Roundup application, which might also stimulate the growth of microbial populations and thereby provide food for earthworms.
One way to determine whether earthworms experience environmental Roundup as a food source is to investigate their foraging behavior. According to optimal foraging theory (Stephens and Krebs 2019), foraging behavior reflects food availability in predictable ways: in habitats where food availability is low, foragers are predicted to move more quickly than in habitats where food availability is high (Lovette and Holmes 1995; Lyons 2005; Beauchamp 2012; Norberg 2021). For example, male American redstarts (Setophaga ruticilla) and semipalmated sandpipers (Calidris pusilla) forage faster in food-scarce environments relative to food-rich environments (Lovette and Holmes 1995; Beauchamp 2012).
In this study, we link an assessment of how exposure to Roundup contamination impacts earthworm body mass and stress test survival time with an assessment of earthworm movement speed. We do this by using cameras to observe the speed at which earthworms displace soil during a 7-day period of exposure, and then quantifying how exposure to a Roundup formulation impacts body mass and stress-test survival time. If earthworms move more slowly in a contaminated environment, optimal foraging theory suggests that they are experiencing a food-rich patch of soil; this inference is strengthened if they concurrently gain body mass. Conversely, if earthworms move more quickly in a contaminated environment relative to the un-contaminated control, they may be experiencing a food-scarce patch of soil, especially if they lose body mass. If the primary effect of exposure to contamination is its toxicity, which requires the engagement of the earthworm immune system (Ghosh 2018), then we propose that stress-test survival time will be shorter for earthworms in the contaminated environment relative to those in the uncontaminated and that those in the contaminated environment will lose body mass relative to those in the uncontaminated environments.
Materials and methods
Overview
We built six ant-farm-style enclosures (described below), and we ran the project over nine consecutive weeks. Each week, three enclosures contained clean soil and three contained contaminated soil, as described below, and on a weekly schedule, we measured 1) amount of soil displaced by earthworms per minute in an enclosure during a 7-day period, 2) change in earthworm mass over a 7-day period, and 3) stress-test survival time for earthworms that had been living in the enclosures for a 7-day period. Each enclosure housed one earthworm, preventing density-dependent effects. Each earthworm was weighed before being placed in an enclosure and then again, at the end of the 7-day period.
Earthworm enclosures
We constructed ant-farm-style enclosures using wood and glass. The completed enclosures measured 26.5 cm × 6.9 cm x 24.3 cm, with two viewing areas, front and back, of 18.0 cm × 16.9 cm each. With an internal width of 2.6 cm, earthworms were always visible from at least one side. See Figure 1.
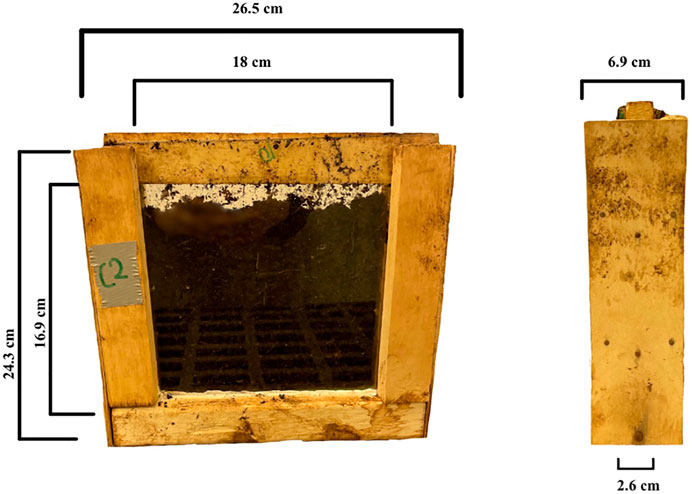
FIGURE 1. The front face of an ant-farm style enclosure used for this experiment. A camera focused on the front face and the back face of each enclosure, and the narrow internal width (2.6 cm) allowed constant visibility within each enclosure.
Soil contamination
We filled each enclosure with OMRI listed Black Gold Garden Compost Blend (Sun Gro Horticulture, Agawam, Massachusetts, United States). OMRI-listed materials are certified by the Organic Materials Review Institute. OMRI is accredited to ISO 17,065 standards by the USDA Quality Assessment Division and ensures that materials used in organic food production, such as the compost used in this project, meet organic standards. This means that the compost used in this experiment was free of fertilizer, pesticides, and animal-care products.
The contaminated enclosures received 26.3 mg of glyphosate per kilogram of soil via Roundup Ready-to-Use III (Bayer Corporation, Whippany, New Jersey, United States), which was purchased at the local garden center. This concentration is consistent with the manufacturer’s instructions (Bayer, 2016) and with that used by other researchers (e.g., Correia and Moreira, 2010; Buch et al., 2013; García-Torres et al., 2014; Pochron et al., 2019, 2020). Enclosures containing contaminated soil thus received 500 g of composted soil, 3 ml of Roundup Ready-to-Use III, and 1 L of Reverse Osmosis water (RO); enclosures containing non-contaminated soil received 505 g compost, 1 L RO water, and 3 ml additional water to ensure equal moisture levels across treatments. To homogenize the soil, water, and contaminant (for the contaminated enclosures), soil was mixed by gloved hand for 10 minutes before it was added to the enclosures. No plants were grown in these enclosures, and no food beyond that provided by the composted soil and contaminant was provided to the earthworms during the 7-day period of this project.
Earthworm body mass
At the beginning of each of the 9 weeks used to run this experiment, we extracted 15 adult earthworms (Eisenia fetida) from the organically raised and maintained stock population described in Pochron et al. (2019). We weighed the earthworms using an American Weigh Scales ACP-200 (accurate to 0.01 g) and selected earthworms so that those living in the contaminated enclosures did not differ in mass from those living in the non-contaminated enclosures, using an ANOVA to test for differences (See Supplementary Table S1). Each enclosure received one earthworm, and enclosures were stored in a Conviron CMP6050 (Controlled Environments Limited, Winnipeg, Canada) growth chamber set to a temperature of 24.2 ± 1.6 °C. The growth chamber was set on a 24-h light cycle to support the camera lighting. At the end of each week, we removed the earthworms from the enclosures and weighed them. To calculate change in body mass, we subtracted the initial from the final body mass. We collected initial body mass, final body mass, and calculated the change in body mass for 27 earthworms in each group. We then measured stress-test survival time as described below.
Stress test survival time
Following Pochron et al. (2017, 2018), the growth chamber programmed to a temperature of 35 °C and light intensity (photon flux) of 250 μmol m2 s−1 was used to provide light and heat stress. Petri dishes containing weighed earthworms were placed into the chamber, exposing them to intense heat and intense light. All Petri dishes were inspected at 5-min intervals to determine the time of death for each earthworm. As per Correia and Moreira (2010) and Pochron et al. (2017), earthworms were classified as dead when they failed to respond to gentle mechanical stimulus. While we planned on collecting stress-test data from 27 earthworms in each treatment, a laboratory error forced us to use only 24 earthworms in each group.
Camera setup
Earthworm movement across enclosure faces was caught by a camera (Arducam Lens Board OV5647 Sensor for Raspberry Pi Camera, Adjustable and Interchangeable Lens M12 Module, Focus and Angle Enhancement for Raspberry Pi 4/3/3 B+, RRID:SCR_022325) connected to a single-board Raspberry Pi 3b + computer via a camera cable strip (Arducam Pi Camera Cable, Octoprint Octopi Webcam, Monitor 3D Printer, 3.28 F T/100CM Long Extension Flex Ribbon Cable). We positioned the cameras 70 cm from the enclosures, arranging them to ensure constant in-focus views of both the enclosures’ fronts and their backs. We programmed a Python script to take photos every 10 min and to store the photos in a one Tb external hard drive attached to each computer. We collected data from January 13th–18 March 2022, producing approximately 1,000 photos per enclosure over each 7-day period. Fluctuations in campus power disrupted the lighting in weeks 4, 5 and 7, reducing the number of images in those weeks.
Speed of earthworm-driven soil displacement
Cameras recorded the value of individual pixels within the image matrix, and when earthworms moved soil, images from adjacent time steps differed from each other, thereby creating variation in pixel values. When earthworms did not move any soil between one photo and the next, pixel values remained unchanged between 10-min time steps. The software subtracted the pixel values found in the time step n from the pixel values in the time step n–1.
To describe the process in greater detail: white pixels in a photo represent a change caused by earthworm-driven soil movement, while black pixels show unmoved soil. The software compared the number of white pixels in one image to the number of white pixels in the consecutive image, yielding the change in pixel number over a 10-min period. Since all cameras had identical resolution and were equidistant from each enclosure, we converted number of pixels into area, where 1.0 pixel = 0.6522 mm2, allowing us to determine how much area earthworms altered per 10-min period. See Figure 2. For each time step, we calculated the area of soil displaced since the previous time step for both the front and the back of the enclosures, and we added those values together.
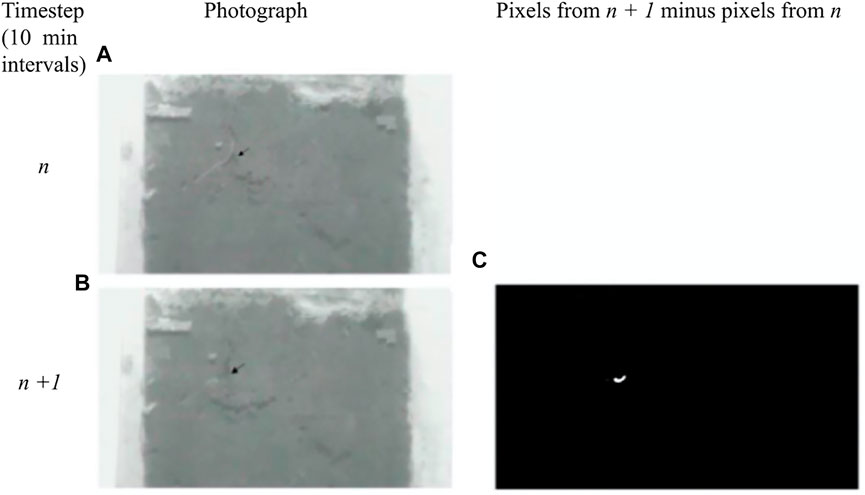
FIGURE 2. Examples of photographs and pixel images generated by this experiment. The cameras photographed enclosure faces once every 10 minutes, as shown in (A) and (B). The earthworm seen in image A had changed its location 10 min later, as shown in image (B). Our software translated the photographs into pixel images and subtracted the number of white pixels in newest image from the number of white pixels in the photo proceeding it, as shown in image (C). The 24 white pixels in C cover an area of 15.7 mm2, showing that earthworms moved 1.57 mm2 of soil per minute.
Initial earthworm size and amount of displaced soil
Variation in initial earthworm body mass, while consistent and small between the two treatments, existed. To ensure that our results were not an artifact of a relationship between body mass and motivation to forage (e.g., smaller earthworms being hungrier and therefore foraging faster), after checking for normality, we ran a regression using initial body mass 7) as the independent variable and total amount of soil displaced on the first day as the dependent variable.
Data analysis
We stored and cleaned data in Microsoft Excel (Version 16.60 RRID:SCR_016137) and conducted statistical tests in StatPlus (StatPlus:mac, AnalystSoft Inc. RRID:SCR_014635) and R. We used a Kolmogorov-Smirnov/Lilliefor Test at the 95% confidence level to check for normality. We used ANOVAs and t-tests to detect differences in means between groups. Means and standard deviations are reported throughout the text. Standard errors of the means are presented in the figures. To further assess the magnitude of differences between two groups on a given variable we calculated effect size, via the Cohen’s d statistic, which assumes that the standard deviations are roughly equal (Cohen, 1988). We used an effect size calculator to find the Cohen’s d (Becker, 2000). To quantify area of soil moved between consecutive photos, we wrote a Python image-subtraction script.
Soil testing
We extracted soil samples from both treatment types and sent them to the Cornell Nutritional Analysis Laboratory (CNAL) for analysis. Specifically, while wearing nitrile gloves, we took 250 ml soil prepared for the uncontaminated enclosures, and we placed it into a Ziplock bag, placed that bag into a second Ziplock bag, and labeled it. We repeated this procedure for the contaminated soil. CNAL measured soil pH, soil carbon via Loss On Ignition (LOI), soil organic matter, and soil micronutrient levels. CNAL calculates pH with an electrode in a 1:1 soil:water suspension, and the value is presented in standard pH units. CNAL calculates percent organic matter via LOI, which measures the change in mass after soil samples are exposed to high temperature (500 °C or 932°F) in a furnace. At these temperatures, carbonaceous materials are oxidized to CO2, while other materials remain. CNAL uses the Modified Morgan extraction procedure for soil testing. The Modified Morgan is a universal extraction procedure used to determine all major nutrients and micronutrients simultaneously. On overview of their methodologies are available in The Cornell Framework (2017).
Results
Change in earthworm body mass
No earthworms in either treatment group died during the 7-days experimental period. To determine the impact of exposure to Roundup Ready-to-Use III on body mass, we assessed change in body mass between the beginning and end of the experiment. Earthworms living in non-contaminated soil for a week slightly increased body mass on average (0.04 g
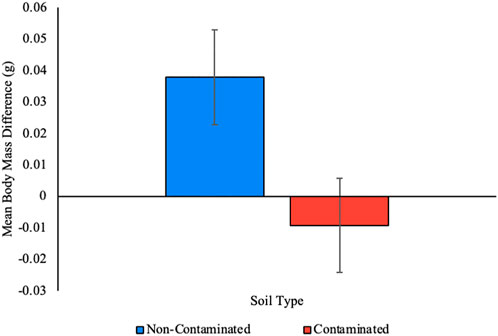
FIGURE 3. The impact of soil contamination on earthworm body mass. Living in contaminated soil for a week causes a slight loss in body mass while living in clean soil causes a slight gain, on average. Error bars represent the standard error of the means. p < 0.05.
Stress-test survival time
Exposure to contamination did not significantly impact stress-test survival time (F = 0.46, DF = 1, 46, p = 0.50). Earthworms that lived in enclosures contaminated with Roundup Ready-to-Use III survived the stress test for a mean of 98.3
Speed of earthworm-driven soil displacement
Because the data for this variable was skewed toward the low values and could not go negative, we used a log10 transformation to calculate the means and perform a two-tailed t-test. We found that earthworms living in non-contaminated soil displaced less soil per minute (36.4 cm2/min) than did earthworms living in soil contaminated with Roundup Ready-to-Use III (55.7 cm2/min). A t-test reports a highly significant difference (t = 17.4, DF = 34,991, p < 2.2e-16). Earthworms living in soil contaminated with Roundup Ready-to-Use III increased the amount of soil displaced per minute by 34.7% relative to earthworms living in non-contaminated soil. The Cohen’s d of 1.6 falls into the “very large” category of difference according to Cohen (1988). (See Figure 4.).
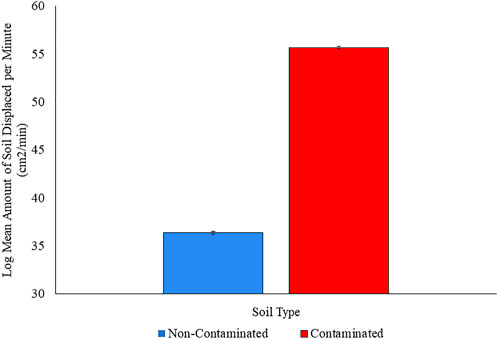
FIGURE 4. Log mean area of soil displaced by earthworms per minute in non-contaminated enclosures and enclosures contaminated with Roundup Ready-to-Use III. Error bars represent the standard error of the means; the standard error of the means is 0.04 for the non-contaminated treatment and 0.12 for the contaminated treatment. p < 0.000001.
Initial earthworm size and amount of displaced soil
Linear regressions show that initial earthworm body mass did not predict amount of soil displaced in the first 24 h of experiment for earthworms in the contaminated or in the non-contaminated enclosures (Non-contaminated: DF = 1, 16; S = 2,368,928.1; F = 0.08, p = 0.78; Contaminated: DF = 1, 16; S = 836,385.8; F = 0.50; p = 0.49). Initial body mass also did not predict the amount of soil displace over the totality of the experiment (Non-contaminated: DF = 1, 16; S = 17,084,450.3; F = 0.07, p = 0.79; Contaminated: DF = 1, 16; S = 6,585,708.3; F = 0.75; p = 0.40).
Soil quality
Adding Roundup Ready-to-Use III at a concentration recommended by the manufacturer and at a concentration used by other researchers does not change the amount of organic matter in soil, soil pH, or soil micronutrients. See Table 1.
Discussion
Understanding the degree to which earthworms experience Roundup-contaminated soil as dangerous or beneficial is an important question because earthworms, which provide a suite of key ecosystem services, live with this contaminant across the globe. This project tested whether contaminated soil could, as proposed by some researchers, benefit earthworms via expansion of the food supply. We did not find support for this hypothesis.
Earthworms living in contaminated soil increased the area of soil displaced per minute by 34.67% relative to earthworms living in un-contaminated soil. This indicates that earthworms moved within contaminated enclosures significantly faster than they did within non-contaminated enclosures. This effect is not driven by initial body mass, and if we interpret this result through the lens of optimal foraging theory (Lovette and Holmes 1995; Lyons 2005; Beauchamp 2012; Stephens and Krebs 2019; Norberg 2021) and assume that natural selection favored earthworms whose behavioral strategies maximized their net energy intake per unit time spent foraging, then increased movement speed indicated that food was scarcer in contaminated than in non-contaminated enclosures (Lovette and Holmes 1995; Lyons 2005; Beauchamp 2012; Norberg 2021). Contaminated and non-contaminated soil did not differ in their percentages of organic material or in micronutrient availability (Table 1), suggesting that food availability was equal in both enclosures, but several researchers have shown that adding glyphosate-based herbicides alters the soil microbial community (Kremer et al., 2005; Schlatter et al., 2018; Meena et al., 2020). Changes in soil microbial communities may change food availability for earthworms, but further studies are needed to verify this hypothesis.
In further support for the hypothesis that adding a glyphosate-based herbicide provided no nutritional benefit to earthworms is the fact that the earthworms in the contaminated enclosures lost body mass while those in the non-contaminated enclosures gained mass, on average. Over a 7-day period, earthworms living in contaminated soil decreased in mass by 2.3% while earthworms living in non-contaminated soil increased in mass by 10.1%. This is consistent with foraging theory-based expectations: earthworms may have moved more in search of unfindable food, metabolizing body mass in their efforts. Earthworms in the non-contaminated enclosures potentially found more food and spent less energy finding it, resulting in an increase in body mass and the displacement of less soil.
Caveats exist, however. While increased motion and decreased body mass are consistent with optimal-foraging-based hypotheses about organisms in food-scarce environments, foraging speed is not determined exclusively by habitat quality. This is why, for example, the foraging speed of prothonotary warblers (Protonotaria citrea) was not associated with food-based habitat quality (Lyons 2005). An ecotoxicology-based explanation may better align with the results.
Coping with environmental contaminants is energetically expensive for invertebrates, and under stress, invertebrates mobilize proteins and lipids as an energy source (Salvio et al., 2016). Internal proteins and lipid concentrations decrease as soil pesticide concentrations increase, indicating high energetic demands under pesticide exposure (Givaudan et al., 2014; Salvio et al., 2016), potentially leading to loss in body mass (Piola et al., 2013). A similar metabolic scenario could be possible for earthworms exposed to soil contaminated with Roundup Ready-to-Use III. A decrease in body mass is a common outcome in ecotoxicological studies (Yasmin 2007; Correia and Moreira 2010; Santos et al., 2011; Garcia-Torres et al., 2014; but see Buch et al., 2013; Piola et al., 2013; Zhou et al., 2013; Santadino et al., 2014; Salvio et al., 2016).
However, earthworms that find themselves needing to pay metabolic costs to cope with contamination can respond via indicators other than body mass. For instance, one earthworm species (Allolobophora chlorotica) increased the activities of enzymes associated with oxidative stress as pesticide concentration increased, while a second (Aporrectodea caliginosa) responded by losing mass (Givaudan et al., 2014). Similarly, when exposed to crumb-rubber contaminated soil, compost earthworms (Eisenia fetida) sometimes maintain growth rates at the cost to resilience as measured by stress-test survival time (Pochron et al., 2017) and sometimes forgo body mass maintenance and invest instead in resilience (Pochron et al., 2018).
In our experiments, living in contaminated soil did not impact earthworm stress-test survival time but did impact earthworm body mass. If Roundup Ready-to-Use III acts as a contaminant that requires metabolic resources to engage immune systems, to remove contaminants, or to repair damaged tissue (Ghosh 2018), then our earthworms might have catabolized body mass for that energy which might then have allowed them to respond to light and heat stress with the same success as the earthworms that did not need to catabolize their body mass.
If the reduced body mass is a response to coping with contamination, then the increase in area of soil displaced per minute might have been driven by attempts to avoid contamination rather than by a need to find food in a resource-scarce environment. Because we homogenized the soil before releasing earthworms into enclosures, earthworms might have not been able to find an uncontaminated position. Researchers frequently report that earthworms actively avoid soil that is contaminated with glyphosate-based herbicides (Verrell, 2004; Casabé et al., 2007; Buch et al., 2013; García-Torres et al., 2014; Salvio et al., 2016; Zaller et al., 2021; but see Santos et al., 2012; Fusilero et al., 2013).
Measures of the impact of glyphosate or glyphosate-based formulations on earthworm behavior are rare. In a series of clever experiments, Zaller and others measured frequency of surface visits as measured by the number of disturbed toothpicks and/or surface castings; they found that application of the herbicide reduced surface visits (Zaller et al., 2014, 2021; Guapp-Bernhausen et al., 2015). However, whether exposure to contamination slowed movement as the contaminant penetrated the soil, or whether the earthworms avoided the surface because it had the highest concentration of contaminant, remains unknown.
Exposure to a common glyphosate-based contaminant both increased the speed with which the earthworms moved through their environment and decreased earthworm body mass. If these results can be extrapolated to behavior outside of a laboratory setting, Roundup contamination might drive an increase in the speed of nutrient cycling, tunnel formation, distribution of organic matter, soil penetrability and the formation of microbial necromasses, at significant cost to the earthworms themselves. If the energy expended in an attempt to escape or metabolize contamination reduces the energy earthworms need to survive and reproduce, the benefit received by the soil will not be sustainable.
Since plants move glyphosate directly into the soil through their roots (Laitinen et al., 2009), and since Roundup use is ubiquitous in agriculture, horticulture, forestry, groundskeeping, and other outdoor industries, many earthworm populations cannot avoid Roundup exposure. Whether earthworms change their behavior and body mass when exposed to this common herbicide due to feeding constraints or in response to pollution, when earthworms could not escape exposure to the herbicide, they increased their movement speed and lost body mass. The glyphosate-based formulation used here, Roundup Ready-to-Use III, did not appear to provide a food source or otherwise increase food availability for earthworms.
If the losses in earthworm body mass discovered here in a laboratory setting scales to a global level, the fact that the majority of our current crops are Roundup Ready and regularly exposed to glyphosate-based herbicides may result in earthworms being decreasingly able to deliver their many ecosystem services and soil biodiversity declining, increasing the challenge of feeding nearly eight billion people. It is critical that we understand the effects of glyphosate on earthworm populations in order to mitigate the damage as effectively as possible.
Data availability statement
The datasets presented in this study can be found in online repositories. The names of the repository/repositories and accession number(s) can be found below: Reserved DOI: 10.17632/49ptgmbsg9.3.
Author contributions
SP supervised the project. SP, MM, and SB designed the study. SB coordinated the protocol execution and success of the project. MM designed the photo-capturing system. CB, MM, and SB designed and wrote the script for data analysis. SB, SS, AC, MK, and AN performed the data collection, analysis, and organization of data. SP and SB performed the data interpretation. SP, MM, and SB wrote the first draft of the manuscript. SP, SB, and SS performed the statistical analysis, coordinated the write-up of this manuscript, and revised the manuscript. SP performed the final approval of the manuscript to be published. All authors reviewed, read, and approved the submitted version of this manuscript.
Funding
This research was funded in part by Stony Brook University’s URECA (Undergraduate Research and Creative Activities) summer Program, which provided a stipend to MM during summer 2019.
Acknowledgments
We thank greenhouse curators Michael Axelrod, John Klumpp, and Sean Halliwell for technical support on this project. We thank Katherine Aubrecht, Director of the Sustainability Studies Program, and Paul Shepson, Dean of the School of Marine and Atmospheric Sciences, for their continued support of undergraduate research opportunities. We thank Kathy Twiss for insightful comments on early drafts of this manuscript. We thank Clara Tucker for statistical support.
Conflict of interest
The authors declare that the research was conducted in the absence of any commercial or financial relationships that could be construed as a potential conflict of interest.
Publisher’s note
All claims expressed in this article are solely those of the authors and do not necessarily represent those of their affiliated organizations, or those of the publisher, the editors and the reviewers. Any product that may be evaluated in this article, or claim that may be made by its manufacturer, is not guaranteed or endorsed by the publisher.
Supplementary material
The Supplementary Material for this article can be found online at: https://www.frontiersin.org/articles/10.3389/fenvs.2022.991494/full#supplementary-material
References
Al-Rajab, A. J., and Hakami, O. M. (2014). Behavior of the non-selective herbicide glyphosate in agricultural soil. Am. J. Environ. Sci. 10 (2), 94–101. doi:10.3844/ajessp.2014.94.101
Alonso, L. L., Demetrio, P. M., Etchegoyen, M. A., and Marino, D. J. (2018). Glyphosate and atrazine in rainfall and soils in agroproductive areas of the pampas region in Argentina. Sci. Total Environ. 645, 89–96. doi:10.1016/j.scitotenv.2018.07.134
Andrade, V. S., Gutierrez, M. F., Regaldo, L., Paira, A. R., Repetti, M. R., and Gagneten, A. M. (2021). Influence of rainfall and seasonal crop practices on nutrient and pesticide runoff from soybean dominated agricultural areas in Pampean streams, Argentina. Sci. Total Environ. 788, 147676. doi:10.1016/j.scitotenv.2021.147676
Angst, G., Mueller, C. W., Prater, I., Angst, Š., Frouz, J., Jílková, V., et al. (2019). Earthworms act as biochemical reactors to convert labile plant compounds into stabilized soil microbial necromass. Commun. Biol. 2 (1), 441–447. doi:10.1038/s42003-019-0684-z
Aparicio, V. C., de Gerónimo, E., Marino, D., Primost, J., Carriquiriborde, P., and Costa, J. L. (2013). Environmental fate of glyphosate and aminomethylphosphonic acid in surface waters and soil of agricultural basins. Chemosphere 93 (9), 1866–1873. doi:10.1016/j.chemosphere.2013.06.041
Balthazor, T. M., and Hallas, L. E. (1986). Glyphosate-degrading microorganisms from industrial activated sludge. Appl. Environ. Microbiol. 51 (2), 432–434. doi:10.1128/aem.51.2.432-434.1986
Barrett, K., and McBride, M. (2005). Oxidative degradation of glyphosate and aminomethylphosphonate by manganese oxide. Environ. Sci. Technol. 39 (23), 9223–9228. doi:10.1021/es051342d
Battaglin, W. A., Meyer, M. T., Kuivila, K. M., and Dietze, J. E. (2014). Glyphosate and its degradation product AMPA occur frequently and widely in U.S. Soils, surface water, groundwater, and precipitation. J. Am. Water Resour. Assoc. 50 (2), 275–290. doi:10.1111/jawr.12159
Bayer, S. (2016). Application reminders for Roundup brand glyphosate agricultural herbicide. AvaliableAt: https://www.roundupreadyplus.com/resourcecenter/application-reminders-for-roundup-brand-agricultural-herbicides (Accessed February 15, 2020).
Beauchamp, G. (2012). Foraging speed in staging flocks of semipalmated sandpipers: Evidence for scramble competition. Oecologia 169 (4), 975–980. doi:10.1007/s00442-012-2269-0
Becker, L. A. (2000). Effect Size Calculators. Available at: https://lbecker.uccs.edu/ (Accessed August 2, 2022).
Benbrook, C. M. (2018). Why regulators lost track and control of pesticide risks: Lessons from the case of glyphosate-based herbicides and genetically engineered-crop technology. Curr. Environ. Health Rep. 5 (3), 387–395. doi:10.1007/s40572-018-0207-y
Bender, E. A., Case, T. J., and Gilpin, M. E. (1984). Perturbation experiments in community ecology: Theory and practice. Ecology 65 (1), 1–13. doi:10.2307/1939452
Botten, N., Wood, L., and Werner, J. (2021). Glyphosate remains in forest plant tissues for a decade or more. For. Ecol. Manage. 493, 119259. doi:10.1016/j.foreco.2021.119259
Brown, G. G., Edwards, C. A., and Brussaard, L. (2004). How earthworms affect plant growth: Burrowing into the mechanisms. Earthworm Ecol. 2, 13–49. doi:10.1201/9781420039719-9
Buch, A. C., Brown, G. G., Niva, C. C., Sautter, K. D., and Sousa, J. P. (2013). Toxicity of three pesticides commonly used in Brazil to Pontoscolex corethrurus (Müller, 1857) and Eisenia andrei (Bouché, 1972). Appl. Soil Ecol. 69, 32–38. doi:10.1016/j.apsoil.2012.12.011
Casabé, N., Piola, L., Fuchs, J., Oneto, M. L., Pamparato, L., Basack, S., et al. (2007). Ecotoxicological assessment of the effects of glyphosate and chlorpyrifos in an Argentine soya field. J. Soils Sediments 7 (4), 232–239. doi:10.1065/jss2007.04.224
Cohen, J. (1988). Statistical power analysis for the behaviors science. 2nd Edn. Hillsdale, NJ: Laurence Erlbaum Associates, Publishers, Hillsdale.
Conrad, A., Schröter-Kermani, C., Hoppe, H. W., Rüther, M., Pieper, S., and Kolossa-Gehring, M. (2017). Glyphosate in German adults–Time trend (2001–2015) of human exposure to a widely used herbicide. Int. J. Hyg. Environ. Health. 220 (1), 8–16.
Correia, F. V., and Moreira, J. C. (2010). Effects of glyphosate and 2, 4-D on earthworms (Eisenia foetida) in laboratory tests. Bull. Environ. Contam. Toxicol. 85 (3), 264–268. doi:10.1007/s00128-010-0089-7
Creamer, C. A., de Menezes, A. B., Krull, E. S., Sanderman, J., Newton-Walters, R., and Farrell, M. (2015). Microbial community structure mediates response of soil C decomposition to litter addition and warming. Soil Biol. Biochem. 80, 175–188. doi:10.1016/j.soilbio.2014.10.008
Drake, H. L., and Horn, M. A. (2007). As the worm turns: The earthworm gut as a transient habitat for soil microbial biomes. Annu. Rev. Microbiol. 61 (1), 169–189. doi:10.1146/annurev.micro.61.080706.093139
Edwards, C., and Bohlen, P. (1996). Biology and ecology of earthworms. London, U.K: Chapman and Hall Press.
Euteneuer, P., Wagentristl, H., Steinkellner, S., Scheibreithner, C., and Zaller, J. G. (2019). Earthworms affect decomposition of soil-borne plant pathogen Sclerotinia sclerotiorum in a cover crop field experiment. Appl. Soil Ecol. 138, 88–93. doi:10.1016/j.apsoil.2019.02.020
Fusilero, M. A., Mangubat, J., Ragas, R. E., Baguinon, N., Taya, H., and Rasco, E. (2013). Weed management systems and other factors affecting the earthworm population in a banana plantation. Eur. J. Soil Biol. 56, 89–94. doi:10.1016/j.ejsobi.2013.03.002
García-Torres, T., Giuffré, L., Romaniuk, R., Ríos, R. P., and Pagano, E. A. (2014). Exposure assessment to glyphosate of two species of annelids. Bull. Environ. Contam. Toxicol. 93 (2), 209–214. doi:10.1007/s00128-014-1312-8
Gaupp-Berghausen, M., Hofer, M., Rewald, B., and Zaller, J. G. (2015). Glyphosate-based herbicides reduce the activity and reproduction of earthworms and lead to increased soil nutrient concentrations. Sci. Rep. 5 (1), 12886–12889. doi:10.1038/srep12886
Ghosh, S. (2018). Environmental pollutants, pathogens and immune system in earthworms. Environ. Sci. Pollut. Res. 25 (7), 6196–6208. doi:10.1007/s11356-017-1167-8
Gill, J. P. K., Sethi, N., Mohan, A., Datta, S., and Girdhar, M. (2018). Glyphosate toxicity for animals. Environ. Chem. Lett. 16 (2), 401–426.
Gillezeau, C., van Gerwen, M., Shaffer, R. M., Rana, I., Zhang, L. P., Sheppard, L., et al. (2019). The evidence of human exposure to glyphosate: A review. Environ. Health 18, 2. doi:10.1186/s12940-018-0435-5
Givaudan, N., Binet, F., Le Bot, B., and Wiegand, C. (2014). Earthworm tolerance to residual agricultural pesticide contamination: Field and experimental assessment of detoxification capabilities. Environ. Pollut. 192, 9–18. doi:10.1016/j.envpol.2014.05.001
Greenwood, K., and McKenzie, B. (2001). Grazing effects on soil physical properties and the consequences for pastures: A review. Aust. J. Exp. Agric. 41 (8), 1231–1250. doi:10.1071/ea00102
Jacob, G., Garbow, J., Hallas, L., Kimack, N., Kishore, G., and Schaefer, J. (1988). Metabolism of glyphosate in Pseudomonas sp. strain LBr. Appl. Environ. Microbiol. 54 (12), 2953–2958. doi:10.1128/aem.54.12.2953-2958.1988
Jager, T., Fleuren, R. H., Hogendoorn, E. A., and De Korte, G. (2003). Elucidating the routes of exposure for organic chemicals in the earthworm, Eisenia andrei (Oligochaeta). Environ. Sci. Technol. 37 (15), 3399–3404. doi:10.1021/es0340578
Kremer, R., Means, N., and Kim, S. (2005). Glyphosate affects soybean root exudation and rhizosphere micro-organisms. Int. J. Environ. Anal. Chem. 85 (15), 1165–1174. doi:10.1080/03067310500273146
la Cecilia, D., Tang, F. H., Coleman, N. V., Conoley, C., Vervoort, R. W., and Maggi, F. (2018). Glyphosate dispersion, degradation, and aquifer contamination in vineyards and wheat fields in the Po Valley, Italy. Water Res. 146, 37–54. doi:10.1016/j.watres.2018.09.008
Laitinen, P., Rämö, S., Nikunen, U., Jauhiainen, L., Siimes, K., and Turtola, E. (2009). Glyphosate and phosphorus leaching and residues in boreal sandy soil. Plant Soil 323 (1-2), 267–283. doi:10.1007/s11104-009-9935-y
Lane, M., Lorenz, N., Saxena, J., Ramsier, C., and Dick, R. P. (2012). The effect of glyphosate on soil microbial activity, microbial community structure, and soil potassium. Pedobiologia 55 (6), 335–342. doi:10.1016/j.pedobi.2012.08.001
Lefrancq, M., Jadas-Hécart, A., La Jeunesse, I., Landry, D., and Payraudeau, S. (2017). High frequency monitoring of pesticides in runoff water to improve understanding of their transport and environmental impacts. Sci. Total Environ. 587, 75–86. doi:10.1016/j.scitotenv.2017.02.022
Li, H., Joshi, S. R., and Jaisi, D. P. (2016). Degradation and isotope source tracking of glyphosate and aminomethylphosphonic acid. J. Agric. Food Chem. 64 (3), 529–538. doi:10.1021/acs.jafc.5b04838
Liu, M., Cao, J., and Wang, C. (2020). Bioremediation by earthworms on soil microbial diversity and partial nitrification processes in oxytetracycline-contaminated soil. Ecotoxicol. Environ. Saf. 189, 109996. doi:10.1016/j.ecoenv.2019.109996
Liu, T., Chen, X., Gong, X., Lubbers, I. M., Jiang, Y., Feng, W., et al. (2019). Earthworms coordinate soil biota to improve multiple ecosystem functions. Curr. Biol. 29 (20), 3420–3429. e3425. doi:10.1016/j.cub.2019.08.045
Lovette, I. J., and Holmes, R. T. (1995). Foraging behavior of American redstarts in breeding and wintering habitats: Implications for relative food availability. Condor 97 (3), 782–791. doi:10.2307/1369186
Lyons, J. E. (2005). Habitat-specific foraging of prothonotary warblers: Deducing habitat quality. Condor 107 (1), 41–49. doi:10.1093/condor/107.1.41
Maqueda, C., Undabeytia, T., Villaverde, J., and Morillo, E. (2017). Behaviour of glyphosate in a reservoir and the surrounding agricultural soils. Sci. Total Environ. 593-594, 787–795. doi:10.1016/j.scitotenv.2017.03.202
McAuliffe, K. S., Hallas, L. E., and Kulpa, C. F. (1990). Glyphosate degradation byAgrobacterium radiobacter isolated from activated sludge. J. Ind. Microbiol. 6 (3), 219–221. doi:10.1007/bf01577700
Meena, R. S., Kumar, S., Datta, R., Lal, R., Vijayakumar, V., Brtnicky, M., et al. (2020). Impact of agrochemicals on soil microbiota and management: A review. Land 9 (2), 34. doi:10.3390/land9020034
Mertens, M., Höss, S., Neumann, G., Afzal, J., and Reichenbecher, W. (2018). Glyphosate, a chelating agent—Relevant for ecological risk assessment? Environ. Sci. Pollut. Res. 25 (6), 5298–5317. doi:10.1007/s11356-017-1080-1
Mesnage, R., and Antoniou, M. N. (2017). Facts and fallacies in the debate on glyphosate toxicity. Front. Public Health 5, 316. doi:10.3389/fpubh.2017.00316
Moore, J. K., Braymer, H. D., and Larson, A. D. (1983). Isolation of a Pseudomonas sp. which utilizes the phosphonate herbicide glyphosate. Appl. Environ. Microbiol. 46 (2), 316–320. doi:10.1128/aem.46.2.316-320.1983
Morowati, M. (2000). Histochemical and histopathological study of the intestine of the earthworm (Pheretima elongata) exposed to a field dose of the herbicide glyphosate. Environmentalist 20 (2), 105–111. doi:10.1023/A:1006704009184
Muangphra, P., Kwankua, W., and Gooneratne, R. (2014). Genotoxic effects of glyphosate or paraquat on earthworm coelomocytes. Environ. Toxicol. 29 (6), 612–620. doi:10.1002/tox.21787
Myers, J. P., Antoniou, M. N., Blumberg, B., Carroll, L., Colborn, T., Everett, L. G., et al. (2016). Concerns over use of glyphosate-based herbicides and risks associated with exposures: A consensus statement. Environ. Health 15 (1), 19–13. doi:10.1186/s12940-016-0117-0
Nguyen, N. K., Dörfler, U., Welzl, G., Munch, J. C., Schroll, R., and Suhadolc, M. (2018). Large variation in glyphosate mineralization in 21 different agricultural soils explained by soil properties. Sci. Total Environ. 627, 544–552. doi:10.1016/j.scitotenv.2018.01.204
Niemeyer, J. C., de Santo, F. B., Guerra, N., Ricardo Filho, A. M., and Pech, T. M. (2018). Do recommended doses of glyphosate-based herbicides affect soil invertebrates? Field and laboratory screening tests to risk assessment. Chemosphere 198, 154–160. doi:10.1016/j.chemosphere.2018.01.127
Norberg, R. Å. (2021). To minimize foraging time, use high-efficiency, energy-expensive search and capture methods when food is abundant but low-efficiency, low-cost methods during food shortages. Ecol. Evol. 11 (23), 16537–16546. doi:10.1002/ece3.8204
Paudel, P., Negusse, A., and Jaisi, D. P. (2015). Birnessite‐catalyzed degradation of glyphosate: A mechanistic study aided by kinetics batch studies and nmr spectroscopy. Soil Sci. Soc. Am. J. 79 (3), 815–825. doi:10.2136/sssaj2014.10.0394
Piola, L., Fuchs, J., Oneto, M. L., Basack, S., Kesten, E., and Casabé, N. (2013). Comparative toxicity of two glyphosate-based formulations to Eisenia andrei under laboratory conditions. Chemosphere 91 (4), 545–551. doi:10.1016/j.chemosphere.2012.12.036
Plaas, E., Meyer-Wolfarth, F., Banse, M., Bengtsson, J., Bergmann, H., Faber, J., et al. (2019). Towards valuation of biodiversity in agricultural soils: A case for earthworms. Ecol. Econ. 159, 291–300. doi:10.1016/j.ecolecon.2019.02.003
Pochron, S., Choudhury, M., Gomez, R., Hussaini, S., Illuzzi, K., Mann, M., et al. (2019). Temperature and body mass drive earthworm (Eisenia fetida) sensitivity to a popular glyphosate-based herbicide. Appl. Soil Ecol. 139, 32–39. doi:10.1016/j.apsoil.2019.03.015
Pochron, S., Nikakis, J., Illuzzi, K., Baatz, A., Demirciyan, L., Dhillon, A., et al. (2018). Exposure to aged crumb rubber reduces survival time during a stress test in earthworms (Eisenia fetida). Environ. Sci. Pollut. Res. 25 (12), 11376–11383. doi:10.1007/s11356-018-1433-4
Pochron, S., Simon, L., Mirza, A., Littleton, A., Sahebzada, F., and Yudell, M. (2020). Glyphosate but not Roundup harms earthworms (Eisenia fetida). Chemosphere 241, 125017. doi:10.1016/j.chemosphere.2019.125017
Pochron, S. T., Fiorenza, A., Sperl, C., Ledda, B., Lawrence Patterson, C., Tucker, C. C., et al. (2017). The response of earthworms ( Eisenia fetida ) and soil microbes to the crumb rubber material used in artificial turf fields. Chemosphere 173, 557–562. doi:10.1016/j.chemosphere.2017.01.091
Roubalova, R., Prochazkova, P., Dvorak, J., Skanta, F., and Bilej, M. (2015). The role of earthworm defense mechanisms in ecotoxicity studies. Invertebr. Surviv. J. 12, 203–213.
Salvio, C., Menone, M. L., Rafael, S., Iturburu, F. G., and Manetti, P. L. (2016). Survival, reproduction, avoidance behavior and oxidative stress biomarkers in the earthworm Octolasion cyaneum exposed to glyphosate. Bull. Environ. Contam. Toxicol. 96 (3), 314–319. doi:10.1007/s00128-015-1700-8
Santadino, M., Coviella, C., and Momo, F. (2014). Glyphosate sublethal effects on the population dynamics of the earthworm Eisenia fetida (Savigny, 1826). Water Air Soil Pollut. 225 (12), 2207. doi:10.1007/s11270-014-2207-3
Santos, M. J. G., Ferreira, M. F. L., Cachada, A., Duarte, A. C., and Sousa, J. P. (2012). Pesticide application to agricultural fields: Effects on the reproduction and avoidance behaviour of Folsomia candida and Eisenia andrei. Ecotoxicology 21 (8), 2113–2122. doi:10.1007/s10646-012-0963-7
Santos, M. J. G., Ferreira, V., Soares, A. M. V. M., and Loureiro, S. (2011). Evaluation of the combined effects of dimethoate and spirodiclofen on plants and earthworms in a designed microcosm experiment. Appl. Soil Ecol. 48 (3), 294–300. doi:10.1016/j.apsoil.2011.04.009
Schimel, J., and Schaeffer, S. (2012). Microbial control over carbon cycling in soil. Front. Microbiol. 3, 348. doi:10.3389/fmicb.2012.00348
Schlatter, D. C., Yin, C. T., Burke, I., Hulbert, S., and Paulitz, T. (2018). Location, root proximity, and glyphosate-use history modulate the effects of glyphosate on fungal community networks of wheat. Microb. Ecol. 76 (1), 240–257. doi:10.1007/s00248-017-1113-9
Schlögl, J., Wimmer, B., Cramaro, L., Wirsching, J., Poll, C., Pagel, H., et al. (2022). Heavy rainfall following a summer drought stimulates soil redox dynamics and facilitates rapid and deep translocation of glyphosate in floodplain soils. Environ. Sci. Process. Impacts 24, 825–838. doi:10.1039/d1em00527h
Schon, N., and Dominati, E. (2020). Valuing earthworm contribution to ecosystem services delivery. Ecosyst. Serv. 43, 101092. doi:10.1016/j.ecoser.2020.101092
Schon, N., Mackay, A., and Minor, M. (2012). Relationship between food resource, soil physical condition, and invertebrates in pastoral soils. Soil Sci. Soc. Am. J. 76 (5), 1644–1654. doi:10.2136/sssaj2011.0375
Sidoli, P., Baran, N., and Angulo-Jaramillo, R. (2016). Glyphosate and AMPA adsorption in soils: Laboratory experiments and pedotransfer rules. Environ. Sci. Pollut. Res. 23 (6), 5733–5742. doi:10.1007/s11356-015-5796-5
Sihtmäe, M., Blinova, I., Künnis-Beres, K., Kanarbik, L., Heinlaan, M., and Kahru, A. (2013). Ecotoxicological effects of different glyphosate formulations. Appl. Soil Ecol. 72, 215–224. doi:10.1016/j.apsoil.2013.07.005
Silva, V., Montanarella, L., Jones, A., Fernández-Ugalde, O., Mol, H. G., Ritsema, C. J., et al. (2018). Distribution of glyphosate and aminomethylphosphonic acid (AMPA) in agricultural topsoils of the European Union. Sci. Total Environ. 621, 1352–1359. doi:10.1016/j.scitotenv.2017.10.093
Stanley, O. N., and Joy, O. A. (2014). Histopathological effects of glyphosate and its toxicity to the earthworm Nsukkadrilus mbae. Br. Biotechnol. J. 4 (2), 149–163. doi:10.9734/bbj/2014/6727
Stephens, D. W., and Krebs, J. R. (2019). Foraging theory. Foraging theory. Princeton, NJ: Princeton University Press in Princeton.
The Cornell Framework (2017). Comprehensive assessment of soil health. 3rd edition. Cornell University. Available at: http://soilhealth.cals.cornell.edu/trainingmanual/ (Accessed August, 2022).
Van Groenigen, J. W., Lubbers, I. M., Vos, H. M., Brown, G. G., De Deyn, G. B., and Van Groenigen, K. J. (2014). Earthworms increase plant production: A meta-analysis. Sci. Rep. 4 (1), 6365–6367. doi:10.1038/srep06365
Verrell, P. A. V. B., E., and Van Buskirk, E. (2004). As the worm turns: Eisenia fetida avoids soil contaminated by a glyphosate-based herbicide. Bull. Environ. Contam. Toxicol. 72219, 219. doi:10.1007/s00128-003-9134-0
Yasmin, S. a. D. S., D., and D’Souza, D. (2007). Effect of pesticides on the reproductive output of Eisenia fetida. Bull. Environ. Contam. Toxicol. 75 (5), 529–532. doi:10.1007/s00128-007-9269-5
Zaller, J. G., Heigl, F., Ruess, L., and Grabmaier, A. (2014). Glyphosate herbicide affects belowground interactions between earthworms and symbiotic mycorrhizal fungi in a model ecosystem. Sci. Rep. 4 (1), 5634–5638. doi:10.1038/srep05634
Zaller, J. G., Weber, M., Maderthaner, M., Gruber, E., Takacs, E., Mortl, M., et al. (2021). Effects of glyphosate-based herbicides and their active ingredients on earthworms, water infiltration and glyphosate leaching are influenced by soil properties. Environ. Sci. Eur. 33 (1), 51. doi:10.1186/s12302-021-00492-0
Keywords: glyphosate, roundup, optimal foraging theory, movement speed, compost worm, body mass, resilience
Citation: Pochron ST, Mezic M, Byrne S, Sasoun S, Casamassima A, Kilic M, Nuzzo A and Beaudet C-E (2022) Exposure to Roundup increases movement speed and decreases body mass in earthworms. Front. Environ. Sci. 10:991494. doi: 10.3389/fenvs.2022.991494
Received: 11 July 2022; Accepted: 07 September 2022;
Published: 23 September 2022.
Edited by:
Liangang Mao, Institute of Plant Protection (CAAS), ChinaReviewed by:
Andrey S. Zaitsev, University of Giessen, GermanyQingming Zhang, Qingdao Agricultural University, China
Copyright © 2022 Pochron, Mezic, Byrne, Sasoun, Casamassima, Kilic, Nuzzo and Beaudet. This is an open-access article distributed under the terms of the Creative Commons Attribution License (CC BY). The use, distribution or reproduction in other forums is permitted, provided the original author(s) and the copyright owner(s) are credited and that the original publication in this journal is cited, in accordance with accepted academic practice. No use, distribution or reproduction is permitted which does not comply with these terms.
*Correspondence: Sharon T Pochron, U2hhcm9uLnBvY2hyb25Ac3Rvbnlicm9vay5lZHU=
†These authors have contributed equally to this work and share second authorship