- 1Gund Institute for Environment, University of Vermont, Burlington, VT, United States
- 2University of Vermont Extension, University of Vermont, Burlington, VT, United States
- 3American Farmland Trust, Northampton, MA, United States
Modifications to continuous corn production systems can reduce environmental impacts and soil degradation, yet the social viability of these modifications is linked to the degree to which they also influence yields and crop quality. In this study, we focus on forage production systems and evaluate how yields, crop quality, soil health indicators, and associated ecosystem services are influenced by corn-hay rotation treatments, cover cropping, and tillage reduction in silage production using a unique 10-year dataset from Borderview Research Farm in Vermont, United States. Physical, chemical, and biological soil health indicators were monitored annually alongside yields and crop quality in a randomized complete block design experiment. We use a mixed model analysis of variance approach to demonstrate significant influences of time and treatments on yields, crop quality and soil health parameters (at p < 0.05). The winter rye cover crop treatment had no significant influence in this study. No-till significantly increased aggregate stability and had no significant effect on other metrics. When cover crop and no-till were combined, they significantly increased soil organic matter content, respiration and aggregate stability. The cover crop, no-till, and no-till cover crop combination treatments had no significant effect on yields or forage quality, suggesting these conservation practices can be adopted without sacrificing yields. Our study also found that corn-hay rotations can significantly increase soil organic matter, respiration, aggregate stability, and crude protein content compared to continuous corn, but they can negatively influence active carbon, total dry matter yield and digestibility. The length of rotation influences the degree to which corn-hay rotations maintain or reduce yields when compared to continuous corn. Shorter rotations of perennial forages (4 years of hay, 6 years of corn) can sustain dry matter yields that are not significantly different from continuous corn, but longer perennial forage rotations (8 years of hay, 2 years of corn) will significantly reduce overall dry matter yields. Among the treatments, no-till in combination with cover cropping in corn silage fields, and a rotation of 4 years of hay to 6 years of corn are likely to achieve the greatest overall benefits in forage production systems.
1 Introduction
Human resource use currently exceeds planetary boundaries (O’Neill et al., 2018) and the sustainable intensification of agriculture is one of the most important pathways to ensure a livable future for humankind. Sustainable intensification broadly refers to increasing or sustaining the production of food while reducing inputs and sustaining the natural resource base (FA0, 2004) and while there are debates about the nuances of this definition, there is consensus that attempts to meet goals of sustainable intensification are rife with tradeoffs (Struik and Kuyper, 2017). Each production type, climate and food system context present different challenges and opportunities to meet goals of sustainability.
In recent years there has been renewed interest in the reintegration of crop and livestock systems as an alternative to continuous annual crop production. This diversification is hoped to sustainably intensify food production while benefiting producer income, crop production, soil properties, and increase environmental and socioeconomic resilience (Kumar et al., 2019). Benefits include closing the loop in nutrient cycles through the provision of manure, improving soil structure and water retention, and decreasing biocide requirements (Garrett et al., 2017). Integrating crops and livestock has been the norm in agricultural history, however, shifts in agricultural research and policy since the industrial revolution have resulted in more specialized and segregated approaches (Garrett et al., 2017). For example, United States farms were substantially more diversified and integrated in the 1970s than they are today. In 1974, 52% of the agricultural area and 19% of the farms utilized a crop-grazing rotation (USDA, 2007), but by 2012, this applied to only 7% of the farms and <2% of the area. Remote sensing studies confirm this trend toward homogenization, with mixed use areas being rapidly converted to continuous annual crops (Lark et al., 2015; Garrett et al., 2017).
The Intergovernmental Panel on Climate Change (IPCC) has identified crop-livestock integration as a resource-efficient and cost-effective agricultural adaptation strategy to sustainably maintain or increase food production (IPCC, 2018). Integrated crop-livestock systems have been shown to increase soil quality, crop yield, and economic returns compared to monoculture crop production in the Unites States (Sekaran et al., 2021). The majority of research to date has focused on the impacts of rotating annual crop production with grazing of forage crops, cover crops, crop residues, and winter grazing with summer crop production (Kumar et al., 2019). However, region-specific issues have a major impact on how crop-livestock systems can be implemented. For example, in colder climates with a short grazing season, livestock performance is strongly related to winter feed management, which can be a significant cost. Stored forage production (such as hay, haylage, and corn silage) is therefore an integral aspect of crop-livestock integration in these climates (Kumar et al., 2019). Yet, the practice of rotating annual crops such as corn with perennial forage has been limited in the United States, and the costs and benefits of these systems are presently understudied.
Aligning production goals with environmental sustainability is critical to achieving sustainable intensification, and environmental stewardship is one of many factors considered by farmers when making agricultural management decisions, alongside financial limitations which constrain their options (White A. et al., 2021; White et al., 2022a). Agricultural sustainability goals can be achieved alongside environmental sustainability goals through improvements in soil health (Neher et al., 2022). Soil health is defined as the continued capacity of a soil to function as a vital living ecosystem that sustains plants, animals, and humans (USDA-NRCS, 2022). This definition acknowledges the role that biological processes play in influencing dynamic soil properties and soil functions that are foundational to sustainability (Neher et al., 2022). Measures of biological, physical and chemical soil health parameters are now widely used as indicators of ecosystem functions and ecosystem services from changes in farming practices and can help assess the effectiveness of conservation practices in meeting multiple goals (Wall et al., 2012; Abbott and Manning, 2015; Adhikari and Hartemink, 2016; White A. et al., 2021; White A. C. et al., 2021; Neher et al., 2022).
The integration of conservation practices, crop diversification, or crop rotation may help farmers protect against weather-related crop stress and increase crop yield while improving soil health and underlying ecosystem services (Nunes et al., 2018; Page et al., 2020; Yang et al., 2020). For example, practices that increase organic matter can mitigate flood damage through increased water holding capacity (Bhadha et al., 2017), increased aggregate stability can decrease erosion (Barthès and Roose, 2017), soil organic carbon increases can contribute to mitigating climate change (Lal et al., 1999) and cover crops can reduce erosion by providing physical cover (De Baets, et al., 2011). However, the nuances of site conditions, soil texture, and the way conservation practices are implemented influence these outcomes, meaning that both synergies and tradeoffs are possible (Palm et al., 2014; Nunes et al., 2018; Page et al., 2020). Context-specific and usable information tailored to realistic production methods, and relevant information on cropping system modifications and subsequent influence on yield and ecosystem services, are needed in every region of the world to support farmers’ decisions.
Corn silage and perennial grass/legume forage cropping systems are prevalent across most dairy producing agricultural areas such as Vermont, United States. For example, corn silage covers 65,560 ha in Vermont, accounting for 13.5% of the agricultural landscape (USDA, 2021). Hay and pasture cover 22.9% (111.290 ha) of the agricultural land (USDA, 2021). Dairy operations feed a mix of annual and perennial forages to meet the dietary requirements of cows, often including a hay, haylage, corn silage, pasture, and various grain concentrates. Conventional management of corn for silage in these systems is characterized as continuous corn planted at a high seeding density. Unlike corn grown for grain, the production of corn silage requires the entire aboveground biomass to be harvested and removed from the field, leaving no crop residue to protect the soil from weather elements through the fall, winter, and spring before the next crop is planted. This can lead to significant soil erosion and declining soil organic matter levels over time (Balík et al., 2020). The sustainable intensification of these annual forage systems has been challenging in Northern New England, primarily a result of a short growing season and difficult landscape. Heavy, cold, and wet soils in the spring encourage spring tillage, providing limited opportunity for adoption of no-till. Rocky and steep slopes limit the land-base available for corn and hay rotations, leading to the best soils being reserved for continuous corn. Silage corn varieties typically require 2,200–2,800 growing degree units (GDUs) to reach maturity and the 30-year average GDD (base 10 °C) in Burlington, Vermont is 2,549 (NOAA, 2022). This means that the time available after harvest to implement conservation practices and establish a cover crop before winter is very limited. In the coming years, variability in precipitation patterns associated with climate change may mean fewer field working days due to wet soil conditions (Tomasek et al., 2017), exacerbating challenges associated with an already short, cold and wet growing season.
In light of these challenges, greater adoption of forage crop rotation, no-till, and cover cropping, may help farmers simultaneously increase soil health, enhance resilience to climate change, and stabilize yields for forage management in integrated livestock systems. Rotation from annual to perennial production systems can increase soil health, and rotating from perennial to annual can give a boost in yields (Stanger and Lauer, 2008; Undersander and Barnett, 2008; Darby et al., 2019). Hay and pasture fields in Vermont have higher soil carbon socks and soil health than corn fields (White et al., 2022b). Extensive research has found that cover cropping increases the overall health of agricultural systems by scavenging excess nutrients (Clark, 2010), increasing water infiltration (Haruna et al., 2018), reducing surface runoff (De Baets, et al., 2011), alleviating compaction pressure (Chen and Weil, 2011), building soil tilth, increasing biodiversity, and building organic matter. Furthermore, cover cropping can help enhance the benefits of other farming practices, such as no-till planting, creating synergies that increase farm financial and environmental sustainability. For example, Sapkota et al. (2012) found that compared to conventional till, no-till systems had 1.12% higher soil organic matter content, 71% more soil microbial biomass, 44% higher soil respiration, greater arthropod abundance, and improved soil structure stability. Long-term no-till corn in the northeastern United States has demonstrated soil health benefits alongside yield increases or maintenance of yields (Nunes et al., 2018).
The goal of this study was therefore to evaluate forage crop yield and quality and soil health metrics in alternative cropping systems that incorporate annual and perennial forage crop rotation, cover crops, and no-tillage, compared to continuous corn silage. We present the results of long-term research on annual production systems in northern New England United States with a novel focus on forage production, which is a critical element of crop-livestock integration in this region. Using a unique 10-year dataset from Borderview Research Farm in Vermont, United States, we evaluate the degree to which these conservation practices and perennial-annual rotations influence dimensions of soil conservation, ecosystem service provisioning and forage production over time. We hypothesize that integrating perennial forage rotations, cover crops, and no till will improve soil health indicators, carbon sequestration, and crop quality and yield in continuous corn production systems, but that temporal tradeoffs and synergies are likely to exist when implementing these practices.
2 Materials and methods
2.1 Study design
Our research draws on data from a long-term replicated plot research trial on corn cropping systems which was established at Borderview Research Farm in Vermont, United States. The experiment was established in 2009. Soil health data was collected alongside yields starting in 2012 and monitored annually until 2021. Forage quality was analyzed starting in 2014 and evaluated annually until 2021.
The experimental design was a randomized complete block with replicated treatments of corn grown in various cropping systems (Table 1). In 2009, there were three treatments each in 6.10 × 15.2 m plots. Four plots were continuous corn (CC 2009) which reflects typical agronomic practices of that time with tillage and no-cover crops. Another four plots were continuous corn with over wintering cover crop (WCCC 2009) which reflects a rotation of continuous tilled corn planted with fall cover crop. There were 12 perennial forage (PF) plots that were planted with a mixture of alfalfa and meadow fescue in 2008. In 2011, a fourth treatment was added when four PF plots were transitioned to no-till corn plots (NT 2011) which reflects continuous corn with no-till practices. The two final treatments (fifth and sixth treatments) are corn-hay rotations with similar management patterns, but in staggered rotation years. In the first rotation (ROTC 2014) four PF plots were transitioned to new corn plots in 2014, and then seeded into PF again in 2020. The second rotation treatment (ROTC 2020) was in PF until it was rotated into corn in 2020. Additionally, in the fall of 2020 the NT plots were split (6.05 × 15.2 m) to maintain NT plots and introduce plots with combined no-till and cover crop practices which will benefit future research.
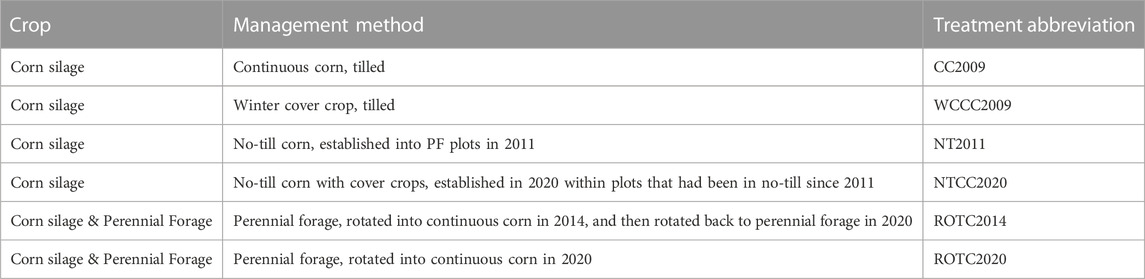
TABLE 1. Corn cropping system treatments evaluated for yield, forage quality and soil health in this study.
2.1.1 Site description
This research takes place in Alburgh, VT (long. 45.009072, lat. −73.307,830) on Amenia silt loam soil (loam skective, mesic, Lithic Eutrudepts). According to the Köppen climate classification system, this region is “humid continental mild summer, wet all year” (Dfb) (PlantMaps, 2022). The 30-year average temperature is 8.22 °C with a 30-year average of 2,625 growing degree days (base 10.0 °C). The 30-year average precipitation amount is 95.0 cm with an additional 216 cm of snow (base 10.0 °C) (NOAA, 2022). The average 30-year winter temperature (December-February) is −4.94 °C (NOAA, 2022).
2.1.2 Field practices
The CC 2009, WCCC 2009, ROTC 2014, and ROTC2020 plots were tilled between 6-May and 16-May with Pottinger Terra Disc (Valparaiso, IN) at 20–25 cm depth for field bed preparation and weed control when in corn. Corn was planted between 7-May and 25-May at an average rate of 84,000 seeds hectare-1 in 76 cm rows with a John Deere 1750 corn planter (Moline, IL). In 2018, a planter malfunction resulted in a seeding rate of 42,000 seeds ha-1. Silage varieties varied over the years with an average relative maturity (RM) of 94, minimum of 86 and maximum of 105. Typical corn starter was applied 4, 8, 8 kg ha-1 of nitrogen N), phosphorus P), and potassium K), respectively. Corn was side-dressed according to the highest nitrate soil test result which was an average of 125 kg ha-1 N when corn was in the V4 stage (between 17-Jun and 5-Jul). Winter cover crop was typically planted in late September with cereal rye (Secale cereale) at a rate of 110 kg ha-1. Cover crops were terminated in NTCC2020 plots with herbicide before corn planting. For early season weed control, herbicide was applied between 14-May and 5-Jun. Later season weed control was achieved through spot spraying with systemic herbicides. Corn was typically harvested between 3-Sep and 18 September It should be noted that in 2020, corn in the ROTC2020 plots was planted after first cut of PF and thus with a later planting date, matured later and had a later harvest date (29-Sep) than any of the other corn plots which were harvested on 3-Sep).
The PF (perennial forage) plots were established with 16.0 kg ha-1 mixture of 30% alfalfa and 70% tall fescue. On 6-May 2020, PF in the ROTC2014 plots was established with 22.5 kg ha-1 mixture of 60% alfalfa and 40% tall fescue. Typically, forage plots were fertilized with an application of 4, 8, 8 kg ha-1 of N, P, and K, respectively after first cut and 60.5 kg ha-1 K after second cut. With the exception of a rotation year into corn where only first cut was taken (ROTC 2020) or rotation into perennial forage (ROTC 2014) when harvest occurred only twice during the first 2 years to encourage establishment of the new forage seeding. Perennial forage plots were harvested three times a year between late May and mid-September.
2.1.3 Sampling and analysis procedures
Indicators of soil health were measured annually. Numerous approaches to assessment of soil health have been developed that move beyond chemical analyses to include biological and physical indicators as well. Our study used the widely adopted Comprehensive Assessment of Soil Health developed by Cornell University’s Soil Health Lab to measure a suite of biological, chemical and physical indicators of soil health (Meobius-Clune et al., 2016). Soil samples were collected from each plot between 26-April and 15-May annually from 2012 to 2021 using the methods described in Moebius-Clune et al. (2016). Composite soil samples were then submitted to the Cornell Soil Health Laboratory for the Comprehensive Assessment of Soil Health (CASH) analysis (Ithaca, NY).
Percent aggregate stability was measured by Cornell Sprinkle Infiltrometer and indicates ability of soil to resist erosion. Predicted percent available water capacity and predicted soil protein (N mg/soil g) was calculated with a Random Forest model from a suite of measured parameters and soil texture (van Es et al., 2019). Percent organic matter was measured by loss on ignition when soils are dried at 105 °C to remove water then ashed for two hours at 500 °C (CASH adaptation from Broadbent, 1965). Total nitrogen is measured with DUMAS combustion methodology. It measured organic (living and non-living) and inorganic (mineral) forms of nitrogen. Active carbon (active C mg/soil kg) was measured with potassium permanganate and is used as an indicator of available carbon (i.e., food source) for the microbial community. Soil respiration (CO2 mg/soil g) is measured by amount of CO2 released over a four-day incubation period and is used to quantify metabolic activity of the soil microbial community (Zibilske, 1994).
Corn silage was harvested with a John Deere 2-row chopper (Moline, IL) and yields weighed in a wagon fitted with scales. Perennial forage was harvested and weighed with a Carter Forage Harvester (Brookston, IN) fitted with scales in one 0.914-m x 15.2-m strips. Dry matter yields were calculated with an approximate two-pound subsample of the harvested material from each strip was collected, weighed, dried and reweighed.
Forage quality was analyzed using the FOSS NIRS (near infrared reflectance spectroscopy) DS2500 Feed and Forage analyzer. Dried and coarsely-ground plot samples were brought to the UVM’s Cereal Grain Testing Laboratory where they were reground using a cyclone sample mill (1 mm screen) from the UDY Corporation. The samples were then analyzed using the FOSS NIRS DS2500 for crude protein (CP), neutral detergent fiber (NDF), and neutral detergent fiber digestibility in 30 h (NDFD30).
2.1.4 Weather data
Monthly total precipitation records for 2012 through 2021 from the weather station in Burlington Vermont, United States were obtained from the NOAA National Weather Service. Precipitation levels for months prior to sampling were summed and plotted to assess relationships with soil health measurements.
2.1.5 Interpretation as ecosystem services
Ecosystem services provide a framework for assessing sustainability of socially relevant ecological outcomes and processes. It is recommended that indicators of ecosystem services be easily measured, sensitive to changes in the system and capture the connection between biophysical changes and socially relevant outcomes (Dale and Polasky, 2007; Olander et al., 2018). In this study we drew from prior work by Neher et al. (2022), Dube et al., 2022, White A. et al. (2021) and White A. C. et al. (2021) to interpret benefit-relevant soil health parameters as ecosystem services. Supporting ecosystem services are defined as those that underpin other ecosystem functions and services (Dominati, 2013). Measures of respiration and active carbon directly reflect the microbial, metabolic, and nutrient cycling activity in the soil that underpin other soil functions and ecosystem services (Meobius-Clune et al., 2016), and are thus interpreted as indicators of soil health supporting ecosystem services. Regulating ecosystem services are the benefits obtained from regulation of ecosystem processes, including climate regulation, water regulation, erosion control, and more (Leemans and De Groot, 2003). In our study, aggregate stability was measured using a simulated rainfall-based test (Meobius-Clune et al., 2016), and is interpreted as a direct indicator of soil conservation and resilience to extreme precipitation (White A. et al., 2021). Soil organic matter content is a direct measure of the dynamic portion of soil carbon content, and net changes in organic carbon content over time is interpreted as an indicator of climate regulation services through carbon storage and sequestration (Dube et al., 2022). Available water capacity is a direct measure of plant available water in soil and was interpreted as an indicator of drought resilience (White A. et al., 2021). Finally, we interpret both yield and forage quality as indicators of impacts of food provisioning services. Identifying ecosystem services in this way is a useful lens to highlight the socially relevant aspects of natural resources and ecosystem functions. In the presentation of results, we bring focus to significant influences and directionality of impact on ecosystem services as in White A. et al. (2021).
2.2 Statistical analysis
We used linear mixed models with repeated measures to evaluate the influence of time and treatment on soil health and yields. This analysis was followed by ANOVA and prediction of treatment means to support our interpretation, inference and conclusions (Gezan and Carvalho, 2018). In addition to evaluating a dependent variable of each metric as observed, we also detrended the data by calculating the ‘deviation from continuous corn’ for each observation, taking the difference between that observation and the mean value for the continuous corn plots in that year. This removed the effects of interannual variability and allowed our analysis to focus on the way the treatments differ from conventional practices (continuous corn). For example, when analyzing aggregate stability, we ran our statistical analysis methods on aggregate stability as measured, as well as the deviation in aggregate stability from continuous corn each year.
2.2.1 Model specifications
We fit linear mixed models in R (Rstudio Team, 2022) using the lme4 package (Bates et al., 2015) to identify the influence of time and treatment on soil health characteristics, yields and forage quality. Treatment, year and block were considered fixed effects. Plot was considered a random effect. Interaction terms between year and treatment, block and plot were considered. The model was refined to include only interactions between year and treatment, by stepwise removal of non-significant terms, to optimize Akaike’s Information Criterion (AIC), resulting in a best fit model as follows:
Where Yr is the year, Trt is the treatment, Trt xYr is the interaction between year and treatment, Blk is the block, and Pl is the plot as a random effect. ANOVA (Type II Wald F tests with Kenward-Roger df) was then conducted on model results using the car package in R (Fox and Weisberg, 2019). Effects were considered significant at a level of p = 0.05. Where significant impacts among treatments were identified, comparisons of means were conducted.
2.2.2 Post hoc tests
To support interpretation of the mixed model analysis, we conducted mean comparison tests where significant influences were identified. For variables with significant time:treatment interactions we ran Holm corrected pairwise t-test comparisons with the continuous corn treatment over time. A p-level of 0.05 was used to determine significant differences between treatments. These post hoc tests are considered secondary and supplemental to the model-based analysis.
3 Results
3.1 Summary
Our observations identified significant temporal, treatment and time:treatment interactions that influenced soil and yield characteristics. Treatments evaluated in our study significantly influenced soil health characteristics, total dry matter yield, and CP and NDF in comparison to continuous corn, but did not significantly influence corn yields, NDF30 or soil available water capacity (Table 2; Table 3). In treatments which rotated corn with perennial forages, overall DM yield was less than in continuous corn, but only significantly less in the ROTC2020 treatment (Table 4). Significant time:treatment interactions indicate that the impact of corn cropping systems on soil health and yield are complex, dynamic, and can be variably affected by management practices. Details of the temporal influence on significant relationships among treatments is supported by secondary analysis in Tables 5 and Table 6.
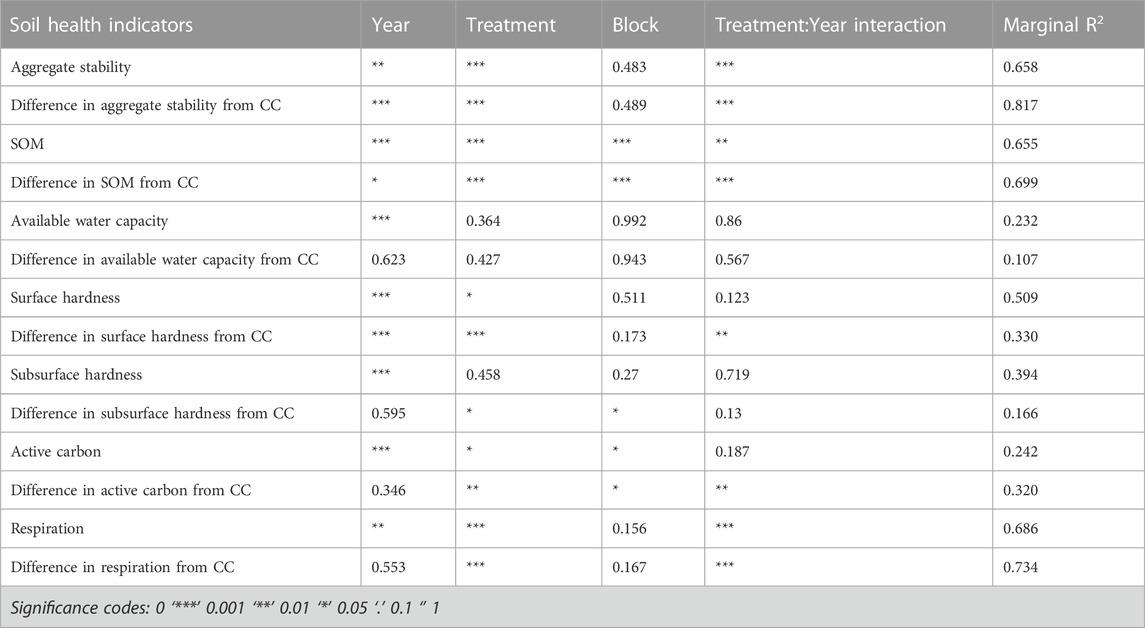
TABLE 2. Significant influences on soil health parameters; ANOVA p-values and Marginal R2 values of repeated measures linear mixed models of soil health parameters, and the deviance of soil health parameters from continuous corn (CC).
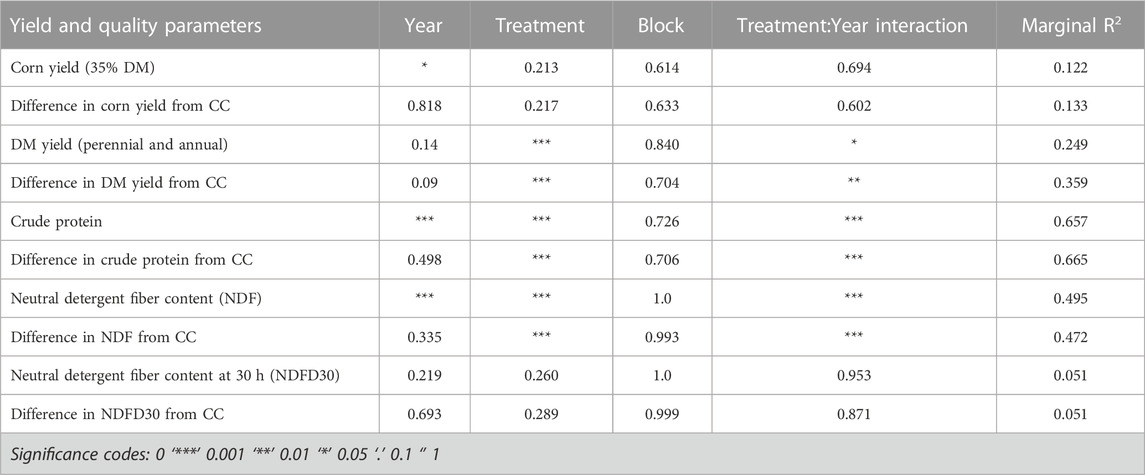
TABLE 3. Significant influences on yields and forage quality; ANOVA p-values and Marginal R2 values of repeated measures linear mixed models of corn yield (35% DM), dry matter yield for corn and forage, and forage quality, as well as the deviance in those metrics from continuous corn (CC).
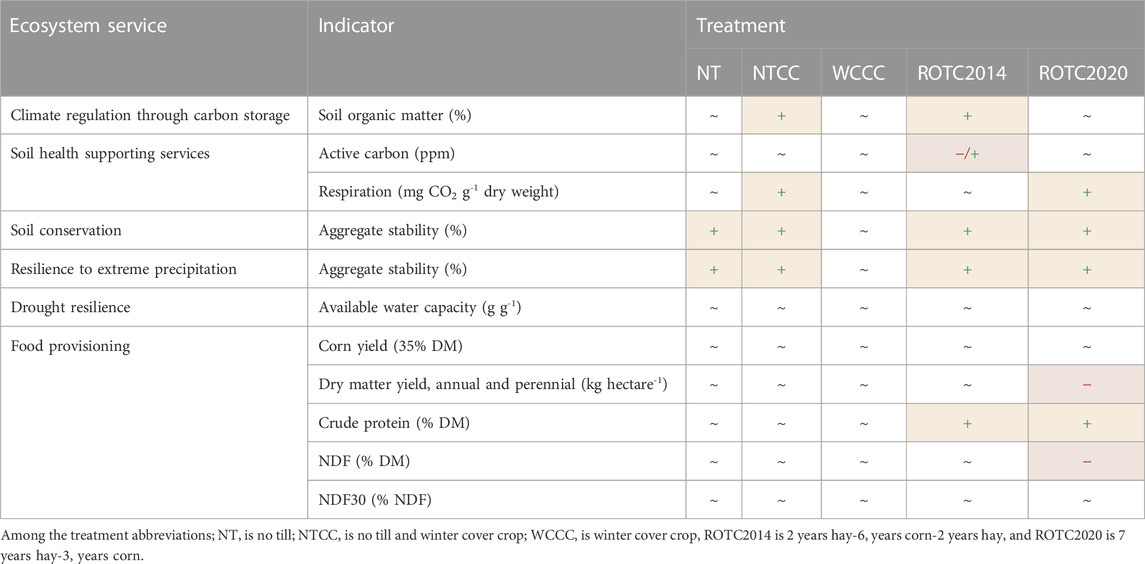
TABLE 4. Significant influences on ecosystem service provisioning by treatment compared to continuous corn (CC). This is based on the repeated measure mixed model that incorporates that influences of treatments, time and time:treatment interactions from 2012 to 2021. The “−” symbol is a negative impact, the “+” symbol is a positive impact, the “∼” symbol indicates is is not significant, and “ −/+” indicates a variable impact depending on year.
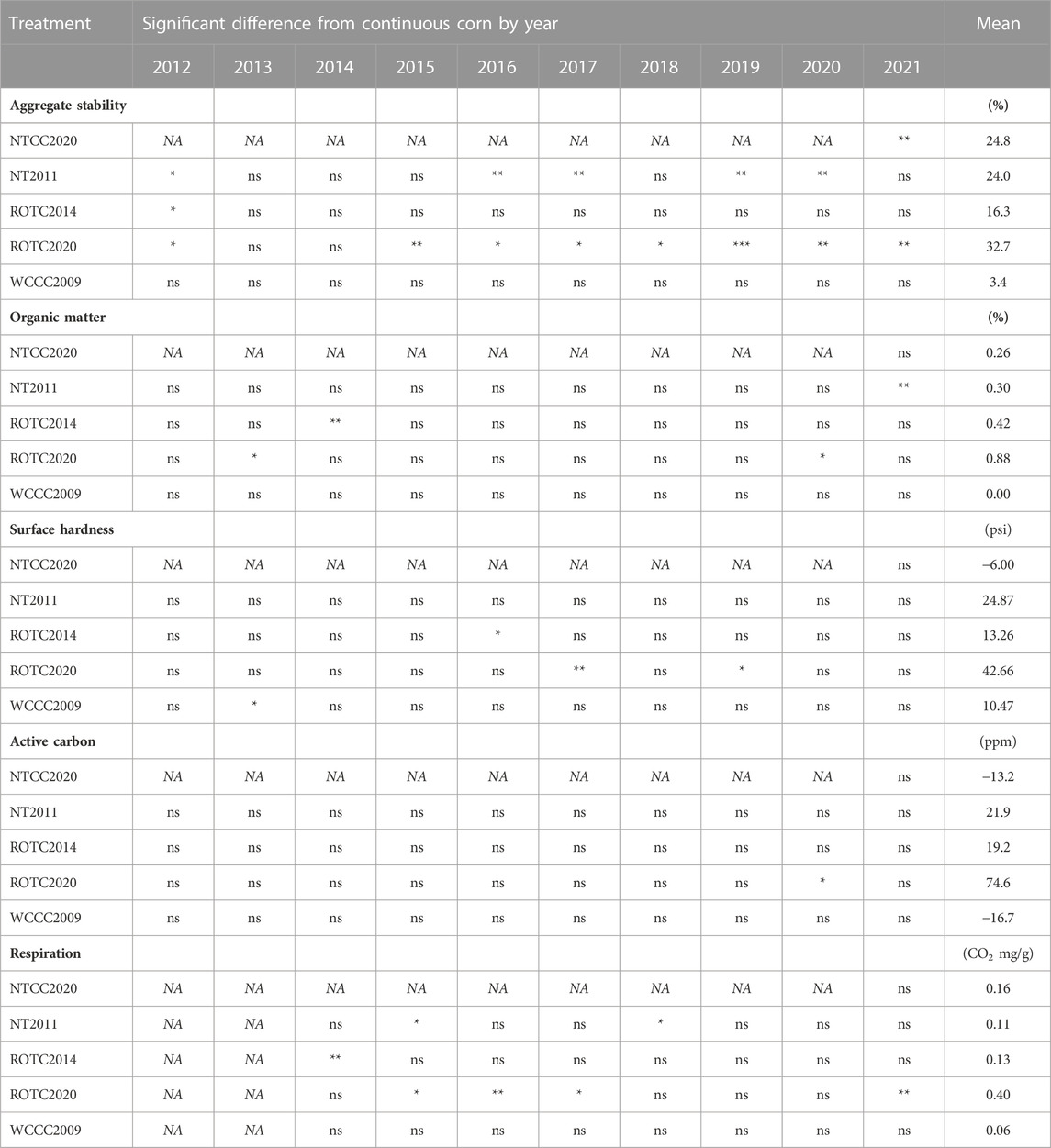
TABLE 5. Significance level of post hoc Holm corrected pairwise t-test comparisons with continuous corn treatment over time for soil variables with significant time:treatment interactions. NA is no data for that year, ns is not significant, * is significant to 0.05, ** is significant to 0.01, and *** is significant to 0.001 or less. Mean values over the entire timeframe are in the farm right column.
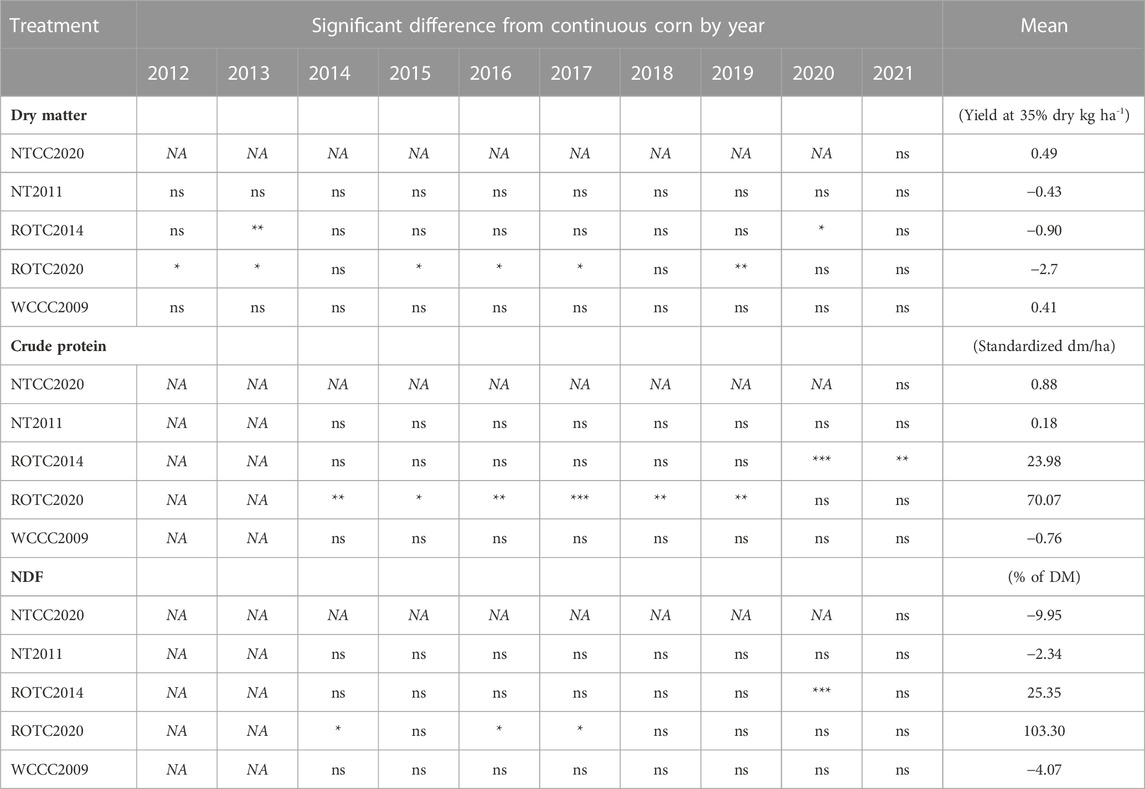
TABLE 6. Significance level of post hoc Holm corrected pairwise t-test comparisons with continuous corn treatment over time for yield variables with significant time:treatment interactions. NA is no data for that year, ns is not significant, * is significant to 0.05, ** is significant to 0.01, and *** is significant to 0.001 or less. Mean values over the entire timeframe are in the farm right column.
3.2 Soil health
3.2.1 Aggregate stability
Aggregate stability was significantly influenced by time (p < 0.001), treatments (p < 0.001), and interactions between time and treatments (p < 0.001) (Table 2). Model outputs indicate deviation in aggregate stability from CC was significantly increased by NTCC (p < 0.001), NT (p = 0.034), ROTC 2014 (p < 0.001), and ROTC 2020 (p < 0.001), but not the WCCC treatment. Significant interactions between time and NT, ROTC 2014, and ROT2020 treatments is reflected in the model output (Supplementary Material S3). The difference in aggregate stability between the ROTC2014 and CC was 23% at the start of the study (2012), and the difference declined after the ROTC2014 rotated into corn in 2014, getting to 9.43% in 2020 (Figure 1, Supplementary Material S2). In the ROTC 2020, aggregate stability relative to CC increased over time, starting at 19.7% more aggregate stability in 2012, and ending at 45.7% more than continuous corn in 2014.
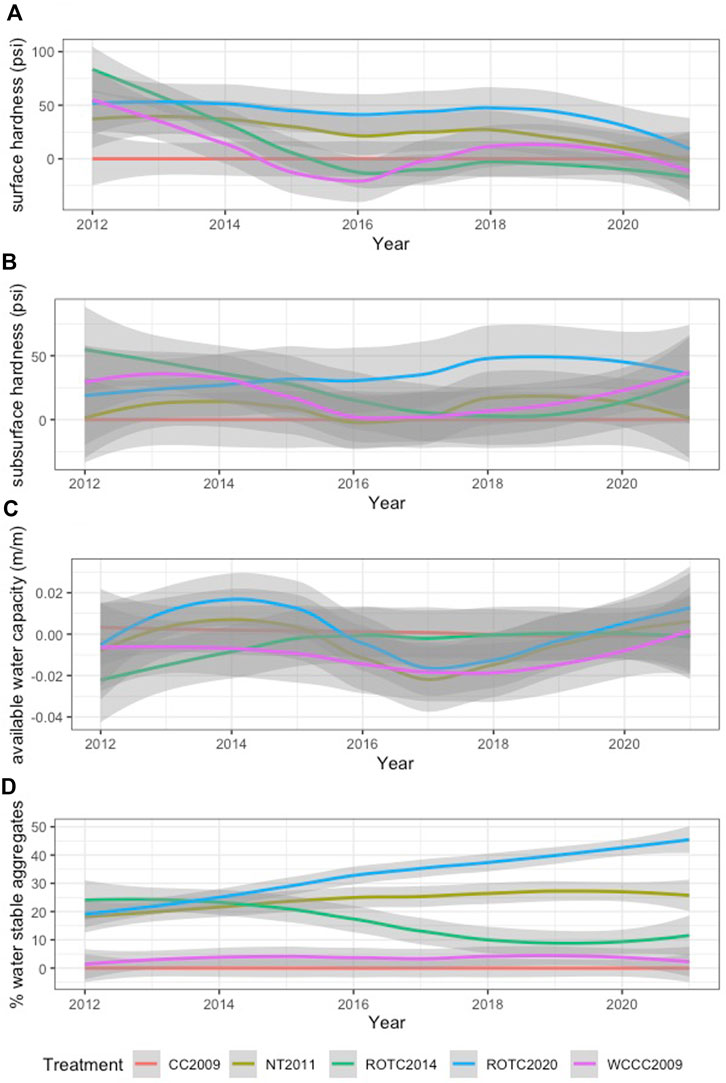
FIGURE 1. Loess plots (locally weighted smoothing) show deviation in soil physical characteristics from continuous corn by treatment from 2012 to 2021: (A) Surface hardness (B) Subsurface hardness (C) Available water capacity (D) Aggregate stability.
3.2.2 Available water capacity
Treatments had no effect on available water capacity. Available water capacity was significantly influenced only by year (p < 0.001) across the study, but the deviation in available water capacity from continuous corn was not significantly influenced by time. Block (p < 0.05) was the only significant influence on the way AWC deviated from continuous corn.
3.2.3 Surface and subsurface hardness
Subsurface hardness was significantly influenced by year (p < 0.001) only, yet the detrended data shows that the deviation in subsurface hardness from that of continuous corn was significantly influenced by treatment (p < 0.001), and block (p < 0.01). The deviation in surface hardness from that of continuous corn was significantly influenced by year (p < 0.001), treatment (p < 0.001), and an interaction between year and treatment (p < 0.001) (Table 2). Deviation in surface hardness from continuous corn was significantly influenced by the ROTC2014 treatment (p < 0.001) and the WCCC treatment (p = 0.045). Significant interactions between time and the ROTC2014 and WCCC treatments were also observed (Supplementary Material S3). In the ROTC2014 treatment, surface hardness was 69 psi greater than continuous corn treatment at the start of the data collection when the rotation was in perennial forages. Following the rotation into corn, surface hardness in the ROTC2014 treatment became more similar to surface hardness in the continuous corn plot. The average difference from continuous corn was 40.6 psi in 2014, 8.12 psi in 2015, and -36 psi in 2016 (Figure 1, Supplementary Material S2). The WCCC treatment experienced a similar trend (Figure 1), starting at 20.5 psi greater than continuous corn in 2012, dropping to 27.75 psi less than continuous corn in 2016, and ending at 12 psi less than continuous corn in 2021. These trends track with the antecedent moisture conditions during sampling time plotted, alongside surface hardness in Figure 2.
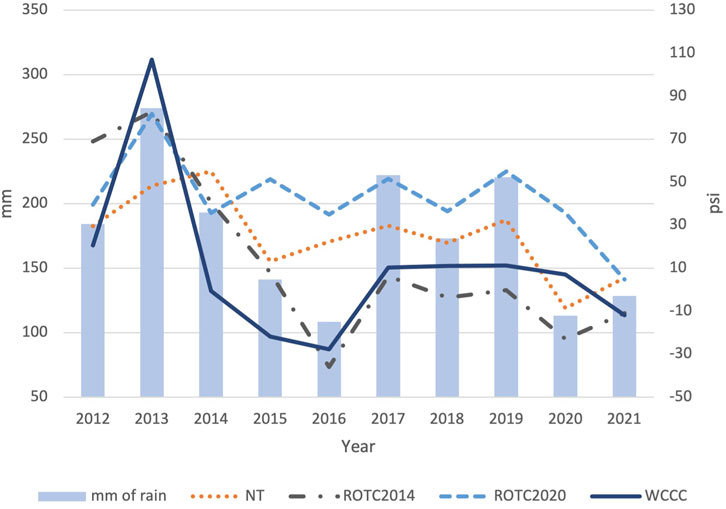
FIGURE 2. Antecedent moisture condition during spring soil sample collection and surface hardness measurements by year. Antecedent moisture condition is total precipitation in April and May months for each year recorded at Burlington International Airport weather station in VT, United States.
3.2.4 Organic matter
Soil organic matter was significantly influenced by time (p < 0.01), treatment (p < 0.001), block (p < 0.001) and interactions between time and treatment (p < 0.001) (Table 2). Model outputs indicate that the deviation in organic matter content from CC was significantly increased by the NTCC treatment (p = 0.019) and the ROTC2014 treatment (p < 0.001). A significant interaction between time and the ROTC2014 treatment was observed (Supplementary Material S3). In 2021, the NTCC treatment had 0.26% more organic matter than the CC treatment (Supplementary Material S2). In 2013, the ROTC2014 treatment had 1.26% more soil organic matter than the CC treatment, and this difference declined annually after it was transitioned to corn in 2014, and 6 years later, in 2019, the ROTC2014 treatment had only 0.19% more organic matter. Following return to perennial forages, the organic matter levels increased slightly to 0.26% greater than the CC treatment (Figure 3, Supplementary Material S2). Organic matter levels were highest in ROTC2020 in all years, but not captured as significant in the model.
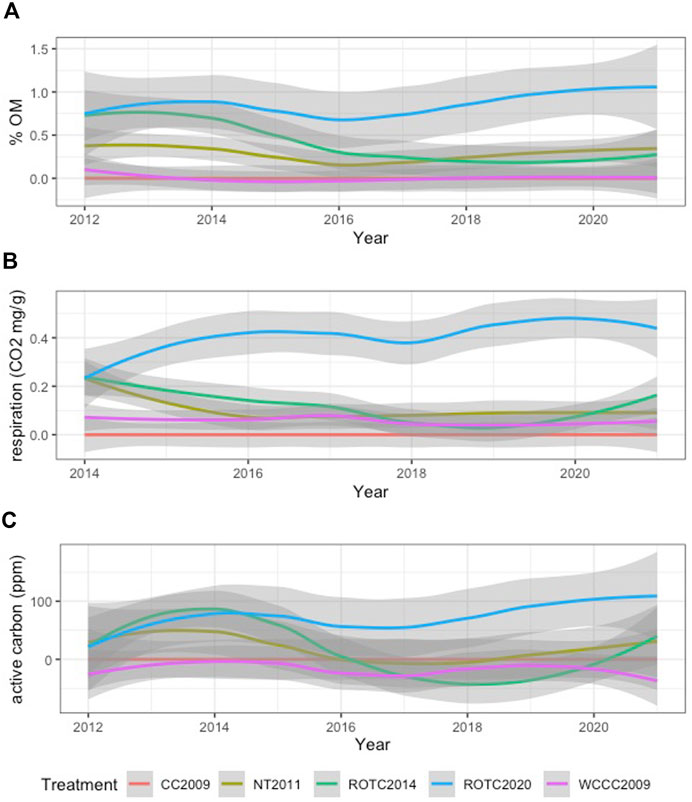
FIGURE 3. Loess plots (locally weighted smoothing) show deviation in soil biological parameters from continuous corn by treatment from 2012 to 2021: (A) Soil organic matter content (B) Respiration (C) Active carbon.
3.2.5 Active carbon
Active carbon was significantly influenced by year (p < 0.001), treatment (p < 0.001), and block (p < 0.001) (Table 2). The deviation in active carbon from that of CC was significantly influenced by treatment (p < 0.001) and the interaction of treatment and year (p < 0.001). Deviation in active carbon from CC was significantly influenced by the ROTC2014 treatment, and the interaction of time with the ROTC2014 treatment (Supplementary Material S3). Our results indicate that active carbon levels were both positively and negatively influenced by the rotation. Active carbon levels were 118 ppm greater in ROTC2014 than CC in 2013, and this difference was reduced over time when the rotation was planted with corn. In 2020, the ROTC2014 treatment had 5.36 ppm active carbon less than the CC treatment, and after it was rotated into perennial forage again the active carbon levels increased to 40 ppm greater than the CC treatment (Figure 3, Supplementary Material S2).
3.2.6 Respiration
Respiration was significantly influenced by year (p < 0.01), treatment (p < 0.001), and an interaction between treatment and time (p < 0.001). The difference in respiration between treatments and CC was influenced by treatment (p < 0.001) and an interaction between treatment and time (p < 0.001) (Table 2). The deviation from CC in respiration was significantly increased by the NTCC and ROTC2020 treatments, and the interaction of time with the ROTC2020 treatment (Supplementary Material S3).
3.3 Yields and forage quality
3.3.1 Corn yield
Corn yields were significantly influenced only by year (p < 0.01) (Table 3). The difference in yield from continuous corn was not significantly influenced by any treatment. This was not calculated for rotation treatments in years when they did not harvest corn, so overall dry matter yields (next section) are a more appropriate comparison.
3.3.2 Dry matter yields
The difference in dry matter (DM) yield from continuous corn was significantly influenced by treatment (p < 0.001) and the interaction of treatments over time (p < 0.001) (Table 3). The model results indicate that only the ROTC2020 treatment significantly influenced the difference in DM yields from continuous corn (Supplementary Material S3). While in perennial forage, the ROTC2020 treatment yielded between 1,793 kg ha-1 and 11,591 kg ha-1 less than the continuous corn treatment, and was significantly less in most years (Table 6). In 2020, the year that rotation returned to corn, it yielded 314 kg ha-1 of dry matter more than the CC treatment. The DM yield differentials were greatest in years when the rotation was planted with perennial forages (Figure 4). Cumulative DM yields over the 10 years of this study were greatest in the WCCC treatment, followed by CC, then NT, ROTC 2014, and then ROTC 2020 (Figure 5).
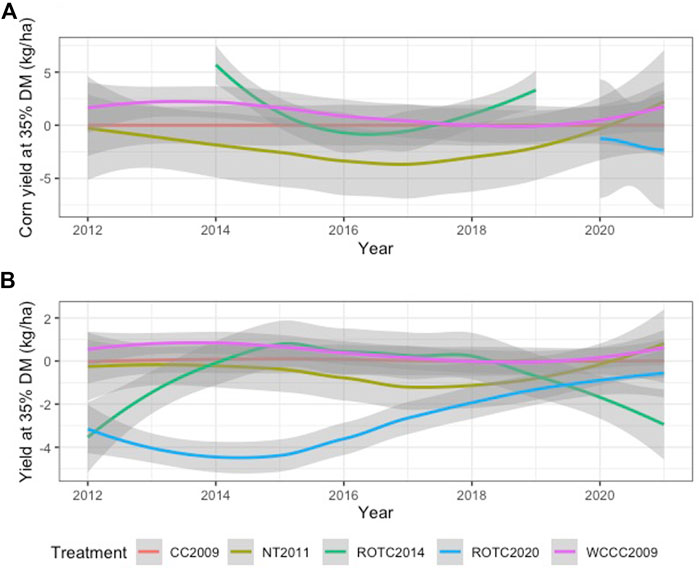
FIGURE 4. Loess plots (locally weighted smoothing) show deviation in (A) corn yield and (B) dry matter from continuous corn by treatment from 2012 to 2021.
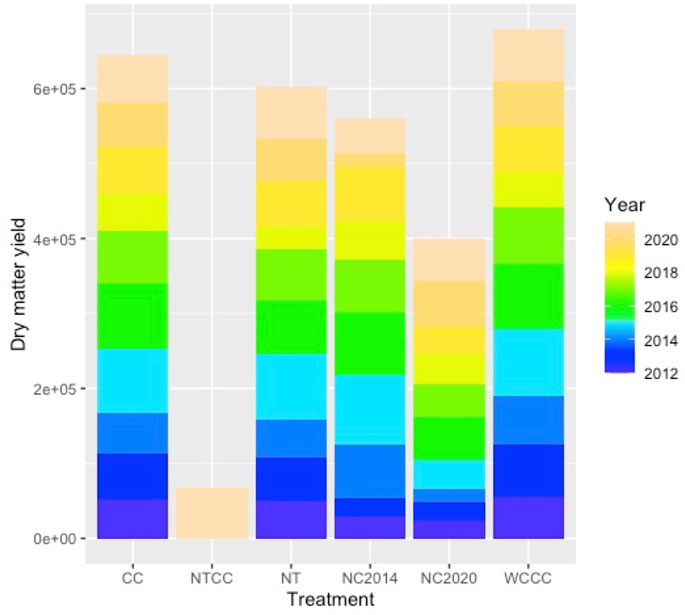
FIGURE 5. Total dry matter yields over the duration of the study by treatment, including dry matter in perennial forage and corn yields.
3.3.3 Crude protein
Treatments and the interaction of treatments with time significantly influence the deviation of CP from CC (p < 0.001) (Table 3). Model results indicate that the ROTC2014 and ROTC2020 treatments significantly influenced differences in CP from continuous corn. In years when perennial forage was harvested from these treatments, CP levels were significantly greater, at levels between 63 and 122 g kg-1 more than CC treatment means (Figure 6; Table 6, Supplementary material S2).
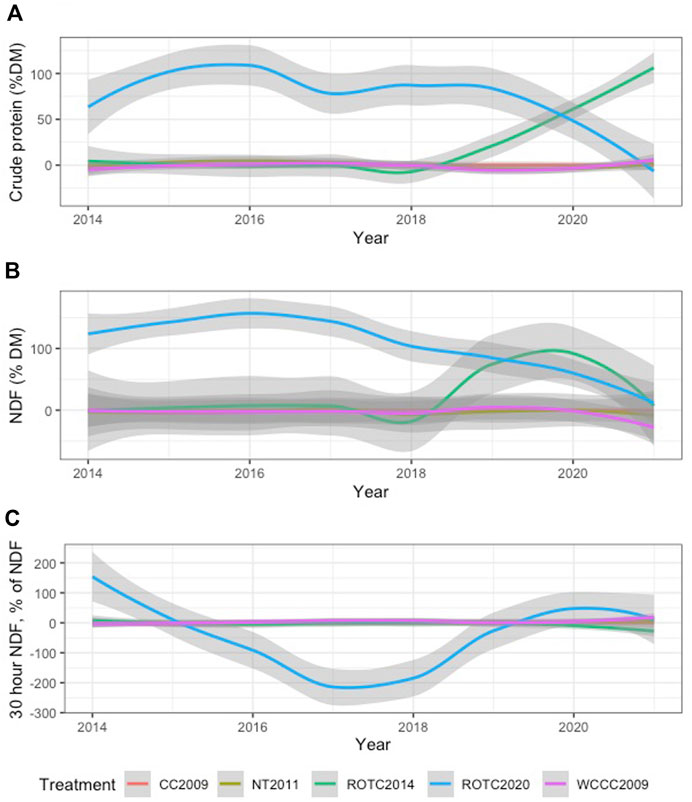
FIGURE 6. Loess plots (locally weighted smoothing) show deviation in forage quality parameters from continuous corn by treatment from 2012 to 2021: (A) Crude protein (B) NDF (C) NDF30.
3.3.4 NDF
The deviation from continuous corn in NDF concentrations was significantly influenced by treatments and the interaction of treatments with time (p < 0.001) (Table 3). Model results indicate the ROTC2020 treatment significantly influenced differences in NDF. The ROTC2020 had higher NDF values indicating that it was lower quality and could potentially limit dry matter intake of livestock. Post hoc comparison of means show the ROTC2020 treatment was significantly higher in 2014, 2016 and 2017, years when it was in hay (Table 6). Similarly, the ROTC2014 was significantly higher in 2020, a year when it was planted in hay (Table 6).
3.3.5 NDF30
Significant influences on NDFD30were not observed in this study (Table 3).
3.4 Ecosystem services
Here we interpret each treatment’s influence on ecosystem service provisioning through the indicators monitored in this study (Table 4). The NT treatment was associated with significant improvements in aggregate stability compared to continuous corn, and therefore enhanced soil conservation and resilience to extreme precipitation ecosystem services relative to continuous corn. The WCCC treatment was not significantly associated with any changes in ecosystem services provisioning in this experiment. The NTCC treatment enhanced climate regulation through carbon storage, soil health, soil conservation and resilience to extreme precipitation ecosystem services through increases in soil organic matter, respiration and aggregate stability relative to continuous corn.
The two rotation treatments also influenced indicators of food provisioning. The ROTC2014 treatment increased soil organic matter, aggregate stability and crude protein in comparison to continuous corn but reduced active carbon. This means that the ROTC2014 treatment enhanced climate regulation through carbon storage, soil conservation, resilience to extreme precipitation, and food provisioning ecosystem services, but reduced one aspect of soil health supporting ecosystem services. The ROTC2020 treatment enhanced respiration, aggregate stability, crude protein and NDF relative to continuous corn, but reduced DM yields. This means that the ROTC2020 treatment enhanced soil health, soil conservation, and resilience to extreme precipitation, but had mixed impacts on indicators of food provisioning, where protein content was enhanced but overall DM yields and digestibility was reduced.
4 Discussion
We used a mixed model analysis of variance approach to evaluate the influence of conservation practices and perennial forage rotations on soil health indicators, yields and forage quality over 10 years. Our analysis method centers on the way alternative practices deviate from the performance of continuous corn, and our results indicate that conservation practices and rotations can be implemented to sustain yields while also enhancing aspects of soil health in temperate northern climates similar to Vermont, United States. This is evidence that corn cropping systems can enhance climate regulation, climate resilience, soil health, soil conservation, and food provisioning services through alternative management to continuous corn, however careful consideration of rotation timings and practices in combination is necessary to achieve this potential. The quantification of these benefits as ecosystem services is valuable to informing the effectiveness and impact of conservation programs. Importantly, in the absence of significant increases in yields over conventional management of continuous corn silage, the benefits of the management practices we evaluated accrue primarily to the environment and society, not the farm. Although farmers in Vermont have a strong stewardship ethic towards soil conservation and ecosystem services, their capability to prioritize and invest in these broader public benefits is limited by their financial capacity (White et al., 2022a). Thus, our research implies that conservation incentive and cost-share programs are critical to enabling farms to incur the additional expenses associated with adoption of these identified practices that provide ecosystem services to public beneficiaries. Foremost, our research suggests that no-till in combination with cover cropping in corn silage fields, and a rotation of 4 years of hay to 6 years of corn are likely to achieve the greatest overall benefits in forage production systems.
4.1 Influence of treatments on dimensions of soil health
In many ways, our results confirm that conservation practices and rotations can enhance some aspects of soil health (i.e., Bottinelli et al., 2017; Sharma et al., 2012; Sharma et al., 2018; VandenBygaart, et al., 2003; Wulanningtyas et al., 2021; Nunes et al., 2018). These soil health enhancements are often associated with sustaining corn yields (Kane, et al., 2021) and forage quality. However, various meta-studies examine research that indicates neutral or negative impacts of conservation practices on soil health, crop yield, or crop quality are possible, and that these outcomes may be influenced by weather, soil type, and other management practices (Marcillo and Miguez, 2017; Lu, 2020; Miner, et al., 2020). Our study joins the growing body of research detailing complexity and tradeoffs associated with the outcomes of conservation practices and the multifaceted reality of soil health. Within the physical soil health characteristics, aggregate stability was positively influenced by some of the conservation practices and lowest in the continuous corn treatment, whereas surface and subsurface hardness were best in the continuous corn treatment and no significant impact on available water capacity was observed.
Surface hardness was significantly influenced by year, treatment and a year:treatment interaction in our study. Mean surface hardness across the 10-year study was between 10 and 42 psi greater in the no-till, rotation and cover crop treatments than continuous corn (Table 5). Although we expected to see improvements in surface hardness from tillage reduction and surface cover, this pattern likely reflects the effect of annual tillage in the continuous corn plots, which loosens the top layer of soil. Our study evaluates corn silage forage systems, which removes all aboveground plant biomass during harvest, leaving little crop residue post-harvest. Although some treatments eliminate tillage, there is no added organic matter to help protect the top layer of soil. If there were manure additions or crop residues, those might provide protection to the top layer of soil minimizing compaction from rainfall and equipment. Precipitation patterns likely also play a role in this observed pattern. We used total precipitation in months of April and May at our study site to approximate antecedent soil moisture levels and rainfall impact on the soil surface prior to sampling (Figure 2) and in years that had more spring rainfall conditions conservation treatments showed greater surface hardness, and an opposite pattern in drier spring seasons.
Increases in tillage have been shown to reduce penetrometer resistance (Mochizuki et al., 2007) and although some research posits that compaction may be alleviated by earthworms and biological processes (Yvan et al., 2012), long term research has shown reduced tillage to increase compaction without evidence of plow pan recovery after 25 years (Schlüter et al., 2018) In our study, subsurface hardness was lowest in the continuous corn treatment. Subsurface hardness was measured with a penetrometer and was highest in plots with perennial or winter roots. It is likely that the dense perennial roots, and even winter cover crop roots, provided resistance to the tool. In future research, bulk density may be a more accurate measure to capture the changes in physical soil characteristics that reflect compaction. The mean winter cover crop treatment was 20.8 psi greater than the continuous corn treatment, and the corn-hay rotation treatments had mean value of 23.5 and 34.5 psi greater than continuous corn. Although the trends for this metric were weak in our study (Figure 1) the potential compaction trade-offs associated with reduced tillage are important to consider in this region, as compaction may restrict root growth and lower yields in the long term.
Cover cropping with winter rye alone had no significant influence on any soil health metrics over a 10-year period of time when compared to continuous corn. This differs from previous research that suggests that cover crops can increase aggregate stability (Ruiz-Comenero et al., 2010). Similarly, although cover crops can supply additions of organic matter, many studies do not correlate cover crops with an increase in soil organic matter (Clark, 2010). This suggests that winter rye cover alone is not sufficient to achieving the respiration or organic matter increases expected based on other research, and highlights that variations on cover crop implementation and management are important to achieving expected benefits of cover crops.
The NT treatment significantly enhanced aggregate stability in our study by an additional 24%. Increases in aggregation have been previously linked to increases in soil biological activity and organic matter gains (i.e., Bottinelli et al., 2017; Kumar et al., 2012), but the NT treatment in our study was not significantly linked to increases in respiration, active carbon or organic matter gains. It has been strongly established that reductions in tillage, or any kind of disturbance, protects the structure of soil, the production of root exudates and the associated microbial activity that lead to aggregation, and these changes are easily detected via increased percentage of water stable aggregates (Wright et al., 1999; Kumar et al., 2012; Nouwakpo et al., 2018).
Despite the limited performance of the WCCC and NT treatments in this study, our analysis identifies the added benefits of combining cover crops with reduced tillage. A single year of no-till with cover cropping introduced at the end of our study significantly enhanced soil respiration by 0.16 CO2 mg/g, aggregate stability by 24.8%, and organic matter by 0.26% in comparison to the continuous corn treatment, suggesting that combinations of conservation practices may have synergistic effects to enhance ecosystem service provisioning without compromising yields or crop quality, as has been identified by Kinoshita, et al. (2017) and Nunes et al. (2018). Specifically, the presence of plant roots, aboveground cover crop residues and no-till management have a synergistic effect on organic matter gains and aggregate stability through the production of glomalin by arbuscular mycorrhizal fungi associated with the roots of plants (Wright et al., 1999; Kumar et al., 2012). Tillage increases microbially mediated decomposition of organic matter and carbon losses by activating respiration with increased oxygen and release of CO2. Soil aggregation also influences SOM decomposition. Aggregation physically protects SOM from microbial decomposition, and controls plant-derived SOM by occluding it into aggregates (Lagomarsino et al., 2012). In our study the measurable soil health benefits of cover cropping alone may have been negated by tillage. Conversely, the treatment with no-till alone lacked organic matter inputs or living roots and only influenced one soil health indicator.
Although reductions in tillage and disturbance can slow organic matter degradation and loss (Six and Paustian, 2014) the limited return of crop residues, and lack of manure or other organic matter inputs is likely key to explaining some of our findings across treatments. Corn crop residues and organic matter additions have been previously linked to the improvement of aggregate stability and organic matter (Nouwakpo et al., 2018). Return of crop residues is also linked to increases in soil organic matter content in no-till systems (Wang et al., 2020). Meta-analysis has linked crop residues to a 5% increase in yields (Lu, 2020). Due to the limited crop residue return in corn silage systems, the incorporation of cover crops or organic matter inputs to no-till systems is crucial to achieving the soil organic matter, carbon storage, and yield enhancements associated with long term no-till in other studies.
In our study a corn-hay rotation, with 2 years hay-6 years corn −2 years in hay (ROTC 2014), had significantly higher organic matter, and aggregate stability compared to CC in all years of the study. Enhanced aggregate stability and organic matter observed in perennial hay and corn rotation treatments align with established mechanistic understandings of soil qualities. Perennial crops have deeper, longer and stronger root systems than annual crops, which improves aggregate stability and can address compaction (Franzluebbers et al., 2000). The continuous supply of root exudates and root biomass in perennial systems that feeds biological activity in the soil, provides added organic matter and improves aeration and enhances nutrient cycling (Kumar et al., 2017).
The overall trend in organic matter in our study suggests that the reduction of organic matter decomposition through reduced tillage has a primary influence on organic matter gains in our study. Our study observed the WCCC treatment had a 10-year average of 0.0% additional organic matter, followed by the single year of NTCC with 0.26% more, and NT with 0.30% more. The treatment with perennial hay in rotation had the highest levels of organic matter. ROTC2020 had a 10-year average of 0.88% more, and ROTC2014 had 0.42% more. Organic carbon additions via roots and aboveground biomass are important to make greater gains, and align with aforementioned mechanisms of the synergistic influence between reduced disturbance and carbon additions to feed soil biology while also slowing losses (Six and Paustian, 2014; Kumar et al., 2017; Wang et al., 2020; Nunes et al., 2018).
Active carbon measures in the final year and across the 10-year average reflect the same pattern (Supplementary Material S2), with ROTC2020 having the highest level, then ROTC 2014, NT, NTCC, and WCCC with the lowest, although active carbon was only significantly influenced by the ROTC2014 treatment and the interaction of time with the ROTC2014 treatment. The model outputs, figure and mean detrended data over time indicate the effect of the treatment was variable over time, reflecting the rotation (Figure 3, Supplementary Material S2, Supplementary Material S3). Active carbon levels were 118 ppm greater in ROTC2014 than CC in 2013, and this difference was reduced over time when the rotation was planted with corn. In 2020, the ROTC2014 treatment had 5.36 ppm active carbon less than the CC treatment, and after it was rotated into perennial forage again the active carbon levels increased to 40 ppm greater than the CC treatment (Figure 3, Supplementary Material S2). Active carbon is a measure of biologically active soil carbon which is more sensitive to management effects than total organic carbon and is closely related to other measures of biological activity and organic carbon (Weil et al., 2003). Here, it illustrates that perennial hay has higher levels of biologically active carbon than continuous corn. When perennial grasses are rotated into corn there is legacy active carbon from the perennial grass plot which lasts approximately 2 years before reaching a similar level to continuous corn (Figure 3, Supplementary Material S2).
Our study found that available water capacity was only influenced by year. No-till, cover cropping, and even rotations with perennial grasses in corn silage forage production systems over a period of 10 years had no influence on the soil’s capacity to infer drought resilience. This is likely due to the limited organic matter returns in these systems. Additions of manure, other organic matter sources, or higher biomass cover cropping could address this aspect of these annual forage productions systems and deserves more research.
4.2 Influence of treatments on yields and forage quality
The treatments NT, NTCC, WCCC, and ROTC2014 treatments evaluated in our study showed neither significant increase or decrease in overall dry matter yields. The long hay rotation, ROTC 2020, had significantly reduced yields compared to continuous corn. Perennial forages in rotation with corn may enhance ecosystem services and crop quality, but the length of time in rotation influences dry matter yields. Total dry matter yields were significantly reduced in the ROTC2020 treatment, with a 10-year average of 2.7 kg ha-1 less than continuous corn but were not significantly less over the 10-year time frame in the ROTC2014 treatment. Our evidence suggests that shorter rotations of perennial forages (4 years of hay, 6 years of corn) can sustain dry matter yields that are not significantly different from continuous corn over a 10-year time frame, but longer perennial forage rotations (8 years of hay, 2 years of corn) will significantly reduce overall dry matter yields over the 10-year time frame. Optimizing rotation durations for a balance of yields and ecosystem services is possible, and further research to optimize rotation length requires more inquiry and long-term research.
This ROTC2014 rotation did not significantly influence dry matter yields over the 10-year period when compared to CC treatments but had significantly higher CP concentrations. The ROTC2014 had a 10-year mean of 24.0% greater CP content than CC. At the annual level, significant differences were observed for both ROTC treatments in years which they were in hay (Table 6). In general, CP tends to be higher in cool-season grasses like meadow fescue than warm-season grasses like corn (Ball et al., 2001). The addition of legume in a perennial forage crop also increases CP and alfalfa is comparatively high in protein (Aponte et al., 2019). According to Capstaff and Miller (2018), “…alfalfa is the highest-yielding perennial forage legume and produces more protein per unit area than other forage legumes.” This suggests that dairy farms in northern temperate climates could transition from continuous corn to corn-hay rotations without compromising overall yield and would improve forage quality, and at the same time enhance the soil’s resilience to extreme precipitation events and storage of carbon. Species composition of hay plantings influences CP levels and should be researched in combination with hay-corn rotations to optimize forage system yields and quality. Rotations can help to diversify forage quality to meet the overall needs of livestock. Farmers seek to balance a feed ration that has significant energy and protein to maximize milk production and quality components. Protein and digestible fiber are produced by growing cool-season grass and legumes mixes (Ball et al., 2001; Aponte et al., 2019). Energy is produced by growing the starch found in corn silage. Additional grain is imported onto the farm to balance any nutritional shortfalls. As expected, rotations with perennial forage increased overall CP concentrations. Although perennial forage can be high in protein, an important component necessary for herd health, milk production, and quality milk, and has comparatively healthier soil and provisioning of associated ecosystem services, due to its lower yields and the need to grow energy, corn acres can take priority especially on prime agricultural land. This has implications for farm systems that move from continuous corn to perennial forage and expansion of land or conversion of land to agricultural production. Thus, optimizing rotations that sustain yields while increasing forage quality can reduce farm inputs and overall landscape footprint.
4.3 Future research needs
The results of our research provide rich fodder for future research on the sustainability of silage corn cropping management. Foremost, our study reflected typical management practice implementation for dairy farmers in the region of study, except that manure applications were not incorporated in the study. This allowed our research to focus on the impact of the practices of interest, but similar research that includes manure additions is needed, as manures are likely to influence soil health parameters though organic matter additions, as well as yields and crop quality through nutrient availability. Second, the limited impact of cover cropping identified in this study suggests that research on modifications to cover cropping implementation in these corn silage production systems is needed in order to establish practice standards among the farming community that will have both environmental and farm benefits. Alternative styles of cover cropping with greater species diversity, biomass, establishment dates or termination methods may enhance ecosystem services and yields in these systems, but carefully executed research is needed to identify which modifications provide the desired impacts. Third, our findings imply research on combinations of conservation practices (sometimes referred to as stacking) that quantify the benefits of management systems, rather than single practices, are needed to inform farmers, and conservation incentive program priorities.
Our research identified a corn-hay rotation that enhanced ecosystem services and sustained yields, but further research is needed to explore the optimization of rotation timings. This kind of research could explore optimization of rotations for yields while still enhancing ecosystem services, or optimization of rotations for ecosystem services that do not reduce yields. For example, there may be a “sweet spot” of a rotation with a longest possible interval of perennial forage yield which does not impact overall dry matter yields. Alternatively, there may be a low threshold for the interval of perennial forages in a rotation which prioritized yields but also sustains increases soil carbon storage over time.
Future research on corn cropping systems should also prioritize evaluation of how practices influence biological diversity, water quality, infiltration, and greenhouse gas emissions, preferably in long enough time frames to capture the temporal dynamics of rotations on outcomes of interest. As well, future research should replicate studies like ours to confirm these findings in different climates and soil types, as those factors often have a dominating influence on soil health characteristics and crop performance.
Our study identified a limited influence on yields from the cropping system adjustments in our study. If conservation practices do not result in increased yield or quality, farmers may have little incentive or financial capacity to adopt them. The costs incurred as labor, time, money, and stress to enhance ecosystem services provisioning are likely to limit adoption (White A. et al., 2021; White et al., 2022a). Incentivizing conservation practice implementation through market recognition, payment for ecosystem services program, land rental, or cost share programs is needed in light of our findings, and specific transdisciplinary research that identifies the economics costs of practices and the conservation inventive program preferences of farmers is needed to complement our findings.
5 Conclusion
Conservation practices and rotations can be implemented to sustain yields in corn silage production systems while also enhancing climate regulation, climate resilience, aspects of soil health, soil conservation and forage quality in temperate northern climates similar to Vermont, United States. However, in the absence of significant increases in yields over conventional management of continuous corn silage, conservation incentive programs are needed to enable farms to adopt these management changes that provide ecosystem services to society. Where farmers are limited in their land access and unable to accommodate perennial forage rotations on all fields, our research suggests that continuous corn silage production systems with low crop residue can be adjusted to enhance ecosystem services without compromising yields. Cover cropping with winter rye alone had no influence on ecosystem services, yields, or crop quality when compared to continuous corn. Despite the limited performance of the winter cover crop treatment in this study, our analysis identifies the added benefits of combining cover crops with reduced tillage. The inclusion of no-till management in corn production systems enhanced aggregate stability in our study, and therefore soil conservation and resilience to extreme precipitation, without compromising yields or crop quality. Thus, a combination of multiple conservation practices should be implemented together to achieve the greatest benefits, and more research that explores the long-term dynamics of practices in combination is needed. No-till in combination with cover cropping in corn silage fields, and a rotation of 4 years of hay to 6 years of corn are likely to achieve the greatest overall benefits in forage production systems. Dairy farms in northern temperate climates could transition from continuous corn to corn-hay rotations without compromising overall yield and would improve forage quality, and at the same time enhance the soil’s resilience to extreme precipitation events and storage of carbon.
Our study identifies modifications to silage corn cropping agroecosystem management which can enhance ecological benefits, without sacrificing yields and forage quality, however careful consideration of rotation timings and practices in combination is necessary to achieve this potential. Significant time:treatment interactions indicate that the impact of corn cropping systems on soil health and yield are complex, dynamic, and can be variably affected by management practices. The quantification of these benefits as ecosystem services is valuable to informing the effectiveness and impact of conservation programs, and understanding trade-offs in dimensions of soil health. This highlights the importance of long-term datasets such as this in advancing our understanding of the environmental and economic implications of alternative cropping practices.
Data availability statement
The original contributions presented in the study are included in the article/Supplementary Material, further inquiries can be directed to the corresponding author.
Author contributions
Conceptualization, AW and HD; Methodology, AW, HD, LR, and BS; Formal Analysis, AW, HD, LR, and BS; Investigation, AW, HD, LR and BS; Writing—Original Draft Preparation, AW, HD, and LR Writing—Review and Editing, AW, HD, LR, and BS; Visualization, AW; Project Administration, HD; Funding Acquisition, HD
Funding
Initial establishment and first years of this project were funded through USDA NIFA HATCH project VT-H01101IR. Additional funding was secured from a grant provided by Ben and Jerry’s Homemade, Inc.
Acknowledgments
The authors would like to thank Roger Rainville and the staff at Borderview Research Farm for their generous help with this research trial as well as Susan, Brouillette, Sarah Ziegler, Henry Blair, John Bruce, Catherine Davidson, and Ivy Krezinksi for their assistance with data collection and entry.
Conflict of interest
The authors declare that the research was conducted in the absence of any commercial or financial relationships that could be construed as a potential conflict of interest.
Publisher’s note
All claims expressed in this article are solely those of the authors and do not necessarily represent those of their affiliated organizations, or those of the publisher, the editors and the reviewers. Any product that may be evaluated in this article, or claim that may be made by its manufacturer, is not guaranteed or endorsed by the publisher.
Supplementary material
The Supplementary Material for this article can be found online at: https://www.frontiersin.org/articles/10.3389/fenvs.2023.1061013/full#supplementary-material
References
Abbott, L. K., and Manning, D. A. (2015). Soil health and related ecosystem services in organic agriculture. Sustain. Agric. Res. 4, 116–201637946. doi:10.5539/sar.v4n3p116
Adhikari, K., and Hartemink, A. E. (2016). Linking soils to ecosystem services—a global review. Geoderma 262, 101–111. doi:10.1016/j.geoderma.2015.08.009
Aponte, A., Samarappuli, D., and Berti, M. T. (2019). Alfalfa–grass mixtures in comparison to grass and alfalfa monocultures. Agron. J. 111 (2), 628–638. doi:10.2134/agronj2017.12.0753
Balík, J., Kulhánek, M., Černý, J., Sedlář, O., and Suran, P. (2020). Soil organic matter degradation in long-term maize cultivation and insufficient organic fertilization. Plants 9 (9), 1217. doi:10.3390/plants9091217
Ball, D. M., Collins, M., Lacefield, G. D., Martin, N. P., Mertens, D. A., Olson, K. E., et al. (2001). Understanding forage quality. Am. Farm Bureau Fed. Publ. 1 (01), 1–15.
Barthès, B., and Roose, E. (2017). Aggregate stability as an indicator of soil susceptibility to runoff and erosion; validation at several levels. Catena 47 (2), 133–149. doi:10.1016/S0341-8162(01)00180-1
Bates, D., Maechler, M., Bolker, B. M., and Walker, S. (2015). Fitting linear mixed-effects models using lme4. J. Stat. Softw. 67, 1–48. doi:10.18637/jss.v067.i01
Bhadha, J. H., Capasso, J. M., Khatiwada, R., Swanson, S., and LaBorde, C. (2017). Raising soil organic matter content to improve water holding capacity. Uf/Ifas 447 (1), 1–5.
Bottinelli, N., Angers, D. A., Hallaire, V., Michot, D., Le Guillou, C., Cluzeau, D., et al. (2017). Tillage and fertilization practices affect soil aggregate stability in a Humic Cambisol of Northwest France. Soil Tillage Res. 170, 14–17. doi:10.1016/j.still.2017.02.008
Broadbent, F. E. (1965). Organic matter, methods of soil analysis. Part 1, physical and mineralogical methods. Am. Soc. Agron. Monogr. 9, 1397–1400. doi:10.2134/agronmonogr9.2.c41
Capstaff, N. M., and Miller, A. J. (2018). Improving the yield and nutritional quality of forage crops. Front. Plant Sci. 9, 535. doi:10.3389/fpls.2018.00535
Chen, G., and Weil, R. (2011). Root growth and yield of maize as affected by soil compaction and cover crops. Soil Tillage Res. 117, 17–27. doi:10.1016/j.still.2011.08.001
A. Clark (Editor) (2010). Managing cover crops profitably. Sustainable agriculture research and education (SARE) program. 3rd ed. (Beltsville, MD.
Dale, V. H., and Polasky, S. (2007). Measures of the effects of agricultural practices on ecosystem services. Ecol. Econ. 64 (2), 286–296. doi:10.1016/j.ecolecon.2007.05.009
Darby, Heather, Ruhl, Lindsey, Bruce, John, and Ziegler, Sara (2019). Annual and perennial forage system impact on soil health and corn silage yield”. in Northwest Crops & Soils Program, 336. Available at:.
De Baets, S., Poesen, J., Meersmans, J., and Serlet, L. (2011). Cover crops and their erosion-reducing effects during concentrated flow erosion. Catena 85 (3), 237–244. doi:10.1016/j.catena.2011.01.009
Dominati, E. J. (2013). Ecosystem services in New Zealand—conditions and trends. Lincoln, New Zealand: Manaaki Whenua Press, 132–142.Natural capital and ecosystem services of soils
Dube, B., White, A., Darby, H., and Ricketts, T. (2022). Vermont payment for ecosystem services technical research report #5. Burlington: University of Vermont.Valuation of soil health ecosystem services
Fox, J., and Weisberg, S. (2019). An R companion to applied regression. 3rd Edition. Thousand Oaks, CA: Sage. Available at: https://socialsciences.mcmaster.ca/jfox/Books/Companion/f
Franzluebbers, A. J., Wright, S. F., and Stuedemann, J. A. (2000). Soil aggregation and glomalin under pastures in the Southern Piedmont USA. Soil Sci. Soc. Am. J. 64 (3), 1018–1026. doi:10.2136/sssaj2000.6431018x
Garrett, R. D., Niles, M., Gil, J., Dy, P., Reis, J., and Valentim, J. (2017). Policies for reintegrating crop and livestock systems: A comparative analysis. Sustainability 9 (3), 473. doi:10.3390/su9030473
Gezan, S A., and Carvalho., Melissa (2018). Analysis of repeated measures for the biological and agricultural sciences. Appl. statistics Agric. Biol. Environ. Sci., 279–297. doi:10.2134/appliedstatistics.2016.0008.c10
Haruna, S. I., Nkongolo, N. V., Anderson, S. H., Eivazi, F., and Zaibon, S. (2018). In situ infiltration as influenced by cover crop and tillage management. J. Soil Water Conservation 73 (2), 164–172. doi:10.2489/jswc.73.2.164
IPCC (2018). Summary for policymakers of IPCC special report on global warming of 1.5°C approved by governments. The international Panel on climate change (IPCC). Available at: http://www.ipcc.ch/report/sr15/.
Kane, D. A., Bradford, M. A., Fuller, E., Odefield, E. E., and Wood, S. A. (2021). Soil organic matter protects US maize yields and lowers crop insurance payouts under drought. Environ. Res. Lett. 16 (4), 044018. Available at: https://iopscience.iop.org/article/10.1088/1748-9326/abe492/pdf (Accessed September, 2022). doi:10.1088/1748-9326/abe492
Kinoshita, R., Schindelbeck, R., and van Es, H. M. (2017). Quantitative soil profile-scale assessment of the sustainability of long-term maize residue and tillage management. Soil Tillage Res. 174, 34–44. doi:10.1016/j.still.2017.05.010
Kumar, A., Dorodnikov, M., Splettstößer, T., Kuzyakov, Y., and Pausch, J. (2017). Effects of maize roots on aggregate stability and enzyme activities in soil. Geoderma 306, 50–57. doi:10.1016/j.geoderma.2017.07.007
Kumar, S., Kadono, A., Lal, R., and Dick, W. (2012). Long-term no-till impacts on organic carbon and properties of two contrasting soils and corn yields in Ohio. Soil Sci. Soc. Am. J. 76 (5), 1798–1809. doi:10.2136/sssaj2012.0055
Kumar, S., Sieverding, H., Lai, L., Thandiwe, N., Wienhold, B., Redfearn, D., et al. (2019). Facilitating crop–livestock reintegration in the northern great plains. Agron. J. 111, 2141–2156. doi:10.2134/agronj2018.07.0441
Lagomarsino, A., Grego, S., and Kandeler, E. (2012). Soil organic carbon distribution drives microbial activity and functional diversity in particle and aggregate-size fractions. Pedobiologia 55 (2), 101–110. doi:10.1016/j.pedobi.2011.12.002
Lal, R., Follett, R. F., Kimble, J., and Cole, C. V. (1999). Managing U.S. cropland to sequester carbon in soil. J. Soil Water Conservation 54 (1), 374–381. Available at: https://www.jswconline.org/content/54/1/374.short.
Lark, T. J., Salmon, M., and Gibbs, H. (2015). Cropland expansion outpaces agricultural and biofuel policies in the United States. Environ. Res. Lett. 10, 044003. doi:10.1088/1748-9326/10/4/044003
Leemans, R., and De Groot, R. S. (2003). Millennium ecosystem assessment: Ecosystems and human well-being: A framework for assessment. Washington, DC: Island Press.
Lu, X. (2020). A meta-analysis of the effects of crop residue return on crop yields and water use efficiency. PLoS One 15 (4), e0231740. doi:10.1371/journal.pone.0231740
Marcillo, G. S., and Miguez, F. E. (2017). Corn yield response to winter cover crops: An updated meta-analysis. J. Soil Water Conservation 72 (3), 226–239. doi:10.2489/jswc.72.3.226
Miner, G. L., Delgado, J. A., Ippolito, J. A., and Stewart, C. E. (2020). Soil health management practices and crop productivity. Agric. Environ. Lett. 5 (1). doi:10.1002/ael2.20023
Mochizuki, M. J., Rangarajan, A., Bellinder, R. R., Björkman, T., and van Es, H. M. (2007). Overcoming compaction limitations on cabbage growth and yield in the transition to reduced tillage. HortScience 42 (7), 1690–1694. doi:10.21273/hortsci.42.7.1690
Moebius-Clune, B. N., Moebius-Clune, D. J., Gugino, B. K., Idowu, O. J., Schindelbeck, R. R., Ristow, A. J., et al. (2016). Comprehensive assessment of soil health – the Cornell framework. Edition 3.2. Geneva, NY: Cornell University.
Neher, D. A., Harris, J. M., Horner, C. E., Scarborough, M. J., Badireddy, A. R., Faulkner, J. W., et al. (2022). Resilient soils for resilient farms: An integrative approach to assess, promote, and value soil health for small-and medium-size farms. Phytobiomes J. 6, 201–206. doi:10.1094/pbiomes-10-21-0060-p
NOAA (2022). NOWData - NOAA online weather data. Available at: https://www.weather.gov/wrh/Climate?wfo=btv (Accessed August 17, 2022).
Nouwakpo, S. K., Song, J., and Gonzalez, J. M. (2018). Soil structural stability assessment with the fluidized bed, aggregate stability, and rainfall simulation on long-term tillage and crop rotation systems. Soil Tillage Res. 178, 65–71. doi:10.1016/j.still.2017.12.009
Nunes, M. R., van Es, H. M., Schindelbeck, R., Ristow, A. J., and Ryan, M. (2018). No-till and cropping system diversification improve soil health and crop yield. Geoderma 328, 30–43. doi:10.1016/j.geoderma.2018.04.031
Olander, L. P., Johnston, R. J., Tallis, H., Kagan, J., Maguire, L. A., Polasky, S., et al. (2018). Benefit relevant indicators: Ecosystem services measures that link ecological and social outcomes. Ecol. Indic. 85, 1262–1272. doi:10.1016/j.ecolind.2017.12.001
O’Neill, D. W., Fanning, A. L., Lamb, W. F., and Steinberger, J. K. (2018). A good life for all within planetary boundaries. Nat. Sustain. 1 (2), 88–95. doi:10.1038/s41893-018-0021-4
Page, K. L., Dang, Y. P., and Dalal, R. C. (2020). The ability of conservation agriculture to conserve soil organic carbon and the subsequent impact on soil physical, chemical, and biological properties and yield. Front. Sustain. food Syst. 4, 31. doi:10.3389/fsufs.2020.00031
Palm, C., Blanco-Canqui, H., DeClerck, F., Gatere, L., and Grace, P. (2014). Conservation agriculture and ecosystem services: An overview. Agric. Ecosyst. Environ. 187, 87–105.
PlantMaps, (2022). Interactive United States köppen climate classification map. Available at: https://www.plantmaps.com/koppen-climate-classification-map-united-states.php (Accessed August 17, 2022).
Rstudio Team (2022). Rstudio. Boston, MA: Integrated Development Environment for R. Rstudio, PBC. Available at: http://www.rstudio.com/.
Ruiz-Comenero, M., Bienes, R., and Marques, M. (2010). Effect of cover crops management in aggregate stability of a vineyard in Central Spain. Geophys. Res. Abstr. 12.
Sapkota, T. B., Mazzoncini, M., Bàrberi, P., Antichi, D., and Silvestri, N. (2012). Fifteen years of no till increase soil organic matter, microbial biomass and arthropod diversity in cover crop-based arable cropping systems. Agron. Sustain. Dev. 32, 853–863. doi:10.1007/s13593-011-0079-0
Schlüter, S., Großmann, C., Diel, J., Wu, G. M., Tischer, S., Deubel, A., et al. (2018). Long-term effects of conventional and reduced tillage on soil structure, soil ecological and soil hydraulic properties. Geoderma 332, 10–19. doi:10.1016/j.geoderma.2018.07.001
Sekaran, U., Lai, L., Ussiri, D. A., Kumar, S., and Clay, S. (2021). Role of integrated crop-livestock systems in improving agriculture production and addressing food security–A review. J. Agric. Food Res. 5, 100190. doi:10.1016/j.jafr.2021.100190
Sharma, P., Sing, G., and Sing, R. (2012). Conservation tillage and optimal water supply enhance microbial enzyme (glucosidase, urease and phosphatase) activities in fields under wheat cultivation during various nitrogen management practices. Archives Agron. Soil Sci. 59 (7), 911–928. doi:10.1080/03650340.2012.690143
Sharma, P., Sing, A., Charanjit, S. K., Brar, A. S., Grover, K. K., Dia, M., et al. (2018). The role of cover crops towards sustainable soil health and agriculture-A review paper. Am. J. Plant Sci. 9 (9), 1935–1951. doi:10.4236/ajps.2018.99140
Six, J., and Paustian, K. (2014). Aggregate-associated soil organic matter as an ecosystem property and a measurement tool. Soil Biol. Biochem. 68, A4–A9. doi:10.1016/j.soilbio.2013.06.014
Stanger, T. F., and Lauer, J. G. (2008). Corn grain yield response to crop rotation and nitrogen over 35 years. Agron. J. 100, 643–650. doi:10.2134/agronj2007.0280
Struik, P. C., and Kuyper, T. W. (2017). Sustainable intensification in agriculture: the richer shade of green. A review. Agron. Sustain. Dev. 37, 39. doi:10.1007/s13593-017-0445-7
Tomasek, B. J., Williams, M. M., and Davis, A. S. (2017). Changes in field workability and drought risk from projected climate change drive spatially variable risks in Illinois cropping systems. PLoS One 12 (2), e0172301. doi:10.1371/journal.pone.0172301
Undersander, D., and Barnett, K. (2008). Value of short rotations for alfalfa profitability. Available at: https://fyi.extension.wisc.edu/forage/value-of-short-rotations-for-alfalfa-profitability/.
United States Department of Agriculture (Usda), (2007). Census of agriculture. Washington, DC, USA: USDA.
USDA (2021). Vermont state agriculture overview. Available at: https://www.nass.usda.gov/Quick_Stats/Ag_Overview/stateOverview.php?state=VERMONT.
USDA-NRCS (2022). Soil health. Available at: https://www.nrcs.usda.gov/conservation-basics/natural-resource-concerns/soils/soil-health (Accessed January 9, 2022).
van Es, H., Scheindelbeck, R., Amsili, J., and March, K. Kurtz. (2019). Predicted available water capacity. Cornell Soil Health Manual Series, Fact Sheet Number 19-05b Available at: https://cpb-us-e1.wpmucdn.com/blogs.cornell.edu/dist/7/9922/files/2021/11/05bWater_Capacity-Predicted.pdf.
VandenBygaart, A. J., Gregorich, E. G., and Angers, E. G. (2003). Influence of agricultural management on soil organic carbon: A compendium and assessment of Canadian studies. Can. J. Soil Sci. 83 (4), 363–380. doi:10.4141/S03-009
Wall, D. H., Behan-Pelletier, V., Jones, T. H., Ritz, K., Six, J., Strong, D. R., et al. (2012). Soil ecology and ecosystem services. Oxford University Press.
Weil, R. R., Islam, K. R., Stine, M. A., Gruver, J. B., and Samson-Liebig, S. E. (2003). Estimating active carbon for soil quality assessment: A simplified method for laboratory and field use. Am. J. Altern. Agric. 18 (1), 3–17. doi:10.1079/ajaa2003003
White, A. C., Darby, H. M., Dube, B. T., Sands, B., Faulkner, J. W., Albers, M., et al. (2021b). Measuring ecosystem services from soil health. Vermont payment for ecosystem services technical research report# 1. Burlington, VT: University of Vermont.
White, A. C., Darby, H. M., Ruhl, L., and Lane, E. (2022b). The state of soil health in Vermont: Summary statistics from Vermont agriculture in 2021. Burlington, VT: University of Vermont.
White, A. C., Faulkner, J. W., Conner, D. S., Méndez, V. E., and Niles, M. T. (2022a). How can you put a price on the environment?” Farmer perspectives on stewardship and payment for ecosystem services. J. Soil Water Conservation 77 (3), 00041–00283. doi:10.2489/jswc.2022.00041
White, A., Faulkner, J. W., Conner, D., Barbieri, L., Adair, E. C., Niles, M. T., et al. (2021a). Measuring the supply of ecosystem services from alternative soil and nutrient management practices: A transdisciplinary, field-scale approach. Sustainability 13 (18), 10303. doi:10.3390/su131810303
Wright, S. F., Starr, J. L., and Paltineanu, I. C. (1999). Changes in aggregate stability and concentration of glomalin during tillage management transition. Soil Sci. Soc. Am. J. 63 (6), 1825–1829. doi:10.2136/sssaj1999.6361825x
Wulanningtyas, H., Gong, Y., Li, P., Sakagami, N., Nishiwaki, J., and Komatsuzaki, M. (2021). A cover crop and no-tillage system for enhancing soil health by increasing soil organic matter in soybean cultivation. Soil Tillage Res. 205, 104749. doi:10.1016/j.still.2020.104749
Yang, T., Siddique, K. H., and Liu, K. (2020). Cropping systems in agriculture and their impact on soil health-A review. Glob. Ecol. Conservation 23, e01118. doi:10.1016/j.gecco.2020.e01118
Yvan, C., Stéphane, S., Stéphane, C., Pierre, B., Guy, R., and Hubert, B. (2012). Role of earthworms in regenerating soil structure after compaction in reduced tillage systems. Soil Biol. Biochem. 55, 93–103. doi:10.1016/j.soilbio.2012.06.013
Keywords: corn silage, soil health, no-till, cover crop, rotation, long-term, sustainable agriculture, perennial forage crop
Citation: White A, Darby H, Ruhl L and Sands B (2023) Long term influence of alternative corn cropping practices and corn-hay rotations on soil health, yields and forage quality. Front. Environ. Sci. 11:1061013. doi: 10.3389/fenvs.2023.1061013
Received: 04 October 2022; Accepted: 13 February 2023;
Published: 27 February 2023.
Edited by:
Reshmi Sarkar, Private and indirectly affiliated with Texas A&M AgriLife Research, United StatesReviewed by:
Ashim Datta, Central Soil Salinity Research Institute (ICAR), IndiaDibakar Roy, Indian Institute of Agricultural Biotechnology (ICAR), India
Copyright © 2023 White, Darby, Ruhl and Sands. This is an open-access article distributed under the terms of the Creative Commons Attribution License (CC BY). The use, distribution or reproduction in other forums is permitted, provided the original author(s) and the copyright owner(s) are credited and that the original publication in this journal is cited, in accordance with accepted academic practice. No use, distribution or reproduction is permitted which does not comply with these terms.
*Correspondence: Heather Darby, aGVhdGhlci5kYXJieUB1dm0uZWR1